- State Key Laboratory Breeding Base of Green Pesticide and Agricultural Bioengineering, Key Laboratory of Green Pesticide and Agricultural Bioengineering, Ministry of Education, Center for R&D of Fine Chemicals of Guizhou University, Guiyang, China
Abscisic acid (ABA) is an important plant endogenous hormone that participates in the regulation of various physiological processes in plants, including the occurrence and development of somatic embryos, seeddevelopment and dormancy. ABA is called “plant stress resistance factor”, while with the limitation of the rapid metabolic inactivation and photoisomerization inactivation of ABA for its large-scale use. Understanding the function and role of ABA in plants is of great significance to promote its application. For decades, scientists have conducted in-depth research on its mechanism of action and signaling pathways, a series of progress were achieved, and hundreds of ABA analogues (similar in structure or function) have been synthesized to develop highly active plant growth regulators and tools to elucidate ABA perception. In this review, we summarize a variety of ABA analogues, especially the ABA receptor analogues, and explore the mechanisms of ABA action and catabolism, which will facilitate the development of novel ABA analogues with high biological activities.
Introduction
The environment that plants depend on for survival is constantly changing, and some adverse conditions, such as extreme temperature, drought, salinization and other abiotic stresses, can seriously affect the growth and development of plants (Young, 2007). Future climate change will lead to a gradual increase in the frequency (Guo et al., 2021). Therefore, it is important to improve the adaptability of plants to these abiotic stresses, especially drought.
Plant hormones play an important role in regulating plant adaptation to abiotic stresses (Waadt et al., 2022). Abscisic acid (ABA) synthesis is one of the fastest responses to stresses in plants (Zhao et al., 2018). ABA is a phytohormone which is named for its function in inhibiting plant growth and promoting leaf shedding. Subsequently, more and more studies show that ABA is also involved in regulating other growth and development processes of plants, such as seed germination, dormancy and stomatal closure. Moreover, ABA has been reported to control the expression of many stress-responsive genes and involve in many kinds of stress responses in plants (Hoth et al., 2002; Seki et al., 2002; Nemhauser et al., 2006).
The studies on the function and application for ABA have made remarkable achievements in the past 20 years. The synthesis, perception and transduction of ABA signals have been summarized (Young, 2007; Fidler et al., 2015; Zhang, et al., 2015), Asghar, Ma and co-workers had surveyed the advances from the functions of ABA (such as the effects on osmotic stress or on the seed dormancy, germination, and plant resistance to transpiration), respectively (Ma et al., 2018; Asghar et al., 2019). However, few summaries for ABA derivatives and structural analogues (especially studies after 2014), biosynthesis, transport and catabolism have not been made. Therefore, this article summarizes the research overview for the ABA based on the following aspects: Firstly, the discovery of ABA receptor structure and signal transduction mechanism, as well as the regulation of ABA on plants under abiotic stress is introduced. Secondly, the current discovery of ABA analogues and their functions are summarized. Finally, the existing challenges are discussed. This brief article gives an overview on the progress for the ABA analogues in the past decades and introduces the latest progress. The aim for this briefly article provides readers with a convenient route to touch this topic, and hopefully serve some educational purpose for graduate students and assist further research in related fields.
ABA biosynthesis, transport and catabolism
As shown in Figures 1A,B, ABA is a sesquiterpenoid phytohormone containing 15 carbon atoms. ABA is synthesized in plants mainly using the C40 indirect pathway (also known as the carotenoid pathway), which is initiated from the cleavage of a C40 precursor known as β-carotene (Izquierdo-Bueno et al., 2018.; Takino el al., 2018). After cyclization and hydroxylation, β-carotene is converted to zeaxanthin (C40), which is catalyzed by zeaxanthin epoxidase (ZEP) to form all-trans-violaxanthin (C40). This pathway then bifurcates into two pathways. All-trans-violaxanthin can be further converted to 9′-cis-neoxanthin (C40) through all-trans-neoxanthin (C40), or it is converted to 9′-cis-violaxanthin (C40) directly by an unknown isomerase (North et al., 2007). Then, 9′-cis-violaxanthin and 9′-cis-neoxanthin both can be catalyzed by 9-cis-epoxycarotenoid dioxygenase (NCED) to produce xanthoxin (the C15 precursor of ABA), which can also act as a growth inhibitor (Anstis et al., 1975; Schwartz et al., 1997). Xanthoxin is presumed to migrate from the plastid to the cytosol (Nambara and Marion-Poll, 2005), where it is converted to abscisic aldehyde. Then abscisic aldehyde is eventually oxidized to ABA by abscisic aldehyde oxidase (AAO3) (Bittner et al., 2001; Cheng et al., 2002).
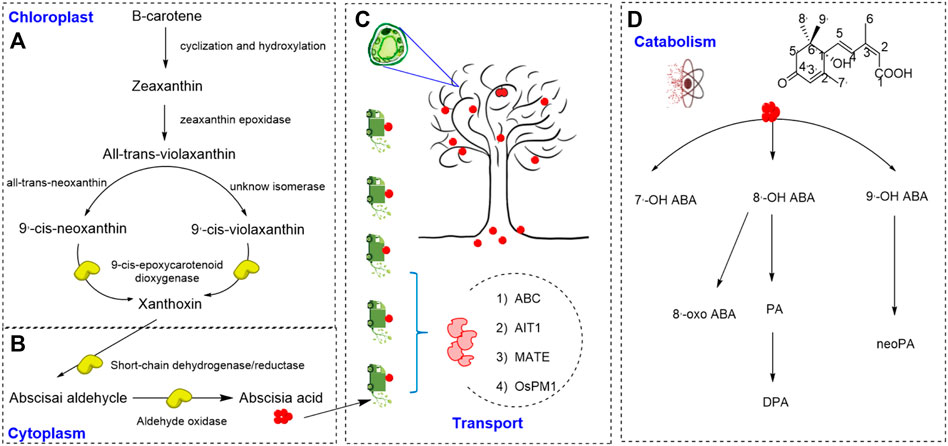
FIGURE 1. ABA biosynthesis, transport and catabolism (A,B). Biosynthetic pathway of abscisic acid (Izquierdo-Bueno et al., 2018; Takino et al., 2018). (C) Transport form of abscisic acid (Kuromori et al., 2010; Kang et al., 2015). (D) The catabolic pathway of abscisic acid (Nambara and Marion-Poll, 2005).
Recent studies showed that stress-induced ABA biosynthesis primarily occurs in vascular tissues and leaves, but ABA functions in various cells (Kuromori et al., 2010; Zhang et al., 2018). Therefore, it is important for ABA to transport among different organs, especially in the systemic stress responses of the whole plant. The active transport of ABA is mediated by many factors, such as ATP-binding cassette (ABC) transporters (Kuromori et al., 2010; Kang et al., 2015), ABA-IMPORTING TRANSPORTER1 (AIT1, which is also known as low-affinity nitrate transporter NRT1.2) (Kanno et al., 2012), DTX type/multidrug and toxic compound extrusion (MATE) transporters (Yu et al., 2014), and AWPM 19 family proteins (OsPM1) (Yao et al., 2018). These ABA transporters have been increasingly shown to be involved in stress responses, which are shown in the Figure 1C.
The catabolism of ABA in plant is controlled via two pathways: hydroxylation and conjugation, as shown in Figure 1D (Nambara and Marion-Poll, 2005). ABA can be hydroxylated via oxidation at three positions of the ring structure, C-7′, C-8′, and C-9′, triggering further inactivation steps, of which C-8′ is the primary site in plants (Cutler and Krochko, 1994). The unstable 8′-hydroxy ABA is converted to phaseic acid (PA) (Kushiro et al., 2004; Nambara and Marion-Poll, 2005), and PA has been reported to selectively activate a subset of ABA receptor PYLs (Weng et al., 2016). PA is then converted to dihydrophaseic acid (DPA), which is further catalyzed to DPA-4-O-β-D-glucoside (DPAG) (Weng et al., 2016). ABA conjugation is another pathway to regulate cellular ABA amounts under both normal and dehydration conditions (Lee et al., 2006; Xu et al., 2012). ABA and hydroxy ABA are conjugated with glucose for inactivation, forming different conjugates. The ABA-glucose ester (ABA-GE) is the predominant form of these conjugates and stored in vacuoles and the apoplast (Dietz et al., 2000; Liu et al., 2015). When the environment changes such as dehydration, ABA-GE is rapidly transformed to active ABA by β-glucosidases (Lee et al., 2006; Xu et al., 2012). The conjugation/deconjug-ation cycle enables plants to phenotypically adapt to their environment through ABA-mediated responses by activating and inactivating ABA rapidly (Chen et al., 2020).
ABA signal transduction and the role of ABA pathways in stress signaling
The ABA signaling pathway has been studied for several decades, and the core ABA signaling components including three protein classes: ABA receptors, negative regulators and positive regulators (Danquah et al., 2014). ABA functions in plants through cellular recognition by the intracellular receptor. Many ABA receptors have been reported so far, such as H subunit of Chloroplast Mg2+-chelatase, G-protein coupled Receptor 2, GPCR-type G protein, and Pyracbactin Resistance/Pyracbactin resistance-like/Regulatory Component of ABA Receptor (PYR/PYL/RCAR) (Guo et al., 2011).
PYR/PYL/RCARs are the most important among these receptors, the discovery of which basically elucidates the ABA signaling pathway (Ma et al., 2009; Park et al., 2009). Most plants encode more than one PYR/PYL/RCAR proteins, and they are differentially expressed in multiple organs, cells during different growth stages (Antoni et al., 2013; Dittrich et al., 2018). Genetic evidences showed that a high level of functional redundancy exists in this gene family, but they are essential for ABA perception, signal transduction and response to stress in plants. PYR/PYL/RCAR mutants displayed impaired ABA responses (Miao et al., 2018; Zhao et al., 2018), while overexpression of PYR/PYL/RCARs enhanced ABA responses to impact abiotic stress tolerance (Santiago et al., 2009; Mega et al., 2019).
As shown in Figure 2A, Protein Phosphatase 2Cs (PP2Cs) and SNF1-related protein kinase 2s (SnRKs) are major negative regulators and positive regulators of ABA signaling, respectively. PYR/PYL/RCARs show conformational change upon binding of ABA, which exposes the interaction surface allowing for favorable binding of some PP2Cs (Cutler et al., 2010). Consequently, the activities of PPC2s are inhibited, allowing activation of SnRK2s. Then SnRK2s activate downstream target proteins, including basic-domain leucine zipper (bZIP) transcription factors, anion channels and NADPH oxidases, to induce ABA responses. In the absence of ABA, PP2Cs are unable to bind to PYR/PYL/RCARs and they inactivate SnRKs by dephosphorylation (Soon et al., 2012). ABA is commonly known as the “stress hormone” that responds to variety of environmental stresses, especially for abiotic stresses (Kanchan et al., 2017). When plants encounter various abiotic stresses, such as salinity, extreme temperature, heavy metal, drought, UV-B, water stresses, endogenous ABA levels increase rapidly due to the increase of ABA biosynthesis and inhibition of ABA degradation. Then the downstream specific signaling pathways are activated and gene expression levels are modified (Kanchan et al., 2017).
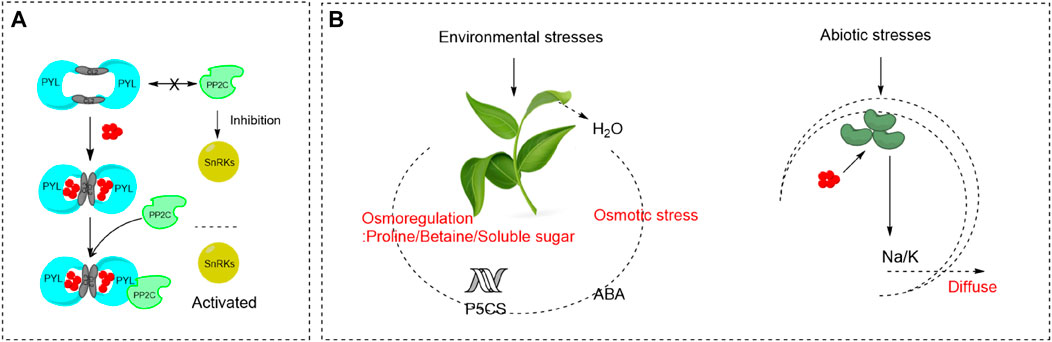
FIGURE 2. ABA signal transduction and the role of ABA pathways in stress signaling (A) The working model of ABA-dependent recognition and inhibition of PP2Cs by PYLs (Cutler et al., 2010; Soon et al., 2012). (B) The role of abscisic acid pathways in stress signaling (Shinozaki and Yamaguchi-Shinozaki, 2000; Finkelstein et al., 2002).
As shown in Figure 2B, osmotic stress caused by environmental stresses, such as high salinity, drought and cold, results in dehydration and inhibition of water uptake in plants. ABA accumulates under osmotic stress conditions and plays an important role in the stress responses and tolerance of plants (Shinozaki and Yamaguchi-Shinozaki 2000; Finkelstein et al., 2002). ABA promotes short-term responses like stomatal closure to maintain water balance (Agurla et al., 2018). For example, under salt stress, ABA is accumulated in plant roots and transported to the leaves, which leads to the decrease of leaf expansion rate and stomatal closure. As a result, the reduced transpiration rate and increased salt transportation in the root cap alleviate the damage causing by salt stress. ABA also stimulates longer-term growth responses through regulation of stress-responsive genes, the products of which may function in dehydration tolerance. Silva-Ortega et al., reported that ABA can regulate the expression of P5CS, which promotes the production and accumulation of osmotic adjustment substances like proline, betaine, and soluble sugar (Silva-Ortega et al., 2008).
Excepting mediating stomatal closure and osmotic regulation, ABA also involves in the balance of ion concentration under abiotic stresses. ABA participates in the modification of ATPase in plasma membrane and vacuolar membrane to provide more driving force for Na+/H+ antiporter, which strengthens Na+ efflux and ion regionalization to alleviate the damage of salt and alkaline stress (Janicka-Russak and Kłobus, 2007; Khadri et al., 2007). Three major pathways involved in the heavy metal detoxification can be triggered by ABA, inhibiting the uptake, altering the translocation from root to shoot, and promoting the conjugation with chelators (Hu et al., 2020). In addition, the decrease of photosynthesis caused by salt, heavy metal, alkaline and cold stress can be effectively relieved by ABA (Saradhi et al., 2010). The addition of exogenous ABA can accelerate the recovery of plant photosystem II, restore the photosynthetic inactivation state of the core complex to normal, maintain the stability of photosynthetic organs, and enhance the photosynthetic efficiency of leaf light capture and conversion (Saradhi et al., 2010). Moreover, ABA can interact with other stress resistance elements, such as antioxidant defense system and the MAPK cascades, to help plants resist environmental stresses (Danquah et al., 2014; Shu et al., 2016; Yang and Guo, 2018).
Chemical manipulation of ABA signaling structural analogues
Considering the importance of ABA signaling in many processes, including stress tolerance, seed germination, root growth and development, and senescence, small molecule chemicals can manipulate ABA signaling showing potential applications in agricultural production. Among them, the most extensively studied are the analogues of ABA receptor (Bari and Jones, 2009). Up to date, many compounds have been identified as significant ABA-agonist/antagonist and can be employed to reverse the excessive/moderate ABA action, which is shown in Figure 3.
In 1997, Rose synthesized compounds 1 and 2 (Rose et al., 1997), and carried out a series of anti-stress experiments, officially started the research on the application of ABA analogues in plants. They found that both compounds 1 and 2 could stimulate the radial conduction of water in maize roots just like ABA. In subsequent studies, analogues 1 and 2 were found to reduce chloride ion concentration and ethylene content in plants. More importantly, they can delay the consumption of CO2 assimilation under adverse conditions. The activity of compound 2 was better than that of abscisic acid, as the activity of compound 1 was similar to that of ABA. Oxidative hydroxylation of 8′- and 9′-methyl is the key to the metabolic inactivation of abscisic acid in plants. Therefore, modification of 8′- and 9′- methyl groups to obtain more stable ABA analogues has become the research direction of many scientists.
Nicholas M. Irvine et al. synthesized an anthracenone analogue of ABA (3) (Irvine et al., 2000), and Takahiro Inoue synthesized new ABA analogues (4 and 5) (Inoue and Oritani, 2000) by regioselective hydrocyanation and possessed a cyano or methoxycarbonyl group at the 6′α-position. Bioactivity tests were performed separately, and the results show that all the compounds were less than ABA, but raised the possibility of structural modification at the 6′α-position. In the same year, Cutler found that compounds (+)-8′-acetylen-ABA (1) and (+)-9' -propargyl-ABA (6) (Cutler et al., 2000) showed better activity than ABA in inhibiting arabidopsis seed germination. Among them (+)-8′-acetylene-ABA (1) can inhibit not only arabidopsis seed germination, but also maize cell growth and wheat embryo germination.
Ueno found that compounds 7 and 8 (Ueno et al., 2005) had no inhibitory effect on cytochrome P450 monooxidase, which might be caused by the fact that the C-8 group was too large to bind with 8' -hydroxylase. When C-8′ methyl hydrogen is replaced by methyl and ethyl groups, 8' -methyl abscisic acid (9) and 8' -ethyl abscisic acid (10) were obtained (Ueno et al., 2005). The results showed that compound 9 was a competitive inhibitor of 8' -hydroxylase, while compound 10 had no inhibitory activity. This may be due to the fact that methoxyl and ethyl have similar stereoscopic sizes and are similarly unable to bind to the active site, so it is thought that methyl group may be the largest group tolerated by the active binding site.
Nyangulu and co-workers (Nyangulu et al., 2006) synthesized tetrahydronaphthone ABA analogue 11 by substituting benzene ring of aromatic hydrocarbon for planar propylene group structure on six rings. When the concentration was 0.33 μmol/L, the inhibition rate of ABA was about 40%, while the inhibition rate of compound 11 was less than 10%. The R configuration of compound 11 was similar to that of S configuration. Compound 12 showed a highly activity in inhibiting germination of Arabidopsis thaliana seeds (Nyangulu et al., 2006). Compounds 13 and 14 were synthesized on the basis of improved compounds 1 and 2 (Nyangulu et al., 2006). They suggested that when the 8' -hydroxylase oxidizes 11 to 13, it inhibited further cyclization of compound 13 to PA analogue due to instability. Suspension culture of maize cells showed that the metabolic rate of compound 11 was similar to ABA. Compounds 13 and 14 exhibited similar activity to ABA at high concentrations. The biggest defect of ABA in practical application is poor photostability.
In order to obtain more stable ABA analogues, Liu has synthesized a photostable cis-2, 3-cyclopropanated abscisic acid analogue. They described the effect of ABA analogue isomers on activity. The photoisomerization study showed that compounds 15 and 16 (Liu et al., 2013) reached cis and inverse equilibrium in 44 and 36 h, respectively under 254 nm UV light, which was much longer than ABA (12 h) and thus had better photostability. The biological activities of compounds 15 and 16 under two modes of seed germination and seedling growth were tested. The results showed that at high concentrations (3 μmol/L and 5 μmol/L), both compounds had better inhibitory effects on seed germination than ABA did. But its inhibitory effect was lower than ABA at low concentration. The inhibitory effect of the two target compounds on the growth of Arabidopsis thaliana seedlings was lower than that of ABA at each concentration. Overall, compound 15 showed higher activity than compound 16. However, in most cases, the biological activity of the cis structure is lower than that of the trans structure, and the difference of the results provides an important basis for further study of the structure-activity relationship of ABA.
Han et al. developed a new ABA analogue named (+) 2′,3′-iso-PhABA (17 and 18) in order to overcome its oxidative inactivation. In vivo experiments, the activity of (+) - iso -PhABA (17) was significantly higher than that of (+)-ABA, including inhibition of lettuce and Arabidopsis seed germination (the inhibititory activity on arabidopsis thaliana seed germination is about 14 times of (+)-ABA), wheat embryo germination and rice seedling elongation. Protein phosphatase 2C (PP2C) activity showed that (+) - iso - PhABA (23) was an effective and selective ABA receptor agonist, which could better bind to PYL10 (Tan et al., 2013).
With the discovery of ABA receptor proteins and the application of chemical genetics in agriculture, Chantel L. Benson synthesized a series of ABA analogues as chemical probes to understand functional redundancy in the ABA receptor family (Benson et al., 2015). In PBI 352 (19), the substitution of hydrogen on C-10 by methyl group for OH induced stomatal closure, but did not affect the selectivity of analogues in Arabidopsis seedling germination or root growth. This selectivity has potential utility in practical applications where temporary drought protection can be provided to plants without reducing concomitant root growth. For all tested RCARs, the analogue PBI 352 (19) significantly reduced its receptor-binding activity compared with (S)-ABA (1), and PBI 352 (19) binds RCAR8 to inhibit ABI2.
Rajagopalan et al., developed a library of ABA analogues containing 240 compounds that are structural variants of the basic molecule S-(+)-ABA. These molecules were synthesized primarily for specific structural activity studies, and one antagonist PBI686 (20), was identified to be active on both RCAR receptors (Rajagopalan et al., 2016). Analogues PBI686 (20) and PBI664 (21) have no agonist activity by themselves, but are effective antagonists of ABA receptors blocking phosphatase binding to receptors.
In 2019, Wan et al., designed and synthesized five analogues of an earlier 2′,3′-iso-PhABA (Wan et al., 2019). Bioassay results showed that the number and position of methyl groups and the substitution of hydrogen atoms on methyl groups have great influence on the activity. Compared with 2′,3′-iso-PhABA, dimethyl-PhABA (22) showed slightly decreased inhibitory activity on seed germination and seedling growth of rice. However, it significantly reduced the ability to inhibit wheat embryo germination. Both demethyl-iso-PhABA (23) and demethyl- PhABA (24) showed weak inhibitory activity, and 11′-methoxy- PhABA (25/26) were more potent than their isomers 30/31 in all bioassays. These results suggested that the preservation of quaternary carbon at the 2′ or 3′ position is necessary for the maintenance of ABA-like biological activity, and the demethylation at the 3′ position is more significant. Among them, 25/26 showed good inhibitory activity and different binding selectivity to ABA receptor PYR1 and PYL2/PYL5.
Che et al., described a class of ABA agonist/antagonist probes, APAn (29) that regulate either agonist or antagonist activity according to the length of the 6′-alkoxy chain (Che et al., 2020). With the extension of alkoxy chain, the receptor binding potential increased gradually, and the inhibitory activity of HAB1 decreased. Theoretical analysis based on molecular docking and molecular dynamics simulations showed that some factors outside the ligand binding pocket in the receptor also affected ligand binding to the receptor. Van der Waals interaction between alkyl chains in APAn made the ligand binding to the ABA receptor tighter. This enhanced binding makes it an antagonist rather than a weakened agonist.
Recently, Wan et al., synthesized a series of ABA analogues and tested their activities (Wan et al., 2022). The results showed that the hydroxyl group of 1′-OH is an important functional group for seed germination and development. Therefore, compound 31 and 33 can inhibit seed germination, but which is not related to stomatal movement and response to drought stress regulated by ABA and its analogues. Methylation of 1′-OH significantly reduced their ability to bind to single receptor PYL5 and slightly enhanced their ability to bind to dimer receptor PYL2. The decreased inhibitory activities of 30, 32, and 34 on seed germination may be related to the decreased affinity of PYL5, which is caused by the destruction of hydrogen bond network. This suggests that PYL5 is a selective receptor closely related to plant growth.
The above section summarized 34 representative ABA analogues, and some have regulatory activity and some have inhibitory activity of P450 oxidase in the decomposition process, which can be used to study the molecular mechanism of ABA action and catabolism. Through the analysis of the activity or enzyme inhibitory activity of different analogues, it is known that there is a certain difference between the ABA active group and the structure inhibited by ABA 8′-hydroxylase, and this difference will help the development of new ABA analogues (An ideal enzyme inhibitor should have the ABA structural features required for substrate specificity, but not the structural features required to activate the ABA signaling pathway). Overall, the modification of site 1 (Figure 4), the spatial size and saturation will directly affect the binding ability of the compound to the target. The difference of the groups on site 2 (Figure 4) may block the binding ability of the downstream phosphatase to the receptor. The modification of site 3 (Figure 4) is to improve the high stability provides a new design idea. The modification (methylation) of site 4 (Figure 4) changes the binding ability of the analogue to the receptor by changing the hydrogen bond network.
ABA function analogues
In recent years, scientists have screened many ABA functional analogues (Figure 5) using chemical genetic methods, their structures are very different from the structure of ABA. Among which, the most representative structures are sulfonamides and amide derivatives.
Pyrabactin, a naphthalene-sulfonamide derivative, is the first synthetic ABA mimic (Park et al., 2009). Pyrabactin displayed several ABA-like activities, such as inhibition of seed germination (Park et al., 2009), induced stomatal closure (Puli and Raghavendra, 2012) and enhanced root hydraulic conductivity (Fan et al., 2015). It could be used to improve the drought tolerance of crop plants. Several structural analogues of pyrabactin have been screened and the structure-activity relationship revealed that pyridyl nitrogen is important for binding affinity biological activity (Helander et al., 2016). Studies also discovered that pyrabactin exhibits distinct responses towards PYL-subtypes. It strongly activates PYR1/PYL1 but acts as an antagonist for PYL2 and PYL3 (Melcher et al., 2010; Miyakawa and Tanokura, 2011).
Okamoto et al., identified sulfonamide AM1 (also named as quinabactin), through a yeast two-hybrid screen (Okamoto et al., 2013). Quinabactin showed a broader spectrum of PYL activity with enhanced bioactivity as compared to pyrabactin. Quinabactin treatments inhibited seed germination, prevented leaf water loss, and promoted drought resistance (Cao et al., 2013). Cao et al. developed a series of AM1 fluorine derivatives (AMFs) by progressively substituting fluorine atoms on the benzyl ring. The fluorine atoms in AMFs increase the plasma membrane permeability and hydrogen bonding network. Among these derivatives, AMF4 was proved to be a better agonist than AM1 or ABA (Cao et al., 2017). Overtveldt et al. designed phosphonamide analogues of pyrabactin, which given a different physiological response by selectively stimulating ABA-signaling pathways (Van Overtveldt et al., 2015). Further efforts exploited the structure of cyanabactin, which has a nitrile and isopropyl substituted monocyclic ring system instead of the bicyclic ring system in pyrabactin and quinabactin (Park et al., 2009; Cao et al., 2013). Using a wheat cell free-based drug screening approach, Nemoto et al. identified two novel ABA receptor agonists with bioactivity, in which JFA1 and JFA2. JFA2 was demonstrated to induce drought tolerance without affecting root growth (Nemoto et al., 2018). Cyanabactin has superior ABA-like activity in vegetative tissues and is highly effective to control plant water use (Vaidya et al., 2017). Recently, Vaidya et al. developed opabactin by a combination of virtual screening, x-ray crystallography, and structure-guided design. Opabactin is the most potent ABA agonist developed, which showed approximately sevenfold increase in receptor affinity relative to ABA and up to 10-fold greater activity in vivo (Vaidya et al., 2019).
As a known fungicide, mandipropamid (Figure 5) was reported to interact with a hextuple PYR1 mutant (PYR1MANDI) and induced stomatal closure and inhibited seed germination in transgenic plants with PYR1MANDI (Park et al., 2015).
Conclusion and outlooks
In this brief review, the research progress of ABA analogues including mechanism of action, signaling pathways, and ABA functional analogs is reviewed. the representative 34 structural analogs and 8 functional analogs are summarized, and the differences in biological activity and catabolism of ABA analogues are systematically analyzed. we conclude that the C-5,, C-6,, C-8,, C-9, space size (functional group size) and saturations could directly affect the binding ability of the compound to the target, methyl may be the largest group that can be accepted. Differences in groups on C-2,, C-3,, C-7, may block the ability of downstream phosphatases to bind to receptors. The improved photostability of C-2, C-3, C-6 provides a new design idea (cyclopropyl). Methylation of C-1, alters the binding capacity of the analogues to the receptor by altering the hydrogen bonding network. Activity analysis of sulfonamide-based ABA functional analogues revealed that pyrabactin exhibits distinct responses towards PYL-subtypes. It strongly activates PYR1/PYL1 but acts as an antagonist for PYL2 and PYL3, and AM1 showed a broader spectrum of PYL activity with higher bioactivity compared to pyrabactin. Opabactin, the most potential ABA agonist to date, showed an approximately sevenfold increase in receptor affinity relative to ABA and up to 10-fold greater activity in vivo.
Currently, studies have revealed that PYLs can be divided into dimer receptors (PYR1, PYL1 to PYL3) and monomeric receptors (PYL4 to PYL13). However, the specific functional differences between them in the ABA signaling pathway are unclear. Utilizing chemical genetics to clarify these differences is an important means for understanding the function of PYLs proteins and an important measure to discover novel ABA functional analogues. Furthermore, on the basis of the predecessors, the in-depth research on the structure-activity relationship of ABA analogues are expected to discover ABA analogues with novel structure, higher activity and better selectivity. Foreseeably, novel ABA functional analogues will play an important role in agriculture.
Author contributions
All authors have read and agreed to the published version of the manuscript. YL, SC, and PW collected and analyzed the refences. YL completed pictures and spreadsheets, conceived and wrote the draft of the manuscript. SG and JW revised and the paper.
Funding
This work was supported by NSFC (National Natural Science Foundation of China) (Nos. 32072445, 21762012), the Program of Introducing Talents to Chinese Universities (D20023) and the Guizhou Science and Technology Project [(2017 5788)], and the Natural Science research project of Guizhou Education Department [KY (2018)009].
Conflict of interest
The authors declare that the research was conducted in the absence of any commercial or financial relationships that could be construed as a potential conflict of interest.
Publisher’s note
All claims expressed in this article are solely those of the authors and do not necessarily represent those of their affiliated organizations, or those of the publisher, the editors and the reviewers. Any product that may be evaluated in this article, or claim that may be made by its manufacturer, is not guaranteed or endorsed by the publisher.
References
Agurla, S., Gahir, S., Munemasa, S., Murata, Y., and Raghavendra, A. S. (2018). Mechanism of stomatal closure in plants exposed to drought and cold stress. Adv. Exp. Med. Biol. 1081, 215–232. doi:10.1007/978-981-13-1244-1_12
Anstis, P. J. P., Friend, J., and Gardner, D. C. J. (1975). The role of xanthoxin in the inhibition of pea seedling growth by red light. Phytochemistry 14 (1), 31–35. doi:10.1016/0031-9422(75)85002-3
Antoni, R., Gonzalez-Guzman, M., Rodriguez, L., Peirats-Llobet, M., Pizzio, G. A., Fernandez, M. A., et al. (2013). PYRABACTIN RESISTANCE1-LIKE8 plays an important role for the regulation of abscisic acid signaling in root. Plant Physiol. 161 (2), 931–941. doi:10.1104/pp.112.208678
Asghar, M. A., Li, Y., Jiang, H., Sun, X., Ahmad, B., Imran, S., et al. (2019). Crosstalk between abscisic acid and auxin under osmotic stress. Agron. J. 111 (5), 2157–2162. doi:10.2134/agronj2018.10.0633
Bari, R., and Jones, J. D. G. (2009). Role of plant hormones in plant defence responses. Plant Mol. Biol. 69 (4), 473–488. doi:10.1007/s11103-008-9435-0
Benson, C. L., Kepka, M., Wunschel, C., Rajagopalan, N., Nelson, K. M., Christmann, A., et al. (2015). Abscisic acid analogs as chemical probes for dissection of abscisic acid responses in Arabidopsis thaliana. Phytochemistry 113, 96–107. doi:10.1016/j.phytochem.2014.03.017
Bittner, F., Oreb, M., and Mendel, R. R. (2001). ABA3 is a molybdenum cofactor sulfurase required for activation of aldehyde oxidase and xanthine dehydrogenase in Arabidopsis thaliana. J. Biol. Chem. 276 (44), 40381–40384. doi:10.1074/jbc.C100472200
Cao, M.-J., Zhang, Y.-L., Liu, X., Huang, H., Zhou, X. E., Wang, W.-L., et al. (2017). Combining chemical and genetic approaches to increase drought resistance in plants. Nat. Commun. 8 (1), 1183. doi:10.1038/s41467-017-01239-3
Cao, M., Liu, X., Zhang, Y., Xue, X., Zhou, X. E., Melcher, K., et al. (2013). An ABA-mimicking ligand that reduces water loss and promotes drought resistance in plants. Cell Res. 23 (8), 1043–1054. doi:10.1038/cr.2013.95
Che, C., Zeng, Y., Xu, Y., Lu, H., Xu, Y., Zhang, X., et al. (2020). APAn, a class of ABA receptor agonism/antagonism switching probes. J. Agric. Food Chem. 68 (32), 8524–8534. doi:10.1021/acs.jafc.0c02154
Chen, K., Li, G. J., Bressan, R. A., Song, C. P., Zhu, J. K., and Zhao, Y. (2020). Abscisic acid dynamics, signaling, and functions in plants. J. Integr. Plant Biol. 62 (1), 25–54. doi:10.1111/jipb.12899
Cheng, W.-H., Endo, A., Zhou, L., Penney, J., Chen, H.-C., Arroyo, A., et al. (2002). A unique short-chain dehydrogenase/reductase in Arabidopsis glucose signaling and abscisic acid biosynthesis and functions. Plant Cell 14 (11), 2723–2743. doi:10.1105/tpc.006494
Cutler, A. J., and Krochko, J. E. (1994). Formation and breakdown of ABA. Trends Plant Sci. 4 (12), 472–478. doi:10.1016/S1360-1385(99)01497-1
Cutler, A. J., Rose, P. A., Squires, T. M., Loewen, M. K., Shaw, A. C., Quail, J. W., et al. (2000). Inhibitors of abscisic acid 8'-hydroxylase. Biochemistry 39, 13614–13624. doi:10.1021/bi0014453
Cutler, S. R., Rodriguez, P. L., Finkelstein, R. R., and Abrams, S. R. (2010). Abscisic acid: Emergence of a core signaling network. Annu. Rev. Plant Biol. 61, 651–679. doi:10.1146/annurev-arplant-042809-112122
Danquah, A., de Zelicourt, A., Colcombet, J., and Hirt, H. (2014). The role of ABA and MAPK signaling pathways in plant abiotic stress responses. Biotechnol. Adv. 32 (1), 40–52. doi:10.1016/j.biotechadv.2013.09.006
Dietz, K. J., Sauter, A., Wichert, K., Messdaghi, D., and Hartung, W. (2000). Extracellular β‐glucosidase activity in barley involved in the hydrolysis of ABA glucose conjugate in leaves. J. Exp. Bot. 51 (346), 937–944. doi:10.1093/jexbot/51.346.937
Dittrich, M., Mueller, H. M., Bauer, H., Peirats-Llobet, M., Rodriguez, P. L., Geilfus, C.-M., et al. (2019). The role of Arabidopsis ABA receptors from the PYR/PYL/RCAR family in stomatal acclimation and closure signal integration. Nat. Plants 5 (9), 1002–1011. doi:10.1038/s41477-019-0490-0
Fan, W., Li, J., Jia, J., Wang, F., Cao, C., Hu, J., et al. (2015). Pyrabactin regulates root hydraulic properties in maize seedlings by affecting PIP aquaporins in a phosphorylation-dependent manner. Plant Physiology Biochem. 94, 28–34. doi:10.1016/j.plaphy.2015.05.005
Fidler, J., Zdunek-Zastocka, E., and Bielawski, W. (2015). Regulation of abscisic acid metabolism in relation to the dormancy and germination of cereal grains. Acta Soc. Bot. Pol. 84 (1), 3–11. doi:10.5586/asbp.2015.004
Finkelstein, R. R., Gampala, S. S., and Rock, C. D. (2002). Abscisic acid signaling in seeds and seedlings. PlantCell 14 (Suppl. l), S15–S45. doi:10.1105/tpc.010441
Guo, J., Yang, X., Weston, D. J., and Chen, J.-G. (2011). Abscisic acid receptors: Past, present and FutureF. J. Integr. Plant Biol. 53 (6), 469–479. doi:10.1111/j.1744-7909.2011.01044.x
Guo, S., He, F., Song, B., and Wu, J. (2021). Future direction of agrochemical development for plant disease in China. Food Energy Secur 10 (4), e293. doi:10.1002/fes3.293
Helander, J. D. M., Vaidya, A. S., and Cutler, S. R. (2016). Chemical manipulation of plant water use. Bioorg. Med. Chem. 24 (3), 493–500. doi:10.1016/j.bmc.2015.11.010
Hoth, S., Morgante, M., Sanchez, J.-P., Hanafey, M. K., Tingey, S. V., and Chua, N.-H. (2002). Genome-wide gene expression profiling in Arabidopsis thaliana reveals new targets of abscisic acid and largely impaired gene regulation in theabi1-1mutant. J. Cell Sci. 115 (Pt 24), 4891–4900. doi:10.1242/jcs.00175
Hu, B., Deng, F., Chen, G., Chen, X., Gao, W., Long, L., et al. (2020). Evolution of abscisic acid signaling for stress responses to toxic metals and metalloids. Front. Plant Sci. 11, 909. doi:10.3389/fpls.2020.00909
Inoue, T., and Oritani, T. (2000). Syntheses of (±)-Methyl 6′α-Demethyl-6′α-cyanoabscisate and (±)-Methyl 6′α-Demethyl-6′α-methoxycarbonylabscisate. Biosci. Biotechnol. Biochem. 64 (5), 1071–1074. doi:10.1271/bbb.64.1071
Irvine, N. M., Rose, P. A., Cutler, A. J., Squires, T. M., and Abrams, S. R. (2000). Anthracenone ABA analogue as a potential photoaffinity reagent for ABA-binding proteins. Phytochemistry 53 (3), 349–355. doi:10.1016/S0031-9422(99)00482-3
Izquierdo-Bueno, I., González-Rodríguez, V. E., Simon, A., Dalmais, B., Pradier, J. M., Le Pêcheur, P., et al. (2018). Biosynthesis of abscisic acid in fungi: Identification of a sesquiterpene cyclase as the key enzyme inBotrytis cinerea. Environ. Microbiol. 20 (7), 2469–2482. doi:10.1111/1462-2920.14258
Janicka-Russak, M., and Kłobus, G. (2007). Modification of plasma membrane and vacuolar H+-ATPases in response to NaCL and ABA. J. Plant Physiology 164 (3), 295–302. doi:10.1016/j.jplph.2006.01.014
Kang, J., Yim, S., Choi, H., Kim, A., Lee, K. P., Lopez-Molina, L., et al. (2015). Abscisic acid transporters cooperate to control seed germination. Nat. Commun. 6, 8113. doi:10.1038/ncomms9113
Kanno, Y., Hanada, A., Chiba, Y., Ichikawa, T., Nakazawa, M., Matsui, M., et al. (2012). Identification of an abscisic acid transporter by functional screening using the receptor complex as a sensor. Proc. Natl. Acad. Sci. U.S.A. 109 (24), 9653–9658. doi:10.1073/pnas.1203567109
Khadri, M., Tejera, N. A., and Lluch, C. (2007). Sodium chloride-ABA interaction in two common bean (Phaseolus vulgaris) cultivars differing in salinity tolerance. Environ. Exp. Bot. 60 (2), 211–218. doi:10.1016/j.envexpbot.2006.10.008
Kim, S. Y. (2007). Recent advances in ABA signaling. J. Plant Biol. 50 (2), 117–121. doi:10.1007/BF03030619
Kuromori, T., Miyaji, T., Yabuuchi, H., Shimizu, H., Sugimoto, E., Kamiya, A., et al. (2010). ABC transporter AtABCG25 is involved in abscisic acid transport and responses. Proc. Natl. Acad. Sci. U.S.A. 107 (5), 2361–2366. doi:10.1073/pnas.0912516107
Kushiro, T., Okamoto, M., Nakabayashi, K., Yamagishi, K., Kitamura, S., Asami, T., et al. (2004). The arabidopsis cytochrome P450 CYP707A encodes ABA 8′-hydroxylases: Key enzymes in ABA catabolism. Embo J. 23 (7), 1647–1656. doi:10.1038/sj.emboj.7600121
Lee, K. H., Piao, H. L., Kim, H.-Y., Choi, S. M., Jiang, F., Hartung, W., et al. (2006). Activation of glucosidase via stress-induced polymerization rapidly increases active pools of abscisic acid. Cell 126 (6), 1109–1120. doi:10.1016/j.cell.2006.07.034
Liu, Z., Yan, J.-P., Li, D.-K., Luo, Q., Yan, Q., Liu, Z.-B., et al. (2015). UDP-Glucosyltransferase71C5, a major glucosyltransferase, mediates abscisic acid homeostasis in arabidopsis. Plant Physiol. 167 (4), 1659–1670. doi:10.1104/pp.15.00053
Ma, Y., Cao, J., He, J., Chen, Q., Li, X., and Yang, Y. (2018). Molecular mechanism for the regulation of ABA homeostasis during plant development and stress responses. Ijms 19 (11), 3643. doi:10.3390/ijms19113643
Ma, Y., Szostkiewicz, I., Korte, A., Moes, D., Yang, Y., Christmann, A., et al. (2009). Regulators of PP2C phosphatase activity function as abscisic acid sensors. Science 324 (5930), 1064–1068. doi:10.1126/science.1172408
Mega, R., Abe, F., Kim, J.-S., Tsuboi, Y., Tanaka, K., Kobayashi, H., et al. (2019). Tuning water-use efficiency and drought tolerance in wheat using abscisic acid receptors. Nat. Plants 5 (2), 153–159. doi:10.1038/s41477-019-0361-8
Melcher, K., Xu, Y., Ng, L.-M., Zhou, X. E., Soon, F.-F., Chinnusamy, V., et al. (2010). Identification and mechanism of ABA receptor antagonism. Nat. Struct. Mol. Biol. 17 (9), 1102–1108. doi:10.1038/nsmb.1887
Miao, C., Xiao, L., Hua, K., Zou, C., Zhao, Y., Bressan, R. A., et al. (2018). Mutations in a subfamily of abscisic acid receptor genes promote rice growth and productivity. Proc. Natl. Acad. Sci. U.S.A. 115 (23), 6058–6063. doi:10.1073/pnas.1804774115
Miyakawa, T., and Tanokura, M. (2011). Regulatory mechanism of abscisic acid signaling. Biophysics 7, 123–128. doi:10.2142/biophysics.7.123
Nambara, E., and Marion-Poll, A. (2005). Abscisic acid biosynthesis and catabolism. Annu. Rev. Plant Biol. 56, 165–185. doi:10.1146/annurev.arplant.56.032604.144046
Nemhauser, J. L., Hong, F., and Chory, J. (2006). Different plant hormones regulate similar processes through largely nonoverlapping transcriptional responses. Cell 126 (3), 467–475. doi:10.1016/j.cell.2006.05.050
Nemoto, K., Kagawa, M., Nozawa, A., Hasegawa, Y., Hayashi, M., Imai, K., et al. (2018). Identification of new abscisic acid receptor agonists using a wheat cell-free based drug screening system. Sci. Rep. 8 (1), 4268. doi:10.1038/s41598-018-22538-9
North, H. M., Almeida, A. D., Boutin, J.-P., Frey, A., To, A., Botran, L., et al. (2007). The Arabidopsis ABA-deficient mutant aba4 demonstrates that the major route for stress-induced ABA accumulation is via neoxanthin isomers. Plant J. Cell Mol. Biol. 50 (5), 810–824. doi:10.1111/j.1365-313X.2007.03094.x
Nyangulu, J. M., Nelson, K. M., Rose, P. A., Gai, Y., Loewen, M., Lougheed, B., et al. (2006). Synthesis and biological activity of tetralone abscisic acid analogues. Org. Biomol. Chem. 4 (7), 1400–1412. doi:10.1039/b509193d
Okamoto, M., Peterson, F. C., Defries, A., Park, S.-Y., Endo, A., Nambara, E., et al. (2013). Activation of dimeric ABA receptors elicits guard cell closure, ABA-regulated gene expression, and drought tolerance. Proc. Natl. Acad. Sci. U.S.A. 110 (29), 12132–12137. doi:10.1073/pnas.1305919110
Park, S.-Y., Fung, P., Nishimura, N., Jensen, D. R., Fujii, H., Zhao, Y., et al. (2009). Abscisic acid inhibits type 2C protein phosphatases via the PYR/PYL family of START proteins. Science 324 (5930), 1068–1071. doi:10.1126/science.1173041
Park, S.-Y., Peterson, F. C., Mosquna, A., Yao, J., Volkman, B. F., and Cutler, S. R. (2015). Agrochemical control of plant water use using engineered abscisic acid receptors. Nature 520 (7548), 545–548. doi:10.1038/nature14123
Puli, M. R., and Raghavendra, A. S. (2012). Pyrabactin, an ABA agonist, induced stomatal closure and changes in signalling components of guard cells in abaxial epidermis of Pisum sativum. J. Exp. Bot. 63 (3), 1349–1356. doi:10.1093/jxb/err364
Rajagopalan, N., Nelson, K. M., Douglas, A. F., Jheengut, V., Alarcon, I. Q., McKenna, S. A., et al. (2016). Abscisic acid analogues that act as universal or selective antagonists of phytohormone receptors. Biochemistry 55, 5155–5164. doi:10.1021/acs.biochem.6b00605
Rose, P. A., Cutler, A. J., Irvine, N. M., Shaw, A. C., Squires, T. M., Loewen, M. K., et al. (1997). 8'-Acetylene ABA: An irreversible inhibitor of ABA 8'-hydroxylase. Bioorg. Med. Chem. Lett. 7 (19), 2543–2546. doi:10.1016/S0960-894X(97)10015-4
Santiago, J., Rodrigues, A., Saez, A., Rubio, S., Antoni, R., Dupeux, F., et al. (2009). Modulation of drought resistance by the abscisic acid receptor PYL5 through inhibition of clade A PP2Cs. Plant J. Cell Mol. Biol. 60 (4), 575–588. doi:10.1111/j.1365-313X.2009.03981.x
Saradhi, P. P., Suzuki, I., Katoh, A., Sakamoto, A., Sharmila, P., Shi, D.-J., et al. (2000). Protection against the photo-induced inactivation of the photosystem II complex by abscisic acid. Plant Cell Environ. 23 (7), 711–718. doi:10.1046/j.1365-3040.2000.00579.x
Schwartz, S. H., Tan, B. C., Gage, D. A., Zeevaart, J. A. D., and McCarty, D. R. (1997). Specific oxidative cleavage of carotenoids by VP14 of maize. Science 276 (5320), 1872–1874. doi:10.1126/science.276.5320.1872
Seki, M., Ishida, J., Narusaka, M., Fujita, M., Nanjo, T., Umezawa, T., et al. (2002). Monitoring the expression pattern of around 7,000 Arabidopsis genes under ABA treatments using a full-length cDNA microarray. Funct. Integr. Genomics 2 (6), 282–291. doi:10.1007/s10142-002-0070-6
Shinozaki, K., and Yamaguchi-Shinozaki, K. (2000). Molecular responses to dehydration and low temperature: Differences and cross-talk between two stress signaling pathways. Curr. Opin. Plant Biol. 3 (3), 217–223. doi:10.1016/s1369-5266(00)80068-0
Shu, S., Gao, P., Li, L., Yuan, Y., Sun, J., and Guo, S. (2016). Abscisic acid-induced H2O2 accumulation enhances antioxidant capacity in pumpkin-grafted cucumber leaves under Ca(NO3)2 stress. Front. Plant Sci. 7, 1489. doi:10.3389/fpls.2016.01489
Silva-Ortega, C. O., Ochoa-Alfaro, A. E., Reyes-Agüero, J. A., Aguado-Santacruz, G. A., and Jiménez-Bremont, J. F. (2008). Salt stress increases the expression of p5cs gene and induces proline accumulation in cactus pear. Plant Physiology Biochem. 46 (1), 82–92. doi:10.1016/j.plaphy.2007.10.011
Soon, F.-F., Ng, L.-M., Zhou, X. E., West, G. M., Kovach, A., Tan, M. H. E., et al. (2012). Molecular mimicry regulates ABA signaling by SnRK2 kinases and PP2C phosphatases. Science 335 (6064), 85–88. doi:10.1126/science.1215106
Takino, J., Kozaki, T., Sato, Y., Liu, C., Ozaki, T., Minami, A., et al. (2018). Unveiling biosynthesis of the phytohormone abscisic acid in fungi: Unprecedented mechanism of core scaffold formation catalyzed by an unusual sesquiterpene synthase. J. Am. Chem. Soc. 140 (39), 12392–12395. doi:10.1021/jacs.8b08925
Tan, Z. H., Han, X. Q., Wan, C., and Xiao, Y. (2013). High-activity benzoiso abscisic acid analogue and preparation method thereof. CN. Patent No 103,435,472. Beijing: China National Intellectual Property Administration.
Ueno, K., Yoneyama, H., Saito, S., Mizutani, M., Sakata, K., Hirai, N., et al. (2005). A lead compound for the development of ABA 8′-hydroxylase inhibitors. Bioorg. Med. Chem. Lett. 15 (23), 5226–5229. doi:10.1016/j.bmcl.2005.08.042
Vaidya, A. S., Helander, J. D. M., Peterson, F. C., Elzinga, D., Dejonghe, W., Kaundal, A., et al. (2019). Dynamic control of plant water use using designed ABA receptor agonists. Science 366 (6464), eaaw8848. doi:10.1126/science.aaw8848
Vaidya, A. S., Peterson, F. C., Yarmolinsky, D., Merilo, E., Verstraeten, I., Park, S.-Y., et al. (2017). A rationally designed agonist defines subfamily IIIA abscisic acid receptors as critical targets for manipulating transpiration. ACS Chem. Biol. 12 (11), 2842–2848. doi:10.1021/acschembio.7b00650
Van Overtveldt, M., Heugebaert, T. S. A., Verstraeten, I., Geelen, D., and Stevens, C. V. (2015). Phosphonamide pyrabactin analogues as abscisic acid agonists. Org. Biomol. Chem. 13 (18), 5260–5264. doi:10.1039/c5ob00137d
Vishwakarma, K., Upadhyay, N., Kumar, N., Yadav, G., Singh, J., Mishra, R. K., et al. (2017). Abscisic acid signaling and abiotic stress tolerance in plants: A review on current knowledge and future prospects. Front. Plant Sci. 08, 161. doi:10.3389/fpls.2017.00161
Waadt, R., Seller, C. A., Hsu, P.-K., Takahashi, Y., Munemasa, S., and Schroeder, J. I. (2022). Plant hormone regulation of abiotic stress responses. Nat. Rev. Mol. Cell Biol. doi:10.1038/s41580-022-00479-6
Wan, C., Hong, Q., Zhang, X., Zeng, Y., Yang, D., Che, C., et al. (2019). Role of the ring methyl groups in 2′,3′-benzoabscisic acid analogues. J. Agric. Food Chem. 67 (17), 4995–5007. doi:10.1021/acs.jafc.8b07068
Wan, C., Yang, D., Liu, R., Lu, H., Che, C., Xu, Y., et al. (2022). 1′-OH of ABA and its analogs is a crucial functional group correspondence to seed germination and development of plants. J. Mol. Struct. 1249, 131650. doi:10.1016/j.molstruc.2021.131650
Weng, J.-K., Ye, M., Li, B., and Noel, J. P. (2016). Co-Evolution of hormone metabolism and signaling networks expands plant adaptive plasticity. Cell 166 (4), 881–893. doi:10.1016/j.cell.2016.06.027
Wenjian, L., Xiaoqiang, H., Yumei, X., Jinlong, F., Yuanzhi, Z., Huizhe, L., et al. (2013). Synthesis, photostability and bioactivity of 2,3-cyclopropanated abscisic acid. Phytochemistry 96, 72–80. doi:10.1016/j.phytochem.2013.09.029
Xu, Z.-Y., Lee, K. H., Dong, T., Jeong, J. C., Jin, J. B., Kanno, Y., et al. (2012). A vacuolar β-glucosidase homolog that possesses glucose-conjugated abscisic acid hydrolyzing activity plays an important role in osmotic stress responses in arabidopsis. Plant Cell 24 (5), 2184–2199. doi:10.1105/tpc.112.095935
Yang, Y., and Guo, Y. (2018). Unraveling salt stress signaling in plants. J. Integr. Plant Biol. 60 (9), 796–804. doi:10.1111/jipb.12689
Yao, L., Cheng, X., Gu, Z., Huang, W., Li, S., Wang, L., et al. (2018). The AWPM-19 family protein OsPM1 mediates abscisic acid influx and drought response in rice. Plant Cell 30 (6), 1258–1276. doi:10.1105/tpc.17.00770
Yu, X.-Z., Wang, D.-Q., and Zhang, X.-H. (2014). Chelator-induced phytoextraction of zinc and copper by rice seedlings. Ecotoxicology 23 (4), 749–756. doi:10.1007/s10646-014-1188-8
Zhang, F.-P., Sussmilch, F., Nichols, D. S., Cardoso, A. A., Brodribb, T. J., and McAdam, S. A. M. (2018). Leaves, not roots or floral tissue, are the main site of rapid, external pressure-induced ABA biosynthesis in angiosperms. J. Exp. Bot. 69 (5), 1261–1267. doi:10.1093/jxb/erx480
Zhang, X. L., Jiang, L., Xin, Q., Liu, Y., Tan, J. X., and Chen, Z. Z. (2015). Structural basis and functions of abscisic acid receptors PYLs. Front. Plant Sci. 6. doi:10.3389/fpls.2015.00088
Keywords: abscisic acid (ABA), analogues, biological activity, mechanism of action, research progress
Citation: Liu Y, Chen S, Wei P, Guo S and Wu J (2022) A briefly overview of the research progress for the abscisic acid analogues. Front. Chem. 10:967404. doi: 10.3389/fchem.2022.967404
Received: 12 June 2022; Accepted: 27 June 2022;
Published: 22 July 2022.
Edited by:
Pei Li, Kaili University, ChinaReviewed by:
Song Bai, Guizhou Institute of Technology, ChinaYong Guo, Zhengzhou University, China
Qilong Zhang, Guizhou Medical University, China
Copyright © 2022 Liu, Chen, Wei, Guo and Wu. This is an open-access article distributed under the terms of the Creative Commons Attribution License (CC BY). The use, distribution or reproduction in other forums is permitted, provided the original author(s) and the copyright owner(s) are credited and that the original publication in this journal is cited, in accordance with accepted academic practice. No use, distribution or reproduction is permitted which does not comply with these terms.
*Correspondence: Jian Wu, d3VqaWFuMjY5MUAxMjYuY29t
†These authors have contributed equally to this work