- 1Pharmaceutical Sciences Research Center, School of Pharmacy, Shiraz University of Medical Sciences, Shiraz, Iran
- 2Department of Chemistry, College of Sciences, Shiraz University, Shiraz, Iran
- 3Department of Chemistry, National Cheng Kung University, Tainan, Taiwan
- 4Centre de Recherche du Centre Hospitalier de l’Université de Montréal (CRCHUM), Montréal, QC, Canada
- 5Department of Microbiology, Faculty of Medicine, Kermanshah University of Medical Sciences, Kermanshah, Iran
- 6Department of Cell and Molecular Sciences, Faculty of Biological Sciences, Kharazmi University, Tehran, Iran
Nanoparticles offer numerous advantages in various fields of science, particularly in medicine. Over recent years, the use of nanoparticles in disease diagnosis and treatments has increased dramatically by the development of stimuli-responsive nano-systems, which can respond to internal or external stimuli. In the last 10 years, many preclinical studies were performed on physically triggered nano-systems to develop and optimize stable, precise, and selective therapeutic or diagnostic agents. In this regard, the systems must meet the requirements of efficacy, toxicity, pharmacokinetics, and safety before clinical investigation. Several undesired aspects need to be addressed to successfully translate these physical stimuli-responsive nano-systems, as biomaterials, into clinical practice. These have to be commonly taken into account when developing physically triggered systems; thus, also applicable for nano-systems based on nanomaterials. This review focuses on physically triggered nano-systems (PTNSs), with diagnostic or therapeutic and theranostic applications. Several types of physically triggered nano-systems based on polymeric micelles and hydrogels, mesoporous silica, and magnets are reviewed and discussed in various aspects.
Introduction
In the last few years, the increased incidence of chronic diseases such as cancer and metabolic disorders has triggered the need for more efficient, specific, and localized treatments that can deliver drugs at the target site in a highly controlled manner and also allow a precise and early diagnosis (Sahle et al., 2018). Nanotechnology-based systems, or nano-systems, are widely used to address these needs, and various techniques have been developed to obtain more specific and personalized treatments (Farjadian et al., 2019a). Nano-systems in the human body can perform the function of carrying an active substance (drug, contrast agent, and biologic molecules) to a defined site or can constitute themselves the active substance (their imaging or therapeutic properties) (Deng et al., 2020). The design and properties of nano-systems permit combining tissue targeting, molecular diagnosis, cellular imaging, and drug delivery approach to obtain a synergic effect and efficacious responses (Mitra et al., 2017).
Nano-theranostics is a rapidly increasing interest with simultaneous diagnosis and therapy, which resulted in the development of “personalized medicine” (Xie et al., 2010; Zhu et al., 2016; Calatayud et al., 2022). The most important properties to consider for this approach where control drug loading capacity, release, and system stability are size, charge, surface properties, shape, in vivo distribution, and toxicity (Wong et al., 2020).
Similarly, nano-systems for diagnostics provide rapid and early disease detection. Several types of nanoparticles, including polymeric micelles and hydrogels, silica, gold, and magnetic nanoparticles, allow us to imagine pathologies and understand the physiological mechanisms of diseases and treatments (Li A. et al., 2021). Nonetheless, their take up in clinical settings has been slow due to the complex pharmacokinetic and pharmacology associated effects (Xie et al., 2010). Several nano-systems can be used as non-invasive contrast agents when paired with a suitable imaging technique. For instance, in whole-body scans, encapsulated nano-contrast agents could be adopted in computed tomography (CT), magnetic resonance imaging (MRI), single-photon emission CT (SPECT), and positron emission tomography (PET) techniques. Conversely, for organ-specific examination, ultrasound, optical imaging (OI), and photoacoustic imaging (PAI) are to be preferred when associated with simpler micro-bubble nano-systems (Sijumon Kunjachan et al., 2012). For imaging purposes, the nano-systems formulation in the size range between 5 and 100 nm permits the acquisition of certain imaging information and allows a rapid and high specific contrast enhancement (Kiessling et al., 2014).
More recently, advanced nano-delivery systems have developed to release the cargo from the carrier at the target site in a temporally and spatially controlled manner while minimizing the side effects of the treatment (Moradi Kashkooli et al., 2020). The optimal nanoparticle sizes for drug delivery systems range between 10 and 100 nm, which are to be exploited for enhanced permeation and retention (EPR) effects (in tumors condition) and to avoid elimination in the spleen (Petros and DeSimone, 2010).
In all cases described earlier, either contrast agents or drugs, various triggers, namely, endogenous or exogenous stimuli, can control the kinetic, release, or enhanced imaging. Endogenous factors include changes in pH, electronic balance, and enzyme concentration. They are used for controlling the drug release and biodistribution from nanocarriers and incrementing more treatment activity at the targeted site (Ahmadi et al., 2020). Exogenous factors are physically induced and include temperature, light, magnetic field, and ultrasound. Unlike endogenous stimuli, which are connected more with disease progression stages, external triggers are controlled and less associated with subject variability (Hosseini et al., 2016; Ghasemiyeh and Mohammadi-Samani, 2021). The choice of using a specific stimulus type is made by considering several factors such as; 1) the pre-designed application, 2) the target site, 3) the expanses, and 4) safety concerns. In combination with imaging agents, exogenous or endogenous stimuli could provide improved platforms for advanced imaging, treatment, or theranostics (Sahle et al., 2018; Li et al., 2019). The physical triggers, nano-systems, and biomedical applications discussed within this review are presented in Figure 1.
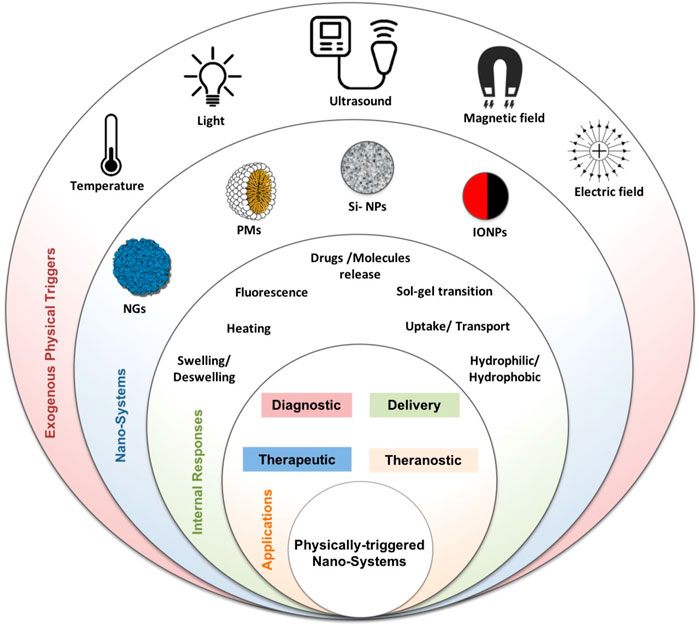
FIGURE 1. Physically triggered nano-systems (PTNSs) schematic image: exogenous physical triggers (temperature, light, ultrasound, magnetic, and electric fields), nano-systems (nanohydrogel (NGs), polymeric micelle (PMs), silica NPs (Si-NPs), iron oxide magnetic NPs (IONPs)), and their internal response and biomedical applications.
Types of exogeneous physical triggers
Temperature is one of the most applied physical triggers. Polymeric systems with a lower critical solution temperature (LCST) mostly undergo a phase transition when they experience temperature above their LCST. In contrast, other polymeric systems that become soluble over heating have an upper critical solution temperature (UCST) (Schmaljohann, 2006a). Thermo-responsive drug delivery systems (DDSs) offer multiple benefits, sometimes by eliminating the urgent demand for invasive surgery and the delivery to hard-access organs (Fitzpatrick et al., 2012; Lencioni and Cioni, 2016).
Light trigger in the form of UV, visible, or near-infrared (NIR), is usually applied as an excitation source. In the case of converting light to heat, known as photothermal therapy (PTT) or photodynamic therapy (PDT), the light source (i.e., NIR) is applied to kill cancer cells (Hashida et al., 2014; Timor et al., 2015; Hu et al., 2012). PDT induces surrounding oxygen molecules to generate cytotoxic singlet oxygen (1O2) or reactive oxygen species (ROS), which will be able to destroy cells (Chen Q. et al., 2015; Chen S. et al., 2015).
Alternating magnetic field (AMF) is another source for designing magneto-responsive DDS or gene delivery systems. Magneto-responsive nano-systems can generate heat upon sensing AMF and can be utilized for magnetic hyperthermia treatment. On the other hand, such particles can be used as contrasting agents to provide the signal-to-noise ratio in magnetic resonance imaging (MRI). Magnetic nanoparticles (MNPs) are a suitable carrier for designing therapeutic systems and have been applied as a diagnostic tool for MRI. MNPs have been applied widely in designing theranostic agents, participating in therapy and diagnosis through MRI (Court et al., 2017).
Sonography is a well-known imaging method based on ultrasound waves created by mechanical oscillations of a piezoelectric material when an alternating current exerts. In the last decades, 3D ultrasound imaging has developed to have three-dimensional images and a better concept of organ volume/area, resulting in an advanced diagnosis of abnormalities in the early stages (Huang and Zeng, 2017).
X-ray computed tomography (CT) is a non-invasive clinical imaging modality that combines X-ray images from different body angles with computer processing techniques and provides valuable anatomical information with high spatial resolution.
In continuous of our previous review articles in the field of pharmaceutics (Farjadian et al., 2018; Entezar-Almahdi et al., 2020; Hoseini-Ghahfarokhi et al., 2020; Zarkesh et al., 2021; Hejabi et al., 2022), in this review, we focus on physically triggered nano-systems (PTNS) with diagnostic, therapeutic and theranostic applications. The PTNS cited in this work spans from polymeric micelles (PMs), nanogels, and silica, to MNPs that are responsive to physical stimulus. Each section presents a specific nano-system, and its applications, as evidenced in the literature; the different mechanisms of action and the specific nano-system response are highlighted when subjected to an exogenous physical stimulus.
In the last 10 years, many preclinical studies were performed on PTNS to develop and optimize stable, precise, and selective therapeutic or diagnostic agents. The systems must meet the requirements of efficacy, toxicity, pharmacokinetics, and safety before clinical investigation.
Interestingly, several PTNS have entered clinical studies, and in this review, we are reporting some examples of PTNS currently under investigation.
In the following sections, we will expand on four types of PTNS. Starting from polymeric micelles and nanogels and we will move into mesoporous silica nanoparticles (MSNs) and MNPs. Conclusions and future outlook present an overall view that PTNS has the potential to be used in medical applications.
Polymeric micelles
Polymeric micelles (PMs) are composed of hydrophilic–hydrophobic segments (amphiphilic diblock, triblock, graft, or either star co-polymers) that are self-assembled in aqueous media. At the same time, their concentration is more than critical micelle concentration (CMC). Some procedures have been applied for micelle production like oil-in-water emulsion, solubilization of the copolymer, and subsequent solvent evaporation, dialysis, and film casting. Variously reported morphologies for PMs are spherical, star, worm, crew-cut, flower-like, unimolecular, toroids, helices, cylindrical, lamellae, and vesicles configurations (Topete et al., 2015).
Micelles encapsulating therapeutic macromolecules have been explored for various diseases due to their ability to enhance drug absorption, control the release of the drug at target sites, and prolong the residence time (Xu et al., 2013). This section discusses temperature, light, and ultrasound-responsive PMs with therapeutic and diagnostic applications.
Polymeric micelles in therapy and diagnosis
The geometrical shapes of PMs depend on external parameters, for example, temperature, solvent, and pH of the medium. These factors significantly affect the length of PMs building blocks. The suitable size of core-corona aggregates of micelles for pharmaceutical applications changed approximately from 10–100 nm (Zhang Y. et al., 2014). Cargos with poor solubility in water can load in the micellar core, and subsequent release can occur through a disintegration procedure. The longer hydrophilic shell (e.g., PEG) extends the micelle stability. It protects the drugs against the external medium, whereas the shorter hydrophobic interior part improves the loading of the lipophilic therapeutic agents such as some drugs, genes, and proteins (Movassaghian et al., 2015; Cabral et al., 2018; Hanafy et al., 2018). Different types of cargo are loaded successfully on the PMs, and their release patterns are investigated (Li N. et al., 2016; Qu et al., 2017). Anticonvulsant drug (clonazepam) (Choi et al., 2006), ophthalmic drugs (e.g., prednisone acetate) (Chang et al., 2008), diazepam (Suksiriworapong et al., 2014), and mainly anticancer drugs, for example, DOX (Panja et al., 2016a), MTX (Tu et al., 2018), PTX (Song et al., 2016), CPT (Meng et al., 2013), curcumin (Yu et al., 2014), cis-Pt (Wang Y. et al., 2017), and ADR (Li et al., 2017). There are different strategies for reaching the drugs to target cells as depicted in Figure 1 (Singh A. et al., 2016). Passive targeting using the EPR effect is the key mechanism and originated from the tendency of nanoparticles (NPs), for example, PMs, for accumulation in tumor cells compared to normal cells. Remaining PMs in tumor tissues for a long time facilitate the sustained release of therapeutic agents into the tumor environment. However, active targeting is based on ligand-mediated targeting and utilizes receptors, for example, FA, HA, carbohydrates, monoclonal antibodies or proteins, and peptides, for example, transferrin, luteinizing hormone, α2-glycoprotein, and aptamer (Amjad et al., 2017; Kesharwani et al., 2018).
Charged drug micelles must be designed to inhibit premature drug delivery before encountering the target cells to develop the therapeutic function and lead to site-specific drug delivery while reducing cytotoxicity. In this regard, the targeting process that applied stimuli-responsive nanocarriers for distinct liberation of the drug in the objective cell environment must be engineered (Joglekar and Trewyn, 2013; Nakayama et al., 2014; Zhou Q. et al., 2018; Li Y. et al., 2018). This involves the manipulation of the PMs to respond to definite physical, chemical, or enzymatic triggers that are distinctive to target cells. Figure 2 represents an operating mechanism of internal (pH, temperature, enzyme, ionic strength, and redox potential) or external stimuli (magnetic field, light, and ultrasound) for intelligent drug delivery of PMs (Biswas et al., 2016). Herein, physically triggered PMs (e.g., temperature, light, and ultrasound-triggered) for therapy and diagnosis are discussed. Examples are mentioned in Table 1, regarding the types of stimuli based on structure, cargo, therapy, diagnosis tools, and in vitro and in vivo assays.
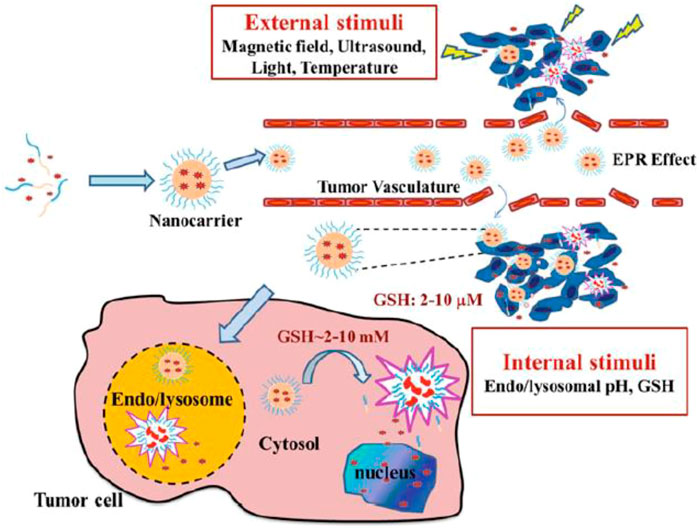
FIGURE 2. Illustrative description of controlled drug delivery of stimuli-responsive PMs. It is reprinted with permission from Springer (Singh A. et al., 2016).
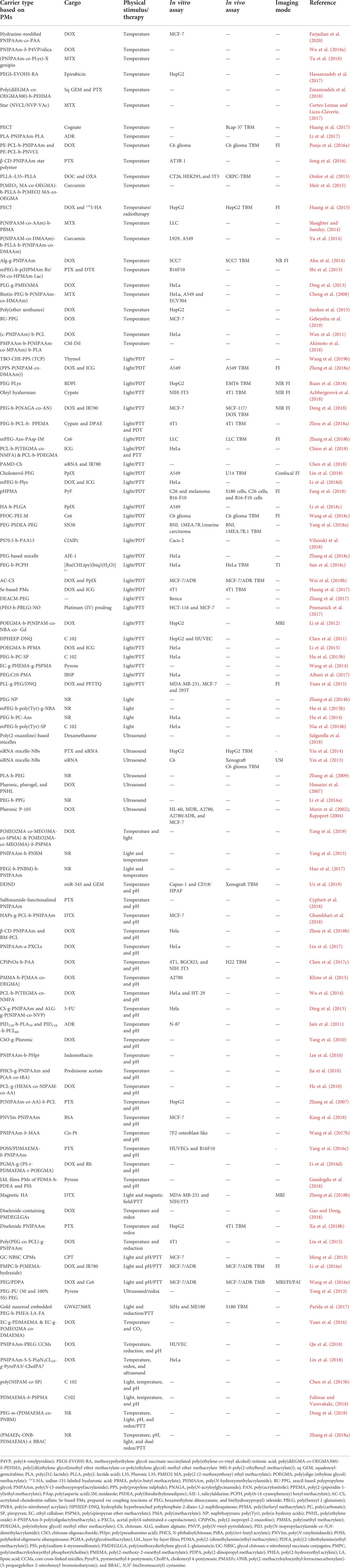
TABLE 1. Physically triggered polymeric micelles concerning the type of stimulus and cargo for in vitro and in vivo investigation, therapy, and diagnosis.
Temperature-triggered polymeric micelles
Temperature is one of the extensively examined stimuli used in DDSs. Throughout heating/cooling operation, temperature alteration prompts the conformational changes of stimuli-responsive PMs to realize temperature-related drug release and intracellular uptake. The structural change of temperature-sensitive PMs induced potential treatment through hyperthermia (Akimoto et al., 2014). The most relevant thermo-responsive co-polymers as drug carriers are PEO–PPO and other polyether amphiphiles, PEO-polyester (e.g., PLA and PCL), and PNIPAAm-based block co-polymers. However, PNIPAAm is extensively studied to engineer thermo-responsive micelles as it displays an LCST of around 32°C near body temperature with sharp phase transition. Above its LCST, PNIPAAm experiences a phase transition from a coiled configuration to a globular configuration. In addition, PHPMA, a highly hydrophilic and biocompatible macromolecule copolymerized with a broad diversity of hydrophobic building blocks, was utilized to produce block co-polymers for the subsequent PMs construction as a substitute for PEG (Sosnik, 2013). Some other LCST-based temperature-responsive polymers as potent drug and/or gene carrier, which have been used so far include, poly(N-vinylalkylamide), poly(N,N-diethylacrylamide), pluronics, tetronics, polysaccharide-, phosphazene, and chitosan-derivatives (Schmaljohann, 2006b).
A new thermo-responsive shell crosslinked nano-system was developed based of PNIPAAm-co-poly(L-lysine) graft co-polymer that is successfully crosslinked with genipin as a natural crosslinking agent (PNIPAAm-co-PLLys)-X genipin (Lai, 2017). MTX was encapsulated on this carrier successfully, and the in vitro drug release profile revealed almost complete drug release after 48 h (Tu et al., 2018). Synthesis of two sets of thermo-sensitive four star-armed PMs based on PNIPAAm or PNVCL was reported using bromine terminated pentaerythritol polycaprolactone (PE-PCL-Br) as a macroinitiator through ATRP. The block co-polymers (PE-PCL-b-PNIPAAm and PE-PCL-b-PNVCL) were conjugated with FA and loaded with DOX to provide DOX-FA-PMs. The cellular uptake and MMT assay study demonstrated effective internalization of these PMs into C6 glioma cancer cells with perfect biocompatibility. The in vivo assay set expressed high prevention of tumor growth in C6 glioma TBM significantly. The location analysis of DOX in the C6 glioma-induced rat model was accomplished through fluorescent imaging (Panja et al., 2016a). Other thermo-triggered drug delivery PMs systems based on PNIPAAm for tumor targeting are prepared through co-polymerization of NIPAAm with other monomers, for example, acrylamide & n-butylmethacrylate (Slaughter and Sunday, 2014) and N,N-dimethylacrylamide (DMAAm) & PLA (Yu et al., 2014) for the production of diblock and triblock copolymers, respectively. Furthermore, alg-g-PNIPAAm (Ahn et al., 2014) and star β-CD-based PNIPAAm (Song et al., 2016) as temperature-responsive PNIPAAm-based PMs for cancer imaging and therapy are developed.
Synthesis of the thermo-responsive micellar-hydrogel system based on poly(CL-co- 1,4,8-trioxa{4.6}spiro-9-undecanone)-b-PEG-b-poly(CL-co-1,4,8-trioxa{4.6}spiro-9-undecanone) (PECT) triblock co-polymer for loading of two therapeutic and diagnostic agents; DOX and 131I isotope and their subsequent co-delivery was developed. Temperature-induced self-aggregation of PECT triblock PMs shows synergetic treatment due to the combined chemoradiotherapy. The results confirmed that PECT could be recognized as a potent agent in the co-delivery of DOX and 131I, in which the system is diagnosable through fluorescent imaging (Huang et al., 2015).
Block copolymers containing aromatic moieties through polymerization of N-(2-benzoyloxypropylmethacrylamide (HPMAm-Bz) or naphthoyl analog (HPMAm-Nt), with N-(2-hydroxypropyl) methacrylamide monolactate (HPMAm-Lacy) via a PEG-based macro-initiator were synthesized [mPEG-p(HPMAm-Bz/Nt-co-HPMAm-Lacy)]. These PMs were loaded with anticancer drugs, PTX and DTX, and their temperature-sensitive release profile was probed. The authors designed these thermosensitive PMs to show that the π–π stacking consequence developed by aromatic functionality improves the loading capacity and stability of their DDSs (Shi et al., 2013). Random poly(ether urethanes) PMs were synthesized with tuneable LCST from 30 to 70°C by changing the PEG content. The results demonstrated accelerated DOX release from the nanocarrier above the LCST (Sardon et al., 2015). Preparation of a novel thermosensitive system of poly(L-glutamate) (PLG) grafted on 2-(2-methoxyethoxy) ethyl methacrylate (MEO2MA) or 2-(2-(2-methoxyethoxy)ethoxy) ethyl methacrylate (MEO3MA) via click reaction was reported. These “hairy-rod” polypeptides were self-assembled into PMs for loading and releasing of DOX (Ding et al., 2013). Recently, a mini-library of temperature-sensitive six arms star polymers by using xanthate RAFT-agent with hexa functionality and N-vinylcaprolactam (NVCL), N-vinylpyrrolidone (py), and vinyl acetate (VAc) monomers through RAFT polymerization was developed. Star polymers of PNVCL with variation in homopolymeric arms were created. The aggregation behavior of PMs and their solubility were related to the block order in the arms of star polymers. Interestingly, these libraries of thermo-responsive six arms star polymers displayed dissimilar operations in MTX encapsulation and delivery (Cortez-Lemus and Licea-Claverie, 2017).
Light-triggered polymeric micelles
UV, visible, NIR, or X-ray as external stimuli cause structural changes in photo-responsive PMs via light-induced reactions tailored by light intensity and wavelength and result in the drug release from PMs (Alatorre-Meda et al., 2013). Light responsive PMs are designed by incorporating chromophores within the core or shell of a micelle or at the interface of micelles’ core-corona. The most applied photochromic compounds are azobenzenes and their derivatives, as they can perform a reversible trans–cis isomeric transformation. Other reported chromophores in light-sensitive micelles are o-nitrobenzyl, coumarin, stilbene, dithienylethene, and DNQ (Movassaghian et al., 2015).
PDT is a favorable treatment based on applying a photosensitizer and light of a particular wavelength for controlling a diversity of tumors. The photosensitizer transforms accessible oxygen to highly ROS in the presence of light irradiation and persuades an intra-tumor cytotoxic response. Hyaluronic acid-b-poly(lactide-co-glycolide) (PLGA/HA) was synthesized following the loading of PpIX as an effective photosensitizer. PLGA/HA-PpIX micelles have considerable capability for in vitro CD44-targeted PDT treatment toward A549 cells (Li X. et al., 2018). Preparation of light-responsive fluorinated polymeric micelle based on perfluorooctanoic acid (PFOC) and branched PEI-loaded photosensitizer Ce6 was developed for PDT and fluorescent imaging. The high efficiency of Ce6-PFOC/PEI in PDT cancer treatment is related to the oxygen-carrying potential of perfluoroalkyl functionalities that supply oxygen and overcome the hypoxia in tumor cells under the conditions of low oxygen content (Wang Q. et al., 2018). A recent report on PMs nanoparticles for PDT treatment is based on the preparation of toluidine blue O-chitosan-poly(propylene sulfide) (TBO-CS-PPS; TCP), following the thymol loading on TCP and subsequent binding to the bacterial biofilm effectively. T-TCP micelles produced ROS by PPS oxidation, triggering thymol delivery, and disrupting biofilm under light irradiation (Wang Z. et al., 2019).
Recently, a system based on light-sensitive micelle plexes NPs was developed for photothermally enhanced delivery. The micelle plexes were produced from poly(amido amine) s modified cholesterol and CXCR4 molecule inhibitor (PAMDCh). NIR dye/IR780 was loaded in cationic PMADCh, which were applied to form IR780@micelle/siRNA polyplexes. Upon laser irradiation, the photothermal effects of IR780 resulted in the disruption of endosomal membranes and facilitated endosomal escape while promoting siRNA transfection (Chen et al., 2018). Synthesis of a set of amphiphilic block co-polymers comprised methyl PEG-b-PLys (mPEG-b-PLys) with urethane, urea, and thiourea functional groups in their structures to induce hydrogen bonding was disclosed. Subsequent encapsulation of anticancer drug, DOX and photothermal agent, and ICG in PMs provided mPEG-b-PLys@DOX-ICG. Constructive effects of strong H-bonding among the inner hydrophobic segment of PMs and at the same time between the hydrophobic parts and drugs include reducing CMCs, increasing micelle stability, improving the drug loading capacity, declining the size of the micelle, and decelerated drug release pattern, respectively. The ICG photothermal effect triggered destabilization of H-bonding and DOX release and considerable enhancement of cytotoxicity under NIR laser irradiation (Li Y. et al., 2018). A novel UV-responsive PMs comprised coumarin ester was announced for killing tumor cells in vitro without loading any drugs. (7-diethylaminocoumarin-4-yl)methyl (DEACM) carbonate was chosen as the photo-sensitive group and conjugated with PEG to PEGylated DEACM. Photolysis of DEACM upon UV irradiation can produce carbon dioxide bubbles during micelles degradation. Indeed, in vitro, multiple tumor ablation was induced without any anticancer drug through 8-s UV exposure (Zhang et al., 2017).
An innovative oxygen-independent photothermally triggered system combined PTT and PDT was developed by self-assembling PEG-b-PCL-b-poly(2-(piperidin-1-yl)ethyl methacrylate) (PEG-b-PCL-b-PPEMA) triblock co-polymer and subsequent co-encapsulation with DPAE (diphenylanthracene endoperoxide) and cypate as a fluorescent dye. By NIR irradiation at tumor cells, the PMs induced efficient hyperthermia by cypate through PTT. They triggered large intracellular singlet O2 production by DPAE through PDT via local photothermal consequence in the interior cores of PMs (Zhou Q. et al., 2018). Another system that applies PTT and PDT combination is a mixed micelle based on the co-assembly of PCL-b-poly(methoxytri(ethylene glycol) methacrylate-co-N-(2-methacrylamido)ethyl folate amide) (PCL-b-P(TEGMA-co-NMFA) and PCL-b-poly(diethyleneglycolmonomethylether methacrylate) (PCL-b-PDEGMA), which is targeted with folate receptor and encapsulated ICG. Upon NIR irradiation, the toxicity induction by the micelle@ICG has effectively inhibited the growth of HeLa cells (Chien et al., 2018).
Ultrasound-triggered polymeric micelles
Using localized ultrasound (US) waves as an external physical stimulus for controlling the drug release from ultrasound-sensitive polymer micelles has achieved growing interest due to ultrasound’s safety, inexpensiveness, and non-invasive nature (Awad et al., 2021). In addition, the US is remote management and a non-contact process. This method can be additionally refined by modifying several characteristics containing the properties of the PMs, the interval time of PMs’ exploitation and US utilization, the type of US wave, and the sonication frequency (Entzian and Aigner, 2021).
Five amphiphilic diblock and triblock co-polymers were produced using hydrophilic and hydrophobic oxazoline-based segments. The synthesized PMs were encapsulated with dexamethasone, and their spontaneous and US-mediated release profile was investigated. The results demonstrated that the US intensifies the amount of dexamethasone release by 6%–105% with due attention to the copolymer type, dexamethasone loading on the carrier, and the time, location, and intensity of stimulation (Salgarella et al., 2018).
An engaging system of siRNA micelle-nanobubble (NB) complexes was designed based on gene delivery for tumor therapy. NB was selected as a promising nanocarrier due to the prosperous production of NBs accompanied by its US sensitivity and the potential for passive accumulation in tumor cells. SiRNA micelle-NB was prepared by the interaction of siRNA micelles with a positive charge based on mPEG-b-PLys diblock co-polymer and gas-cored liposomes with a negative charge. The US-mediated siRNA transfection, which gives rise to surpassed therapeutic operation and cancer cell apoptosis, was investigated in vitro and in vivo. Furthermore, NB diagnosable material in this system has successfully resulted in extravascular ultrasonic imaging (Yin et al., 2013). Later, the co-delivery of siRNA and PTX with this system was reported because of the synergistic result of the two therapeutic agents. Tumor growth was impeded by applying a low amount of PTX in animal models bearing human HepG2 xenograft (Yin et al., 2014).
Multi-triggered polymeric micelles
Nowadays, designing novel polymeric micelles with the capability of dual or even multiple responsiveness is of great interest. PMs systems with dual responsiveness with at least one physically trigger are dual thermal/light-responsive (Tang et al., 2016; Yang et al., 2019), dual thermal/pH-responsive (Zhang et al., 2007; He et al., 2010; Jia et al., 2010; Lee et al., 2010; Yang et al., 2010; Jain et al., 2011; Ding et al., 2013; Wu et al., 2014; Khine et al., 2015; Chen et al., 2017c; Zhou Z. et al., 2018; Ghamkhari et al., 2018; Uz et al., 2019), dual light/magnet-responsive (Zheng S. et al., 2018), dual thermal/redox-responsive (Liu et al., 2015; Xu J. W. et al., 2018; Gao and Dong, 2018), dual light/pH-responsive (Meng et al., 2013; Li Z. et al., 2016; Wang T. et al., 2016), dual ultrasound/reduction-responsive (Tong et al., 2013), dual light/reduction (Parida et al., 2017), and dual thermal/CO2-responsive (Yuan et al., 2016). Triple-triggered PMs are thermal/reduction/pH-responsive (Sun et al., 2018b; Qu et al., 2018), thermal/ultrasound/reduction-responsive (Lin et al., 2018), and light/temperature/pH- responsive (Falireas and Vamvakaki, 2018). Even quadruple temperature, pH, redox, and UV light (Dong et al., 2018), quintuple light, temperature, pH, and dual redox stimuli-responsive PMs (Zhang K. et al., 2018) are synthesized and applied in the controlled delivery of therapeutic cargos.
Systems with dual photo-responsive and temperature-responsive characteristics are created by adding chromophore functional groups to the thermo-responsive systems (Belmonte et al., 2020). Recently, the dual thermal/light-responsive PMs by the preparation of random and block co-polymers comprised light-sensitive spiropyran, and oligo (ethylene glycol) methylethermethacrylate was reported. UV light irradiation and heating of the sample stimulated increased drug release (Yang et al., 2019).
Combining pH-responsive co-polymer with a photosensitizer was established to overcome the drug resistance in targeted tumor therapy through chemo-PTT. Light-sensitive o-nitrobenzyl succinate (NBSC) that was grafted onto glycol chitosan (GC) and then subsequent crosslinking with glutaraldehyde (GA) lead to the formation of the dual light/pH-responsive-crosslinked PMs (CPMs). Fast release of CPT was observed during light irradiation at low pH (Meng et al., 2013). A multitasking micelle for combinational photo/chemotherapy displayed MR, fluorescence, and PA multimodal tumor imaging was developed. The micelle was composed of a diblock copolymer PEG-b-poly (tertbutylmethylacrylate-co-hydroxylmethacrylate), photosensitizer Ce6, Gd3+ as MR T1-weighted MR agent, and prodrug DOX. Upon NIR laser irradiation, the micelle induced ROS creation and local heat production for PDT and PTT and diagnosed via PAI. In addition, the micelle could construct a magnetic resonance signal at an acidic medium to implement MRI (Wang T. et al., 2016).
Among dual responsive PMs, thermal/pH-responsive is the most studied one. The most recent report is the dual delivery nanoscale device (DDND) based on a pentablock copolymer system composed of amphiphilic pentablock copolymers based on Pluronic ® F127 copolymers and various amine-containing methacrylate monomers for combined delivery of microRNA (miR-345) and GEM (Uz et al., 2019).
Dual thermal/pH-responsive supramolecular micelles were designed from star polymer β-CD-PNIPAAm and benzimidazole terminated PCL (β-CD-PNIPAAm and BM-PCL). The delivery of DOX from supramolecular PMs was accelerated at low pH at 37°C (Zhou Z. et al., 2018). Another thermal/pH-responsive PMs was introduced based on four-armed star-like PMs that were prepared from hyperbranched aliphatic polyesters (HAPs)-g-PLC-b-PNIPAAm block co-polymer. The first block (PCL) is prepared via ROP, and the chain extension with the second block (PNIPAAm) is performed via RAFT polymerization. DTX was loaded on these biodegradable PMs with high encapsulation efficiency, and the release rate was controlled via pH and temperature (Ghamkhari et al., 2018).
A creative quadruple responsive copolymer based on PEG-ss-poly (DMAEMA)-co-poly (2-nitrobenzyl methacrylate) [PEG-ss-(PDMAEMA-co-PNBM)] comprising disulfides unit was developed. Self-assembling this copolymer generates PMs with hydrophobic PDMAEMA-co-PNBM core and hydrophilic PEG coronas that are multi-responsive to temperature, light (UV), pH, and reduction (via the presence of dithiothreitol, DTT). The in vitro release profile was investigated by the use of hydrophobic NR drug. Each stimulus has a specific effect on these multi-responsive PMs; as the temperature changed the size of the micelle, the micelle swelled in low pH, a few amounts of DTT disarranged the micelle configuration, and irradiation of UV light caused the dissolution of the micelle construction (Dong et al., 2018).
Nanogels
The term “nanogels” (NanoGel™) is used to define nanosized particles (10–100 nm) formed by crosslinked polymer networks, which are able to swell in a suitable solvent and absorb large quantities of water (Molina et al., 2015; Shah et al., 2020).
Crosslinks are essential for nanohydrogel structural stability because they prevent polymer chain dissolution in the aqueous environment. NGs, also called hydrogel nanoparticles, are found in a wide variety of applications in biomedical fields, such as DDS and bioimaging (Sasaki and Akiyoshi, 2010; Lu et al., 2010; Mauri et al., 2021).
The recent development of different preparation techniques made it possible to regulate the essential final parameters such as size, shape, and yield. NGs are prepared by various methods of co-polymerization via reaction among hydrophilic monomers and difunctional cross-linkers or physical cross-linking agents (Mauri et al., 2021).
Traditional uncontrolled free-radical polymerization in the presence of a cross-linker combines the two processes of polymerization and crosslinking in one reaction (Neamtu et al., 2017). NGs have emerged as a platform to encapsulate versatile therapeutic agents within their networks to be applied for therapeutic applications (Soni et al., 2016b; Saracoglu and Ozmen, 2021). This section comprehensively discusses external stimuli-responsive NGs and their current applications in biomedical fields.
Nanogels in therapy and diagnosis
The most important NGs’ features are ease of preparation, biocompatibility, degradability, swelling in aqueous media, the high adsorption capacity of therapeutic agents, small particle sizes, electromobility, and colloidal stability (Ghasemiyeh and Mohammadi-Samani, 2019).
NGs are good carriers for DDS due to their specific properties (Soni et al., 2016b; Mohammadi M. et al., 2020):
• The small particle size and easy surface manipulation inhibit rapid phagocytic cell clearance and promote drug targeting by passive and active strategies.
• Controlled and sustained drug release properties at target sites enabled them to promote activity tasks and reduce other side effects.
• High drug loading potent without chemical reactions is suitable to preserve drug activity.
• Tiny particles’ volume allows them to penetrate into a particular tissue (e.g., tumor site) through the paracellular or transcellular passages (Gonçalves et al., 2010).
• Both types of drugs (hydrophilic and hydrophobic) could be entrapped in NG networks.
However, using expensive techniques for preparing some NGs and removing solvents and surfactants from final products are disadvantages of such systems (Zhou et al., 2020).
NGs can be classified as non-responsive and stimuli-responsive. Non-responsive NGs swell consequently to the water absorption. In contrast, responsive NGs can swell/deswell after exposure to environmental changes (such as temperature, pH, light, electric field, ultrasound, or magnetic field (Lim et al., 2014).
As a powerful nanoscale candidate for DDSs, the capability of NGs for loading and carrying medications has been explored by many researchers (Soni et al., 2016a).
According to the chemical composition of the stimulus-responsive NGs, they begin to swell or shrink followed by releasing the cargo as the stimulus is switched “ON.” Therefore, the external stimulus can control the release profile of the therapeutic agents locally and yield a higher effective treatment (Figure 3). NGs are also used as a carrier for imaging probes and contrast agents. Small molecules based on gadolinium (Gd) and manganese (Mn) are introduced in nanohydrogels to induce contrast in MRI (Soleimani et al., 2013; Gallo et al., 2020). Lux et al. (2015) developed a copper-64 cross-linked NG for PET radiotracers of tumors and metastases. Furthermore, hybrid systems based on nanohydrogels and inorganic NPs (quantum dots and AuNPs) are being developed for optical imaging purposes (Molina et al., 2015).
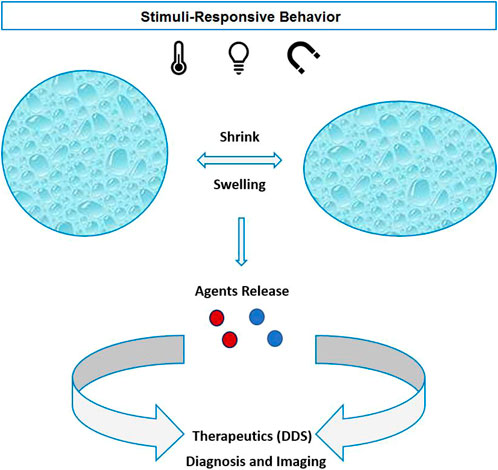
FIGURE 3. Physical stimuli-responsive nanohydrogels with shrink/swelling behavior and active agents released for therapeutics or diagnostic purposes.
Currently, just a few NGs formulations for subcutaneous delivery have reached clinical trials (Kitano et al., 2006; Kageyama et al., 2013; Saito et al., 2014; Jiang L. et al., 2020) because some parameters have to be optimized, such as rapid spleen clearance, the surface charge that influence opsonization, degradation kinetics, and burst drug releases (Soni et al., 2016b).
This section reviews the NG-based drug carriers responding to physical factors. Table 2 summarizes recent studies in this field.
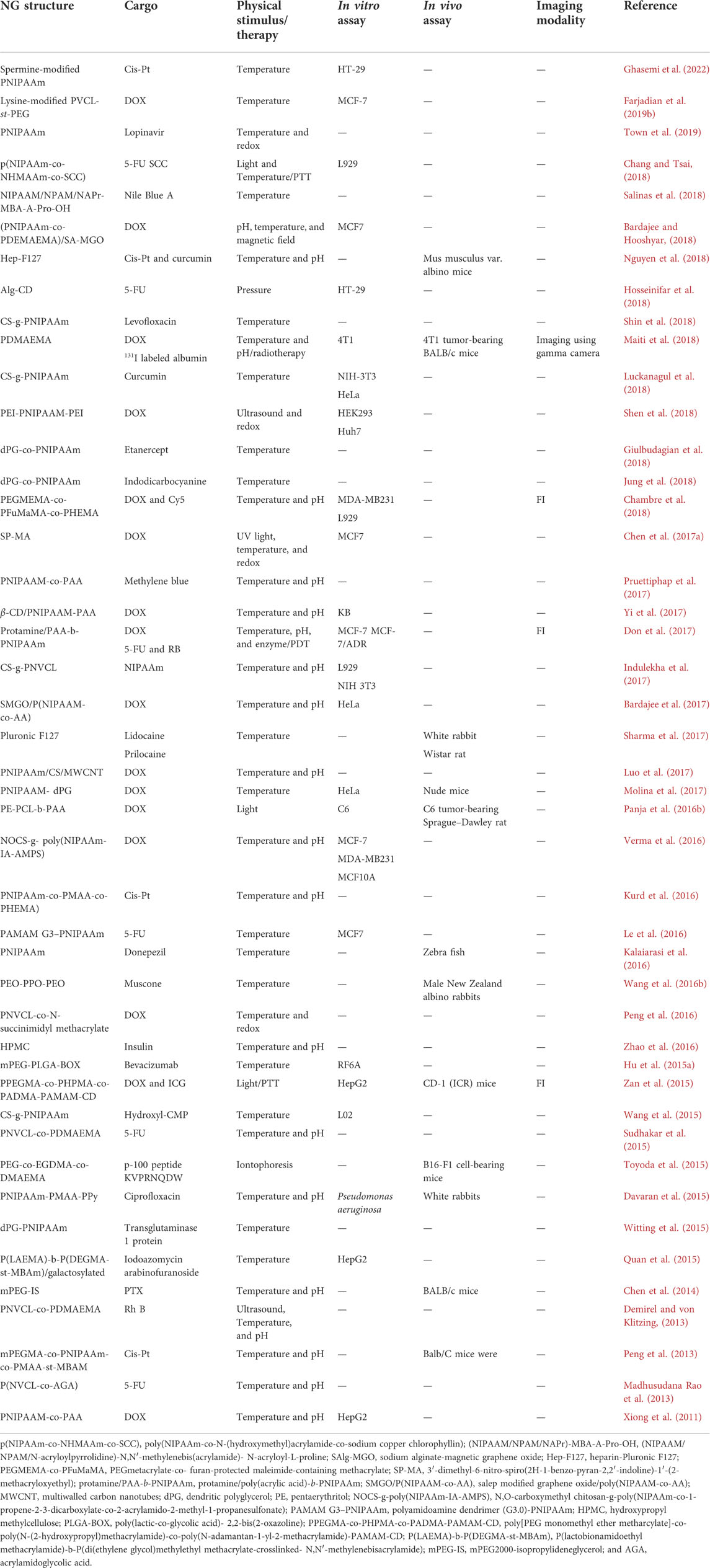
TABLE 2. Physically triggered NGs concerning the type of stimulus and cargo for in vitro and in vivo investigation, therapy, and diagnosis.
Thermo-responsive nanogels
Thermo-responsive NGs can be classified into two main groups (Hosseini et al., 2016; González-Ayón et al., 2020). In one type of such NGs, the size increment could be occurred by elevating the temperature; in the other one, the NGs are shrunk beyond the volume phase transition temperature (VPTT). As a remarkable precursor for synthesizing thermal responsive compositions, PNIPAAm is in the spotlight of such studies due to the LCST (i.e., 32°C) close to the human body temperature (Priya James et al., 2014). Exploiting the dependency of PNIPAAm phase transition to the temperature tolerance, many NG-based DDSs have been developed and extensively investigated for drug-loading cytotoxicity and biodegradability assessment and temperature-dependent releasing profile (Le et al., 2016; Luo et al., 2017; Bardajee and Hooshyar, 2018; Yang Q. et al., 2018; Chang and Tsai, 2018; Town et al., 2019).
As a theranostic multi-responsive NGs, PDMAEMA was synthesized to load chemotherapy drug (DOX) and radioisotope (131I) labeled albumin simultaneously. The NGs showed a proper responsivity to temperature and pH so as it was in a solution form at room temperature while transformed into the gel near body physiological temperature. Moreover, the drug release accelerated in tumor cells due to pH effect. Surprisingly, the nanocarrier exhibited a biodegradable manner at 37°C and pH of 5.8. Furthermore, MTT assay on 4T1 cells revealed that it could be biocompatible and cause significant growth inhibition by sustained drug release. Administration of PDMAEMA gel @131I-BSA/DOX in BALB/c mice with 4T1-induced murine breast cancer showed excellent tumor growth inhibition among the other groups. In addition, gamma imaging showed remarkable retention of 131I-BSA in the tumor about 48 h after injection (Maiti et al., 2018).
Recently, HIV drug lopinavir was loaded in a polymeric NG (PNIPAAm) prepared in different sizes (Town et al., 2019). The injectable NG disclosed a dual responsive behavior with changes in temperature and ionic strength, which promise it as an intelligent carrier with a high potential of loading and releasing the drug. A multi-responsive NG was designed to achieve more effective drug release by copolymerizing PNIPAAm and PDMAEMA containing sodium alginate and magnetic GO. Exposure to various temperatures and pH and the presence of a magnetic field affected the DOX release rate. The cytotoxicity test on MCF-7 cells indicated desirable biocompatibility for the synthesized NG (Bardajee and Hooshyar, 2018). Another nano-platform conjugated with NOCC was developed so that the DOX release was dependent on temperature. The viability tests unveiled that the MCF-7 and MDA-MB231 tumor cells are more killed than MCF10A normal cells.
Moreover, they observed cell cycle arrest triggering apoptosis death in MCF-7 cells by the nano-carriers (Verma et al., 2016). Poly (NIPAAM-MAA-VP) was used as a nanocarrier with dual responsiveness to thermal/pH stimulation to release ciprofloxacin’s antibacterial agent. It showed a substantial result in animal models (Davaran et al., 2015).
Thermo- and pH-responsive NG delivery system based on lysine modified-polyvinylcaprlactam (PVCL) conjugated with DOX was established and showed high uptake in the MCF-7 cell line. The synthetic strategy depicted in Figure 4 was based on RAFT through copolymerization of PVCL with PEG diacrylate to form PVCL–PEG. Then, PVCL–PEG was modified with L-lysine aminoacid to form PVCL-Lys, which has thermo sensitivity around 38°C and an amino-free site for conjugation of DOX (Farjadian et al., 2019b). Finally, in a pH-responsive linkage, Schiff base reaction, DOX was linked to NG and formed PVCL-DOX.
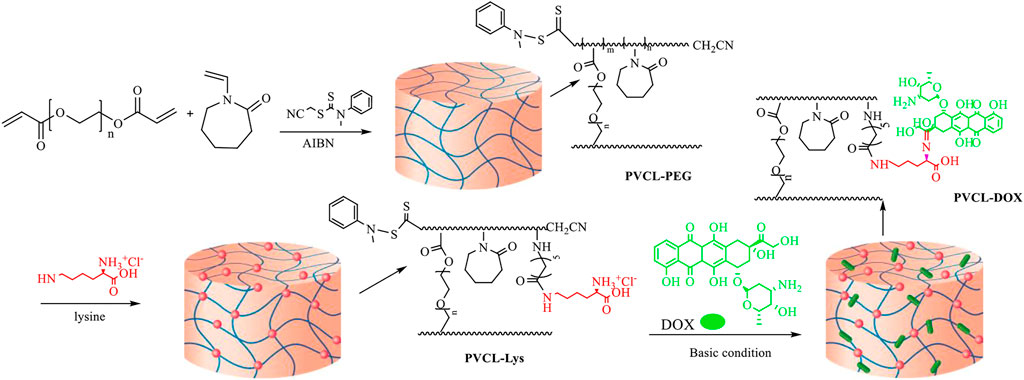
FIGURE 4. Synthetic strategy in forming PVCL-DOX NG (Farjadian et al., 2019b). No permission is required.
Light-responsive nanogels
Taking advantage of light penetrating the materials, the light-responsive DDSs have become the spotlight of many studies in the field of drug delivery (Jiang Z. et al., 2020). For instance, PPEGMA-co-PHPMA-co-PADMA-PAMAM-CD NG was fabricated to load with ICG and DOX, exhibiting strong NIR-light sensitivity. Using PTT and chemotherapy simultaneously leads to effective results in in vitro and in vivo assays (Zan et al., 2015). In another research, laser light-triggered DOX release from PE-PCL-b-PAA NG was studied. The NG has a significant destructive effect on the proliferation of C6 glioma cancer cells and an inhibition effect on tumor growth of C6 tumor-bearing Sprague–Dawley rats (Panja et al., 2016b). Recently, by merging the synergistic effect of hyperthermia and drug release from a thermo-sensitive NG, a notable cell killing was achieved. The p(NIPAAm-co-NHMAAm-co-SCC) NG was loaded with 5-FU and sodium copper chlorophyllin (SCC), which produces heat exposure to green light (Chang and Tsai, 2018). Isomerization of hydrophobic spiropyran to hydrophilic merocyanine due to UV light exposure caused swelling up the SP-MA NG. In addition, the authors claimed that MCF-7 cell killing increases after treatment with UV-irradiated DOX-loaded NG (Chen S. et al., 2017). In a study combination of GO as a photothermal agent, DOX and HA led to a novel NG, making it a multi-purpose carrier. The elevation of temperature due to absorbing NIR light by GO can enhance DOX release from NG. The platform showed acceptable biocompatibility on MDCK cells, while an effective killing trend on the A549 cell line (Khatun et al., 2015).
A novel type of transdermal microneedle arrays made of alginate hydrogel with PLA and the peptide-nucleic acid coating was applied for mRNA sampling from skin interstitial fluid. Microneedles were removed from skins, and in a UV-triggered release process, the adsorbed mRNA was released and detected. This technology enables the detection of mRNA-based biomarkers and could be recognized as a specific tool in personalized medicine (Al Sulaiman et al., 2019). A light-responsive transdermal delivery system based on PHEMA and PEG dimethacrylate nanohydrogel was also applied for Ibu delivery (Hardy et al., 2016).
Other stimuli-responsive nanogels
Owing to the interesting properties of ultrasonic waves like bio-safety, penetration in soft tissue and controllability, it has been utilized as a powerful tool for stimulating DDSs. Loading of perfluorohexane (PFH) on PEI-PNIPAAM-PEI NG resulted in an ultrasound-responsive structure that releases DOX immediately after cavitation induced by ultrasonic waves. The DOX-loaded NG showed comparable cytotoxicity on HEK293 and Huh7 cell lines compared to free DOX. It also demonstrated more cell growth inhibition in tumor cells than in normal ones (Shen et al., 2018).
Improving wound healing and increased therapeutic gain for frostbite were reported after treatment of rats’ skin with GLT NG containing triterpenoids drug in combination with ultrasound waves (Shen et al., 2016). Pressure as another physical tool to stimulate nano-hydrogel to release anticancer drug (5-FU) was used on Alg-CD nano-carriers, in which the mortality rate of colon cancer cells (HT-29) was elevated for 5-FU-loaded Alg-CD NG in comparison with free 5-FU (Hosseinifar et al., 2018). Iontophoresis using a small electric current has proven as a non-invasive method to enhance transdermal drug delivery. To overcome the low accumulation of antigens in the epidermis, NGs containing gp-100 peptide KVPRNQDWL were developed. The anticancer effect of the NG was explored on B16-F1 cell-bearing mice in combination with iontophoresis where the tumor growth was significantly suppressed by the treatment method (Toyoda et al., 2015).
Liposomes for delivery of therapeutic and imaging agents
Among the other nanostructures to deliver the cargo to the desired site, liposomes are one of the first and most investigated nanocarriers due to their biocompatibility, low side effects or cytotoxicity, ease in biodegradation under physiological conditions, and desired properties in loading and delivery of the entrapped cargo. The spherical shape of liposomes formed by the bilayer of lipids allows them to mimic the cell membrane and load both hydrophobic and hydrophilic drugs (Bangham and Horne, 1964). Due to the different extravasation behavior of various types of tumors, the passive release of the drug by liposomes proved a low performance, and many studies have extensively investigated the potential of liposomes to respond to physical stimulus sources to release the drugs in a time- and location-dependent manner (Ta and Porter, 2013). Once liposomes carrying drugs accumulate at the desired site, external sources like hyperthermia or light generators focusing on the site can trigger the drug release process via the disordering of lipid shells of the liposomes.
Thermo-responsive liposomes
Using particular physical sources to increase the temperature above the physiological condition but not more than 42°C converted mild hyperthermia into a powerful tool for targeting drug delivery purposes. As the sources can act selectively in ON/OFF modes during a predefined interval and irradiate in a user-adjusted location, they have shown promising results in combination with chemotherapy agents (Franckena et al., 2007). Hyperthermia would enhance the therapeutic effect by the increase in blood flow and expansion of vascular pores to improve the extravasation of liposomes into the tumor as well as by exploiting the thermo-responsive property of liposomes to release the drugs more effectively (Huang et al., 1994; Kong et al., 2000b). According to the literature, thermo-sensitive liposomes are classified into three main categories: 1) traditional thermo-sensitive liposomes (TTSL), 2) lysolipid-containing thermos-sensitive liposomes (LTSL), and 3) polymer-modified thermo-sensitive liposomes (PTSL).
Considering the phase transition from gel to liquid, Yatvin et al. (1978) developed the first version of TTSLs, in which the liposome starts to melt at the transition temperature (Tc) while experiencing heating (Figure 5). Although they showed the great potential of liposomes to respond to hyperthermia, they owned a low amount and rate of neomycin release. The issue was addressed by introducing the other lipids to the liposome structure, which leads a 100 times greater release rate at 44°C than 37°C (Bassett et al., 1986; Gaber et al., 1995). In another study, it has been shown that dFdC (pyrimidine analog Gemcitabine) is effectively released from the liposome at 43°C compared to 37°C (80% vs. 20%) (Limmer et al., 2014).
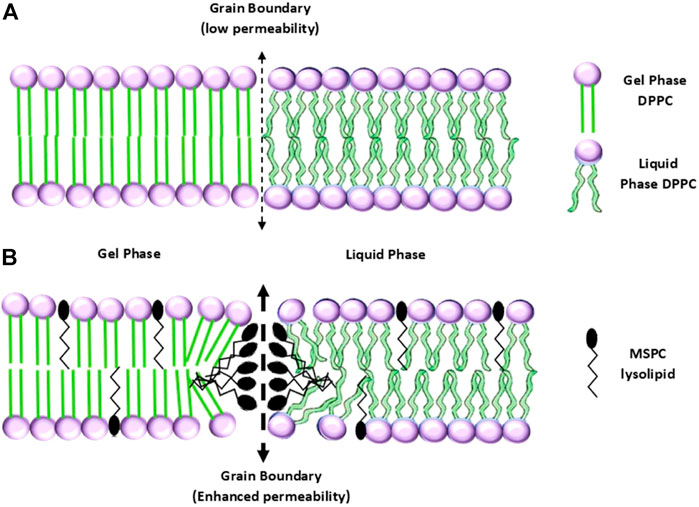
FIGURE 5. (A) Configurations of a TTSL (up) and an LTSL (down) before, and (B) after the transition from gel to liquid phase (Abuwatfa et al., 2022). No permission is required.
As for in vitro and in vivo studies, the vinorelbine-containing liposomes (Thermo-Vin) were designed and investigated at 37°C or 42°C (using an RF generator) on H22 cancer cells. The in-vitro study exhibited that both Free-Vin and Thermo-Vin groups significantly decreased the H22 cell viability after 24 h. Moreover, the accumulation of the drug into the tumor was 15 times greater for the Thermo-Vin+RF group than the Free-Vin one. The Kaplan–Meier analysis showed that the Thermo-Vin+RF group owned the longest mice survival among all groups (Wang S. et al., 2016). Another study investigating the capability of TTSLs to carry dual drugs of DOX and vincristine proved that the liposomes released around 85% of both cargos under 42°C temperature only in the first 5 min, while at 37°C the amount was less than 10% even for 30 min heating (Li M. et al., 2016). Another thermo-sensitive liposome carrying DTX (DTX-TL) showed a release amount of 40 and 15% for 42 and 37°C, respectively. The tumor growth analysis unveiled that the human breast tumor-bearing mice treated with DTX-TL had the greatest inhibitory impact on the tumors (Zhang H. et al., 2014).
As discussed in many studies, the TTSLs are stimulated under long-time exposure to high temperatures, in which healthy tissue necrosis may occur. To overcome the shortage of high thermal doses by TTSLs, the concept of incorporating lysolipids or thermo-sensitive polymers into the liposomes was proposed. In this regard, the modified liposomes release the drug under mild hyperthermia (39–42°C) in a burst manner, allowing the liposomes to be more clinically applicable (Abuwatfa et al., 2022).
The idea of modifying thermo-responsive liposomes using lipids with single-cyl chains-lysolipids dates to 1999 by Anyarambhatla and Needham (1999). The lysolipids give rise to a lower Tc while triggering a rapid release of the drug due to their accumulation at the boundary and making stable pores (Figure 5) (Landon et al., 2011). For example, the incorporation of a lysolipid into liposomes decreased the Tc from 43 to 40°C, while a half amount of the drug was rapidly released after 20 s of heating. In addition to taking advantage of falling below the necrosis threshold, the LTSL exhibited a promising in vivo result in prohibiting tumor growth (Needham et al., 2000). In another study, it has been reported that LTSL-containing DOX in combination with hyperthermia has a significant therapeutic impact on the FaDu human tumor-bearing mice. The highest accumulation of DOX in the tumor was attributed to the group treated with LTSL under 42°C heating (Kong et al., 2000a). At the clinical trial level, ThermoDOX is the only thermo-sensitive liposomal product based on lysolipid, which underwent clinical trials (HEAT trials) (Bulbake et al., 2017). At this time, the ThermoDOX is used in ongoing clinical trials in combination with focused ultrasound (PanDOX: NCT04852367) and magnetic resonance-guided high-intensity focused ultrasound (HIFU) (de Maar et al., 2020).
Since, in the biological environment, LTSL suffers the gradual desorption of lysolipids from the liposome shell (Ta et al., 2010; de Smet et al., 2011), incorporating thermos-responsive polymers into the liposomes is another approach to bring transition temperature below the thermal necrosis threshold (Bi et al., 2019). Once the thermo-sensitive polymer experiences a temperature over LCST, it onsets to shrinkage and dehydration, which causes disorder in the integrity of the liposome shell. It releases the entrapped drug. As an early work, hydrophobic PNIPAAm was integrated into the liposome structure by Ringsdorf et al. (1991). For another study, modification of DPPC liposomes with p(NIPAAm-co-AAm) led to a synergistic effect in the release of DOX at 40°C compared to the unmodified version (65% vs. 40% drug release). The combination of NIPAAm and AAc followed by incorporation into the liposome yielded a PTSL that released 65% DOX within 5 min of hyperthermia exposure (39°C). In comparison, at physiological temperature, the PTSL prevents around 90% of the drug from being released (Han et al., 2006). In addition, the impact of a DOX-loaded PTSL in combination with MR-guided focused ultrasound was investigated in vitro on MCF-7 breast cancer cells and in vivo on tumor-bearing Fischer rats. The study showed a great potential of the PTSL as a thermo-sensitive drug carrier (Ta et al., 2014). In a recent study, it has been proven that P(NIPAAm-DMAAm)-DSPE integrated into liposomes released a higher amount of PTX at 40°C compared to unmodified liposomes. The PTSL showed low cytotoxicity while carrying the drug. They reduced the viability of A549 lung cancer cells (Xi et al., 2020).
Light- and ultrasound-responsive liposomes
The other physical stimuli sources, rather than hyperthermia generators, aid the liposomal nanocarriers to release their content effectively inside the target on-demand without inducing intense side effects like thermal necrosis. These types of physical sources, such as light or magnetic fields, can penetrate the deep-sited targets and stimulate the liposomes, whereas minimally involving the tissues passed through by them (Weissleder, 2001). The liposomes sensitive to these types of physical sources undergo either immediate structural changes or indirect membrane disruption due to the thermal reaction to the source. Generally, liposomes react to electromagnetic waves via two mechanisms of photophysical and photochemical activations (Leung and Romanowski, 2012).
During the photo-physical mechanism, the liposomes lose their membrane integrity due to photo-thermal conversion without any chemical changes. Indeed, the energy of photons emitted by the light source is absorbed and converted to thermal/mechanical energies, which lead to the disordering of the liposome shell and the release of content (Chen et al., 2020). The photo-absorbers mediating the photo-thermal process include the molecular absorbers and plasmon resonant nanoparticles.
The lipophilic molecular absorbers could be integrated into the liposomes’ lipid bilayers while the hydrophilic absorbers are encapsulated into the liposomes. Once the light absorbers are irradiated by the source, they will act as a photo-thermal transducer and convert the light-to-heat effectively followed by releasing the content into the extra-liposomal environment (Ng et al., 2016). An early study showed that the calcein-loaded liposomes tended to release the content significantly under exposure to 488 and 577 nm laser lights. The photo-thermal release of the drug in the buffer was attributed to the photo-absorption of calcein at the 488 nm wavelength, while the photo-absorption of hemoglobin at 577 nm caused the drug release in blood samples (Khoobehi et al., 1990). An in vivo study combined an argon laser and adenosine diphosphate (ADP)-loaded liposomes showed the most efficient therapeutic gain of photocoagulation (Khoobehi et al., 1992). To visualize the release pattern of a thermo-sensitive liposome containing luciferin, transgenic reporter mice were administrated by the nano-carriers and then irradiated by a 527 nm laser. The in vivo bioluminescence imaging demonstrated that the liposomes immediately could release the molecular dye upon irradiation by the laser (Mackanos et al., 2009). On the other hand, some lipophilic dyes integrated into the liposome membrane showed a photolysis behavior after exposure to visible electromagnetic waves (Gregersen et al., 2010).
As another type of photo-thermal moieties, plasmonic nanoparticles can generate heat via harmonic oscillation of their free electrons triggered by the electric field of the electromagnetic waves. In addition to the great potential in enhancing CT image contrast (Cole et al., 2015) and radiotherapy gain (Zabihzadeh et al., 2018), the gold nanoparticles exhibit a significant plasmonic property in a wide range of wavelengths. Similar to the molecular absorbers, the gold nanostructures could be incorporated into the aqueous portion or on the surface of the liposomes. For instance, the gold-coated liposomes containing DOX showed a controllable manner in DOX release upon exposure to near infrared laser and caused to increase in cancer cell fatality (Wu et al., 2011). As for gold nanoparticles anchored on the liposome surface, the nano-hybrid carrier released about 70% of the encapsulated content while exposed to a 532 nm laser beam. The viability assay of breast cancer cells showed that DOX-loaded nanocarrier under laser beam irradiation significantly reduced cell viability (Qin et al., 2011). Although non-plasmonic gold nanoparticles were also proposed as photo-thermal agents to be integrated into the liposome structures, they mainly need a photon source with a shorter wavelength like UV and a long irradiation interval (Paasonen et al., 2007; An et al., 2010).
Also, liposomes release the encapsulated content via photochemical pathways using photo-sensitive lipid molecules embedded in the bilayer envelope. Photo-isomerization is one of the mechanisms in which light-sensitive moiety like azobenzene gives rise to conformational changes upon irradiation by a photon source (Chen et al., 2020). The azobenzene-based liposomes mainly activate at UV range source, limiting their clinical application due to the short penetration of UV electromagnetic waves into the body (Kano et al., 1981). More recently, a unique strategy was used to release the DOX under exposure to NIR-irradiation. They incorporated up-converting nanoparticles into DOX-loaded liposomes to convert the NIR beam to UV/blue light in situ, followed by photo-isomerization of azobenzene, destabilizing the membrane and releasing the drug (Yao et al., 2016).
On the other hand, the photo-cleavage mechanism triggers drug release from liposomes after exposure of photo-cleavable structures like plasmalogens to light sources. For example, a nano-system proved a photo-cleavage property during irradiation at 365 nm, followed by the release of penicillin (Goto et al., 2019). Moreover, photo-polymerization—the crosslinking of lipids under the incidence of photons—elevated the fluorescent agent release more than 100 times using a UV source (Bondurant and O’Brien, 1998). In a more recent study, liposomes equipped with a photo-sensitizer (verteporfin) experienced an adequate release of PAC1R antisense oligonucleotides under UV exposure due to the generation of ROS by verteporfin. The ROS act as a destabilizer of liposomes and endolysosomal membranes, where the release of the gene-silencing content subsequently decreases the PAC1R fluorescence intensity by 74% (Chen et al., 2017b).
Taking advantage of ultrasound waves, many studies have investigated the impact of mechanical waves on stimulating liposomes containing imaging or therapeutic agents. Thanks to the penetration of the ultrasound wave deeply and locally into the body while inducting low side effects, which extensively benefit patients in diagnostic and therapeutic procedures. Interestingly, the MRI-HIFU modality generates heating in the exposed site where thermo-sensitive nano-carriers like liposomes release their content (Zhou, 2011). Moreover, the system is capable to trace the drug biodistribution and pharmacokinetic pattern using MRI contrast agents embedded in the liposomes. For instance, a thermo-sensitive ultra-magnetic liposome was developed to carry an anti-vascularization agent (Combretastatin A4 phosphate). They showed that the thermo-responsive nano-carriers release the encapsulated content significantly upon exposure to HIFU and they significantly prohibited the tumor growth in CT26 tumor-bearing mice (Thébault et al., 2020). More recently, sono-sensitive liposomes-containing DOX were conjugated to monoclonal antibody Trastuzumab caused higher uptake in HER2+ breast cancer cells. The combination of the liposomes with low-frequency ultrasound showed a synergistic effect on cancer cell mortality (Elamir et al., 2021). In another study, gadolinium- and DOX-loaded liposomes were activated upon HIFU exposure, in which they release about whole encapsulated MRI contrast and anticancer contents. The MDA-MB-231 tumor-bearing mice treated with the liposomes and HIFU experienced a significant inhibition in tumor growth (Amrahli et al., 2021).
Mesoporous silica nanoparticles
Among all different types of inorganic or hybrid organic/inorganic-based materials, silica is under the spotlight of research in nanomedicine. Silica is considered as a safe material for administration in food and cosmetics by US FDA (BernArdos and KouřimsKá, 2013). Silica NPs with several distinguishing features like easy functionalization during synthesis or postmodification and biocompatibility could be considered as potent nanocarrier for therapeutic purposes. Porous types of silica NPs have a higher surface area that makes them as an ideal adsorbent of molecules, ions, and therapeutic agents (Farjadian et al., 2015; Farjadian et al., 2017a; Farjadian et al., 2019c; Taqanaki et al., 2019; Mohammadi H. et al., 2020). Mesoporous silica nanoparticles (MSNs) with pore sizes between 2 and 50 nm have a tailorable structure with a high surface area and pore volumes and decoration possibility with various functional groups and have found distinguished applications as host of therapeutic agents (Farjadian et al., 2019c).
MSNs need a uniform particle size and pore volume large enough to enhance loading capacity and, if required, surface engineering properties on the external and internal surfaces need to be an ideal carrier for a drug. These parameters can be controlled during the synthesis by varying the reaction mixture’s pH, temperature, surfactant concentration, and silica source. The synthesis of MSNs occurs by the liquid crystal template mechanism wherein hydrolysis and condensation of silica could occur at the surface of surfactant micelles, and silica precursor (tetraethyl orthosilicate) transforms into solid silica (Narayan et al., 2018).
This section reports some examples of MSNs responsive to exogenous physical triggers and their application in the biomedical fields.
Mesoporous silica nanoparticles in therapy and diagnosis
Within the unique properties of MSNs’ pores to be capped by gatekeepers, the possibility of designing smart engineered delivery agents becomes possible. MSNs have found diverse applications in medicine, catalysts, adsorbents, and sensors (Kankala et al., 2020).
MSNs utilization for cargo loading like small molecules (i.e., ions and drugs) and macromolecules such as proteins and genetic materials (i.e., DNA, RNA, siRNA, etc.), have made them a good platform for DDSs. Today, researchers are exploring oral and injection formulas of MSNs for therapeutic purposes. Farjadian et al. (2015) have introduced a novel approach to applying MSNs as an antidote agent for several toxicities.
Otherwise, by integrating metallic cores inside MSNs’ shells (metal@MSN), the MSNs with devoted properties of metal cores have been prepared (Zhang et al., 2021). Such novel innovations in preparing MSNs’ nanocomposites have allowed to utilization MSNs as a carrier of therapeutic agents with simultaneous imaging modalities in MRI and PET (Farjadian et al., 2019c). In this section, several types of MSNs used for therapeutic and imaging purposes regarding their response to physical stimulus are categorized and discussed.
Temperature-responsive mesoporous silica nanoparticles
Among physically triggered therapeutic systems, the thermo-responsive strategy has been widely applied, especially in cancer therapy. MSNs could be engineered to deliver cargo in response to temperature changes, while modified with thermo-responsive polymers such as PNIPAAm. DDSs of this type are able to release drugs on the variation of temperature around their adjusted LCST. This system could be applied for efficient delivery through encountering temperature increase of the tumor site or for hyperthermia. As earliest model, Ibu delivery was investigated by mesostructured cellular foam modified by PNIPAAm inside nano-valves using the ATRP method (Wang T. et al., 2016). In an eco-friendly and straightforward route, PEO-b-PNIPAAm was utilized both as structure-directing and thermo-responsive agents while loaded with Ibu, and the system proved to have a thermosensitive release profile (Bathfield et al., 2016).
A multifunctional platform of MSN coated with thermos/pH-responsive polymers (pNIPAAm-co-pMA) was developed for co-delivery of paclitaxel, 5-Fu, cis-platin, and siRNA (targeting ABCG2) while also targeted with FA and the system was abbreviated as MSN@pNIPAAm-pMA/FA. The results demonstrated that both anticancer drug and siRNA were successfully delivered to CD133+ cancer cells by designed MSNs. In vivo studies showed that downregulation of ABCG2 parallel with enhanced efficiency of chemotherapeutic drugs and induced apoptosis in carcinoma cells of laryngeal (Qi et al., 2016). Another interesting example was reported for co-delivery of evodiamine and berberine (hydrophobic drugs) as an herbal Chinese medicine with synergistic antitumor activity. Lipid-coated MSN with thermo/pH polymeric coating (lipid-MSN@PNIPAAm-co-PMA) has proven to be an excellent smart carrier for the delivery of these two drugs in vitro and in vivo analyses.
A remotely targeted thermo/pH on-demand delivery and diagnosis system based on gold and magnetic NPs embedded silica nano-shuttles (MGNSs) coated with pNIPAM-co-pMA (MGNS@ PNIPAM-co-PAA) loaded with DOX was created, and the assembled structure was named as nano golf balls. Distinguished applications of the aforementioned structure were investigated through magnetic-field transport in; 1) glass capillary tubes as simulator of delivery through blood vessels, 2) porous hydrogels as simulator of human tissue (e.g., BBB, muscles, tendon, cartilage, and bone). The in vitro DOX release pattern was assessed in differentiated human neurons and epithelial HeLa cells (Wang D. et al., 2017).
The presence of diagnosable materials in the core of thermo-responsive MSNs has resulted in the formation of theranostic systems. MNP core-modified thermo-responsive agent coated with MSN (MNP@PNIPAAm-co-DMAEMA@MSN) was successfully created for MTX delivery and MR imaging.
MSN capped with paraffin showed to have a thermo-sensitive cargo release profile since paraffin could be melted at a certain temperature (Aznar et al., 2011). By this means AuNSts-coated MSNs were capped by paraffin for efficient DOX delivery through PTT (Hernández Montoto et al., 2018).
Photo-triggered mesoporous silica nanoparticles for therapeutic and imaging
Light is one of the most powerful sources of exogenous stimuli and could be considered a safe treatment protocol (Li Y. et al., 2021). On the other hand, photo-responsive agents could be diagnosed through well-known imaging techniques such as photoacoustic, photothermal, and NIR (Shin et al., 2021). The intensity of photon sources could be easily tuned to visible, UV, and NIR by the sensitivity of therapeutic agents. Designing theranostic MSNs-based materials could be a step forward in facilitating the clinical trial requirements of these materials. NIR photon has been recognized as less risky with more efficacies in cells regarding UV and visible emissions (Chen et al., 2021). Several combinatorial techniques would provide MSNs to be photoresponsive. Several examples of such materials have been prepared through intra and/or extra pore modifications or gate capping with photo-responsive agents (Moodley and Singh, 2021). First reports in preparation of photochromism in a mesostructured silica appeared in early 2000. In these reports, several photochromic dyes like spiropyrane (Wirnsberger et al., 2000; Schomburg et al., 2001), azobenzene (Liu et al., 2003), and consequent photo controlled release was well performed by coumarin-modified MS material (Mal et al., 2003) were implemented.
Cyclodextrins (CD) with a self-assembled structure capable of host and guest interplay could potentially be applied as a gatekeeper of MSNs’ pores (Yi et al., 2018). Azo-benzene and related derivatives are recognized as photoresponsive agents, which possess their unique capability by cis and trans isomerization upon visible light and irradiation with UV light. Several combinations of CD with trans azo-benzene have been reported as photoresponsive gatekeepers of MSNs in drug delivery with the capability of gate opening while encountering UV irradiation through the formation of cis-isomer of azo-benzene with less CD interaction (Wang and Wu, 2016b; Zhao et al., 2017).
In recent years, some inorganic-based nanomaterials with emitting properties, including gold NPs, QDs (i.e., CdS, CuS,W2S, and Mo2S), and also organic-based fluorophores NPs like graphene QDs and carbon-dots with photo and thermal sensitivity have been applied as MSNs gatekeepers in designing DDSs (Table 3) (Wen et al., 2017; Yan et al., 2020). This part discusses some prominent types of such photoresponsive gatekeepers in combination with DDSs of MSNs. Theranostic MSNs with gold NPs and carbon QDs were applied for DOX delivery and showed highly trackable in cancer cell lines by fluorescence imaging (Akbarian et al., 2022). Gold-capped nanovalves of MSNs could efficiently act as photoresponsive switches for drug delivery. AuNPS has been demonstrated to be an efficient photoresponsive capping agent of MSN for the paclitaxel delivery system, which had revealed a “zero premature release” pattern before irradiation with a light source (Vivero-Escoto et al., 2009). Rod-shaped MSN with high cargo loading capacity and preferable cell internalization has been capped with gold nanorods (GNRs) for NIR photothermal therapy. Loading nano-pores with photosensitizer having an anticancer activity (Ce6) and irradiation source were performed by photodynamic therapy (PDT). Furthermore, the therapy was combined with dual-mode imaging by NIR fluorescence (NIRF) and photoacoustic (PA) (Sun et al., 2017).
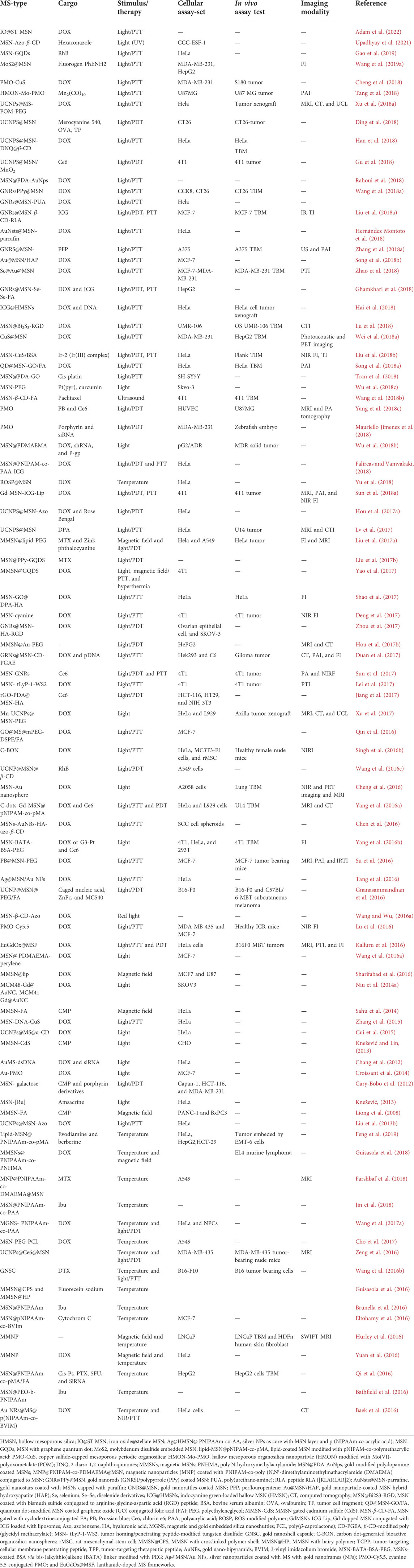
TABLE 3. Physically triggered MSN concerning the type of stimulus and cargo for in vitro and in vivo investigation, therapy, and diagnosis.
One of the well-known types of QDs NPs is CuS, with low-cost availability and low toxicity, which has found applications in PTT due to intrinsic capability in NIR adsorption. An exciting paradigm of CuS-capped MSNs was reported as a controllable DOX delivery in PTT. The CuS NPs were conjugated to nanopores through double-stranded oligonucleotide sequences (MSN-DNA-CuS) that could be de-hybridized in encountering localized heat (Zhang et al., 2015). Another example is the CuS capped to yolk/shell structure of periodic mesoporous organosilica (PMO-CuS) NPs that provided triple-responsive nanoplatforms with efficient performance in the DOX delivery system via PTT (Cheng et al., 2018).
CdS also applied as a gatekeeper in designing a photoresponsive camptothecine delivery system of MNP-coated MSN (via carbamate photoresponsive linkage) (Knežević and Lin, 2013). Carbon dot (C-dot)-capped MSN was a powerful fluorescent agent in designing DDS with bioimaging capability (Jiao et al., 2016).
Mo2S-embedded MSN has exhibited to be an excellent PT agent and while fabricated with fluorogen PhENH2 and targeted with folic acid (FA) showed to be a potent agent in targeted PTT and fluorescence imaging (Wang J. et al., 2019). A novel PT-inducing developed capping agent of MSN is graphene quantum dots (GQDs) that successfully practiced in the rhodamine B delivery system (Gao et al., 2019). Other examples include GQDs-capped MMSN for PTT and magnetic hyperthermia in the DOX delivery system (Yao et al., 2017). Bismuth sulfide (Bi2S3) NPs have also been recognized as a NIR light absorbing and contrast agent of CT imaging. Recently, two novel types of theranostic light-responsive MSNs systems with Bi2S3 have been reported (Li L. et al., 2018; Lu et al., 2018). MSN-coated Bi2S3 targeted with trastuzumab (Bi2S3-MSN-trastuzumab) loaded with DOX was created for simultaneous PTT and CT imaging (Li L. et al., 2018). Another system was explicitly designed for osteosarcoma by conjugating RGD (Bi2S3-MSN-RGD) to the system for DOX delivery and malignant tumor PTT (Lu et al., 2018).
Other developments in the establishment of light-responsive nano-carriers lay in the formation of core/shell structure of common responsive elements even with MSN as a core (MSN@Shell) or in the opposite, MSN as a shell (core@MSN). Metallic-based NPs with emitting properties have played a distinct role in designing theranostic agents. In this regard, gold-coated MMSNs (MNP-coated MSN) were designed for PTT and dual MR and CT imaging (Hou X. et al., 2017). Polydopamine-gold nanoparticle was applied as a shell for MSN (MSN@PDA-Au), proved to be an effective DOX delivery system triggered by NIR, and showed to be potent in PTT (Rahoui et al., 2018). On the other hand, gold NPs (AuNPs) and gold nanorods (GNRs) have been efficiently applied as the core of MSNs.
A dual imaging nanotheranostic system based on biodegradable gold nanorattle (GNR)-coated MSN filled (GNRs@MSN) with perfluoropentane (PFP) was developed for melanoma PTT and ultrasound (US) and photoacoustic (PA) imaging. A schematic illustrating synthesis, in vivo injection, and PTT and dual US/PA imaging are presented in Figure 6. Upon NIR irradiation, the GNRs generated heat that induces PFP to have a liquid/gas phase transition, which resulted in nano-bubbles formation. Nano-bubbles convert to microbubbles that can improve EPR and enhance signals of US (Li C. et al., 2018). Several other examples of photo-responsive AuNPs-based MSN systems are presented in Table 3.
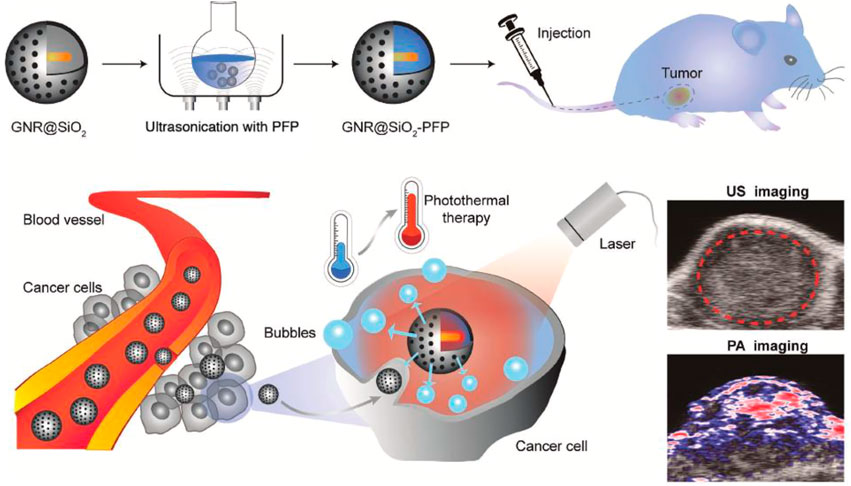
FIGURE 6. Schematic illustration of GNRS@MSN filled with PFP from synthesis, in vivo injection, cell entrance, PTT, and dual-mode imaging (US and PA). Reprinted with permission from Wiley (Li C. et al., 2018).
Newly established up-converted NPs (UCNPs), known as an unusual combination of lanthanide ions, showed to be potent photo-responsive agents due to their capacity in adsorbing NIR and changing to UV or visible emission (Escudero et al., 2016). UCNPs could be employed as the core of MSN as an NIR-responsive system and detected through fluorescence (Wang et al., 2011). Several examples of such systems are presented in Table 3.
Multifunctional luminescent-based UCNPs of NaYF4:Yb3+/Er3+ with MSN coating and thermoresponsive capped were developed for DOX delivery (Zhang et al., 2013). Multi-color emitted MSN designed UCNPs@MSN-Azo-coated UCNPs ((NaYF4: Yb, Tm) @ 0.6(NaYF4: Yb, Er) capped with azobenzene and loaded with RB and DOX was developed for DDs via PDT. The UCNPs capable of emitting UV: blue and green: red multi-band light was acted as a stimulus of light-responsive Azo and photo-sensitizer of RB. The mechanism of action of this multifunctional nano platform in blood vessels is depicted in Figure 6. After the accumulation of NPs in the tumor site due to the EPR effect, the NIR light source was excited with the potential of deep penetrating that could activate UCNPs. The green emission could be adsorbed by RB and produce ROS for PDT. At the same time, UV/blue emission would activate Azo to open the gates for DOX release (Hou B. et al., 2017).
Magnetic field responsive mesoporous silica nanoparticles
Magnetic responsive MSN-based materials, with MNP in their composition, are recognized as powerful carriers for efficient delivery systems and utilization in MR imaging. Several theranostic systems have been developed based on the MNP core and MSN shell (MMSN). Some examples of these potent systems are discussed here. Multifunctional superparamagnetic iron oxide-based MSN with dibenzo-crown ethers (MMSN-crown ethers) periphery with trimodal responsiveness to pH/ultrasound and magnetic field was developed for DOX delivery. Ultrasonic waves could stimulate sodium or cesium ions binding with crown ethers with pH-responsive binding that act as gatekeepers of the system. In vitro MRI analysis showed the high relaxivity of the NPs in the magnetic field, potent theranostic agents (Lee et al., 2013).
A nanoassembly of MMSN coated with chitosan (charge converted polymer-g-FA and citraconic anhydride) targeted by TAT peptide (MMSN@CS/TAT) and loaded by CMP as DNA-toxin antitumor drug was established. MRI observed a high accumulation of NPs in the tumor site. Upon this phenomenon, the FA acts as a target for receptor-mediated endocytosis in the cancer cells. Through endocytosis into the lysosomes, the polymeric layer charges’ reversed from negative to positive and separated from MMSN, and TAT peptide assisted the carrier entrance to nucleus, where CMP could induce apoptosis (Li et al., 2014). A novel microwave-triggered system for etoposide targeting via external magnetic field was created based on magnetic/Fe3O4 core and zinc oxide/ZnO interlayer coated by mesoporous silica (MNP@ZnO2@MS). The ZnO interlayer could act as an absorber of microwave irradiation with an excellent thermal response. These magneto-responsive particles showed sustained control release upon microwave irradiation (Qiu et al., 2014). A maghemite core MSN capped with 1-tetradecanol (TD) molecule (MMSN-TD) as a heat-triggered agent was developed for delivery of DOX. This system showed to be diagnosable via MRI, and a plausible mode of release was established through TD gate-keeping at 40°C (Cho et al., 2017). A multifunctional magneto-responsive MSN was developed for multimodal imaging (PAI, MRI, and FI) and chemo and photodynamic therapy. By this mean, Fe3O4 as core with MSN shell and protective layer of lipid-PEG and lipid-PEG-MTX was created (MMSN-lipid-PEG-MTX) while loaded with DOX and zinc phthalocyanine (ZnPc) (MMSN-lipid-PEG-MTX/DOX&ZnPc) as a photosensitizer. The presence of lipid-PEG-MTX in periphery of MMSN was effective in increasing dispersion stability and preventing drug leakage while decreasing hemolytic activity. The magnetic core provided magneto targeting to the tumor site, and the MTX conjugate would selectively kill cancer cells by overexpressing folate receptors. ZnPc was loaded in the lipid layer and served as a fluorescence tracking agent in FI and a source of PDT ROS.
Magnetic nanoparticles in therapy and diagnosis
The original idea of using magnetic particles to deliver medicinal agents to a specific body area was formed in the late 1970s. This method injects the whole drug/magnetic carrier into the body through venous or arterial vessels (Kodama and materials, 1999). Then, by applying an external magnetic field and creating a magnetic gradient in a specific body area, a set of drugs/magnetic carriers are transported to that location (motor site) by the circulation and accumulation (Meyer et al., 2001). When the accumulation of particles in the motor site is well performed, the therapeutic agents (drugs) are separated from their magnetic carrier and released at the tumor site (Shubayev et al., 2009). Drug-releasing mediators can include enzymatic activation, changes in physiological conditions such as changes in ambient pH, changes in osmolality (the degree of solubility of a solution per unit of solvent), or changes in ambient temperature (Allen and Cullis, 2004). Physical triggers in releasing medical agents from MNPs are mostly temperature and magnetic fields due to MNPs hyperthermia and magnetic properties (Kauscher et al., 2019). Other stimuli are less important for these particles. This means, in this part of the review we discuss hyperthermia and magnetic therapy. Furthermore, MRI is the most important imaging modality for these particles (Farjadian et al., 2017b).
Targeted drug delivery is more relevant to cancer treatment because the main challenge in cancer treatment is to target and kill cancer cells so that they have as little effect on healthy cells as possible (Bae and Park, 2011). One of the aims of nanotechnology is to put drugs on carriers (NPs), then send, and release them into specific cells, which is called targeted drug delivery. By using magnetic nanoparticles and generating a magnetic field, the drug can be intelligently delivered to the desired tissue without damaging other tissues (Shen et al., 2013).
Diagnosis of the disease in the early stages is essential for improvement and treatment methods. Iron oxide nanoparticles are currently the only magnetic nanomaterials used in clinical medicine as a contrast agent in MRI and as a carrier in drug delivery (Dadfar et al., 2019). These particles have also found applications in separating cancer cells through functionalization with antibodies (Haghighi et al., 2019; Haghighi et al., 2020). Experiments performed on iron oxide nanoparticles over the years show that these particles have no immediate or long-term toxic effects in vivo, and the presence of some nanoparticles with nanocarriers enhances their effect on cancer cells (Liu G. et al., 2013).
Temperature-triggered magnetic nanoparticles
Cancer treatment is one of the most critical challenges, facing medical knowledge and drug delivery. Because conventional cancer treatments such as chemotherapy, surgery, and radiation are not very effective in treating some cancers such as glioblastoma, hyperthermia can be considered a safe approach to the definitive treatment of such cancers (Chang et al., 2018). Treatment of cancer by induced excitation of biocompatible superparamagnetic nanoparticles by intermittent magnetic field heating of specific organs or tissues to temperatures around 41–47°C to treat cancer is called hyperthermia (Chang et al., 2021). The hyperthermia process with magnetic ferrofluid suggests the possibility of specific heat localization; cancer cells are more sensitive to temperature than normal cells (Das et al., 2019). Superparamagnetic nanoparticles can cause excess heat to be transferred to the target area through oscillations of magnetic moments within the nanoparticles (Xiao and Du, 2020). Thus, solid cancer cells will be killed while normal tissue cells will remain at a temperature below 41–47°C. The heating potential depends to a large extent on the size and shape of the particles. Therefore, using single-order magnetic particles in nanosized is preferred over multi-order particles in micro size (Ha et al., 2018). Because nanoparticles can withstand alternating magnetic fields, the amount of heat generated varies depending on the frequency, the intensity of the magnetic field, and the time it takes to be in the field. In this case, after the particles are placed inside the cells, and then the body is placed in a magnetic field, these particles produce localized heat in the cancerous mass and accelerate the destruction of the tumor (Behrouzkia et al., 2016). Therefore, hyperthermia will effectively increase the treatment of solid cancer (Cole et al., 2011). To wrap up all data on magnetic approaches in the clinical sector, here are some recently published articles (Table 4). For most of them, doxorubicin has been considered as cargo; however, some of them did not include any extra medicinal cargo, just by magnetic-mediated hyperthermia trying to fight the target, cancer cells. To better explain the recent contents, mentioning some references in the table would be helpful. In the study by Huang et al. (2020), gelatin was used as a matrix to encapsulate doxorubicin. Then the complex was covered by the outer layer of alginate and magnetic iron oxide particles (core-shell nano-system).
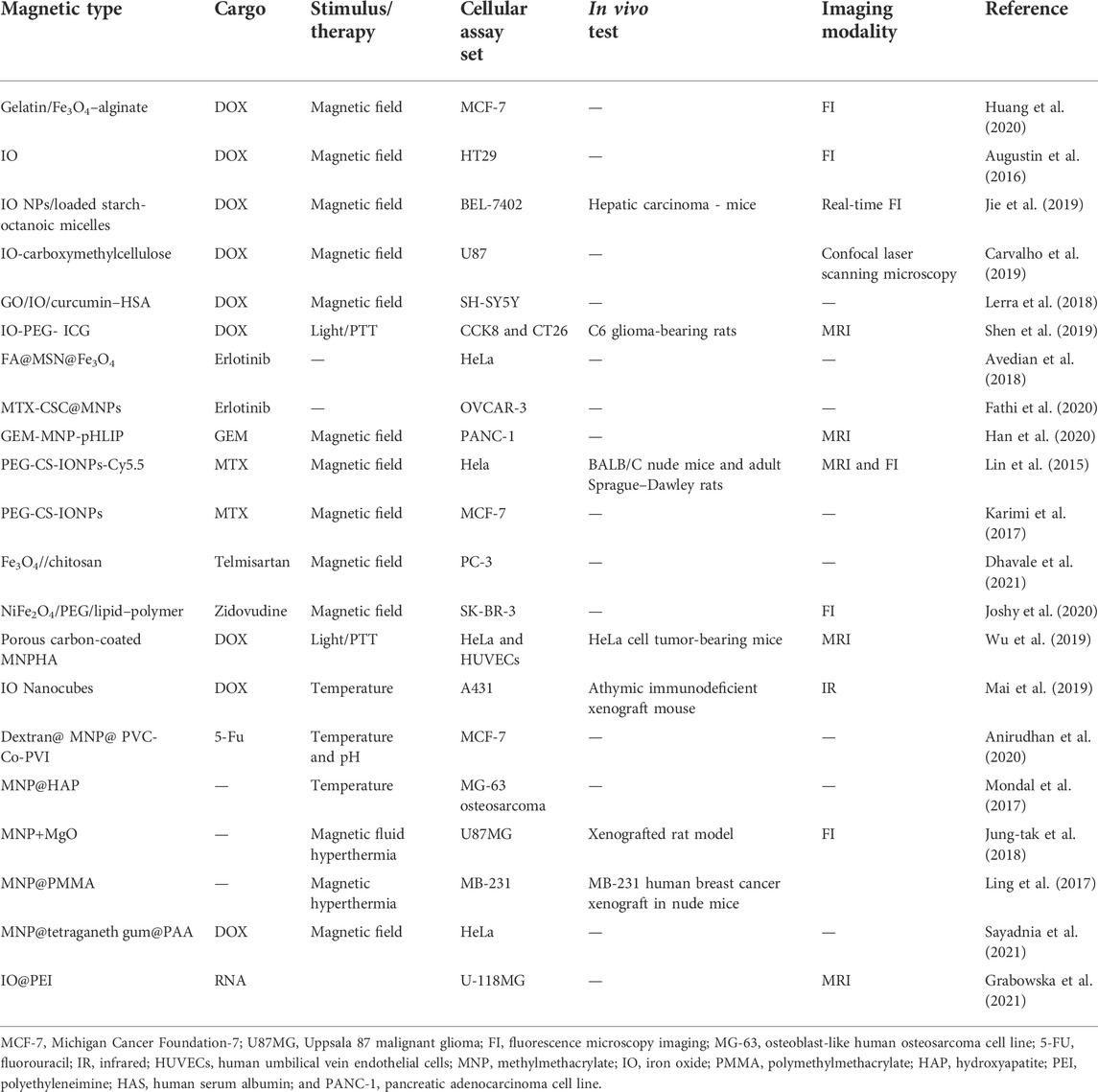
TABLE 4. Physically triggered MNP concerning the type of stimulus and cargo for in vitro and in vivo investigation, therapy, and diagnosis.
However, the size was relatively large (401 nm), after 6 hours of the synthesized particles reaching the nucleus of MCF 7 cancer cells. Then, after 12 h, it was observed that the cell death rate was reduced by 50%. In another study by Baek et al. (2016), a three-component system was used. In the system’s center, gold nanoparticles were included around the nucleus by DOX-containing MSN designed as drug reservoirs. Then a temperature-sensitive gate (poly(N-isopropylacrylamide)-based N-butyl imidazolium copolymer) was used to finalize the invention. In addition to labeling, gold particles in this design produce heat under NIR, which eventually leads to the opening of the gates and release of the drug. Another study was about the synthesis pH-responsive system (Carvalho et al., 2019).
Other studies have focused on other forms of matter. In the study of Ling et al., polymethylmethacrylate (PMMA)-Fe3O4 in the form of liquid-to-solid transition state was used. This system will be able to suppress the MB-231 breast cancer xenograft model in mice from the injection route in the liquid state. It was seen that after injecting this system, the cancer site was considered with high accuracy, without showing any leakage in other locations (Ling et al., 2017). Other cases of research have followed a more or less similar pattern. In Anirudhan and Christa’s study, glycidyl methacrylate grafted dextran was used to coat iron oxide nanoparticles and N-vinylcaprolactam and N-vinylimidazole monomers were used to create temperature and pH sensitivities. After studying the drug release at different acid strengths, it was found that the designed system was able to follow a release pattern similar to the cancerous environment (Anirudhan et al., 2020).
Nanopharmaceuticals on the market
In the end, it is necessary to mention some of the nano-systems that have been able to enter the field of treatment. Among the types of nanoparticles mentioned so far, nanocrystals, liposome and lipid-based nano-systems, polymer-based nanoparticles, protein-based nano-systems, and metal-based nanopharmaceuticals have been successful in the field of therapy (Farjadian et al., 2019a). The following Table 5 introduces this category and mentions some examples.
Conclusion and future outlook
The key properties of physical stimuli-responsive therapeutic/diagnostic systems compared to the direct delivery of therapeutic agents are their ability to release the drug in a stimuli-responsive manner, high drug loading capability, lower toxicity, synergistic therapeutic efficacy, and biocompatibility. Table 6 compares the types of physical triggers and the advantages and disadvantages of such strategies. The proper nanocarrier design and structural optimization are crucial challenges to physical stimuli-responsive nano drug delivery systems. Multiple lines of evidence indicate that it mainly relies on empirical analysis, such as the type of disease, the characteristics of the drugs, and the nanocarrier physicochemical properties. These areas required further investigation and evaluation. As for future perspectives, the data that would be achieved from these extensive and comprehensive studies lead to the development of more well-designed physical stimuli-responsive nanoparticles capable of reaching the market, providing a novel generation of nanocarriers more suitable for clinical application with minimum adverse side effects and maximum efficiency.
Another promising approach is to focus on nanoparticles with the potential to respond to multiple triggers. For instance, the light source has a high point focusing accuracy but is very poor at penetrating tissue. On the other hand, ultrasound waves have the best permeability to the tissue, but they are weak to have the accuracy of targeting like light. It is possible to generate a system with the positive points of both origin systems and synergistically plays significant strengthening effects in the treatment process by combining these two physical systems. Although physical stimuli-responsive nanoparticles are primarily evaluated for preclinical applications, they will shortly be an essential part of the clinician’s armory in addressing drug delivery and diagnosis.
Author contributions
All authors listed have made a substantial, direct, and intellectual contribution to the work and approved it for publication.
Acknowledgments
FF would like to acknowledge the Research Council of Shiraz University of Medical Sciences to support this work (Grant No. 98-01-36-21465/20837).
Conflict of interest
The authors declare that the research was conducted in the absence of any commercial or financial relationships that could be construed as a potential conflict of interest.
Publisher’s note
All claims expressed in this article are solely those of the authors and do not necessarily represent those of their affiliated organizations, or those of the publisher, the editors, and the reviewers. Any product that may be evaluated in this article, or claim that may be made by its manufacturer, is not guaranteed or endorsed by the publisher.
References
Abuwatfa, W. H., Awad, N. S., Pitt, W. G., and Husseini, G. A. (2022). Thermosensitive polymers and thermo-responsive liposomal drug delivery systems. Polymers 14, 925. doi:10.3390/polym14050925
Achbergerová, E., Šmejkalová, D., Huerta-Angeles, G., Souček, K., Hermannová, M., Vágnerová, H., et al. (2018). In vivo monitoring of tumor distribution of hyaluronan polymeric micelles labeled or loaded with near-infrared fluorescence dye. Carbohydr. Polym. 198, 339–347. doi:10.1016/j.carbpol.2018.06.082
Adam, A., Harlepp, S., Ghilini, F., Cotin, G., Freis, B., Goetz, J., et al. (2022). Core-shell iron oxide@ stellate mesoporous silica for combined near-infrared photothermia and drug delivery: Influence of pH and surface chemistry. Colloids Surfaces A Physicochem. Eng. Aspects 640, 128407. doi:10.1016/j.colsurfa.2022.128407
Ahmadi, S., Rabiee, N., Bagherzadeh, M., Elmi, F., Fatahi, Y., Farjadian, F., et al. (2020). Stimulus-responsive sequential release systems for drug and gene delivery. Nano today 34, 100914. doi:10.1016/j.nantod.2020.100914
Ahn, D. G., Lee, J., Park, S. Y., Kwark, Y. J., and Lee, K. Y. (2014). Doxorubicin-loaded alginate-g-poly(N-isopropylacrylamide) micelles for cancer imaging and therapy. ACS Appl. Mat. Interfaces 6, 22069–22077. doi:10.1021/am505444c
Aibani, N., Da Costa, P. F., Masterson, J., Marino, N., Raymo, F. M., Callan, J., et al. (2017). The integration of triggered drug delivery with real time quantification using FRET; creating a super ‘smart’drug delivery system. J. Control. Release 264, 136–144. doi:10.1016/j.jconrel.2017.08.013
Akbarian, M., Gholinejad, M., Mohammadi-Samani, S., Farjadian, F. J. M., and Materials, M. (2022). Theranostic mesoporous silica nanoparticles made of multi-nuclear gold or carbon quantum dots particles serving as pH responsive drug delivery system. Microporous Mesoporous Mat. 329, 111512. doi:10.1016/j.micromeso.2021.111512
Akimoto, J., Ito, Y., Okano, T., and Nakayama, M. (2018). Controlled aggregation behavior of thermoresponsive polymeric micelles by introducing hydrophilic segments as corona components. J. Polym. Sci. Part A Polym. Chem. 56, 1695–1704. doi:10.1002/pola.29050
Akimoto, J., Nakayama, M., and Okano, T. (2014). Temperature-responsive polymeric micelles for optimizing drug targeting to solid tumors. J. Control. Release 193, 2–8. doi:10.1016/j.jconrel.2014.06.062
Al Sulaiman, D., Chang, J. Y. H., Bennett, N. R., Topouzi, H., Higgins, C. A., Irvine, D. J., et al. (2019). Hydrogel-coated microneedle arrays for minimally invasive sampling and sensing of specific circulating nucleic acids from skin interstitial fluid. ACS Nano 13, 9620–9628. doi:10.1021/acsnano.9b04783
Alatorre-Meda, M., Alvarez-Lorenzo, C., Concheiro, A., and Taboada, P. (2013). UV and near-IR triggered release from polymeric micelles and nanoparticles. Smart Mater. drug Deliv. 1, 304.
Alconcel, S. N., Baas, A. S., and Maynard, H. D. (2011). FDA-approved poly (ethylene glycol)–protein conjugate drugs. Polym. Chem. 2, 1442–1448. doi:10.1039/c1py00034a
Allen, T. M., and Cullis, P. R. J. S. (2004). Drug delivery systems: Entering the mainstream. Science 303, 1818–1822. doi:10.1126/science.1095833
Amjad, M. W., Kesharwani, P., Amin, M. C. I. M., and Iyer, A. K. (2017). Recent advances in the design, development, and targeting mechanisms of polymeric micelles for delivery of siRNA in cancer therapy. Prog. Polym. Sci. 64, 154–181. doi:10.1016/j.progpolymsci.2016.09.008
Amrahli, M., Centelles, M., Cressey, P., Prusevicius, M., Gedroyc, W., Xu, X. Y., et al. (2021). MR-labelled liposomes and focused ultrasound for spatiotemporally controlled drug release in triple negative breast cancers in mice. Nanotheranostics 5, 125–142. doi:10.7150/ntno.52168
An, X., Zhang, F., Zhu, Y., and Shen, W. (2010). Photoinduced drug release from thermosensitive AuNPs-liposome using a AuNPs-switch. Chem. Commun. 46, 7202–7204. doi:10.1039/c0cc03142a
Anirudhan, T., Christa, J. J. J. O. D. D. S., and Technology, (2020). Temperature and pH sensitive multi-functional magnetic nanocomposite for the controlled delivery of 5-fluorouracil, an anticancer drug. J. Drug Deliv. Sci. Technol. 55, 101476. doi:10.1016/j.jddst.2019.101476
Anyarambhatla, G. R., and Needham, D. (1999). Enhancement of the phase transition permeability of DPPC liposomes by incorporation of MPPC: A new temperature-sensitive liposome for use with mild hyperthermia. J. liposome Res. 9, 491–506. doi:10.3109/08982109909035549
Augustin, E., Czubek, B., Nowicka, A. M., Kowalczyk, A., Stojek, Z., and Mazerska, Z. J. T. I. V. (2016). Improved cytotoxicity and preserved level of cell death induced in colon cancer cells by doxorubicin after its conjugation with iron-oxide magnetic nanoparticles. Toxicol. Vitro 33, 45–53. doi:10.1016/j.tiv.2016.02.009
Avedian, N., Zaaeri, F., Daryasari, M. P., Javar, H. A., Khoobi, M. J. J. O. D. D. S., and Technology, (2018). pH-sensitive biocompatible mesoporous magnetic nanoparticles labeled with folic acid as an efficient carrier for controlled anticancer drug delivery. J. Drug Deliv. Sci. Technol. 44, 323–332. doi:10.1016/j.jddst.2018.01.006
Awad, N. S., Paul, V., Alsawaftah, N. M., Ter Haar, G., Allen, T. M., Pitt, W. G., et al. (2021). Ultrasound-responsive nanocarriers in cancer treatment: A review. ACS Pharmacol. Transl. Sci. 4, 589–612. doi:10.1021/acsptsci.0c00212
Aznar, E., Mondragón, L., Ros‐Lis, J. V., Sancenón, F., Marcos, M. D., Martínez‐Máñez, R., et al. (2011). Finely tuned temperature‐controlled cargo release using paraffin‐capped mesoporous silica nanoparticles. Angew. Chem. Int. Ed. Engl. 50, 11368–11371. doi:10.1002/ange.201102756
Bae, Y. H., and Park, K. J. J. O. C. R. (2011). Targeted drug delivery to tumors: Myths, reality and possibility. J. Control. Release 153, 198–205. doi:10.1016/j.jconrel.2011.06.001
Baek, S., Singh, R. K., Kim, T. H., Seo, J. W., Shin, U. S., Chrzanowski, W., et al. (2016). Triple hit with drug carriers: PH- and temperature-responsive theranostics for multimodal chemo- and photothermal therapy and diagnostic applications. ACS Appl. Mat. Interfaces 8, 8967–8979. doi:10.1021/acsami.6b00963
Bangham, A. D., and Horne, R. (1964). Negative staining of phospholipids and their structural modification by surface-active agents as observed in the electron microscope. J. Mol. Biol. 8, 660–IN10. doi:10.1016/s0022-2836(64)80115-7
Bardajee, G. R., Hooshyar, Z., Farsi, M., Mobini, A., and Sang, G. (2017). Synthesis of a novel thermo/pH sensitive nanogel based on salep modified graphene oxide for drug release. Mater. Sci. Eng. C 72, 558–565. doi:10.1016/j.msec.2016.11.109
Bardajee, G. R., and Hooshyar, Z. (2018). Thermo/pH/magnetic-triple sensitive poly(N-isopropylacrylamide-co-2-dimethylaminoethyl) methacrylate)/sodium alginate modified magnetic graphene oxide nanogel for anticancer drug delivery. Polym. Bull. Berl. 75, 5403–5419. doi:10.1007/s00289-018-2329-7
Bassett, J. B., Anderson, R. U., and Tacker, J. R. (1986). Use of temperature-sensitive liposomes in the selective delivery of methotrexate and cis-platinum analogues to murine bladder tumor. J. Urology 135, 612–615. doi:10.1016/s0022-5347(17)45761-2
Bathfield, M., Reboul, J., Cacciaguerra, T., Lacroix-Desmazes, P., and Gerardin, C. (2016). Thermosensitive and drug-loaded ordered mesoporous silica: A direct and effective synthesis using PEO-b-PNIPAM block copolymers. Chem. Mat. 28, 3374–3384. doi:10.1021/acs.chemmater.6b00595
Behrouzkia, Z., Joveini, Z., Keshavarzi, B., Eyvazzadeh, N., and Aghdam, R. Z. J. O. M. J. (2016). Oman Med. J. 31, 89–97. doi:10.5001/omj.2016.19
Belmonte, A., Ussembayev, Y. Y., Bus, T., Nys, I., Neyts, K., and Schenning, A. P. (2020). Structural color actuators: Dual light and temperature responsive micrometer‐sized structural color actuators (small 1/2020). Small 16, 2070005. doi:10.1002/smll.202070005
Bernardos, A., and Kouřimská, L. (2013). Applications of mesoporous silica materials in food – a review. Czech J. Food Sci. 31, 99–107. doi:10.17221/240/2012-cjfs
Bi, H., Xue, J., Jiang, H., Gao, S., Yang, D., Fang, Y., et al. (2019). Current developments in drug delivery with thermosensitive liposomes. Asian J. Pharm. Sci. 14, 365–379. doi:10.1016/j.ajps.2018.07.006
Biswas, S., Kumari, P., Lakhani, P. M., and Ghosh, B. (2016). Recent advances in polymeric micelles for anti-cancer drug delivery. Eur. J. Pharm. Sci. 83, 184–202. doi:10.1016/j.ejps.2015.12.031
Bobo, D., Robinson, K. J., Islam, J., Thurecht, K. J., and Corrie, S. R. (2016). Nanoparticle-based medicines: A review of FDA-approved materials and clinical trials to date. Pharm. Res. 33, 2373–2387. doi:10.1007/s11095-016-1958-5
Bondurant, B., and O'brien, D. F. (1998). Photoinduced destabilization of sterically stabilized liposomes. J. Am. Chem. Soc. 120, 13541–13542. doi:10.1021/ja983112n
Brandt, J., Henning, S., Michler, G., Hein, W., Bernstein, A., and Schulz, M. (2010). Nanocrystalline hydroxyapatite for bone repair: An animal study. J. Mat. Sci. Mat. Med. 21, 283–294. doi:10.1007/s10856-009-3859-1
Brunella, V., Jadhav, S. A., Miletto, I., Berlier, G., Ugazio, E., Sapino, S., et al. (2016). Hybrid drug carriers with temperature-controlled on-off release: A simple and reliable synthesis of PNIPAM-functionalized mesoporous silica nanoparticles. React. Funct. Polym. 98, 31–37. doi:10.1016/j.reactfunctpolym.2015.11.006
Bulbake, U., Doppalapudi, S., Kommineni, N., and Khan, W. (2017). Liposomal formulations in clinical use: An updated review. Pharmaceutics 9, 12. doi:10.3390/pharmaceutics9020012
Cabral, H., Miyata, K., Osada, K., and Kataoka, K. (2018). Block copolymer micelles in nanomedicine applications. Chem. Rev. 118, 6844–6892. doi:10.1021/acs.chemrev.8b00199
Calatayud, D. G., Neophytou, S., Nicodemou, E., Giuffrida, S. G., Ge, H., and Pascu, S. I. (2022). Nano-theranostics for the sensing, imaging and therapy of prostate cancers. Front. Chem. 10, 830133. doi:10.3389/fchem.2022.830133
Carvalho, S. M., Leonel, A. G., Mansur, A. A., Carvalho, I. C., Krambrock, K., and Mansur, H. S. J. B. S. (2019). Bifunctional magnetopolymersomes of iron oxide nanoparticles and carboxymethylcellulose conjugated with doxorubicin for hyperthermo-chemotherapy of brain cancer cells. Biomater. Sci. 7, 2102–2122. doi:10.1039/c8bm01528g
Chambre, L., Degirmenci, A., Sanyal, R., and Sanyal, A. (2018). Multi-functional nanogels as theranostic platforms: Exploiting reversible and nonreversible linkages for targeting, imaging, and drug delivery. Bioconjug. Chem. 29, 1885–1896. doi:10.1021/acs.bioconjchem.8b00085
Chang, C., Wei, H., Quan, C. Y., Li, Y. Y., Liu, J., Wang, Z. C., et al. (2008). Fabrication of thermosensitive PCL‐PNIPAAm‐PCL triblock copolymeric micelles for drug delivery. J. Polym. Sci. A. Polym. Chem. 46, 3048–3057. doi:10.1002/pola.22645
Chang, D., Lim, M., Goos, J. A., Qiao, R., Ng, Y. Y., Mansfeld, F. M., et al. (2018). Biologically targeted magnetic hyperthermia: Potential and limitations. Front. Pharmacol. 9, 831. doi:10.3389/fphar.2018.00831
Chang, M., Hou, Z., Wang, M., Li, C., and Lin, J. J. a. M. (2021). Recent advances in hyperthermia therapy‐based synergistic immunotherapy. Adv. Mat. 33, 2004788. doi:10.1002/adma.202004788
Chang, R., and Tsai, W. B. (2018). Fabrication of photothermo-responsive drug-loaded nanogel for synergetic cancer therapy. Polymers 10, 1098. doi:10.3390/polym10101098
Chang, Y. T., Liao, P. Y., Sheu, H. S., Tseng, Y. J., Cheng, F. Y., and Yeh, C. S. (2012). Near-Infrared light-responsive intracellular drug and sirna release using au nanoensembles with oligonucleotide-capped silica shell. Adv. Mat. 24, 3309–3314. doi:10.1002/adma.201200785
Chen, C., Liu, G., Liu, X., Pang, S., Zhu, C., Lv, L., et al. (2011). Photo-responsive, biocompatible polymeric micelles self-assembled from hyperbranched polyphosphate-based polymers. Polym. Chem. 2, 1389–1397. doi:10.1039/c1py00094b
Chen, D., Yu, H., Sun, K., Liu, W., and Wang, H. (2014). Dual thermoresponsive and pH-responsive self-assembled micellar nanogel for anticancer drug delivery. Drug Deliv. 21, 258–264. doi:10.3109/10717544.2013.838717
Chen, G., Ding, L., Wu, P., Zhou, Y., Sun, M., Wang, K., et al. (2018). Polymeric micelleplexes for improved photothermal endosomal escape and delivery of siRNA. Polym. Adv. Technol. 29, 2593–2600. doi:10.1002/pat.4372
Chen, J., Li, Q., Wang, F., Yang, M., Xie, L., and Zeng, X. (2021). Biosafety, nontoxic nanoparticles for VL–NIR photothermal therapy against oral squamous cell carcinoma. ACS omega 6, 11240–11247. doi:10.1021/acsomega.1c00101
Chen, Q., Ke, H., Dai, Z., and Liu, Z. (2015a). Nanoscale theranostics for physical stimulus-responsive cancer therapies. Biomaterials 73, 214–230. doi:10.1016/j.biomaterials.2015.09.018
Chen, S., Bian, Q., Wang, P., Zheng, X., Lv, L., Dang, Z., et al. (2017a). Photo, pH and redox multi-responsive nanogels for drug delivery and fluorescence cell imaging. Polym. Chem. 8, 6150–6157. doi:10.1039/c7py01424d
Chen, S., Jiang, F., Cao, Z., Wang, G., and Dang, Z.-M. (2015b). Photo, pH, and thermo triple-responsive spiropyran-based copolymer nanoparticles for controlled release. Chem. Commun. 51, 12633–12636. doi:10.1039/c5cc04087f
Chen, W., Deng, W., and Goldys, E. M. (2017b). Light-triggerable liposomes for enhanced endolysosomal escape and gene silencing in PC12 cells. Mol. Ther. - Nucleic Acids 7, 366–377. doi:10.1016/j.omtn.2017.04.015
Chen, W., Goldys, E. M., and Deng, W. (2020). Light-induced liposomes for cancer therapeutics. Prog. lipid Res. 79, 101052. doi:10.1016/j.plipres.2020.101052
Chen, W., Su, L., Zhang, P., Li, C., Zhang, D., Wu, W., et al. (2017c). Thermo and pH dual-responsive drug-linked pseudo-polypeptide micelles with a comb-shaped polymer as a micellar exterior. Polym. Chem. 8, 6886–6894. doi:10.1039/c7py01389b
Chen, X., Liu, Z., Parker, S. G., Zhang, X., Gooding, J. J., Ru, Y., et al. (2016). Light-induced hydrogel based on tumor-targeting mesoporous silica nanoparticles as a theranostic platform for sustained cancer treatment. ACS Appl. Mat. Interfaces 8, 15857–15863. doi:10.1021/acsami.6b02562
Cheng, B., He, H., Huang, T., Berr, S. S., He, J., Fan, D., et al. (2016). Gold nanosphere gated mesoporous silica nanoparticle responsive to near-infrared light and redox potential as a theranostic platform for cancer therapy. J. Biomed. Nanotechnol. 12, 435–449. doi:10.1166/jbn.2016.2195
Cheng, C., Wei, H., Shi, B. X., Cheng, H., Li, C., Gu, Z. W., et al. (2008). Biotinylated thermoresponsive micelle self-assembled from double-hydrophilic block copolymer for drug delivery and tumor target. Biomaterials 29, 497–505. doi:10.1016/j.biomaterials.2007.10.004
Cheng, X., Li, D., Lin, A., Xu, J., Wu, L., Gu, H., et al. (2018). Fabrication of multifunctional triple-responsive platform based on cus-capped periodic mesoporous organosilica nanoparticles for chemo-photothermal therapy. Int. J. Nanomedicine 13, 3661–3677. doi:10.2147/ijn.s167407
Chien, Y. Y., Wang, T. Y., Liao, P. W., Wu, W. C., and Chen, C. Y. (2018). Folate-conjugated and dual stimuli-responsive mixed micelles loading indocyanine green for photothermal and photodynamic therapy. Macromol. Biosci. 18, 1700409. doi:10.1002/mabi.201700409
Cho, I. H., Shim, M. K., Jung, B., Jang, E. H., Park, M. J., Kang, H. C., et al. (2017). Heat shock responsive drug delivery system based on mesoporous silica nanoparticles coated with temperature sensitive gatekeeper. Microporous Mesoporous Mater. 253, 96–101. doi:10.1016/j.micromeso.2017.06.042
Choi, C., Chae, S. Y., and Nah, J.-W. (2006). Thermosensitive poly (N-isopropylacrylamide)-b-poly (ε-caprolactone) nanoparticles for efficient drug delivery system. Polymer 47, 4571–4580. doi:10.1016/j.polymer.2006.05.011
Cole, A. J., Yang, V. C., and David, A. E. J. T. I. B. (2011). Cancer theranostics: The rise of targeted magnetic nanoparticles. Trends Biotechnol. 29, 323–332. doi:10.1016/j.tibtech.2011.03.001
Cole, L. E., Ross, R. D., Tilley, J. M., Vargo-Gogola, T., and Roeder, R. K. (2015). Gold nanoparticles as contrast agents in x-ray imaging and computed tomography. Nanomedicine 10, 321–341. doi:10.2217/nnm.14.171
Cortez-Lemus, N. A., and Licea-Claverie, A. (2017). Preparation of a mini-library of thermo-responsive star (NVCL/NVP-VAc) polymers with tailored properties using a hexafunctional xanthate RAFT agent. Polymers 10, 20. doi:10.3390/polym10010020
Court, K. A., Hatakeyama, H., Wu, S. Y., Lingegowda, M. S., Rodriguez-Aguayo, C., Lopez-Berestein, G., et al. (2017). HSP70 inhibition synergistically enhances the effects of magnetic fluid hyperthermia in ovarian cancer. Mol. Cancer Ther. 16, 966–976. doi:10.1158/1535-7163.mct-16-0519
Croissant, J., Salles, D., Maynadier, M., Mongin, O., Hugues, V., Blanchard-Desce, M., et al. (2014). Mixed periodic Mesoporous Organosilica Nanoparticles and core-shell systems, application to in vitro two-photon imaging, therapy, and drug delivery. Chem. Mat. 26, 7214–7220. doi:10.1021/cm5040276
Cui, L., Zhang, F., Wang, Q., Lin, H., Yang, C., Zhang, T., et al. (2015). NIR light responsive core-shell nanocontainers for drug delivery. J. Mat. Chem. B 3, 7046–7054. doi:10.1039/c5tb00709g
Cyphert, E. L., Von Recum, H. A., Yamato, M., and Nakayama, M. (2018). Surface sulfonamide modification of poly(N-isopropylacrylamide)-based block copolymer micelles to alter pH and temperature responsive properties for controlled intracellular uptake. J. Biomed. Mat. Res. A 106, 1552–1560. doi:10.1002/jbm.a.36356
Dadfar, S. M., Roemhild, K., Drude, N. I., Von Stillfried, S., Knüchel, R., Kiessling, F., et al. (2019). Iron oxide nanoparticles: Diagnostic, therapeutic and theranostic applications. Adv. Drug Deliv. Rev. 138, 302–325. doi:10.1016/j.addr.2019.01.005
Das, P., Colombo, M., Prosperi, D. J. C., and Biointerfaces, S. B. (2019). Recent advances in magnetic fluid hyperthermia for cancer therapy. Colloids Surfaces B Biointerfaces 174, 42–55. doi:10.1016/j.colsurfb.2018.10.051
Davaran, S., Lotfipour, F., Sedghipour, N., Sedghipour, M. R., Alimohammadi, S., and Salehi, R. (2015). Preparation and in vivo evaluation of in situ gel system as dual thermo-/pH-responsive nanocarriers for sustained ocular drug delivery. J. Microencapsul. 32, 511–519. doi:10.3109/02652048.2015.1065915
De Maar, J. S., Suelmann, B. B. M., Braat, M., Van Diest, P. J., Vaessen, H. H. B., Witkamp, A. J., et al. (2020). Phase I feasibility study of magnetic resonance guided high intensity focused ultrasound-induced hyperthermia, lyso-thermosensitive liposomal doxorubicin and cyclophosphamide in de novo stage IV breast cancer patients: Study protocol of the i-GO study. BMJ Open 10, e040162. doi:10.1136/bmjopen-2020-040162
De Smet, M., Heijman, E., Langereis, S., Hijnen, N. M., and Grüll, H. (2011). Magnetic resonance imaging of high intensity focused ultrasound mediated drug delivery from temperature-sensitive liposomes: An in vivo proof-of-concept study. J. Control. Release 150, 102–110. doi:10.1016/j.jconrel.2010.10.036
Demirel, G. B., and Von Klitzing, R. (2013). A new multiresponsive drug delivery system using smart nanogels. ChemPhysChem 14, 2833–2840. doi:10.1002/cphc.201300149
Deng, Y., Huang, L., Yang, H., Ke, H., He, H., Guo, Z., et al. (2017). Cyanine‐anchored silica nanochannels for light‐driven synergistic thermo‐chemotherapy. Small 13, 1602747. doi:10.1002/smll.201602747
Deng, Y., Käfer, F., Chen, T., Jin, Q., Ji, J., and Agarwal, S. (2018). Let there be light: Polymeric micelles with upper critical solution temperature as light-triggered heat nanogenerators for combating drug-resistant cancer. Small 14, 1802420. doi:10.1002/smll.201802420
Deng, Y., Zhang, X., Shen, H., He, Q., Wu, Z., Liao, W., et al. (2020). Application of the nano-drug delivery system in treatment of cardiovascular diseases. Front. Bioeng. Biotechnol. 7, 489. doi:10.3389/fbioe.2019.00489
Dhavale, R. P., Dhavale, R., Sahoo, S., Kollu, P., Jadhav, S., Patil, P., et al. (2021). Chitosan coated magnetic nanoparticles as carriers of anticancer drug telmisartan: pH-responsive controlled drug release and cytotoxicity studies. J. Phys. Chem. Solids 148, 109749. doi:10.1016/j.jpcs.2020.109749
Ding, B., Shao, S., Yu, C., Teng, B., Wang, M., Cheng, Z., et al. (2018). Large-pore mesoporous-silica-coated upconversion nanoparticles as multifunctional immunoadjuvants with ultrahigh photosensitizer and antigen loading efficiency for improved cancer photodynamic immunotherapy. Adv. Mat. 30, 1802479. doi:10.1002/adma.201802479
Ding, J., Zhao, L., Li, D., Xiao, C., Zhuang, X., and Chen, X. (2013). Thermo-responsive "hairy-rod" polypeptides for smart antitumor drug delivery. Polym. Chem. 4, 3345–3356. doi:10.1039/c3py00144j
Don, T. M., Lu, K. Y., Lin, L. J., Hsu, C. H., Wu, J. Y., and Mi, F. L. (2017). Temperature/pH/Enzyme triple-responsive cationic protein/PAA-b-PNIPAAm nanogels for controlled anticancer drug and photosensitizer delivery against multidrug resistant breast cancer cells. Mol. Pharm. 14, 4648–4660. doi:10.1021/acs.molpharmaceut.7b00737
Dong, Y., Ma, X., Huo, H., Zhang, Q., Qu, F., and Chen, F. (2018). Preparation of quadruple responsive polymeric micelles combining temperature‐, pH‐, redox‐, and UV‐responsive behaviors and its application in controlled release system. J. Appl. Polym. Sci. 135, 46675. doi:10.1002/app.46675
Duan, S., Yang, Y., Zhang, C., Zhao, N., and Xu, F. J. (2017). NIR-responsive polycationic gatekeeper-cloaked hetero-nanoparticles for multimodal imaging-guided triple-combination therapy of cancer. Small 13, 1603133. doi:10.1002/smll.201603133
Elamir, A., Ajith, S., Sawaftah, N. A., Abuwatfa, W., Mukhopadhyay, D., Paul, V., et al. (2021). Ultrasound-triggered herceptin liposomes for breast cancer therapy. Sci. Rep. 11, 7545. doi:10.1038/s41598-021-86860-5
Eltohamy, M., Seo, J.-W., Hwang, J.-Y., Jang, W.-C., Kim, H.-W., and Shin, U. S. (2016). Ionic and thermo-switchable polymer-masked mesoporous silica drug-nanocarrier: High drug loading capacity at 10 C and fast drug release completion at 40 C. Colloids Surfaces B Biointerfaces 144, 229–237. doi:10.1016/j.colsurfb.2016.04.023
Emamzadeh, M., Desmaële, D., Couvreur, P., and Pasparakis, G. (2018). Dual controlled delivery of squalenoyl-gemcitabine and paclitaxel using thermo-responsive polymeric micelles for pancreatic cancer. J. Mat. Chem. B 6, 2230–2239. doi:10.1039/c7tb02899g
Entezar-Almahdi, E., Mohammadi-Samani, S., Tayebi, L., and Farjadian, F. (2020). Recent advances in designing 5-fluorouracil delivery systems: A stepping stone in the safe treatment of colorectal cancer. Int. J. Nanomedicine 15, 5445–5458. doi:10.2147/ijn.s257700
Entzian, K., and Aigner, A. (2021). Drug delivery by ultrasound-responsive nanocarriers for cancer treatment. Pharmaceutics 13, 1135. doi:10.3390/pharmaceutics13081135
Escudero, A., Carrillo-Carrión, C., Zyuzin, M. V., and Parak, W. J. (2016). Luminescent rare-earth-based nanoparticles: A summarized overview of their synthesis, functionalization, and applications. Top. Curr. Chem. (Cham). 374, 48. doi:10.1007/s41061-016-0049-8
Falireas, P. G., and Vamvakaki, M. (2018). Triple-responsive block copolymer micelles with synergistic pH and temperature response. Macromolecules 51, 6848–6858. doi:10.1021/acs.macromol.8b00810
Fang, J., Šubr, V., Islam, W., Hackbarth, S., Islam, R., Etrych, T., et al. (2018). N-(2-hydroxypropyl)methacrylamide polymer conjugated pyropheophorbide-a, a promising tumor-targeted theranostic probe for photodynamic therapy and imaging. Eur. J. Pharm. Biopharm. 130, 165–176. doi:10.1016/j.ejpb.2018.06.005
Farjadian, F., Ahmadpour, P., Samani, S. M., and Hosseini, M. (2015). Controlled size synthesis and application of nanosphere MCM-41 as potent adsorber of drugs: A novel approach to new antidote agent for intoxication. Microporous Mesoporous Mater. 213, 30–39. doi:10.1016/j.micromeso.2015.04.002
Farjadian, F., Ghasemi, A., Gohari, O., Roointan, A., Karimi, M., and Hamblin, M. R. (2019a). Nanopharmaceuticals and nanomedicines currently on the market: Challenges and opportunities. Nanomedicine 14, 93–126. doi:10.2217/nnm-2018-0120
Farjadian, F., Ghasemi, S., Andami, Z., and Tamami, B. (2020). Thermo-responsive nanocarrier based on poly (N-isopropylacrylamide) serving as a smart doxorubicin delivery system. Iran. Polym. J. 29, 197–207. doi:10.1007/s13726-020-00785-w
Farjadian, F., Ghasemi, S., Heidari, R., and Mohammadi-Samani, S. (2017a). In vitro and in vivo assessment of EDTA-modified silica nano-spheres with supreme capacity of iron capture as a novel antidote agent. Nanomedicine Nanotechnol. Biol. Med. 13, 745–753. doi:10.1016/j.nano.2016.10.012
Farjadian, F., Moghoofei, M., Mirkiani, S., Ghasemi, A., Rabiee, N., Hadifar, S., et al. (2018). Bacterial components as naturally inspired nano-carriers for drug/gene delivery and immunization: Set the bugs to work? Biotechnol. Adv. 36, 968–985. doi:10.1016/j.biotechadv.2018.02.016
Farjadian, F., Moradi, S., and Hosseini, M. (2017b). Thin chitosan films containing super-paramagnetic nanoparticles with contrasting capability in magnetic resonance imaging. J. Mat. Sci. Mat. Med. 28, 47–10. doi:10.1007/s10856-017-5854-2
Farjadian, F., Rezaeifard, S., Naeimi, M., Ghasemi, S., Mohammadi-Samani, S., Welland, M. E., et al. (2019b). Temperature and pH-responsive nano-hydrogel drug delivery system based on lysine-modified poly (vinylcaprolactam). Int. J. Nanomedicine 14, 6901–6915. doi:10.2147/ijn.s214467
Farjadian, F., Roointan, A., Mohammadi-Samani, S., and Hosseini, M. (2019c). Mesoporous silica nanoparticles: Synthesis, pharmaceutical applications, biodistribution, and biosafety assessment. Chem. Eng. J. 359, 684–705. doi:10.1016/j.cej.2018.11.156
Farshbaf, M., Salehi, R., Annabi, N., Khalilov, R., Akbarzadeh, A., and Davaran, S. (2018). pH- and thermo-sensitive MTX-loaded magnetic nanocomposites: synthesis, characterization, and in vitro studies on A549 lung cancer cell and MR imaging. Drug Dev. Industrial Pharm. 44, 452–462. doi:10.1080/03639045.2017.1397686
Fathi, M., Barar, J., Erfan-Niya, H., and Omidi, Y. J. I. J. O. B. M. (2020). Methotrexate-conjugated chitosan-grafted pH-and thermo-responsive magnetic nanoparticles for targeted therapy of ovarian cancer. Int. J. Biol. Macromol. 154, 1175–1184. doi:10.1016/j.ijbiomac.2019.10.272
Feng, Y., Li, N. X., Yin, H. L., Chen, T. Y., Yang, Q., and Wu, M. (2019). Thermo- and pH-responsive, lipid-coated, mesoporous silica nanoparticle-based dual drug delivery system to improve the antitumor effect of hydrophobic drugs. Mol. Pharm. 16, 422–436. doi:10.1021/acs.molpharmaceut.8b01073
Fitzpatrick, S. D., Fitzpatrick, L. E., Thakur, A., Mazumder, M. A., and Sheardown, H. (2012). Temperature-sensitive polymers for drug delivery. Expert Rev. Med. Devices 9, 339–351. doi:10.1586/erd.12.24
Franckena, M., Wit, R. D., Ansink, A. C., Notenboom, A., Canters, R. A., Fatehi, D., et al. (2007). Weekly systemic cisplatin plus locoregional hyperthermia: An effective treatment for patients with recurrent cervical carcinoma in a previously irradiated area. Int. J. Hyperth. 23, 443–450. doi:10.1080/02656730701549359
Gaber, M. H., Hong, K., Huang, S. K., and Papahadjopoulos, D. (1995). Thermosensitive sterically stabilized liposomes: Formulation and in vitro studies on mechanism of doxorubicin release by bovine serum and human plasma. Pharm. Res. 12, 1407–1416. doi:10.1023/a:1016206631006
Gallo, E., Diaferia, C., Di Gregorio, E., Morelli, G., Gianolio, E., and Accardo, A. (2020). Peptide-based soft hydrogels modified with gadolinium complexes as MRI contrast agents. Pharmaceuticals 13, 19. doi:10.3390/ph13020019
Gao, Y., and Dong, C. M. (2018). Triple redox/temperature responsive diselenide‐containing homopolypeptide micelles and supramolecular hydrogels thereof. J. Polym. Sci. Part A Polym. Chem. 56, 1067–1077. doi:10.1002/pola.28984
Gao, Y., Zhong, S., Xu, L., He, S., Dou, Y., Zhao, S., et al. (2019). Mesoporous silica nanoparticles capped with graphene quantum dots as multifunctional drug carriers for photo-thermal and redox-responsive release. Microporous Mesoporous Mater. 278, 130–137. doi:10.1016/j.micromeso.2018.11.030
Gary-Bobo, M., Hocine, O., Brevet, D., Maynadier, M., Raehm, L., Richeter, S., et al. (2012). Cancer therapy improvement with mesoporous silica nanoparticles combining targeting, drug delivery and PDT. Int. J. Pharm. 423, 509–515. doi:10.1016/j.ijpharm.2011.11.045
Gebeyehu, B. T., Lee, A.-W., Huang, S.-Y., Muhabie, A. A., Lai, J.-Y., Lee, D.-J., et al. (2019). Highly stable photosensitive supramolecular micelles for tunable, efficient controlled drug release. Eur. Polym. J. 110, 403–412. doi:10.1016/j.eurpolymj.2018.12.005
Ghamkhari, A., Sarvari, R., Ghorbani, M., and Hamishehkar, H. (2018). Novel thermoresponsive star-liked nanomicelles for targeting of anticancer agent. Eur. Polym. J. 107, 143–154. doi:10.1016/j.eurpolymj.2018.08.008
Ghasemi, S., Owrang, M., Javaheri, F., and Farjadian, F. (2022). Spermine modified PNIPAAm nano-hydrogel serving as thermo-responsive system for delivery of cisplatin. Macromol. Res. 30, 314–324. doi:10.1007/s13233-022-0035-7
Ghasemiyeh, P., and Mohammadi-Samani, S. (2019). Hydrogels as drug delivery systems; pros and cons. Trends Pharm. Sci. 5, 7–24.
Ghasemiyeh, P., and Mohammadi-Samani, S. (2021). Polymers blending as release modulating tool in drug delivery. Front. Mat. 8. doi:10.3389/fmats.2021.752813
Giulbudagian, M., Yealland, G., Honzke, S., Edlich, A., Geisendorfer, B., Kleuser, B., et al. (2018). Breaking the barrier - potent anti-inflammatory activity following efficient topical delivery of etanercept using thermoresponsive nanogels. Theranostics 8, 450–463. doi:10.7150/thno.21668
Gnanasammandhan, M. K., Idris, N. M., Bansal, A., Huang, K., and Zhang, Y. (2016). Near-IR photoactivation using mesoporous silica-coated NaYF4:Yb, Er/Tm upconversion nanoparticles. Nat. Protoc. 11, 688–713. doi:10.1038/nprot.2016.035
Gonçalves, C., Pereira, P., and Gama, M. (2010). Self-assembled hydrogel nanoparticles for drug delivery applications. Materials 3, 1420–1460. doi:10.3390/ma3021420
González-Ayón, M. A., Licea-Claverie, A., and Sañudo-Barajas, J. A. (2020). Different strategies for the preparation of galactose-functionalized thermo-responsive nanogels with potential as smart drug delivery systems. Polymers 12, 2150. doi:10.3390/polym12092150
Goto, Y., Ueda, M., Sugikawa, K., Yasuhara, K., and Ikeda, A. (2019). Light-triggered hydrophilic drug release from liposomes through removal of a photolabile protecting group. RSC Adv. 9, 166–171. doi:10.1039/c8ra08584f
Grabowska, M., Grześkowiak, B. F., Rolle, K., and Mrówczyński, R. (2021). “Magnetic nanoparticles as a carrier of dsRNA for gene therapy,” in Bio-carrier vectors (Springer), 69–81.
Gradishar, W. J., Tjulandin, S., Davidson, N., Shaw, H., Desai, N., Bhar, P., et al. (2005). Phase III trial of nanoparticle albumin-bound paclitaxel compared with polyethylated castor oil–based paclitaxel in women with breast cancer. J. Clin. Oncol. 23, 7794–7803. doi:10.1200/jco.2005.04.937
Gregersen, K. A., Hill, Z. B., Gadd, J. C., Fujimoto, B. S., Maly, D. J., and Chiu, D. T. (2010). Intracellular delivery of bioactive molecules using light-addressable nanocapsules. ACS Nano 4, 7603–7611. doi:10.1021/nn102345f
Gu, T., Cheng, L., Gong, F., Xu, J., Li, X., Han, G., et al. (2018). Upconversion composite nanoparticles for tumor hypoxia modulation and enhanced near-infrared-triggered photodynamic therapy. ACS Appl. Mat. Interfaces 10, 15494–15503. doi:10.1021/acsami.8b03238
Guisasola, E., Asín, L., Beola, L., De La Fuente, J. M., Baeza, A., and Vallet-Regí, M. (2018). Beyond traditional hyperthermia: In vivo cancer treatment with magnetic-responsive mesoporous silica nanocarriers. ACS Appl. Mat. Interfaces 10, 12518–12525. doi:10.1021/acsami.8b02398
Guisasola, E., Baeza, A., Talelli, M., Arcos, D., and Vallet-Regi, M. (2016). Design of thermoresponsive polymeric gates with opposite controlled release behaviors. RSC Adv. 6, 42510–42516. doi:10.1039/c6ra02260j
Gundogdu, D., Bütün, V., and Erel‐Göktepe, I. (2018). Preparation of layer‐by‐layer films with remarkably different pH‐stability and release properties using dual responsive block copolymer micelles. Macromol. Chem. Phys. 219, 1800128. doi:10.1002/macp.201800128
Ha, Y., Ko, S., Kim, I., Huang, Y., Mohanty, K., Huh, C., et al. (2018). Recent advances incorporating superparamagnetic nanoparticles into immunoassays. ACS Appl. Nano Mat. 1, 512–521. doi:10.1021/acsanm.7b00025
Haghighi, A. H., Faghih, Z., Khorasani, M. T., and Farjadian, F. (2019). Antibody conjugated onto surface modified magnetic nanoparticles for separation of HER2+ breast cancer cells. J. Magnetism Magnetic Mater. 490, 165479. doi:10.1016/j.jmmm.2019.165479
Haghighi, A. H., Khorasani, M. T., Faghih, Z., and Farjadian, F. (2020). Effects of different quantities of antibody conjugated with magnetic nanoparticles on cell separation efficiency. Heliyon 6, e03677. doi:10.1016/j.heliyon.2020.e03677
Hai, L., Jia, X., He, D., Zhang, A., Wang, T., Cheng, H., et al. (2018). DNA-functionalized hollow mesoporous silica nanoparticles with dual cargo loading for near-infrared responsive synergistic chemo-photothermal treatment of cancer cells. ACS Appl. Nano Mat. 1, 3486–3497. doi:10.1021/acsanm.8b00657
Han, H. D., Shin, B. C., and Choi, H. S. (2006). Doxorubicin-encapsulated thermosensitive liposomes modified with poly(N-isopropylacrylamide-co-acrylamide): Drug release behavior and stability in the presence of serum. Eur. J. Pharm. Biopharm. 62, 110–116. doi:10.1016/j.ejpb.2005.07.006
Han, H., Hou, Y., Chen, X., Zhang, P., Kang, M., Jin, Q., et al. (2020). Metformin-induced stromal depletion to enhance the penetration of gemcitabine-loaded magnetic nanoparticles for pancreatic cancer targeted therapy. J. Am. Chem. Soc. 142, 4944–4954. doi:10.1021/jacs.0c00650
Han, R. L., Shi, J. H., Liu, Z. J., Hou, Y. F., and Wang, Y. (2018). Near-infrared light-triggered hydrophobic-to-hydrophilic switch nanovalve for on-demand cancer therapy. ACS Biomater. Sci. Eng. 4, 3478–3486. doi:10.1021/acsbiomaterials.8b00437
Hanafy, N., El-Kemary, M., and Leporatti, S. (2018). Micelles structure development as a strategy to improve smart cancer therapy. Cancers 10, 238. doi:10.3390/cancers10070238
Hardy, J. G., Larrañeta, E., Donnelly, R. F., Mcgoldrick, N., Migalska, K., Mccrudden, M. T. C., et al. (2016). Hydrogel-forming microneedle arrays made from light-responsive materials for on-demand transdermal drug delivery. Mol. Pharm. 13, 907–914. doi:10.1021/acs.molpharmaceut.5b00807
Hashida, Y., Tanaka, H., Zhou, S., Kawakami, S., Yamashita, F., Murakami, T., et al. (2014). Photothermal ablation of tumor cells using a single-walled carbon nanotube-peptide composite. J. Control. Release 173, 59–66. doi:10.1016/j.jconrel.2013.10.039
Hassanzadeh, F., Farzan, M., Varshosaz, J., Khodarahmi, G. A., Maaleki, S., and Rostami, M. (2017). Poly (ethylene-co-vinyl alcohol)-based polymeric thermo-responsive nanocarriers for controlled delivery of epirubicin to hepatocellular carcinoma. Res. Pharm. Sci. 12, 107–118. doi:10.4103/1735-5362.202449
He, Y., Zhang, Y., Xiao, Y., and Lang, M. (2010). Dual-response nanocarrier based on graft copolymers with hydrazone bond linkages for improved drug delivery. Colloids Surfaces B Biointerfaces 80, 145–154. doi:10.1016/j.colsurfb.2010.05.038
Hejabi, F., Abbaszadeh, M. S., Taji, S., O'neill, A., Farjadian, F., and Doroudian, M. (2022). Nanocarriers: A novel strategy for the delivery of CRISPR/cas systems. Front. Chem. 10, 957572. doi:10.3389/fchem.2022.957572
Hernández Montoto, A., Montes, R., Samadi, A., Gorbe, M., Terrés, J. M., Cao-Milán, R., et al. (2018). Gold nanostars coated with mesoporous silica are effective and nontoxic photothermal agents capable of gate keeping and laser-induced drug release. ACS Appl. Mat. Interfaces 10, 27644–27656. doi:10.1021/acsami.8b08395
Hoseini-Ghahfarokhi, M., Mirkiani, S., Mozaffari, N., Sadatlu, M. a. A., Ghasemi, A., Abbaspour, S., et al. (2020). Applications of graphene and graphene oxide in smart drug/gene delivery: Is the world still flat? Int. J. Nanomedicine 15, 9469–9496. doi:10.2147/ijn.s265876
Hosseini, M., Farjadian, F., and Makhlouf, A. S. H. (2016). “Smart stimuli-responsive nano-sized hosts for drug delivery,” in Industrial applications for intelligent polymers and coatings (Cham: Springer), 1–26.
Hosseinifar, T., Sheybani, S., Abdouss, M., Hassani Najafabadi, S. A., and Shafiee Ardestani, M. (2018). Pressure responsive nanogel base on Alginate-Cyclodextrin with enhanced apoptosis mechanism for colon cancer delivery. J. Biomed. Mat. Res. A 106, 349–359. doi:10.1002/jbm.a.36242
Hou, B., Yang, W., Dong, C., Zheng, B., Zhang, Y., Wu, J., et al. (2017a). Controlled co-release of doxorubicin and reactive oxygen species for synergistic therapy by NIR remote-triggered nanoimpellers. Mater. Sci. Eng. C 74, 94–102. doi:10.1016/j.msec.2017.02.016
Hou, X., Wang, X., Liu, R., Zhang, H., Liu, X., and Zhang, Y. (2017b). Facile synthesis of multifunctional Fe3O4@SiO2@Au magneto-plasmonic nanoparticles for MR/CT dual imaging and photothermal therapy. RSC Adv. 7, 18844–18850. doi:10.1039/c7ra00925a
Hu, C. C., Chaw, J. R., Chen, Y. C., Chen, C. F., and Liu, H. W. (2015a). A novel thermo-responsive nanogel for intraocular drug delivery. J. Comput. Theor. Nanosci. 12, 762–768. doi:10.1166/jctn.2015.3799
Hu, D., Li, Y., Niu, Y., Li, L., He, J., Liu, X., et al. (2014). Photo-responsive reversible micelles based on azobenzene-modified poly (carbonate) s via azide–alkyne click chemistry. RSC Adv. 4, 47929–47936. doi:10.1039/c4ra07345b
Hu, D., Peng, H., Niu, Y., Li, Y., Xia, Y., Li, L., et al. (2015b). Reversibly light-responsive biodegradable poly(carbonate) micelles constructed via CuAAC reaction. J. Polym. Sci. Part A Polym. Chem. 53, 750–760. doi:10.1002/pola.27499
Hu, S. H., Chen, Y. W., Hung, W. T., Chen, I. W., and Chen, S. Y. (2012). Quantum-dot-tagged reduced graphene oxide nanocomposites for bright fluorescence bioimaging and photothermal therapy monitored in situ. Adv. Mat. 24, 1748–1754. doi:10.1002/adma.201104070
Huang, C.-H., Chuang, T.-J., Ke, C.-J., and Yao, C.-H. J. P. (2020). Doxorubicin–Gelatin/Fe3O4–Alginate dual-layer magnetic nanoparticles as targeted anticancer drug delivery vehicles. Polym. (Basel). 12, 1747. doi:10.3390/polym12081747
Huang, P., Song, H., Zhang, Y., Liu, J., Cheng, Z., Liang, X. J., et al. (2017). FRET-enabled monitoring of the thermosensitive nanoscale assembly of polymeric micelles into macroscale hydrogel and sequential cognate micelles release. Biomaterials 145, 81–91. doi:10.1016/j.biomaterials.2017.07.012
Huang, P., Zhang, Y., Wang, W., Zhou, J., Sun, Y., Liu, J., et al. (2015). Co-delivery of doxorubicin and 131I by thermosensitive micellar-hydrogel for enhanced in situ synergetic chemoradiotherapy. J. Control. Release 220, 456–464. doi:10.1016/j.jconrel.2015.11.007
Huang, Q., and Zeng, Z. (2017). A review on real-time 3D ultrasound imaging technology. BioMed Res. Int. 2017, 6027029. doi:10.1155/2017/6027029
Huang, S. K., Stauffer, P. R., Hong, K., Guo, J. W., Phillips, T. L., Huang, A., et al. (1994). Liposomes and hyperthermia in mice: Increased tumor uptake and therapeutic efficacy of doxorubicin in sterically stabilized liposomes. Cancer Res. 54, 2186–2191.
Huo, H., Ma, X., Dong, Y., and Qu, F. (2017). Light/temperature dual-responsive ABC miktoarm star terpolymer micelles for controlled release. Eur. Polym. J. 87, 331–343. doi:10.1016/j.eurpolymj.2016.12.038
Hurley, K. R., Ring, H. L., Etheridge, M., Zhang, J., Gao, Z., Shao, Q., et al. (2016). Predictable heating and positive MRI contrast from a mesoporous silica-coated iron oxide nanoparticle. Mol. Pharm. 13, 2172–2183. doi:10.1021/acs.molpharmaceut.5b00866
Husseini, G. A., De La Rosat, M. a. D., Gabuji, T., Zeng, Y., Christensen, D. A., and Pitt, W. G. (2007). Release of doxorubicin from unstabilized and stabilized micelles under the action of ultrasound. J. Nanosci. Nanotechnol. 7, 1028–1033. doi:10.1166/jnn.2007.218
Indulekha, S., Arunkumar, P., Bahadur, D., and Srivastava, R. (2017). Dual responsive magnetic composite nanogels for thermo-chemotherapy. Colloids Surfaces B Biointerfaces 155, 304–313. doi:10.1016/j.colsurfb.2017.04.035
Jain, S., Coulter, J. A., Hounsell, A. R., Butterworth, K. T., Mcmahon, S. J., Hyland, W. B., et al. (2011). Cell-specific radiosensitization by gold nanoparticles at megavoltage radiation energies. Int. J. Radiat. Oncology*Biology*Physics 79, 531–539. doi:10.1016/j.ijrobp.2010.08.044
Jia, L., Zhong, W., Wen, T., and Bian, F. (2010). Thermo and pH dual-responsive micelles of N-phthaloylchitosan-g-poly(N- isopropylacrylamide) and poly(acrylic acid-co-tert-butyl acrylate) for drug delivery. J. Macromol. Sci. Part A 47, 909–917. doi:10.1080/10601325.2010.501638
Jiang, L., Lei, Z., Vader, P., Hennink, W. E., Engbersen, J. F., and Schiffelers, R. M. (2020a). Poly (amido amine)-based nanogels for delivery of mRNA. Nat. synthetic nanoparticles Deliv. Biol. 17.
Jiang, W., Mo, F., Jin, X., Chen, L., Xu, L. J., Guo, L., et al. (2017). Tumor-targeting photothermal heating-responsive nanoplatform based on reduced graphene oxide/mesoporous silica/hyaluronic acid nanocomposite for enhanced photodynamic therapy. Adv. Mat. Interfaces 4, 1700425. doi:10.1002/admi.201700425
Jiang, Z., Tan, M. L., Taheri, M., Yan, Q., Tsuzuki, T., Gardiner, M. G., et al. (2020b). Strong, self‐healable, and recyclable visible‐light‐responsive hydrogel actuators. Angew. Chem. Int. Ed. Engl. 132, 7115–7122. doi:10.1002/ange.201916058
Jiao, J., Liu, C., Li, X., Liu, J., Di, D., Zhang, Y., et al. (2016). Fluorescent carbon dot modified mesoporous silica nanocarriers for redox-responsive controlled drug delivery and bioimaging. J. colloid interface Sci. 483, 343–352. doi:10.1016/j.jcis.2016.08.033
Jie, L., Lang, D., Kang, X., Yang, Z., Du, Y., Ying, X. J. J. O. N., et al. (2019). Superparamagnetic iron oxide nanoparticles/doxorubicin-loaded starch-octanoic micelles for targeted tumor therapy. J. Nanosci. Nanotechnol. 19, 5456–5462. doi:10.1166/jnn.2019.16548
Jin, X., Wang, Q., Sun, J., Panezai, H., Bai, S., and Wu, X. (2018). P(NIPAM-co-AA)@BMMs with mesoporous silica core and controlled copolymer shell and its fractal characteristics for dual pH- and temperature-responsive performance of ibuprofen release. Int. J. Polym. Mater. Polym. Biomaterials 67, 131–142. doi:10.1080/00914037.2017.1309544
Joglekar, M., and Trewyn, B. G. (2013). Polymer‐based stimuli‐responsive nanosystems for biomedical applications. Biotechnol. J. 8, 931–945. doi:10.1002/biot.201300073
Joshy, K., Augustine, R., Mayeen, A., Alex, S. M., Hasan, A., Thomas, S., et al. (2020). NiFe 2 O 4/poly (ethylene glycol)/lipid–polymer hybrid nanoparticles for anti-cancer drug delivery. New J. Chem. 44, 18162–18172. doi:10.1039/d0nj01163k
Jung, S., Nagel, G., Giulbudagian, M., Calderón, M., Patzelt, A., Knorr, F., et al. (2018). Temperature-enhanced follicular penetration of thermoresponsive nanogels. Z. fur Phys. Chem. 232, 805–817. doi:10.1515/zpch-2017-1080
Jung-Tak, J., Jooyoung, L., Jiyun, S. J. a. M., Ju, E., Kim, M., Kim, Y. I., et al. (2018). Giant magnetic heat induction of magnesium-doped γ-Fe 2 O 3 superparamagnetic nanoparticles for completely killing tumors. Adv. Mat. 30, 1704362. doi:10.1002/adma.201704362
Kageyama, S., Wada, H., Muro, K., Niwa, Y., Ueda, S., Miyata, H., et al. (2013). Dose-dependent effects of NY-ESO-1 protein vaccine complexed with cholesteryl pullulan (CHP-NY-ESO-1) on immune responses and survival benefits of esophageal cancer patients. J. Transl. Med. 11, 246. doi:10.1186/1479-5876-11-246
Kalaiarasi, S., Arjun, P., Nandhagopal, S., Brijitta, J., Iniyan, A. M., Vincent, S. G. P., et al. (2016). Development of biocompatible nanogel for sustained drug release by overcoming the blood brain barrier in zebrafish model. J. Appl. Biomed. 14, 157–169. doi:10.1016/j.jab.2016.01.004
Kalluru, P., Vankayala, R., Chiang, C. S., and Hwang, K. C. (2016). Unprecedented “all-in-one” lanthanide-doped mesoporous silica frameworks for fluorescence/MR imaging and combination of NIR light triggered chemo-photodynamic therapy of tumors. Adv. Funct. Mat. 26, 7908–7920. doi:10.1002/adfm.201603749
Kang, J.-H., Hwang, J.-Y., Seo, J.-W., Kim, H.-S., and Shin, U. S. (2018). Small intestine-and colon-specific smart oral drug delivery system with controlled release characteristic. Mater. Sci. Eng. C 91, 247–254. doi:10.1016/j.msec.2018.05.052
Kankala, R. K., Han, Y. H., Na, J., Lee, C. H., Sun, Z., Wang, S. B., et al. (2020). Nanoarchitectured structure and surface biofunctionality of mesoporous silica nanoparticles. Adv. Mat. 32, 1907035. doi:10.1002/adma.201907035
Kano, K., Tanaka, Y., Ogawa, T., Shimomura, M., and Kunitake, T. (1981). Photoresponsive artificial membrane. Regulation of membrane permeability of liposomal membrane by photoreversible cis‐trans isomerization of azobenzenes. Photochem. Photobiol. 34, 323–329. doi:10.1111/j.1751-1097.1981.tb09004.x
Karimi, Z., Abbasi, S., Shokrollahi, H., Yousefi, G., Fahham, M., Karimi, L., et al. (2017). Pegylated and amphiphilic Chitosan coated manganese ferrite nanoparticles for pH-sensitive delivery of methotrexate: Synthesis and characterization. Mater. Sci. Eng. C 71, 504–511. doi:10.1016/j.msec.2016.10.008
Kauscher, U., Holme, M. N., Björnmalm, M., and Stevens, M. M. J. a. D. D. R. (2019). Physical stimuli-responsive vesicles in drug delivery: Beyond liposomes and polymersomes. Adv. Drug Deliv. Rev. 138, 259–275. doi:10.1016/j.addr.2018.10.012
Kesharwani, S. S., Kaur, S., Tummala, H., and Sangamwar, A. T. (2018). Multifunctional approaches utilizing polymeric micelles to circumvent multidrug resistant tumors. Colloids Surfaces B Biointerfaces 173, 581–590. doi:10.1016/j.colsurfb.2018.10.022
Kesisoglou, F., Panmai, S., and Wu, Y. (2007). Nanosizing—Oral formulation development and biopharmaceutical evaluation. Adv. drug Deliv. Rev. 59, 631–644. doi:10.1016/j.addr.2007.05.003
Khatun, Z., Nurunnabi, M., Nafiujjaman, M., Reeck, G. R., Khan, H. A., Cho, K. J., et al. (2015). A hyaluronic acid nanogel for photo-chemo theranostics of lung cancer with simultaneous light-responsive controlled release of doxorubicin. Nanoscale 7, 10680–10689. doi:10.1039/c5nr01075f
Khine, Y. Y., Jiang, Y., Dag, A., Lu, H., and Stenzel, M. H. (2015). Dual-responsive pH and temperature sensitive nanoparticles based on methacrylic acid and di(ethylene glycol) methyl ether methacrylate for the triggered release of drugs. Macromol. Biosci. 15, 1091–1104. doi:10.1002/mabi.201500057
Khoobehi, B., Char, C. A., Peyman, G. A., and Schuele, K. M. (1990). Study of the mechanisms of laser-induced release of liposome-encapsulated dye. Lasers Surg. Med. 10, 303–309. doi:10.1002/lsm.1900100312
Khoobehi, B., Peyman, G. A., Bhatt, N., and Moshfeghi, D. (1992). Laser-induced experimental vascular occlusion using liposome-encapsulated ADP. Lasers Surg. Med. 12, 609–614. doi:10.1002/lsm.1900120607
Kiessling, F., Mertens, M. E., Grimm, J., and Lammers, T. (2014). Nanoparticles for imaging: Top or flop? Radiology 273, 10–28. doi:10.1148/radiol.14131520
Kitano, S., Kageyama, S., Nagata, Y., Miyahara, Y., Hiasa, A., Naota, H., et al. (2006). HER2-specific T-cell immune responses in patients vaccinated with truncated HER2 protein complexed with nanogels of cholesteryl pullulan. Clin. Cancer Res. 12, 7397–7405. doi:10.1158/1078-0432.ccr-06-1546
Knežević, N. Ž., and Lin, V. S.-Y. (2013). A magnetic mesoporous silica nanoparticle-based drug delivery system for photosensitive cooperative treatment of cancer with a mesopore-capping agent and mesopore-loaded drug. Nanoscale 5, 1544–1551. doi:10.1039/c2nr33417h
Knežević, N. Z. (2013). Visible light responsive anticancer treatment with an amsacrine-loaded mesoporous silica-based nanodevice. RSC Adv. 3, 19388–19392. doi:10.1039/c3ra43492c
Kodama, R. J. J. O. M., and Materials, M. (1999). Magnetic nanoparticles. J. Magn. Magn. Mat. 200, 359–372. doi:10.1016/s0304-8853(99)00347-9
Kong, G., Anyarambhatla, G., Petros, W. P., Braun, R. D., Colvin, O. M., Needham, D., et al. (2000a). Efficacy of liposomes and hyperthermia in a human tumor xenograft model: Importance of triggered drug release. Cancer Res. 60, 6950–6957.
Kong, G., Braun, R. D., and Dewhirst, M. W. (2000b). Hyperthermia enables tumor-specific nanoparticle delivery: Effect of particle size. Cancer Res. 60, 4440–4445.
Kurd, K., Khandagi, A. A., Davaran, S., and Akbarzadeh, A. (2016). Cisplatin release from dual-responsive magnetic nanocomposites. Artif. Cells Nanomed. Biotechnol. 44, 1031–1039. doi:10.3109/21691401.2015.1008513
Landon, C. D., Park, J.-Y., Needham, D., and Dewhirst, M. W. (2011). Nanoscale drug delivery and hyperthermia: The materials design and preclinical and clinical testing of low temperature-sensitive liposomes used in combination with mild hyperthermia in the treatment of local cancer. Open nanomed. J. 3, 24–37. doi:10.2174/1875933501103010038
Le, P. N., Nguyen, N. H., Nguyen, C. K., and Tran, N. Q. (2016). Smart dendrimer-based nanogel for enhancing 5-fluorouracil loading efficiency against MCF7 cancer cell growth. Bull. Mat. Sci. 39, 1493–1500. doi:10.1007/s12034-016-1274-z
Lee, R. S., Chen, W. H., and Huang, Y. T. (2010). Synthesis and characterization of dual stimuli-responsive block copolymers based on poly(N-isopropylacrylamide)-b-poly(pseudoamino acid). Polymer 51, 5942–5951. doi:10.1016/j.polymer.2010.10.001
Lee, S. F., Zhu, X. M., Wang, Y. X. J., Xuan, S. H., You, Q., Chan, W. H., et al. (2013). Ultrasound, pH, and magnetically responsive crown-ether-coated core/shell nanoparticles as drug encapsulation and release systems. ACS Appl. Mat. Interfaces 5, 1566–1574. doi:10.1021/am4004705
Lei, Q., Wang, S. B., Hu, J. J., Lin, Y. X., Zhu, C. H., Rong, L., et al. (2017). Stimuli-responsive "cluster bomb" for programmed tumor therapy. ACS Nano 11, 7201–7214. doi:10.1021/acsnano.7b03088
Lencioni, R., and Cioni, D. (2016). RFA plus lyso-thermosensitive liposomal doxorubicin: In search of the optimal approach to cure intermediate-size hepatocellular carcinoma. Hepat. Oncol. 3, 193–200. doi:10.2217/hep-2016-0005
Lerra, L., Farfalla, A., Sanz, B., Cirillo, G., Vittorio, O., Voli, F., et al. (2018). Graphene oxide functional nanohybrids with magnetic nanoparticles for improved vectorization of doxorubicin to neuroblastoma cells. Pharmaceutics 11, 3. doi:10.3390/pharmaceutics11010003
Leung, S. J., and Romanowski, M. (2012). Light-activated content release from liposomes. Theranostics 2, 1020–1036. doi:10.7150/thno.4847
Li, A., Zhao, J., Fu, J., Cai, J., and Zhang, P. (2021a). Recent advances of biomimetic nano-systems in the diagnosis and treatment of tumor. Asian J. Pharm. Sci. 16, 161–174. doi:10.1016/j.ajps.2019.08.001
Li, C., Zhang, Y., Li, Z., Mei, E., Lin, J., Li, F., et al. (2018a). Theranostics: Light-Responsive biodegradable nanorattles for cancer theranostics (adv. Mater. 8/2018). Adv. Mat. 30, 1870049. doi:10.1002/adma.201870049
Li, F., Xie, C., Cheng, Z., and Xia, H. (2016a). Ultrasound responsive block copolymer micelle of poly (ethylene glycol)–poly (propylene glycol) obtained through click reaction. Ultrason. sonochemistry 30, 9–17. doi:10.1016/j.ultsonch.2015.11.023
Li, H., Li, J., Ke, W., and Ge, Z. (2015). A near-infrared photothermal effect-responsive drug delivery system based on indocyanine green and doxorubicin-loaded polymeric micelles mediated by reversible diels-alder reaction. Macromol. Rapid Commun. 36, 1841–1849. doi:10.1002/marc.201500337
Li, L., Lu, Y., Jiang, C., Zhu, Y., Yang, X., Hu, X., et al. (2018b). Actively targeted deep tissue imaging and photothermal‐chemo therapy of breast cancer by antibody‐functionalized drug‐loaded X‐ray‐responsive bismuth sulfide@ mesoporous silica core–shell nanoparticles. Adv. Funct. Mat. 28, 1704623. doi:10.1002/adfm.201704623
Li, L., Yang, W. W., and Xu, D. G. (2019). Stimuli-responsive nanoscale drug delivery systems for cancer therapy. J. Drug Target 27, 423–433. doi:10.1080/1061186X.2018.1519029
Li, M., Li, Z., Yang, Y., Wang, Z., Yang, Z., Li, B., et al. (2016b). Thermo-sensitive liposome co-loaded of vincristine and doxorubicin based on their similar physicochemical properties had synergism on tumor treatment. Pharm. Res. 33, 1881–1898. doi:10.1007/s11095-016-1924-2
Li, N., Zhao, L., Qi, L., Li, Z., and Luan, Y. (2016c). Polymer assembly: Promising carriers as co-delivery systems for cancer therapy. Prog. Polym. Sci. 58, 1–26. doi:10.1016/j.progpolymsci.2015.10.009
Li, P., Zhang, Z., Su, Z., and Wei, G. (2017). Thermosensitive polymeric micelles based on the triblock copolymer poly(d, l-lactide)-b-poly(N-isopropyl acrylamide)-b-poly(d, l-lactide) for controllable drug delivery. J. Appl. Polym. Sci. 134, 45304. doi:10.1002/app.45304
Li, X., Xie, C., Xia, H., and Wang, Z. (2018c). pH and ultrasound dual-responsive polydopamine-coated mesoporous silica nanoparticles for controlled drug delivery. Langmuir 34, 9974–9981. doi:10.1021/acs.langmuir.8b01091
Li, Y., Lv, W., Wang, L., Zhang, Y., Yang, L., Wang, T., et al. (2021b). Photo-triggered nucleus targeting for cancer drug delivery. Nano Res. 14, 2630–2636. doi:10.1007/s12274-020-3264-0
Li, Y., Qian, Y., Liu, T., Zhang, G., and Liu, S. (2012). Light-triggered concomitant enhancement of magnetic resonance imaging contrast performance and drug release rate of functionalized amphiphilic diblock copolymer micelles. Biomacromolecules 13, 3877–3886. doi:10.1021/bm301425j
Li, Y., Yu, A., Li, L., and Zhai, G. (2018d). The development of stimuli-responsive polymeric micelles for effective delivery of chemotherapeutic agents. J. drug Target. 26, 753–765. doi:10.1080/1061186x.2017.1419477
Li, Y., Zheng, X., Wu, K., and Lu, M. (2016d). Synthesis and self-assembly of a dual thermal and pH-responsive ternary graft copolymer for sustained release drug delivery. RSC Adv. 6, 2571–2581. doi:10.1039/c5ra23625h
Li, Z., Dong, K., Huang, S., Ju, E., Liu, Z., Yin, M., et al. (2014). A smart nanoassembly for multistage targeted drug delivery and magnetic resonance imaging. Adv. Funct. Mat. 24, 3612–3620. doi:10.1002/adfm.201303662
Li, Z., Wang, H., Chen, Y., Wang, Y., Li, H., Han, H., et al. (2016e). pH- and NIR light-responsive polymeric prodrug micelles for hyperthermia-assisted site-specific chemotherapy to reverse drug resistance in cancer treatment. Small 12, 2731–2740. doi:10.1002/smll.201600365
Lim, H. L., Hwang, Y., Kar, M., and Varghese, S. (2014). Smart hydrogels as functional biomimetic systems. Biomater. Sci. 2, 603–618. doi:10.1039/c3bm60288e
Limmer, S., Hahn, J., Schmidt, R., Wachholz, K., Zengerle, A., Lechner, K., et al. (2014). Gemcitabine treatment of rat soft tissue sarcoma with phosphatidyldiglycerol-based thermosensitive liposomes. Pharm. Res. 31, 2276–2286. doi:10.1007/s11095-014-1322-6
Lin, J., Li, Y., Li, Y., Wu, H., Yu, F., Zhou, S., et al. (2015). Drug/dye-loaded, multifunctional PEG–chitosan–iron oxide nanocomposites for methotraxate synergistically self-targeted cancer therapy and dual model imaging. ACS Appl. Mat. Interfaces 7, 11908–11920. doi:10.1021/acsami.5b01685
Lin, Y.-K., Fang, J.-Y., Wang, S.-W., and Lee, R.-S. (2018). Synthesis and characterization of triple-responsive PNiPAAm-S-S-P(αN 3 CL-g-alkyne) copolymers bearing cholesterol and fluorescence monitor. React. Funct. Polym. 130, 29–42. doi:10.1016/j.reactfunctpolym.2018.05.008
Lin, Y. K., Wang, S. W., Yu, Y. C., and Lee, R. S. (2017). Thermoresponsive and acid-cleavable amphiphilic copolymer micelles for controlled drug delivery. Int. J. Polym. Mater. Polym. Biomaterials 66, 943–954. doi:10.1080/00914037.2017.1291514
Ling, Y., Tang, X., Wang, F., Zhou, X., Wang, R., Deng, L., et al. (2017). Highly efficient magnetic hyperthermia ablation of tumors using injectable polymethylmethacrylate–Fe 3 O 4. RSC Adv. 7, 2913–2918. doi:10.1039/c6ra20860f
Liong, M., Lu, J., Kovochich, M., Xia, T., Ruehm, S. G., Nel, A. E., et al. (2008). Multifunctional inorganic nanoparticles for imaging, targeting, and drug delivery. ACS Nano 2, 889–896. doi:10.1021/nn800072t
Liu, G., Gao, J., Ai, H., and Chen, X. J. S. (2013a). Applications and potential toxicity of magnetic iron oxide nanoparticles. Small 9, 1533–1545. doi:10.1002/smll.201201531
Liu, G., Ma, J., Li, Y., Li, Q., Tan, C., Song, H., et al. (2017a). Core-interlayer-shell Fe3O4@mSiO2@lipid-PEG-methotrexate nanoparticle for multimodal imaging and multistage targeted chemo-photodynamic therapy. Int. J. Pharm. 521, 19–32. doi:10.1016/j.ijpharm.2017.01.068
Liu, H., Li, C., Tang, D., An, X., Guo, Y., and Zhao, Y. (2015). Multi-responsive graft copolymer micelles comprising acetal and disulfide linkages for stimuli-triggered drug delivery. J. Mat. Chem. B 3, 3959–3971. doi:10.1039/c5tb00473j
Liu, J., Bu, W., Pan, L., and Shi, J. (2013b). NIR-triggered anticancer drug delivery by upconverting nanoparticles with integrated azobenzene-modified mesoporous silica. Angew. Chem. Int. Ed. Engl. 52, 4471–4475. doi:10.1002/ange.201300183
Liu, J., Liang, H., Li, M., Luo, Z., Zhang, J., Guo, X., et al. (2018a). Tumor acidity activating multifunctional nanoplatform for NIR-mediated multiple enhanced photodynamic and photothermal tumor therapy. Biomaterials 157, 107–124. doi:10.1016/j.biomaterials.2017.12.003
Liu, N., Chen, Z., Dunphy, D. R., Jiang, Y. B., Assink, R. A., and Brinker, C. J. (2003). Photoresponsive nanocomposite formed by self‐assembly of an azobenzene‐modified silane. Angew. Chem. Int. Ed. 42, 1731–1734. doi:10.1002/anie.200250189
Liu, X., Shou, D., Chen, C., Mao, H., Kong, Y., and Tao, Y. (2017b). Core-shell structured polypyrrole/mesoporous SiO2 nanocomposite capped with graphene quantum dots as gatekeeper for irradiation-controlled release of methotrexate. Mater. Sci. Eng. C 81, 206–212. doi:10.1016/j.msec.2017.08.001
Liu, X., Yang, T., Han, Y., Zou, L., Yang, H., Jiang, J., et al. (2018b). In situ growth of CuS/SiO2-based multifunctional nanotherapeutic agents for combined photodynamic/photothermal cancer therapy. ACS Appl. Mat. Interfaces 10, 31008–31018. doi:10.1021/acsami.8b10339
Lu, N., Tian, Y., Tian, W., Huang, P., Liu, Y., Tang, Y., et al. (2016). Smart cancer cell targeting imaging and drug delivery system by systematically engineering periodic mesoporous organosilica nanoparticles. ACS Appl. Mat. Interfaces 8, 2985–2993. doi:10.1021/acsami.5b09585
Lu, W., Zhang, G., Zhang, R., Flores, L. G., Huang, Q., Gelovani, J. G., et al. (2010). Tumor site–specific silencing of NF-κB p65 by targeted hollow gold nanosphere–mediated photothermal transfection. Cancer Res. 70, 3177–3188. doi:10.1158/0008-5472.can-09-3379
Lu, Y., Li, L., Lin, Z., Li, M., Hu, X., Zhang, Y., et al. (2018). Enhancing osteosarcoma killing and CT imaging using ultrahigh drug loading and NIR-responsive bismuth Sulfide@Mesoporous silica nanoparticles. Adv. Healthc. Mat. 7, 1800602. doi:10.1002/adhm.201800602
Luckanagul, J. A., Pitakchatwong, C., Ratnatilaka Na Bhuket, P., Muangnoi, C., Rojsitthisak, P., Chirachanchai, S., et al. (2018). Chitosan-based polymer hybrids for thermo-responsive nanogel delivery of curcumin. Carbohydr. Polym. 181, 1119–1127. doi:10.1016/j.carbpol.2017.11.027
Luo, Y.-L., Zhang, X.-Y., Fu, J.-Y., Xu, F., and Chen, Y.-S. (2017). Novel temperature and pH dual-sensitive PNIPAM/CMCS/MWCNT semi-IPN nanohybrid hydrogels: Synthesis, characterization, and DOX drug release. Int. J. Polym. Mater. Polym. Biomaterials 66, 398–409. doi:10.1080/00914037.2016.1233418
Lux, J., White, A. G., Chan, M., Anderson, C. J., and Almutairi, A. (2015). Nanogels from metal-chelating crosslinkers as versatile platforms applied to copper-64 PET imaging of tumors and metastases. Theranostics 5, 277–288. doi:10.7150/thno.10904
Lv, R., Yang, P., Chen, G., Gai, S., Xu, J., and Prasad, P. N. (2017). Dopamine-mediated photothermal theranostics combined with up-conversion platform under near infrared light. Sci. Rep. 7, 13562. doi:10.1038/s41598-017-13284-5
Mackanos, M. A., Larabi, M., Shinde, R., Simanovskii, D. M., Guccione, S., and Contag, C. H. (2009). Laser-induced disruption of systemically administered liposomes for targeted drug delivery. J. Biomed. Opt. 14, 044009. doi:10.1117/1.3174410
Madhusudana Rao, K., Mallikarjuna, B., Krishna Rao, K. S. V., Siraj, S., Chowdoji Rao, K., and Subha, M. C. S. (2013). Novel thermo/pH sensitive nanogels composed from poly(N-vinylcaprolactam) for controlled release of an anticancer drug. Colloids Surfaces B Biointerfaces 102, 891–897. doi:10.1016/j.colsurfb.2012.09.009
Mai, B. T., Balakrishnan, P. B., Barthel, M. J., Piccardi, F., Niculaes, D., Marinaro, F., et al. (2019). Thermoresponsive iron oxide nanocubes for an effective clinical translation of magnetic hyperthermia and heat-mediated chemotherapy. ACS Appl. Mater. Interfaces 11, 5727–5739. doi:10.1021/acsami.8b16226
Maiti, D., Chao, Y., Dong, Z., Yi, X., He, J., Liu, Z., et al. (2018). Development of a thermosensitive protein conjugated nanogel for enhanced radio-chemotherapy of cancer. Nanoscale 10, 13976–13985. doi:10.1039/c8nr03986k
Mal, N. K., Fujiwara, M., and Tanaka, Y. (2003). Photocontrolled reversible release of guest molecules from coumarin-modified mesoporous silica. Nature 421, 350–353. doi:10.1038/nature01362
Marin, A., Sun, H., Husseini, G. A., Pitt, W. G., Christensen, D. A., and Rapoport, N. Y. (2002). Drug delivery in pluronic micelles: Effect of high-frequency ultrasound on drug release from micelles and intracellular uptake. J. Control. Release 84, 39–47. doi:10.1016/s0168-3659(02)00262-6
Mauri, E., Giannitelli, S. M., Trombetta, M., and Rainer, A. (2021). Synthesis of nanogels: Current trends and future outlook. Gels 7, 36. doi:10.3390/gels7020036
Mauriello Jimenez, C., Aggad, D., Croissant, J. G., Tresfield, K., Laurencin, D., Berthomieu, D., et al. (2018). Photodynamic therapy: Porous porphyrin-based organosilica nanoparticles for NIR two-photon photodynamic therapy and gene delivery in zebrafish (adv. Funct. Mater. 21/2018). Adv. Funct. Mat. 28, 1870143. doi:10.1002/adfm.201870143
Meir, R., Shamalov, K., Betzer, O., Motiei, M., Horovitz-Fried, M., Yehuda, R., et al. (2015). Nanomedicine for cancer immunotherapy: Tracking cancer-specific T-cells in vivo with gold nanoparticles and CT imaging. ACS Nano 9, 6363–6372. doi:10.1021/acsnano.5b01939
Meng, L., Huang, W., Wang, D., Huang, X., Zhu, X., and Yan, D. (2013). Chitosan-based nanocarriers with pH and light dual response for anticancer drug delivery. Biomacromolecules 14, 2601–2610. doi:10.1021/bm400451v
Meyer, D. E., Shin, B., Kong, G., Dewhirst, M., and Chilkoti, A. J. J. O. C. R. (2001). Drug targeting using thermally responsive polymers and local hyperthermia. J. Control. Release 74, 213–224. doi:10.1016/s0168-3659(01)00319-4
Mitra, A. K., Mandal, A., and Cholkar, K. (2017). Emerging nanotechnologies for diagnostics, drug delivery and medical devices.
Mohammadi, H., Heidari, R., Niknezhad, S. V., Jamshidzadeh, A., and Farjadian, F. (2020a). In vitro and in vivo evaluation of succinic acid-substituted mesoporous silica for ammonia adsorption: Potential application in the management of hepatic encephalopathy. Int. J. Nanomedicine 15, 10085–10098. doi:10.2147/ijn.s271883
Mohammadi, M., Arabi, L., and Alibolandi, M. (2020b). Doxorubicin-loaded composite nanogels for cancer treatment. J. Control. Release 328, 171–191. doi:10.1016/j.jconrel.2020.08.033
Molina, M., Asadian-Birjand, M., Balach, J., Bergueiro, J., Miceli, E., and Calderon, M. (2015). Stimuli-responsive nanogel composites and their application in nanomedicine. Chem. Soc. Rev. 44, 6161–6186. doi:10.1039/c5cs00199d
Molina, M., Wedepohl, S., Miceli, E., and Calderón, M. (2017). Overcoming drug resistance with on-demand charged thermoresponsive dendritic nanogels. Nanomedicine 12, 117–129. doi:10.2217/nnm-2016-0308
Mondal, S., Manivasagan, P., Bharathiraja, S., Santha Moorthy, M., Nguyen, V. T., Kim, H. H., et al. (2017). Hydroxyapatite coated iron oxide nanoparticles: A promising nanomaterial for magnetic hyperthermia cancer treatment. Nanomater. (Basel). 7, 426. doi:10.3390/nano7120426
Moodley, T., and Singh, M. (2021). Current stimuli-responsive mesoporous silica nanoparticles for cancer therapy. Pharmaceutics 13, 71. doi:10.3390/pharmaceutics13010071
Moradi Kashkooli, F., Soltani, M., and Souri, M. (2020). Controlled anti-cancer drug release through advanced nano-drug delivery systems: Static and dynamic targeting strategies. J. Control. Release 327, 316–349. doi:10.1016/j.jconrel.2020.08.012
Movassaghian, S., Merkel, O. M., and Torchilin, V. P. (2015). Applications of polymer micelles for imaging and drug delivery. WIREs. Nanomed. Nanobiotechnol. 7, 691–707. doi:10.1002/wnan.1332
Murguia-Favela, L., Min, W., Loves, R., Leon-Ponte, M., and Grunebaum, E. (2020). Comparison of elapegademase and pegademase in ADA-deficient patients and mice. Clin. Exp. Immunol. 200, 176–184. doi:10.1111/cei.13420
Nakayama, M., Akimoto, J., and Okano, T. (2014). Polymeric micelles with stimuli-triggering systems for advanced cancer drug targeting. J. drug Target. 22, 584–599. doi:10.3109/1061186x.2014.936872
Narayan, R., Nayak, U. Y., Raichur, A. M., and Garg, S. (2018). Mesoporous silica nanoparticles: A comprehensive review on synthesis and recent advances. Pharmaceutics 10, 118. doi:10.3390/pharmaceutics10030118
Neamtu, I., Rusu, A. G., Diaconu, A., Nita, L. E., and Chiriac, A. P. (2017). Basic concepts and recent advances in nanogels as carriers for medical applications. Drug Deliv. 24, 539–557. doi:10.1080/10717544.2016.1276232
Needham, D., Anyarambhatla, G., Kong, G., and Dewhirst, M. W. (2000). A new temperature-sensitive liposome for use with mild hyperthermia: Characterization and testing in a human tumor xenograft model. Cancer Res. 60, 1197–1201.
Nesbitt, A., Fossati, G., Bergin, M., Stephens, P., Stephens, S., Foulkes, R., et al. (2007). Mechanism of action of certolizumab pegol (CDP870): In vitro comparison with other anti-tumor necrosis factor α agents. Inflamm. bowel Dis. 13, 1323–1332. doi:10.1002/ibd.20225
Ng, K. K., Weersink, R. A., Lim, L., Wilson, B. C., and Zheng, G. (2016). Controlling spatial heat and light distribution by using photothermal enhancing auto-regulated liposomes (PEARLs). Angew. Chem. Int. Ed. Engl. 55, 10157–10161. doi:10.1002/ange.201605241
Nguyen, N. T., Nguyen, N. N. T., Tran, N. T. N., Le, P. N., Nguyen, T. B. T., Nguyen, N. H., et al. (2018). Synergic activity against MCF-7 breast cancer cell growth of nanocurcumin-encapsulated and cisplatin-complexed nanogels. Molecules 23, 3347. doi:10.3390/molecules23123347
Niu, N., He, F., Ma, P., Gai, S., Yang, G., Qu, F., et al. (2014a). Up-conversion nanoparticle assembled mesoporous silica composites: Synthesis, plasmon-enhanced luminescence, and near-infrared light triggered drug release. ACS Appl. Mat. Interfaces 6, 3250–3262. doi:10.1021/am500325w
Niu, Y., Li, Y., Lu, Y., and Xu, W. (2014b). Spiropyran-decorated light-responsive amphiphilic poly (α-hydroxy acids) micelles constructed via a CuAAC reaction. RSC Adv. 4, 58432–58439. doi:10.1039/c4ra11550c
Omlor, A. J., Nguyen, J., Bals, R., and Dinh, Q. T. (2015). Nanotechnology in respiratory medicine. Respir. Res. 16, 64. doi:10.1186/s12931-015-0223-5
Paasonen, L., Laaksonen, T., Johans, C., Yliperttula, M., Kontturi, K., and Urtti, A. (2007). Gold nanoparticles enable selective light-induced contents release from liposomes. J. Control. release 122, 86–93. doi:10.1016/j.jconrel.2007.06.009
Panja, S., Dey, G., Bharti, R., Kumari, K., Maiti, T. K., Mandal, M., et al. (2016a). Tailor-made temperature-sensitive micelle for targeted and on-demand release of anticancer drugs. ACS Appl. Mat. Interfaces 8, 12063–12074. doi:10.1021/acsami.6b03820
Panja, S., Dey, G., Bharti, R., Mandal, P., Mandal, M., and Chattopadhyay, S. (2016b). Metal ion ornamented ultrafast light-sensitive nanogel for potential in vivo cancer therapy. Chem. Mat. 28, 8598–8610. doi:10.1021/acs.chemmater.6b03440
Parida, S., Maiti, C., Rajesh, Y., Dey, K. K., Pal, I., Parekh, A., et al. (2017). Gold nanorod embedded reduction responsive block copolymer micelle-triggered drug delivery combined with photothermal ablation for targeted cancer therapy. Biochimica Biophysica Acta - General Subj. 1861, 3039–3052. doi:10.1016/j.bbagen.2016.10.004
Peng, H., Huang, X., Oppermann, A., Melle, A., Weger, L., Karperien, M., et al. (2016). A facile approach for thermal and reduction dual-responsive prodrug nanogels for intracellular doxorubicin delivery. J. Mat. Chem. B 4, 7572–7583. doi:10.1039/c6tb01285j
Peng, J., Qi, T., Liao, J., Chu, B., Yang, Q., Li, W., et al. (2013). Controlled release of cisplatin from pH-thermal dual responsive nanogels. Biomaterials 34, 8726–8740. doi:10.1016/j.biomaterials.2013.07.092
Petros, R. A., and Desimone, J. M. (2010). Strategies in the design of nanoparticles for therapeutic applications. Nat. Rev. Drug Discov. 9, 615–627. doi:10.1038/nrd2591
Pramanick, S., Kim, J., Kim, J., Saravanakumar, G., Park, D., and Kim, W. J. (2017). Synthesis and characterization of nitric oxide-releasing platinum (IV) prodrug and polymeric micelle triggered by light. Bioconjug. Chem. 29, 885–897. doi:10.1021/acs.bioconjchem.7b00749
Priya James, H., John, R., Alex, A., and Anoop, K. R. (2014). Smart polymers for the controlled delivery of drugs – A concise overview. Acta Pharm. Sin. B 4, 120–127. doi:10.1016/j.apsb.2014.02.005
Pruettiphap, M., Rempel, G. L., Pan, Q., and Kiatkamjornwong, S. (2017). Morphology and drug release behavior of N-isopropylacrylamide/acrylic acid copolymer as stimuli-responsive nanogels. Iran. Polym. J. 26, 957–969. doi:10.1007/s13726-017-0571-8
Qi, X., Yu, D., Jia, B., Jin, C., Liu, X., Zhao, X., et al. (2016). Targeting CD133+ laryngeal carcinoma cells with chemotherapeutic drugs and siRNA against ABCG2 mediated by thermo/pH-sensitive mesoporous silica nanoparticles. Tumor Biol. 37, 2209–2217. doi:10.1007/s13277-015-4007-9
Qin, C., Fei, J., Cai, P., Zhao, J., and Li, J. (2016). Biomimetic membrane-conjugated graphene nanoarchitecture for light-manipulating combined cancer treatment in vitro. J. Colloid Interface Sci. 482, 121–130. doi:10.1016/j.jcis.2016.07.031
Qin, G., Li, Z., Xia, R., Li, F., O’neill, B. E., Goodwin, J. T., et al. (2011). Partially polymerized liposomes: Stable against leakage yet capable of instantaneous release for remote controlled drug delivery. Nanotechnology 22, 155605. doi:10.1088/0957-4484/22/15/155605
Qiu, H., Cui, B., Li, G., Yang, J., Peng, H., Wang, Y., et al. (2014). Novel Fe3O4@ZnO@mSiO2 Nanocarrier for targeted drug delivery and controllable release with microwave irradiation. J. Phys. Chem. C 118, 14929–14937. doi:10.1021/jp502820r
Qu, J., Wang, Q.-Y., Chen, K.-L., Luo, J.-B., Zhou, Q.-H., and Lin, J. (2018). Reduction/temperature/pH multi-stimuli responsive core cross-linked polypeptide hybrid micelles for triggered and intracellular drug release. Colloids Surfaces B Biointerfaces 170, 373–381. doi:10.1016/j.colsurfb.2018.06.015
Qu, Y., Chu, B., Shi, K., Peng, J., and Qian, Z. (2017). Recent progress in functional micellar carriers with intrinsic therapeutic activities for anticancer drug delivery. J. Biomed. Nanotechnol. 13, 1598–1618. doi:10.1166/jbn.2017.2475
Quan, S., Wang, Y., Zhou, A., Kumar, P., and Narain, R. (2015). Galactose-based thermosensitive nanogels for targeted drug delivery of iodoazomycin arabinofuranoside (IAZA) for theranostic management of hypoxic hepatocellular carcinoma. Biomacromolecules 16, 1978–1986. doi:10.1021/acs.biomac.5b00576
Rahoui, N., Jiang, B., Hegazy, M., Taloub, N., Wang, Y., Yu, M., et al. (2018). Gold modified polydopamine coated mesoporous silica nano-structures for synergetic chemo-photothermal effect. Colloids Surfaces B Biointerfaces 171, 176–185. doi:10.1016/j.colsurfb.2018.07.015
Rapoport, N. (2004). Combined cancer therapy by micellar-encapsulated drug and ultrasound. Int. J. Pharm. 277, 155–162. doi:10.1016/j.ijpharm.2003.09.048
Ringsdorf, H., Venzmer, J., and Winnik, F. M. (1991). Interaction of hydrophobically-modified poly-N-isopropylacrylamides with model membranes—Or playing a molecular accordion. Angew. Chem. Int. Ed. Engl. 30, 315–318. doi:10.1002/anie.199103151
Ruan, Z., Miao, W., Yuan, P., Le, L., Jiao, L., Hao, E., et al. (2018). High singlet oxygen yield photosensitizer based polypeptide nanoparticles for low-power near-infrared light imaging-guided photodynamic therapy. Bioconjug. Chem. 29, 3441–3451. doi:10.1021/acs.bioconjchem.8b00576
Sahle, F. F., Gulfam, M., and Lowe, T. L. (2018). Design strategies for physical-stimuli-responsive programmable nanotherapeutics. Drug Discov. Today 23, 992–1006. doi:10.1016/j.drudis.2018.04.003
Sahu, S., Sinha, N., Bhutia, S. K., Majhi, M., and Mohapatra, S. (2014). Luminescent magnetic hollow mesoporous silica nanotheranostics for camptothecin delivery and multimodal imaging. J. Mat. Chem. B 2, 3799–3808. doi:10.1039/c3tb21669a
Saito, T., Wada, H., Yamasaki, M., Miyata, H., Nishikawa, H., Sato, E., et al. (2014). High expression of MAGE-A4 and MHC class I antigens in tumor cells and induction of MAGE-A4 immune responses are prognostic markers of CHP-MAGE-A4 cancer vaccine. Vaccine 32, 5901–5907. doi:10.1016/j.vaccine.2014.09.002
Salgarella, A. R., Zahoranová, A., Šrámková, P., Majerčíková, M., Pavlova, E., Luxenhofer, R., et al. (2018). Investigation of drug release modulation from poly(2-oxazoline) micelles through ultrasound. Sci. Rep. 8, 9893. doi:10.1038/s41598-018-28140-3
Salinas, Y., Castilla, A. M., and Resmini, M. (2018). An L-proline based thermoresponsive and pH-switchable nanogel as a drug delivery vehicle. Polym. Chem. 9, 2271–2280. doi:10.1039/c8py00308d
Saracoglu, P., and Ozmen, M. M. (2021). Starch based nanogels: From synthesis to miscellaneous applications. Starch‐. Starke 73, 2100011. doi:10.1002/star.202100011
Sardon, H., Tan, J. P. K., Chan, J. M. W., Mantione, D., Mecerreyes, D., Hedrick, J. L., et al. (2015). Thermoresponsive random poly(ether urethanes) with tailorable LCSTs for anticancer drug delivery. Macromol. Rapid Commun. 36, 1761–1767. doi:10.1002/marc.201500247
Sasaki, Y., and Akiyoshi, K. (2010). Nanogel engineering for new nanobiomaterials: From chaperoning engineering to biomedical applications. Chem. Rec. 10, 366–376. doi:10.1002/tcr.201000008
Sayadnia, S., Arkan, E., Jahanban‐Esfahlan, R., Sayadnia, S., and Jaymand, M. J. P. F. a. T. (2021). Tragacanth gum‐based pH‐responsive magnetic hydrogels for “smart” chemo/hyperthermia therapy of solid tumors. Polym. Adv. Technol. 32, 262–271. doi:10.1002/pat.5082
Schmaljohann, D. (2006a). Thermo- and pH-responsive polymers in drug delivery. Adv. Drug Deliv. Rev. 58, 1655–1670. doi:10.1016/j.addr.2006.09.020
Schmaljohann, D. (2006b). Thermo-and pH-responsive polymers in drug delivery. Adv. drug Deliv. Rev. 58, 1655–1670. doi:10.1016/j.addr.2006.09.020
Schomburg, C., Wark, M., Rohlfing, Y., Schulz-Ekloff, G., and Wöhrle, D. (2001). Photochromism of spiropyran in molecular sieve voids: Effects of host–guest interaction on isomer status, switching stability and reversibility. J. Mat. Chem. 11, 2014–2021. doi:10.1039/b101516h
Shah, S., Rangaraj, N., Laxmikeshav, K., and Sampathi, S. (2020). Nanogels as drug carriers–Introduction, chemical aspects, release mechanisms and potential applications. Int. J. Pharm. 581, 119268. doi:10.1016/j.ijpharm.2020.119268
Shao, L., Zhang, R., Lu, J., Zhao, C., Deng, X., and Wu, Y. (2017). Mesoporous silica coated polydopamine functionalized reduced graphene oxide for synergistic targeted chemo-photothermal therapy. ACS Appl. Mat. Interfaces 9, 1226–1236. doi:10.1021/acsami.6b11209
Sharifabad, M. E., Mercer, T., and Sen, T. (2016). Drug-loaded liposome-capped mesoporous core-shell magnetic nanoparticles for cellular toxicity study. Nanomedicine 11, 2757–2767. doi:10.2217/nnm-2016-0248
Sharma, G., Kamboj, S., Thakur, K., Negi, P., Raza, K., and Katare, O. P. (2017). Delivery of thermoresponsive-tailored mixed micellar nanogel of lidocaine and prilocaine with improved dermatokinetic profile and therapeutic efficacy in topical anaesthesia. AAPS PharmSciTech 18, 790–802. doi:10.1208/s12249-016-0561-8
Shen, C., Shen, B., Shen, G., Li, J., Zhang, F. C., Xu, P., et al. (2016). Therapeutic effects of nanogel containing triterpenoids isolated from Ganoderma lucidum (GLT) using therapeutic ultrasound (TUS) for frostbite in rats. Drug Deliv. 23, 2643–2650. doi:10.3109/10717544.2015.1044051
Shen, C., Wang, X., Zheng, Z., Gao, C., Chen, X., Zhao, S., et al. (2019). Doxorubicin and indocyanine green loaded superparamagnetic iron oxide nanoparticles with PEGylated phospholipid coating for magnetic resonance with fluorescence imaging and chemotherapy of glioma. Int. J. Nanomedicine 14, 101–117. doi:10.2147/ijn.s173954
Shen, J.-M., Gao, F.-Y., Yin, T., Zhang, H.-X., Ma, M., Yang, Y.-J., et al. (2013). cRGD-functionalized polymeric magnetic nanoparticles as a dual-drug delivery system for safe targeted cancer therapy. Pharmacol. Res. 70, 102–115. doi:10.1016/j.phrs.2013.01.009
Shen, N., Lei, B., Wang, Y., Xu, S., and Liu, H. (2018). Redox/ultrasound dual stimuli-responsive nanogel for precisely controllable drug release. New J. Chem. 42, 9472–9481. doi:10.1039/c8nj00392k
Shi, Y., Van Steenbergen, M. J., Teunissen, E. A., Novo, L., Gradmann, S., Baldus, M., et al. (2013). Π-Π Stacking increases the stability and loading capacity of thermosensitive polymeric micelles for chemotherapeutic drugs. Biomacromolecules 14, 1826–1837. doi:10.1021/bm400234c
Shin, B., Kim, J., Vales, T. P., Yang, S. K., Kim, J. K., Sohn, H., et al. (2018). Thermoresponsive drug controlled release from chitosan-based hydrogel embedded with poly(N-isopropylacrylamide) nanogels. J. Polym. Sci. Part A Polym. Chem. 56, 1907–1914. doi:10.1002/pola.29073
Shin, Y., Husni, P., Kang, K., Lee, D., Lee, S., Lee, E. S., et al. (2021). Recent advances in pH-or/and photo-responsive nanovehicles. Pharmaceutics 13, 725. doi:10.3390/pharmaceutics13050725
Shubayev, V. I., Pisanic, T. R., and Jin, S. (2009). Magnetic nanoparticles for theragnostics. Adv. Drug Deliv. Rev. 61, 467–477. doi:10.1016/j.addr.2009.03.007
Sijumon Kunjachan, J. J., Mertens, M. E., Storm, G., Kiessling, F., Jayapaul, J., and Lammers, T. (2012). Theranostic systems and strategies for monitoring nanomedicine-mediated drug targeting. Curr. Pharm. Biotechnol. 13, 609–622. doi:10.2174/138920112799436302
Singh, A., Tran, T.-H., and Amiji, M. M. (2016a). “Redox-responsive nano-delivery systems for cancer therapy,” in Intracellular delivery III (Berlin, Germany: Springer), 255–269.
Singh, R. K., Patel, K. D., Mahapatra, C., Kang, M. S., and Kim, H. W. (2016b). C-dot generated bioactive organosilica nanospheres in theranostics: Multicolor luminescent and photothermal properties combined with drug delivery capacity. ACS Appl. Mat. Interfaces 8, 24433–24444. doi:10.1021/acsami.6b07494
Slaughter, G., and Sunday, J. (2014). Fabrication of enzymatic glucose hydrogel biosensor based on hydrothermally grown ZnO nanoclusters. IEEE Sens. J. 14, 1573–1576. doi:10.1109/jsen.2014.2298359
Soleimani, A., Martínez, F., Economopoulos, V., Foster, P. J., Scholl, T. J., and Gillies, E. R. (2013). Polymer cross-linking: A nanogel approach to enhancing the relaxivity of MRI contrast agents. J. Mat. Chem. B 1, 1027–1034. doi:10.1039/c2tb00352j
Song, X., Wen, Y., Zhu, J. L., Zhao, F., Zhang, Z. X., and Li, J. (2016). Thermoresponsive delivery of paclitaxel by β-cyclodextrin-based poly(N-isopropylacrylamide) star polymer via inclusion complexation. Biomacromolecules 17, 3957–3963. doi:10.1021/acs.biomac.6b01344
Song, Y.-Y., Li, C., Yang, X.-Q., An, J., Cheng, K., Xuan, Y., et al. (2018a). Graphene oxide coating core–shell silver sulfide@ mesoporous silica for active targeted dual-mode imaging and chemo-photothermal synergistic therapy against tumors. J. Mat. Chem. B 6, 4808–4820. doi:10.1039/c8tb00940f
Song, Z., Liu, Y., Shi, J., Ma, T., Zhang, Z., Ma, H., et al. (2018b). Hydroxyapatite/mesoporous silica coated gold nanorods with improved degradability as a multi-responsive drug delivery platform. Mater. Sci. Eng. C 83, 90–98. doi:10.1016/j.msec.2017.11.012
Soni, K. S., Desale, S. S., and Bronich, T. K. (2016a). Nanogels: An overview of properties, biomedical applications and obstacles to clinical translation. J. Control. Release 240, 109–126. doi:10.1016/j.jconrel.2015.11.009
Soni, K. S., Desale, S. S., and Bronich, T. K. (2016b). Nanogels: An overview of properties, biomedical applications and obstacles to clinical translation. J. Control. Release 240, 109–126. doi:10.1016/j.jconrel.2015.11.009
Sosnik, A. (2013). T emperature-and pH-sensitive polymeric micelles for drug encapsulation, release and. Smart Mater. drug Deliv. 1, 115.
Su, Y. Y., Teng, Z., Yao, H., Wang, S. J., Tian, Y., Zhang, Y. L., et al. (2016). A multifunctional PB@mSiO2-PEG/DOX nanoplatform for combined photothermal-chemotherapy of tumor. ACS Appl. Mat. Interfaces 8, 17038–17046. doi:10.1021/acsami.6b01147
Sudhakar, K., Madhusudana Rao, K., Subha, M. C. S., Chowdoji Rao, K., and Rotimi Sadiku, E. (2015). Development of dual responsive 5-fluorouracil loaded poly(N-vinylcaprolactam) based nanogels for targeted drug delivery applications. Polym. Sci. Ser. B 57, 638–644. doi:10.1134/s1560090415060160
Suksiriworapong, J., Rungvimolsin, T., Atitaya, A., Junyaprasert, V. B., and Chantasart, D. (2014). Development and characterization of lyophilized diazepam-loaded polymeric micelles. Aaps PharmScitech 15, 52–64. doi:10.1208/s12249-013-0032-4
Sun, Q., You, Q., Pang, X., Tan, X., Wang, J., Liu, L., et al. (2017). A photoresponsive and rod-shape nanocarrier: Single wavelength of light triggered photothermal and photodynamic therapy based on AuNRs-capped & Ce6-doped mesoporous silica nanorods. Biomaterials 122, 188–200. doi:10.1016/j.biomaterials.2017.01.021
Sun, Q., You, Q., Wang, J., Liu, L., Wang, Y., Song, Y., et al. (2018a). Theranostic nanoplatform: Triple-modal imaging-guided synergistic cancer therapy based on liposome-conjugated mesoporous silica nanoparticles. ACS Appl. Mat. Interfaces 10, 1963–1975. doi:10.1021/acsami.7b13651
Sun, W., Wang, Y., Zhang, W., Ying, H., Wang, P. J. C., and Biointerfaces, S. B. (2018b). Novel surfactant peptide for removal of biofilms. Colloids Surfaces B Biointerfaces 172, 180–186. doi:10.1016/j.colsurfb.2018.08.029
Sun, W., Wen, Y., Thiramanas, R., Chen, M., Han, J., Gong, N., et al. (2018c). Red-light-controlled release of drug–Ru complex conjugates from metallopolymer micelles for phototherapy in hypoxic tumor environments. Adv. Funct. Mat. 28, 1804227. doi:10.1002/adfm.201804227
Ta, T., Bartolak-Suki, E., Park, E.-J., Karrobi, K., Mcdannold, N. J., and Porter, T. M. (2014). Localized delivery of doxorubicin in vivo from polymer-modified thermosensitive liposomes with MR-guided focused ultrasound-mediated heating. J. Control. Release 194, 71–81. doi:10.1016/j.jconrel.2014.08.013
Ta, T., Convertine, A. J., Reyes, C. R., Stayton, P. S., and Porter, T. M. (2010). Thermosensitive liposomes modified with poly(N-isopropylacrylamide-co-propylacrylic acid) copolymers for triggered release of doxorubicin. Biomacromolecules 11, 1915–1920. doi:10.1021/bm1004993
Ta, T., and Porter, T. M. (2013). Thermosensitive liposomes for localized delivery and triggered release of chemotherapy. J. Control. release 169, 112–125. doi:10.1016/j.jconrel.2013.03.036
Tang, H., Yao, L., Yang, J., Li, W., Teng, Z., and Xu, C. (2016). Near-infrared-light-induced fast drug release platform: Mesoporous silica-coated gold nanoframes for thermochemotherapy. Part. Part. Syst. Charact. 33, 316–322. doi:10.1002/ppsc.201600062
Tang, W., Fan, W., Wang, Z., Zhang, W., Zhou, S., Liu, Y., et al. (2018). Acidity/reducibility dual-responsive hollow mesoporous organosilica nanoplatforms for tumor-specific self-assembly and synergistic therapy. ACS Nano 12, 12269–12283. doi:10.1021/acsnano.8b06058
Taqanaki, E. R., Heidari, R., Monfared, M., Tayebi, L., Azadi, A., and Farjadian, F. (2019). EDTA-modified mesoporous silica as supra adsorbent of copper ions with novel approach as an antidote agent in copper toxicity. Int. J. Nanomedicine 14, 7781–7792. doi:10.2147/ijn.s218760
Thébault, C. J., Ramniceanu, G., Boumati, S., Michel, A., Seguin, J., Larrat, B., et al. (2020). Theranostic MRI liposomes for magnetic targeting and ultrasound triggered release of the antivascular CA4P. J. Control. Release 322, 137–148. doi:10.1016/j.jconrel.2020.03.003
Timor, R., Weitman, H., Waiskopf, N., Banin, U., and Ehrenberg, B. (2015). PEG-phospholipids coated quantum rods as amplifiers of the photosensitization process by FRET. ACS Appl. Mat. Interfaces 7, 21107–21114. doi:10.1021/acsami.5b04318
Tong, R., Xia, H., and Lu, X. (2013). Fast release behavior of block copolymer micelles under high intensity focused ultrasound/redox combined stimulus. J. Mat. Chem. B 1, 886–894. doi:10.1039/c2tb00222a
Topete, A., Barbosa, S., and Taboada, P. (2015). Intelligent micellar polymeric nanocarriers for therapeutics and diagnosis. J. Appl. Polym. Sci. 132. doi:10.1002/app.42650
Town, A. R., Taylor, J., Dawson, K., Niezabitowska, E., Elbaz, N. M., Corker, A., et al. (2019). Tuning HIV drug release from a nanogel-based in situ forming implant by changing nanogel size. J. Mat. Chem. B 7, 373–383. doi:10.1039/c8tb01597j
Toyoda, M., Hama, S., Ikeda, Y., Nagasaki, Y., and Kogure, K. (2015). Anti-cancer vaccination by transdermal delivery of antigen peptide-loaded nanogels via iontophoresis. Int. J. Pharm. 483, 110–114. doi:10.1016/j.ijpharm.2015.02.024
Tran, A. V., Shim, K., Vo Thi, T. T., Kook, J. K., An, S. S. A., and Lee, S. W. (2018). Targeted and controlled drug delivery by multifunctional mesoporous silica nanoparticles with internal fluorescent conjugates and external polydopamine and graphene oxide layers. Acta Biomater. 74, 397–413. doi:10.1016/j.actbio.2018.05.022
Tu, Y. L., Wang, C. C., and Chen, C. Y. (2018). Preparation of shell crosslinked nanoencapsulate for drug carriers by using poly(N-isopropyl acrylamide)-co-poly(L-lysine) grafted copolymer. J. Polym. Res. 25, 134. doi:10.1007/s10965-018-1527-1
Upadhyay, N., Tilekar, K., Safuan, S., Kumar, A. P., Stalin, J., Ruegg, C., et al. (2021). Recent anti‐angiogenic drug discovery efforts to combat cancer. ChemistrySelect 6, 5689–5700. doi:10.1002/slct.202101792
Uz, M., Kalaga, M., Pothuraju, R., Ju, J., Junker, W. M., Batra, S. K., et al. (2019). Dual delivery nanoscale device for miR-345 and gemcitabine co-delivery to treat pancreatic cancer. J. Control. Release 294, 237–246. doi:10.1016/j.jconrel.2018.12.031
Verma, N. K., Purohit, M. P., Equbal, D., Dhiman, N., Singh, A., Kar, A. K., et al. (2016). Targeted smart pH and thermoresponsive N, O-carboxymethyl chitosan conjugated nanogels for enhanced therapeutic efficacy of doxorubicin in MCF-7 breast cancer cells. Bioconjug. Chem. 27, 2605–2619. doi:10.1021/acs.bioconjchem.6b00366
Vilsinski, B. H., Witt, M. A., Barbosa, P. M., Montanha, M. C., Nunes, C. S., Bellettini, I. C., et al. (2018). Formulation of chloroaluminum phthalocyanine incorporated into PS-b-PAA diblock copolymer nanomicelles. J. Mol. Liq. 271, 949–958. doi:10.1016/j.molliq.2018.09.034
Vivero-Escoto, J. L., Slowing, I. I., Wu, C.-W., and Lin, V. S.-Y. (2009). Photoinduced intracellular controlled release drug delivery in human cells by gold-capped mesoporous silica nanosphere. J. Am. Chem. Soc. 131, 3462–3463. doi:10.1021/ja900025f
Wan, X., Liu, T., and Liu, S. (2011). Synthesis of amphiphilic tadpole-shaped linear-cyclic diblock copolymers via ring-opening polymerization directly initiating from cyclic precursors and their application as drug nanocarriers. Biomacromolecules 12, 1146–1154. doi:10.1021/bm101463d
Wang, B., Chen, K., Yang, R., Yang, F., and Liu, J. (2014). Stimulus-responsive polymeric micelles for the light-triggered release of drugs. Carbohydr. Polym. 103, 510–519. doi:10.1016/j.carbpol.2013.12.062
Wang, D., Wei, W., Singh, A., He, G. S., Kannan, R., Tan, L. S., et al. (2017a). Nonlinear photoacoustic imaging by in situ multiphoton upconversion and energy transfer. ACS Photonics 4, 2699–2705. doi:10.1021/acsphotonics.7b00399
Wang, D., and Wu, S. (2016a). Red-light-responsive supramolecular valves for photocontrolled drug release from mesoporous nanoparticles. Langmuir 32, 632–636. doi:10.1021/acs.langmuir.5b04399
Wang, D., and Wu, S. (2016b). Red-light-responsive supramolecular valves for photocontrolled drug release from mesoporous nanoparticles. Langmuir 32, 632–636. doi:10.1021/acs.langmuir.5b04399
Wang, G., Dong, J., Yuan, T., Zhang, J., Wang, L., and Wang, H. (2016a). Visible light and pH responsive polymer-coated mesoporous silica nanohybrids for controlled release. Macromol. Biosci. 16, 990–994. doi:10.1002/mabi.201600008
Wang, G., Nie, Q., Zang, C., Zhang, B., Zhu, Q., Luo, G., et al. (2016b). Self-assembled thermoresponsive nanogels prepared by reverse micelle → positive micelle method for ophthalmic delivery of muscone, a poorly water-soluble drug. J. Pharm. Sci. 105, 2752–2759. doi:10.1016/j.xphs.2016.02.014
Wang, H., Han, R. L., Yang, L. M., Shi, J. H., Liu, Z. J., Hu, Y., et al. (2016c). Design and synthesis of core-shell-shell upconversion nanoparticles for NIR-induced drug release, photodynamic therapy, and cell imaging. ACS Appl. Mat. Interfaces 8, 4416–4423. doi:10.1021/acsami.5b11197
Wang, J., Han, J., Zhu, C., Han, N., Xi, J., Fan, L., et al. (2018a). Gold nanorods/polypyrrole/m-SiO2 core/shell hybrids as drug nanocarriers for efficient chemo-photothermal therapy. Langmuir 34, 14661–14669. doi:10.1021/acs.langmuir.8b02667
Wang, J., Jiao, Y., and Shao, Y. (2018b). Mesoporous silica nanoparticles for dual-mode chemo-sonodynamic therapy by low-energy ultrasound. Materials 11, 2041. doi:10.3390/ma11102041
Wang, J., Xu, M., Wang, K., and Chen, Z. (2019a). Stable mesoporous silica nanoparticles incorporated with MoS2 and AIE for targeted fluorescence imaging and photothermal therapy of cancer cells. Colloids Surfaces B Biointerfaces 174, 324–332. doi:10.1016/j.colsurfb.2018.11.030
Wang, M., Abbineni, G., Clevenger, A., Mao, C., and Xu, S. (2011). Upconversion nanoparticles: Synthesis, surface modification and biological applications. Nanomedicine Nanotechnol. Biol. Med. 7, 710–729. doi:10.1016/j.nano.2011.02.013
Wang, Q., Li, J. M., Yu, H., Deng, K., Zhou, W., Wang, C. X., et al. (2018c). Fluorinated polymeric micelles to overcome hypoxia and enhance photodynamic cancer therapy. Biomater. Sci. 6, 3096–3107. doi:10.1039/c8bm00852c
Wang, S., Mei, X. G., Goldberg, S. N., Ahmed, M., Lee, J. C., Gong, W., et al. (2016d). Does thermosensitive liposomal vinorelbine improve end-point survival after percutaneous radiofrequency ablation of liver tumors in a mouse model? Radiology 279, 762–772. doi:10.1148/radiol.2015150787
Wang, T., Wang, D., Yu, H., Wang, M., Liu, J., Feng, B., et al. (2016e). Intracellularly acid-switchable multifunctional micelles for combinational photo/chemotherapy of the drug-resistant tumor. ACS Nano 10, 3496–3508. doi:10.1021/acsnano.5b07706
Wang, Y., Wang, J., Xu, H., Ge, L., and Zhu, J. (2015). Investigation of dual-sensitive nanogels based on chitosan and N-isopropylacrylamide and its intelligent drug delivery of 10-hydroxycamptothecine. Drug Deliv. 22, 803–813. doi:10.3109/10717544.2014.883219
Wang, Y., Zheng, S., Chang, H., Tsai, H., and Liang, M. (2017b). Microwave-assisted synthesis of thermo-and pH-responsive antitumor drug carrier through reversible addition-fragmentation chain transfer polymerization. Express Polym. Lett. 11, 293–307. doi:10.3144/expresspolymlett.2017.29
Wang, Z., Bai, H., Lu, C., Hou, C., Qiu, Y., Zhang, P., et al. (2019b). Light controllable chitosan micelles with ROS generation and essential oil release for the treatment of bacterial biofilm. Carbohydr. Polym. 205, 533–539. doi:10.1016/j.carbpol.2018.10.095
Wei, Q., Chen, Y., Ma, X., Ji, J., Qiao, Y., Zhou, B., et al. (2018a). High-efficient clearable nanoparticles for multi-modal imaging and image-guided cancer therapy. Adv. Funct. Mat. 28, 1704634. doi:10.1002/adfm.201704634
Wei, X., Liu, L., Guo, X., Wang, Y., Zhao, J., and Zhou, S. (2018b). Light-activated ROS-responsive nanoplatform codelivering apatinib and doxorubicin for enhanced chemo-photodynamic therapy of multidrug-resistant tumors. ACS Appl. Mat. Interfaces 10, 17672–17684. doi:10.1021/acsami.8b04163
Weissleder, R. (2001). A clearer vision for in vivo imaging. Nat. Biotechnol. 19, 316–317. doi:10.1038/86684
Wen, J., Yang, K., Liu, F., Li, H., Xu, Y., and Sun, S. (2017). Diverse gatekeepers for mesoporous silica nanoparticle based drug delivery systems. Chem. Soc. Rev. 46, 6024–6045. doi:10.1039/c7cs00219j
Wirnsberger, G., Scott, B., Chmelka, B., and Stucky, G. (2000). Fast response photochromic mesostructures. Adv. Mat. 12, 1450–1454. doi:10.1002/1521-4095(200010)12:19<1450::aid-adma1450>3.0.co;2-4
Witting, M., Molina, M., Obst, K., Plank, R., Eckl, K. M., Hennies, H. C., et al. (2015). Thermosensitive dendritic polyglycerol-based nanogels for cutaneous delivery of biomacromolecules. Nanomedicine Nanotechnol. Biol. Med. 11, 1179–1187. doi:10.1016/j.nano.2015.02.017
Wong, X. Y., Sena-Torralba, A., Álvarez-Diduk, R., Muthoosamy, K., and Merkoçi, A. (2020). Nanomaterials for nanotheranostics: Tuning their properties according to disease needs. ACS Nano 14, 2585–2627. doi:10.1021/acsnano.9b08133
Wu, C., Chen, X., and He, Z. (2018a). Polymer/silica hybrid hollow nanoparticles with channels and thermo-responsive gatekeepers for drug storage and release. Colloid Polym. Sci. 296, 1961–1969. doi:10.1007/s00396-018-4397-5
Wu, C., Yu, C., and Chu, M. (2011). A gold nanoshell with a silica inner shell synthesized using liposome templates for doxorubicin loading and near-infrared photothermal therapy. Int. J. Nanomedicine 6, 807–813. doi:10.2147/ijn.s16701
Wu, F., Sun, B., Chu, X., Zhang, Q., She, Z., Song, S., et al. (2019). Hyaluronic acid-modified porous carbon-coated Fe3O4 nanoparticles for magnetic resonance imaging-guided photothermal/chemotherapy of tumors. Langmuir 35, 13135–13144. doi:10.1021/acs.langmuir.9b02300
Wu, M., Lin, X., Tan, X., Li, J., Wei, Z., Zhang, D., et al. (2018b). Photoresponsive nanovehicle for two independent wavelength light-triggered sequential release of P-gp shRNA and doxorubicin to optimize and enhance synergistic therapy of multidrug-resistant cancer. ACS Appl. Mat. Interfaces 10, 19416–19427. doi:10.1021/acsami.8b03823
Wu, P., Zhou, D., Huang, Y., and Li, J. (2018c). Light-stimulus dual-drug responsive nanoparticles for photoactivated therapy using mesoporous silica nanospheres. Chem. Res. Chin. Univ. 34, 676–683. doi:10.1007/s40242-018-8077-2
Wu, W. C., Huang, C. M., and Liao, P. W. (2014). Dual-sensitive and folate-conjugated mixed polymeric micelles for controlled and targeted drug delivery. React. Funct. Polym. 81, 82–90. doi:10.1016/j.reactfunctpolym.2014.05.003
Xi, L., Li, C., Wang, Y., Gong, Y., Su, F., and Li, S. (2020). Novel thermosensitive polymer-modified liposomes as nano-carrier of hydrophobic antitumor drugs. J. Pharm. Sci. 109, 2544–2552. doi:10.1016/j.xphs.2020.05.006
Xiao, Y., and Du, J. J. J. O. M. C. B. (2020). Superparamagnetic nanoparticles for biomedical applications. J. Mater. Chem. B 8, 354–367.
Xie, J., Lee, S., and Chen, X. (2010). Nanoparticle-based theranostic agents. Adv. drug Deliv. Rev. 62, 1064–1079. doi:10.1016/j.addr.2010.07.009
Xiong, W., Wang, W., Wang, Y., Zhao, Y., Chen, H., Xu, H., et al. (2011). Dual temperature/pH-sensitive drug delivery of poly(N-isopropylacrylamide-co-acrylic acid) nanogels conjugated with doxorubicin for potential application in tumor hyperthermia therapy. Colloids Surfaces B Biointerfaces 84, 447–453. doi:10.1016/j.colsurfb.2011.01.040
Xu, J., Han, W., Cheng, Z., Yang, P., Bi, H., Yang, D., et al. (2018a). Bioresponsive and near infrared photon co-enhanced cancer theranostic based on upconversion nanocapsules. Chem. Sci. 9, 3233–3247. doi:10.1039/c7sc05414a
Xu, J., He, F., Cheng, Z., Lv, R., Dai, Y., Gulzar, A., et al. (2017). Yolk-Structured upconversion nanoparticles with biodegradable silica shell for FRET sensing of drug release and imaging-guided chemotherapy. Chem. Mat. 29, 7615–7628. doi:10.1021/acs.chemmater.7b03461
Xu, J. W., Ge, X., Lv, L. H., Xu, F., and Luo, Y. L. (2018b). Dual-stimuli-responsive paclitaxel delivery nanosystems from chemically conjugate self-assemblies for carcinoma treatment. Macromol. Rapid Commun. 39, 1800628. doi:10.1002/marc.201800628
Xu, W., Ling, P., and Zhang, T. (2013). Polymeric micelles, a promising drug delivery system to enhance bioavailability of poorly water-soluble drugs. J. Drug Deliv. 2013, 1–15. doi:10.1155/2013/340315
Yan, T., He, J., Liu, R., Liu, Z., and Cheng, J. (2020). Chitosan capped pH-responsive hollow mesoporous silica nanoparticles for targeted chemo-photo combination therapy. Carbohydr. Polym. 231, 115706. doi:10.1016/j.carbpol.2019.115706
Yang, D., Yang, G., Gai, S., He, F., Lv, R., Dai, Y., et al. (2016a). Imaging-guided and light-triggered chemo-/photodynamic/photothermal therapy based on Gd (III) chelated mesoporous silica hybrid spheres. ACS Biomater. Sci. Eng. 2, 2058–2071. doi:10.1021/acsbiomaterials.6b00462
Yang, F., Cao, Z., and Wang, G. (2015). Micellar assembly of a photo-and temperature-responsive amphiphilic block copolymer for controlled release. Polym. Chem. 6, 7995–8002. doi:10.1039/c5py01435b
Yang, G., Sun, X., Liu, J., Feng, L., and Liu, Z. (2016b). Light-responsive, singlet-oxygen-triggered on-demand drug release from photosensitizer-doped mesoporous silica nanorods for cancer combination therapy. Adv. Funct. Mat. 26, 4722–4732. doi:10.1002/adfm.201600722
Yang, L.-Y., Zhou, H., Yang, Y., Tong, Y.-N., Peng, L.-S., Zhu, B.-H., et al. (2018a). Protective effects of a nanoemulsion adjuvant vaccine (2C-Staph/NE) administered intranasally against invasive Staphylococcus aureus pneumonia. RSC Adv. 8, 9996–10008. doi:10.1039/c7ra13630g
Yang, L., Guo, C., Jia, L., Liang, X., Liu, C., and Liu, H. (2010). Dual responsive copolymer micelles for drug controlled release. J. Colloid Interface Sci. 350, 22–29. doi:10.1016/j.jcis.2010.04.023
Yang, Q., Peng, J., Chen, C., Xiao, Y., Tan, L., Xie, X., et al. (2018b). Targeting delivery of rapamycin with anti-collagen IV peptide conjugated Fe3O4@Nanogels system for vascular restenosis therapy. J. Biomed. Nanotechnol. 14, 1208–1224. doi:10.1166/jbn.2018.2588
Yang, Z., Liu, X., Xu, X., Chen, S., Li, F., Zhu, X., et al. (2016c). Temperature and pH dual-responsive polyhedral oligomeric silsesquioxane/poly [2-(dimethyl amino)-ethyl methacrylate]-bPoly (N-isopropylacrylamide) hybrid materials synthesized via RAFT polymerization and thiol-ene reaction: Potential candidates for absorption of organic dyes. Sci. Adv. Mat. 8, 1901–1907. doi:10.1166/sam.2016.3001
Yang, Z. L., Tian, W., Wang, Q., Zhao, Y., Zhang, Y. L., Tian, Y., et al. (2018c). Oxygen-evolving mesoporous organosilica coated prussian blue nanoplatform for highly efficient photodynamic therapy of tumors. Adv. Sci. (Weinh). 5, 1700847. doi:10.1002/advs.201700847
Yang, Z., Zou, H., Liu, H., Xu, W., and Zhang, L. (2019). Self-assembly and drug release control of dual-responsive copolymers based on oligo(ethylene glycol)methyl ether methacrylate and spiropyran. Iran. Polym. J. 28, 39–49. doi:10.1007/s13726-018-0677-7
Yao, C., Wang, P., Li, X., Hu, X., Hou, J., Wang, L., et al. (2016). Near-infrared-triggered azobenzene-liposome/upconversion nanoparticle hybrid vesicles for remotely controlled drug delivery to overcome cancer multidrug resistance. Adv. Mat. 28, 9341–9348. doi:10.1002/adma.201503799
Yao, X., Niu, X., Ma, K., Huang, P., Grothe, J., Kaskel, S., et al. (2017). Graphene quantum dots-capped magnetic mesoporous silica nanoparticles as a multifunctional platform for controlled drug delivery, magnetic hyperthermia, and photothermal therapy. Small 13, 1602225. doi:10.1002/smll.201602225
Yatvin, M. B., Weinstein, J. N., Dennis, W. H., and Blumenthal, R. (1978). Design of liposomes for enhanced local release of drugs by hyperthermia. Science 202, 1290–1293. doi:10.1126/science.364652
Yi, P., Wang, Y., He, P., Zhan, Y., Sun, Z., Li, Y., et al. (2017). Study on β-cyclodextrin-complexed nanogels with improved thermal response for anticancer drug delivery. Mater. Sci. Eng. C 78, 773–779. doi:10.1016/j.msec.2017.04.096
Yi, S., Zheng, J., Lv, P., Zhang, D., Zheng, X., Zhang, Y., et al. (2018). Controlled drug release from cyclodextrin-gated mesoporous silica nanoparticles based on switchable host-guest interactions. Bioconjug. Chem. 29, 2884–2891. doi:10.1021/acs.bioconjchem.8b00416
Yin, T., Wang, P., Li, J., Wang, Y., Zheng, B., Zheng, R., et al. (2014). Tumor-penetrating codelivery of siRNA and paclitaxel with ultrasound-responsive nanobubbles hetero-assembled from polymeric micelles and liposomes. Biomaterials 35, 5932–5943. doi:10.1016/j.biomaterials.2014.03.072
Yin, T., Wang, P., Li, J., Zheng, R., Zheng, B., Cheng, D., et al. (2013). Ultrasound-sensitive siRNA-loaded nanobubbles formed by hetero-assembly of polymeric micelles and liposomes and their therapeutic effect in gliomas. Biomaterials 34, 4532–4543. doi:10.1016/j.biomaterials.2013.02.067
Yu, F., Wu, H., Tang, Y., Xu, Y., Qian, X., and Zhu, W. (2018). Temperature-sensitive copolymer-coated fluorescent mesoporous silica nanoparticles as a reactive oxygen species activated drug delivery system. Int. J. Pharm. 536, 11–20. doi:10.1016/j.ijpharm.2017.11.025
Yu, J., Chu, X., and Hou, Y. (2014). Stimuli-responsive cancer therapy based on nanoparticles. Chem. Commun. 50, 11614–11630. doi:10.1039/c4cc03984j
Yuan, W., Zou, H., and Shen, J. (2016). Amphiphilic graft copolymers with ethyl cellulose backbone: Synthesis, self-assembly and tunable temperature-CO2 response. Carbohydr. Polym. 136, 216–223. doi:10.1016/j.carbpol.2015.09.052
Yuan, Y., Wang, Z., Cai, P., Liu, J., Liao, L.-D., Hong, M., et al. (2015). Conjugated polymer and drug co-encapsulated nanoparticles for chemo-and photo-thermal combination therapy with two-photon regulated fast drug release. Nanoscale 7, 3067–3076. doi:10.1039/c4nr06420h
Zabihzadeh, M., Hoseini-Ghahfarokhi, M., Bayati, V., Teimoori, A., Ramezani, Z., Assarehzadehgan, M.-A., et al. (2018). Enhancement of radio-sensitivity of colorectal cancer cells by gold nanoparticles at 18 MV energy. Nanomedicine J. 5, 120–111.
Zan, M., Li, J., Huang, M., Lin, S., Luo, D., Luo, S., et al. (2015). Near-infrared light-triggered drug release nanogels for combined photothermal-chemotherapy of cancer. Biomater. Sci. 3, 1147–1156. doi:10.1039/c5bm00048c
Zarkesh, K., Entezar-Almahdi, E., Ghasemiyeh, P., Akbarian, M., Bahmani, M., Roudaki, S., et al. (2021). Drug-based therapeutic strategies for COVID-19-infected patients and their challenges. Future Microbiol. 16, 1415–1451. doi:10.2217/fmb-2021-0116
Zeng, L., Pan, Y., Zou, R., Zhang, J., Tian, Y., Teng, Z., et al. (2016). 808 nm-excited upconversion nanoprobes with low heating effect for targeted magnetic resonance imaging and high-efficacy photodynamic therapy in HER2-overexpressed breast cancer. Biomaterials 103, 116–127. doi:10.1016/j.biomaterials.2016.06.037
Zhang, H., Gong, W., Wang, Z.-Y., Yuan, S.-J., Xie, X.-Y., Yang, Y.-F., et al. (2014a). Preparation, characterization, and pharmacodynamics of thermosensitive liposomes containing docetaxel. J. Pharm. Sci. 103, 2177–2183. doi:10.1002/jps.24019
Zhang, H. (2016). Onivyde for the therapy of multiple solid tumors. Onco. Targets. Ther. 9, 3001–3007. doi:10.2147/ott.s105587
Zhang, H., Xia, H., Wang, J., and Li, Y. (2009). High intensity focused ultrasound-responsive release behavior of PLA-b-PEG copolymer micelles. J. Control. Release 139, 31–39. doi:10.1016/j.jconrel.2009.05.037
Zhang, K. H., Liu, J. T., Ma, X., Lei, L., Li, Y., Yang, H., et al. (2018b). Temperature, pH, and reduction triple-stimuli-responsive inner-layer crosslinked micelles as nanocarriers for controlled release. J. Appl. Polym. Sci. 135, 46714. doi:10.1002/app.46714
Zhang, K., Liu, J., Guo, Y., Li, Y., Ma, X., and Lei, Z. (2018a). Synthesis of temperature, pH, light and dual-redox quintuple-stimuli-responsive shell-crosslinked polymeric nanoparticles for controlled release. Mater. Sci. Eng. C 87, 1–9. doi:10.1016/j.msec.2018.02.005
Zhang, L., Guo, R., Yang, M., Jiang, X., and Liu, B. (2007). Thermo and pH dual-responsive nanoparticles for anti-cancer drug delivery. Adv. Mat. 19, 2988–2992. doi:10.1002/adma.200601817
Zhang, L., Li, Y., Jin, Z., Yu, J. C., and Chan, K. M. (2015). An NIR-triggered and thermally responsive drug delivery platform through DNA/copper sulfide gates. Nanoscale 7, 12614–12624. doi:10.1039/c5nr02767e
Zhang, M., Li, D., Wang, G., Li, J., and Sun, H. (2014b). Highly efficient light-induced reversible micellization of amphiphilic polymer based on photochromism of naphthopyran in aqueous solution. Dyes Pigments 105, 232–237. doi:10.1016/j.dyepig.2014.02.016
Zhang, R., Wang, X., Fan, N., and Li, J. (2021). Enhanced anticancer performances of doxorubicin loaded macro-mesoporous silica nanoparticles with host-metal-guest structure. Microporous Mesoporous Mater. 310, 110589. doi:10.1016/j.micromeso.2020.110589
Zhang, X., Yang, P., Dai, Y., Ma, P., Li, X., Cheng, Z., et al. (2013). Multifunctional up-converting nanocomposites with smart polymer brushes gated mesopores for cell imaging and thermo/pH dual-responsive drug controlled release. Adv. Funct. Mat. 23, 4067–4078. doi:10.1002/adfm.201300136
Zhang, Y., Guan, Y., Ge, S., Yuan, A., Wu, J., and Hu, Y. (2017). Light-responsive CO2 bubble-generating polymeric micelles for tumor cell ablation. Medchemcomm 8, 405–407. doi:10.1039/c6md00625f
Zhang, Y., Huang, Y., and Li, S. (2014c). Polymeric micelles: Nanocarriers for cancer-targeted drug delivery. Aaps Pharmscitech 15, 862–871. doi:10.1208/s12249-014-0113-z
Zhang, Y., Wang, C. X., and Huang, S. W. (2018c). Aggregation-induced emission (AIE) polymeric micelles for imaging-guided photodynamic cancer therapy. Nanomaterials 8, 921. doi:10.3390/nano8110921
Zhao, D., Shi, X., Liu, T., Lu, X., Qiu, G., and Shea, K. J. (2016). Synthesis of surfactant-free hydroxypropyl methylcellulose nanogels for controlled release of insulin. Carbohydr. Polym. 151, 1006–1011. doi:10.1016/j.carbpol.2016.06.055
Zhao, J., He, Z., Li, B., Cheng, T., and Liu, G. (2017). AND logic-like pH-and light-dual controlled drug delivery by surface modified mesoporous silica nanoparticles. Mater. Sci. Eng. C 73, 1–7. doi:10.1016/j.msec.2016.12.056
Zhao, T., Chen, L., Li, Q., and Li, X. (2018). Near-infrared light triggered drug release from mesoporous silica nanoparticles. J. Mat. Chem. B 6, 7112–7121. doi:10.1039/c8tb01548a
Zheng, Q., He, Y., Tang, Q., Wang, Y., Zhang, N., Liu, J., et al. (2018a). An NIR-guided aggregative and self-immolative nanosystem for efficient cancer targeting and combination anticancer therapy. Mol. Pharm. 15, 4985–4994. doi:10.1021/acs.molpharmaceut.8b00599
Zheng, S., Han, J., Jin, Z., Kim, C. S., Park, S., Kim, K. P., et al. (2018b). Dual tumor-targeted multifunctional magnetic hyaluronic acid micelles for enhanced MR imaging and combined photothermal-chemotherapy. Colloids Surfaces B Biointerfaces 164, 424–435. doi:10.1016/j.colsurfb.2018.02.005
Zhou, H., Xu, H., Li, X., Lv, Y., Ma, T., Guo, S., et al. (2017). Dual targeting hyaluronic acid - RGD mesoporous silica coated gold nanorods for chemo-photothermal cancer therapy. Mater. Sci. Eng. C 81, 261–270. doi:10.1016/j.msec.2017.08.002
Zhou, Q., Zhang, L., Yang, T., and Wu, H. (2018a). Stimuli-responsive polymeric micelles for drug delivery and cancer therapy. Int. J. Nanomedicine 13, 2921–2942. doi:10.2147/ijn.s158696
Zhou, W., Yang, G., Ni, X., Diao, S., Xie, C., and Fan, Q. (2020). Recent advances in crosslinked nanogel for multimodal imaging and cancer therapy. Polymers 12, 1902. doi:10.3390/polym12091902
Zhou, Y.-F. (2011). High intensity focused ultrasound in clinical tumor ablation. World J. Clin. Oncol. 2, 8. doi:10.5306/wjco.v2.i1.8
Zhou, Z., Li, G., Wang, N., Guo, F., Guo, L., and Liu, X. (2018b). Synthesis of temperature/pH dual-sensitive supramolecular micelles from β-cyclodextrin-poly(N-isopropylacrylamide) star polymer for drug delivery. Colloids Surfaces B Biointerfaces 172, 136–142. doi:10.1016/j.colsurfb.2018.08.031
Zhu, Y., Wang, X., Chen, J., Zhang, J., Meng, F., Deng, C., et al. (2016). Bioresponsive and fluorescent hyaluronic acid-iodixanol nanogels for targeted X-ray computed tomography imaging and chemotherapy of breast tumors. J. Control. Release 244, 229–239. doi:10.1016/j.jconrel.2016.08.027
Glossary
5-FU 5-fluorouracil
ADR adriamycin
AgNPs silver nanoparticles
Alg alginate
ATRP atom transfer radical polymerization
AuNPs gold nanoparticles
Azo azobenzene
BDPI pyrrole-substituted iodinated BODIPY
BSA bovine serum albumin
C 102 Coumarin 102
CD cyclodextrin,
CdS cadmium sulfide
Ce6 chlorin e6
Chol cholesterol
cis-Pt cis-platin
ClAlPc chloroaluminum phthalocyanine
CMC critical micelle concentration
CMP camptothecin
CNTs carbon nanotubes
CPT camptothecin
CS chitosan
CT computed tomography
DDSs drug delivery systems
DFM dark-field microscopy
DOX doxorubicin
DTX docetaxel
EAPs electric active polymers
EPR enhanced permeation and retention
FA folic acid
FDA Food and Drug Administration
FI fluorescence imaging
GEM gemcitabine
GNP gold nanoparticle
GO graphene oxide
GQDs graphene QDs
HA hyaluronic acid
HMSN hallow MSN
Ibu ibuprofen
ICG indocyanine green
IONP iron oxide nanoparticles
IR780 photoresponsive NIR dye
LCST lower critical solution temperature
MMSN MNP core with MS shell
MNP magnetic nanoparticles
MRI magnetic resonance imaging
MS mesoporous silica
MSN mesoporous silica nanoparticles
MTX methotrexate
NGs nanogels
NIR near-infrared
NIRI near-infrared imaging
NPs nanoparticles
NR Nile Red
NRs nano-rattles
NRS nano-rods
Nsts nano-stars
OI optical imaging
OVA ovalbumin
Ox oxide
OXA oxaliplatin
PAA polyacrylic acid
PAAm poly acrylamide
PAI photoacoustic imaging
PAS poly aspartic acid
PB prussian blue
PCL poly(caprolactone)
PDA polydopamine
PDMAEMA poly(2-dimethylaminoethyl methacrylate)
PDT photodynamic therapy
PEG polyethyleneglycol
PEI polyethyleneimine
PEO polyethylene oxide
PHEMA poly(2-hydroxyethylmethacrylate
PHPMA poly(N-hydroxypropyl)methacrylamide
PLA poly(lactic acid)
PLGA polyglycolic acid
Plys poly(l-lysine)
PMAA poly(methacrylic acid)
PMs polymeric micelles
PNIPAAm poly(N-isopropylacrylamide)
PNVCL poly(N-vinylcaprolactam)
PpIX protoporphyrin IX
PPO polypropylene oxide
PPy polypyrrole
PS polystyrene
PTNS physically triggered nano-systems
PTT photothermal therapy
PTX paclitaxel
Py pyridine
PyF pyropheophorbide-a
QDs quantum dots
RAFT reversible addition-fragmentation chain transfer polymerization
RB Rose Bengal
rGO reduced graphene oxide
RhB rhodamine B
ROP ring-opening polymerization
ROS reactive oxygen species
SHG second harmonic generation
SN38 camptothecin analog
SNA spherical nucleic acid
SPECT single-photon emission CT
TBM tumor-bearing mice
TI thermal imaging
TNF tumor necrosis factor
UCST upper critical solution temperature
UPCNS up-conversion NPs
USI ultrasound imaging
UV ultra violet
Vis visible
ZnPc zinc phthalocyanine
Keywords: drug delivery, diagnosis, theranostic, micelles, hydrogel, mesoporous silica, magnetic nanoparticles, liposomes
Citation: Farjadian F, Ghasemi S, Akbarian M, Hoseini-Ghahfarokhi M, Moghoofei M and Doroudian M (2022) Physically stimulus-responsive nanoparticles for therapy and diagnosis. Front. Chem. 10:952675. doi: 10.3389/fchem.2022.952675
Received: 25 May 2022; Accepted: 02 August 2022;
Published: 14 September 2022.
Edited by:
Sanjay Singh, National Institute of Animal Biotechnology (NIAB), IndiaReviewed by:
Santanu Patra, Khon Kaen University, ThailandStergios Pispas, National Hellenic Research Foundation, Greece
Copyright © 2022 Farjadian, Ghasemi, Akbarian, Hoseini-Ghahfarokhi, Moghoofei and Doroudian. This is an open-access article distributed under the terms of the Creative Commons Attribution License (CC BY). The use, distribution or reproduction in other forums is permitted, provided the original author(s) and the copyright owner(s) are credited and that the original publication in this journal is cited, in accordance with accepted academic practice. No use, distribution or reproduction is permitted which does not comply with these terms.
*Correspondence: Fatemeh Farjadian, ZmFyamFkaWFuX2ZAc3Vtcy5hYy5pcg==, Soheila Ghasemi, Z2hhc2VtaXNAc2hpcmF6dS5hYy5pcg==, Mohammad Doroudian, ZG9yb3VkaWFuQGtodS5hYy5pcg==