- 1Energy Science/Energy Engineering, Department of Engineering Sciences and Mathematics, Luleå University of Technology, Luleå, Sweden
- 2Faculty of Environment and Life, Beijing University of Technology, Beijing, China
CO2 capture is essential for both mitigating CO2 emissions and purifying/conditioning gases for fuel and chemical production. To further improve the process performance with low environmental impacts, different strategies have been proposed, where developing liquid green absorbent for capturing CO2 is one of the effective options. Ionic liquids (IL)/deep eutectic solvents (DES) have recently emerged as green absorbents with unique properties, especially DESs also benefit from facile synthesis, low toxicity, and high biodegradability. To promote their development, this work summarized the recent research progress on ILs/DESs developed for CO2 capture from the aspects of those physical- and chemical-based, and COSMO-RS was combined to predict the properties that are unavailable from published articles in order to evaluate their performance based on the key properties for different IL/DES-based technologies. Finally, top 10 ILs/DESs were listed based on the corresponding criteria. The shared information will provide insight into screening and further developing IL/DES-based technologies for CO2 capture.
1 Introduction
Nowadays, the energy supply highly depends on carbonaceous fuels, especially fossil fuels, including petroleum, coal, and conventional/unconventional natural gas (Wang and Song, 2020). The combustion of these fuels in mobile energy systems, power plants, and industrial factories results in the emission of greenhouse gases (Wang and Song, 2020). CO2 is considered as the most important anthropogenic greenhouse gas, leading to ocean acidification, global warming, land desertification, and rising sea level (Sanz-Perez et al., 2016). Also, it is delineated that the concentration of CO2 in the atmosphere has significantly soared from 340 to 408 ppm in the 1980–2019 time period (Ghanbari et al., 2020). Thus, CO2 capture from flue gases has gained considerable attention among industrial and academic authorities.
On the other hand, CO2 separation is needed to produce renewable fuels to reduce CO2 emissions from the transportation sector. For instance, biomass gasification offers a possibility to produce more carbon-neutral transportation fuels, such as methanol, hydrogen, and synthetic hydrocarbons, where CO2 removal is always required (Shahbaz et al., 2021). In the production of synthetic hydrocarbons with the Fischer-Tropsch technology, the inert CO2 is removed to increase the efficiency and selectivity of C5+; in the methanol production, CO2 removal is made to obtain a favorable ratio of the gas mixture to increase the production yield; while in the hydrogen production, CO2 separation is needed to purify hydrogen. In parallel, bio-methane production and its usage as vehicle fuels provide an alternative and important pathway to mitigate CO2 emissions from the transportation sector. In general, the raw biogas generated in the anaerobic digestion contains CO2 of around 30–50 vol%. To be used as vehicle fuels, CO2 removal from the raw biogas (i.e., biogas upgrading) is needed to lower the CO2 content to 2–6 vol% (Ahmed et al., 2021). Besides, bio-carbon capture, utilization and/or storage (bio-CCUS) from the bioenergy sector is of considerable interest towards negative carbon emissions, further highlighting the importance of CO2 capture/separation.
Currently, the available technologies for CO2 separation mainly include absorption, adsorption, membrane, and cryogenic separation, and they are very costly ($50–100/ton-CO2) combined with other challenges (Mondal et al., 2012). For example, the aqueous amine-based technology (absorption) is energy-intensive, and volatility (secondary pollution), degradation, and corrosion are other deficiencies; the selectivity of the membrane is often lost in the presence of H2; adsorption is energy consuming and usually requires large-scale operations; the Rectisol process needs high power for refrigeration. Further development is needed to decrease the cost of CO2 separation with marginal or no environmental influence, where solvent-based technologies have been suggested as the most suitable candidates considering the tolerance for the impurities from the gas streams.
To develop solvent-based technology for CO2 separation, different kinds of absorbents have been proposed, where ionic liquids (ILs) have drawn significant attention because 1) they are nonvolatile, nonflammable, environmentally benign, and thermal stable; 2) their chemical and physical properties are tunable, making it possible to design a specific IL for a specific CO2 capture process; and 3) relatively high CO2 solubility and selectivity as well as relatively low energy-demand for solvent regeneration. Additionally, a new class of ILs or IL analogous, i.e., deep eutectic solvents (DESs), share similar properties to the conventional ILs together with other additional appealing properties, such as their simple preparation processes, low cost, biodegradability, and environmentally friendly materials used in synthesis (Sarmad et al., 2017a).
Owing to the unique properties of both ILs and DESs, intensive work has been conducted, and results have been reported all the time, calling for a new survey of the latest research. Also, the extensive work imposes the challenge to screen and suggest proper ILs and DESs for a specific purpose, which is related to the compositions and conditions of the streams needed for CO2 separation as well as other available resources, such as waste-heat or the availability of steam, etc. To the best of our knowledge, it is still unclear which ILs and DESs within those developed are more desirable for a specific IL/DES-based technology. Indeed, process simulation and evaluation are conducted, but just after a very brief and quantitative comparison of some properties. Especially in some works, the important properties, e.g., viscosity and/or selectivity, are not included or concerned sufficiently, making it difficult to convince the reliability of the simulation results (Hospital-Benito et al., 2021). All these issues call for a proper screen, selection, and suggestion of promising ILs and DESs for further study and development.
To screen ILs/DESs, properties are needed, which can be determined experimentally or predicted theoretically. Theoretical models have been developed to predict properties of ILs/DESs qualitatively or quantitively, and the models include Quantitative Structure Property Relationship (QSPR) method (Eike et al., 2004), regular solution theory (Kilaru et al., 2008), Molecular dynamics (Kerlé et al., 2009), Monte Carlo (Shah and Maginn, 2005), group contribution method (Kim et al., 2005; Zhang et al., 2021b; Zhou et al., 2021), Conductor-like Screening Model for Real Solvents (COSMO-RS) method (Liu et al., 2021), statistical associating fluid theory (SAFT)-based equation of state (Ji et al., 2012; Ji and Zhu, 2013; Ji et al., 2014; Shen et al., 2015; Sun et al., 2019; Alkhatib et al., 2020; Sun, 2020), etc. Based on the properties, ILs and DESs are screened with different criteria, for example, thermodynamic CO2 absorption capacity (CO2 solubility and Henry’s constant) (Liu et al., 2021) and (Henry’s constant, selectivity, relative polarity, and molar volumes) (Sumon and Henni, 2011), thermodynamic and kinetic performances for CO2 absorption (CO2 absorption capacity, viscosity, melting point, and Henry’s constant) (Farahipour et al., 2016; Zhang et al., 2021a) as well as CO2 chemical absorption properties (chemical equilibrium constants, Henry’s constants, and reaction enthalpies) (Moya et al., 2020), etc. Also, based on the thermodynamic analysis under specific operation conditions, screening has been conducted for both physical-based ILs and DESs (Zhang et al., 2016). In recent years, Zhou’s group has developed data-driven machine learning models to predict both the thermodynamic and kinetic properties of CO2 absorption with ILs/DESs (e.g., the CO2 solubility, absorbent viscosity and CO2 mass transfer property) (Song et al., 2020), providing a more comprehensive route for screening the promising ILs or DESs. However, the models are dependent on a large number of reliable experimental data, while the widely used COSMO-based models have a prior feature, resulting in the robust application value, especially when experimental data are missing. Moreover, COSMO-RS can combine with process simulation via Aspen to further evaluate the performance and conduct techno-economic analysis (García-Gutiérrez et al., 2016; De Riva et al., 2018). Therefore, COSMO-RS is a powerful tool for prior screening ILs/DESs.
In this work, the latest developed ILs and DESs were surveyed and collected, which were further combined with those have been collected in other review articles to provide a complete database. The IL/DES-based technologies were introduced, and the important properties were identified for each technology, providing criteria for IL/DES screening. Because not all the properties of ILs/DESs were provided, COSMO-RS was combined to predict the properties required in screening. Subsequently, the desirable ILs and DESs were screened, selected, and suggested for different IL/DES-based technologies.
2 Technologies for CO2 separation with ionic liquids/deep eutectic solvents
2.1 Ionic liquids/deep eutectic solvents as solvents
Over the past two decades, ILs/DESs as absorbents for CO2 capture have been widely studied (Smith et al., 2014; Zeng et al., 2017; Hansen et al., 2020). The extensive attention and research are based on their desirable properties, such as negligible vapor pressure, stable thermodynamic and chemical properties, tunable and designable structures, and good solubility for CO2. In particular, the negligible vapor pressure can simplify the CO2 absorption process.
2.1.1 Ionic liquids as solvents
Blanchard et al. first reported that supercritical CO2 could be effectively dissolved in IL [C4MIM][PF6] at 25°C and 40 MPa, but ILs were insoluble in CO2 (Blanchard et al., 2001). Since then, research on the CO2 capture by ILs as absorbents has been reported extensively. According to the difference in capture mechanisms, ILs can be treated as two classes, i.e., the physical-based absorbents and chemical-based absorbents. The former represents the conventional ILs being able to interact with CO2 physically, while the latter represents the functionalized ILs being able to interact with CO2 chemically. In conventional ILs, anions usually play a leading role in the CO2 capture, while the capture effect is relatively less affected by cations (Anthony et al., 2005; Ramdin et al., 2012; Dai et al., 2017).
Similar to other physical absorbents, the CO2 absorption capacity of these physical-based ILs is inferior to those of traditional organic amines. In order to further enhance and improve the CO2 absorption capacity, researchers developed functionalized ILs with chemical interaction sites, such as carboxyl-based ILs, amino-based ILs, and amino acid-based ILs (Zeng et al., 2017). Bates et al. (2002) first prepared the amino-functionalized IL [NH2P-BIN][BF4] to capture CO2, with an absorption capacity up to 0.50 mol/mol. Xue et al. (2011) reported the CO2 solubility in amine-functionalized IL [AemMIM][Tau] up to 0.90 mol/mol. Later, the functionalized ILs with the anions introduced by amines were proposed. For example, Gurkan et al. (2010) presented two amino acid-functionalized ILs, [P66614][Pro] and [P66614][Met], both of which exhibited CO2 absorption capacity up to 0.90 mol/mol, near a 1:1 stoichiometry, and subsequently, an array of non-amino-functionalized ILs were developed (Lin et al., 2019b). To further improve the absorption capacity, Wang et al. prepared and synthesized the pyridine-based IL [P66614][3-OMe3-2-Op] (Luo et al., 2014), demonstrating the absorption capacity of up to 1.60 mol/mol under 20°C and 0.1 MPa because of the two interaction sites presented between oxygen and nitrogen in the anion and CO2.
Since the industry is more interested in the mass absorption capacity, researchers have made great efforts to improve and enhance the mass absorption capacity of ILs for CO2 capture. For example, Zhang et al. (2013) developed a novel kind of bisamino-functionalized IL, whose mass absorption capacity of CO2 can reach 18.5%. Chen et al. (2016) prepared a functionalized IL [P4442]2 [IDA] with the highest molar CO2 absorption capacity (1.69 mol/mol), corresponding to the mass absorption capacity of 2.84 mol/kg under 40°C and 0.1 MP. However, the viscosity of [P4442]2 [IDA] can be increased by almost 15 times after the absorption of CO2.
In a real process of CO2 separation, in addition to the CO2 absorption capacity (solubility), viscosity is also a key factor to consider. This is particularly essential for the IL-based technology, as the viscosity of most developed IL is much higher than the conventional solvents (Jiang et al., 2019a). Functionalized ILs have an even higher viscosity than conventional ones, and the absorption with CO2 is, in general, accompanied by a chemical reaction that further increases the viscosity (Liu et al., 2009; Sistla and Khanna, 2015; Ma et al., 2019). Therefore, it is necessary to develop ILs with low viscosity. Recently, Zhang et al. found an effective method to reduce the viscosity of ILs by introducing ether groups into cations, and increasing the number of ether-oxygen bonds decreased the viscosity (Zeng et al., 2015). Adding molecular solvent to an IL-based hybrid is another effective method to reduce viscosity. For example, when an organic amine solvent is added to ILs, the viscosity is significantly reduced, while the CO2 absorption capacity is not significantly weakened (Zhao et al., 2011).
Furthermore, in practical CO2 capture processes, the CO2 selectivity to other gases should be carefully considered (Ma et al., 2018). This is because ILs will serve as either a physical absorbent only, or the absorbent with both chemical and physical contributions (Ma et al., 2021) that is different from the conventional chemical absorbents. For instance, in the CO2 capture from natural gas or biogas, Zeng et al. (2015) designed and synthesized ether-based functionalized ILs, and their selectivity of CO2/CH4 was improved by 50% compared with non-functionalized ILs. Additionally, the interaction between IL and CO2, in general, is weak compared with the amine-based absorbents, and the focus has been specifically concerned on the desorption enthalpy in the research.
2.1.2 Deep eutectic solvents as solvents
As a new type of “green solvents,” DESs can be regarded as IL analogous, and it has unique advantages such as lower preparation cost, biodegradability, and more eco-friendly properties when compared with ILs (Zhang et al., 2012; Smith et al., 2014). DESs have received wide attention for gas separation, especially CO2 capture (Smith et al., 2014; Hansen et al., 2020). When DESs are used as absorbents for CO2 capture, they can be divided into physical- and chemical-based absorbents, just like ILs. Most conventional DESs capture CO2 by means of physical absorption, while functionalized DESs like superbase-based DESs can capture CO2 by means of chemical absorption (Sarmad et al., 2017b). The study by Sarmad et al. (2017b) showed that DESs are promising alternatives to ILs because the solubility of CO2 in DESs is even higher than the similar IL counterparts. Recently, Huang et al. (Liu et al., 2019) found that the CO2 solubility in the DES (ChCl + urea) can be accommodated by the HBA/HBD molar ratios, following the order of 1:2 > 1:1.5 > 1:2.5, which leads to the fact that the selectivities of gas pairs for CO2/H2S and CO2/CH4 can be tuned by controlling the HBA/HBD molar ratios. Their study also explored the mechanism of CO2 capture at the molecular level, that is, it was dominated by the free volume inside DESs. However, the ChCl-based DESs have higher viscosities than the conventional ILs (Smith et al., 2014; Hansen et al., 2020).
In order to improve CO2 absorption capacity, superbase-based DESs with chemical absorption have been developed. Wang et al. (2010a) used DESs/ILs and superbase mixtures to prepare the superbase-based DES/ILs. This study clarified the role of the superbase in CO2 capture, that is, the superbase enables abstracting acidic hydrogens from the imidazolium ring of cation in ILs to enhance the affinity with CO2, increasing CO2 capture performance. Other publications have also proved that adding superbases or basic groups into the DES/IL systems can evidently improve the CO2 absorption capacity (Wang et al., 2010b; Wang et al., 2011). It should be noted that the addition of these superbases or basic groups always increases viscosity, which is unfavorable to the absorption and increases the energy usage for transporting absorbent in the CO2 separation process.
In short, both ILs and DESs show strong potential for industrial application in CO2 capture, thereby replacing traditional organic amine absorbents to overcome solvent volatility loss, equipment corrosion caused by organic amine absorbents as well as high energy usage for absorbent regeneration. Physical-based ILs show relatively low CO2 solubility and selectivity of CO2 to other gases while relatively low viscosity also. The task-specific functionalized ILs enhance CO2 solubility and selectivity, but generally possess high viscosity, complex preparation steps, high synthesis cost, and high absorption enthalpy. In comparison, DESs have received extensive research attention with the simpler preparation process, lower synthesis cost, and greener biodegradability, but generally have higher viscosities than ILs.
When ILs/DESs are used as a liquid absorbent, and the physical absorption-based technology is chosen, the CO2 mass absorption capacity is important, the viscosity needs a particular concern, and the selectivity is another important property when screening and suggesting ILs. Instead, for the chemical absorption-based technology, the CO2 mass absorption capacity, the viscosity, including that after CO2 absorption, and the desorption enthalpy are three primary properties, while the selectivity may need to concern a certain extent.
2.2 Nano-confined ionic liquids/deep eutectic solvents
2.2.1 Nano-confined ionic liquids
In order to overcome the high viscosity for functionalized ILs and low selectivity for conventional ILs as liquid absorbents, the so-called nano-confined ILs, that is, ILs are confined in nanoporous matrices, such as metal-organic frameworks (MOFs), molecular sieves, silica, and porous carbons, with their spatial geometrical dimensions have been proposed. In this case, either or both the nanoporous matrix (rigid host) and IL (soft guest) can be suitably modified for task-specific separation processes. Nano-confined ILs can effectively reduce the amount of ILs and overcome their viscosity issues in the potential application, resulting in hybrid materials with distinct advantages like low energy usage for regeneration, high selectivity, and recoverable CO2 sorption. It was found that the conventional nano-confined ILs in silica could enhance CO2 sorption capacity and rate. Shi and Luebke (2013) studied CO2 sorption performance in [HMIM][Tf2N] confined into silica slit pores with widths from 2.5 to 4.5 nm using molecular simulations. It was found that the CO2 solubility in the confined IL is significantly higher than that in the relevant bulk IL due to the increased molar volume of confined ILs. In addition to the conventional ILs, confining functionalized ILs have also been reported for CO2 capture. Zhang et al. (2006) first supported the tetrabutylphosphonium amino acid ILs on silica gels to achieve fast and reversible CO2 sorption with the capacity of 0.50 mol/mol. It is worth noting that, when most of the pore surface of support is occupied by the immobilized IL, the CO2 sorption capacity of nano-confined ILs is dominated by the immobilized ILs rather than solid adsorbent (Ren et al., 2012). Similar to the bulk ILs, the CO2 sorption capacity is always dependent on the IL molecular structures. For instance, in terms of a list of amino acid-based ILs confined in porous silica, the CO2 sorption performance was mainly dominated by anions, and the highest CO2 sorption capacity was presented at the IL with the maximum number of amino groups. Also, the nano-confined IL with the longer cationic alkyl side chain corresponds to the lower CO2 sorption capacity (Ruckart et al., 2015). These studies and findings indicate that it is essential to put the focus on ILs, and CO2 absorption capacity of bulk ILs can be used as an index to screen and suggest ILs for confinement.
In some cases, the performance of confined ILs depends on both ILs and supports. Research has demonstrated that MOF is a promising class of materials for confining ILs (Zhang et al., 2017). Ban et al. (2015) confined [BMIM][Tf2N] into the nanocages of ZIF-8 to achieve a highly selective gas separation for CO2/N2 and CO2/CH4 by adjusting the molecular sieving properties of ZIF-8. The molecular simulation by Vicent-Luna et al. (2013) indicated that adding ILs into the pore of MOFs can intensify the CO2 sorption capacity at low pressures. Whereas the sorption behavior of N2 and CH4 cannot be affected. These findings may be ascribed to the “like dissolves like” principle. The IL@MOF composites can significantly increase the selectivity of CO2/N2, and a higher IL concentration in MOFs corresponds to a higher selectivity (Xue et al., 2016). Furthermore, the selectivity of CO2 separation can be improved by achieving a better dispersion behavior of ILs in MOFs, which explains MOF is a better candidate than the covalent organic framework (COF) (Xue et al., 2016). Han et al. (2021) synthesized a novel IL-ZIF-IL composite with the shell-interlayer-core structure using [TETA]L and ZIF-8, and investigated its CO2 capture performance. The IL-ZIF-IL composite exhibited a strong molecular sieving ability, and its performance can be adjusted by controlling the IL loading, thereby changing the thickness of the outer IL layer in the IL-ZIF-IL composite. Under the different operating conditions, the selectivity of CO2/CH4 (50:50) and CO2/N2 (15:85) mixtures was as high as 260–1990 and 1,688–5,572, respectively. This fully demonstrates the potential of nano-confined ILs in ZIF-8 for the selective separation of CO2. It also indicates that the selectivity in the IL-confined technology can be tuned by adjusting other parameters than IL itself.
Zeolites or molecular sieves are also a class of promising porous materials to confine ILs for CO2 capture (Yu et al., 2014; Ahmad et al., 2018). For example, Yu et al. (2014) employed conventional ILs ([CnMIM][Br], n = 4, 6, 8, and 10) as ship in a bottle synthesized in NaY zeolite to prepare [CnMIM][Br]@NaY composites successfully. In this study, [CnMIM][Br] was incorporated inside NaY, leading to higher stability of the confined ILs than the bulk counterparts. Among the studied ILs, [C4MIM][Br] has the highest CO2 sorption capacity of up to 0.456 mol/kg, which proved the potential of confined IL in zeolites for CO2 capture. Ahmad et al. (2018) prepared poly [VEMIM][Tf2N]/zeolite composite and found that poly [VEMIM][Tf2N]/zeolite composite can improve CO2 sorption capacity when compared with the single poly [VEMIM][Tf2N].
In addition, combining ILs and membrane materials to prepare IL-based membranes is another alternative strategy to avoid the high viscosity of ILs, and more importantly, the gas separation performance can also be efficiently improved. Here, most of the work is based on polyIL as the supported polymer membrane. Compared to a pure polyIL membrane, the polyIL-IL composite membrane containing free IL can increase the permeability of CO2, N2, and CH4 by 300%–600% (Bara et al., 2008). For mixed matrix membranes (MMMs) containing polyILs or polymers, free ILs and porous particles, the presence of ILs can improve the interfacial adhesion between particles and organic polymers, increasing CO2 permeability and selectivity (Hudiono et al., 2010; Hudiono et al., 2011). Hao et al. (2013) investigated poly (RTIL)/RTIL/ZIF-8 MMMs for natural gas sweetening and post-combustion CO2 capture. In their study, the poly (RTIL) was [VBIM][Tf2N], and the RTIL was [EMIM][BF4], [EMIM][Tf2N], and [EMIM][B(CN)4]. It was found that the [VBIM][Tf2N]/[EMIM][B(CN)4]/ZIF-8 system showed the impressive CO2/N2 separation performance with the selectivity of up to 21 at 35°C and 3.5 bar.
2.2.2 Nano-confined deep eutectic solvents
DESs, as a novel class of IL analogous, have also been confined into solid materials to capture CO2. Lin et al. (2021) synthesized two-dimension materials of nano-confined DESs using DES (ChCl + ethylene glycol) and the nanoslits of titanium carbide (Ti3C2Tx), and the properties of this material were determined by hydrogen bonds (HBs) between DESs and solid materials. The nano-confined DES demonstrated good thermal stability, long-term durability, and relatively high selectivity of CO2 separation, in which the selectivity of CO2/N2, CO2/CH4, and CO2/H2 are as high as 319.15, 249.01, and 12.38, respectively. Amira et al. (2018) first reported the DES-based membranes by incorporating DES + ethylene glycol (1:3) into polyvinylidene fluoride-co-polytetrafluoroethylene to capture CO2. It was found that the CO2 permeation was up to 25.5 × 103 GPU (gas permeation unit), but the selectivity of CO2/N2 was only 2.0. (Lin et al., 2019a) further developed the DES-based membranes and prepared the graphene oxide (GO) supported DES membranes by confining ChCl + ethylene glycol with different HBA/HBD molar ratios into the GO nanoslits. The composite membranes demonstrated remarkable permeation selectivity of CO2/N2 (>400), CO2/CH4 (>300), and CO2/H2 (>20), superior thermodynamic stability, and long-term endurance. The intensifying mechanism of CO2 capture was also investigated by using molecular dynamics simulations, that is, GO provided nanoconfined space for the DES, resulting in the unique spatial configuration, as well the interaction of DES-GO weakened the original interaction between DES molecules, which enlarges the free volume and prompts the gas diffusion.
It is evident that the nano-confined DESs/ILs can overcome the major shortages of bulk ILs/DESs e.g., fluidity, high viscosity, and slow diffusion. However, it should be noted that the nano-confined DESs/ILs as a class of hybrid material consisted of non-freely moving liquids and porous solids, and the loss of liquid phase cannot be negligible. Moreover, nano-confined DESs/ILs as macroscopic solid materials may change the CO2 capture process from absorption to adsorption (or membrane separation), which is suitable for the CO2 streams with low CO2 concentration and low gas flow rate, instated of those with high-concentration CO2 capture at high gas flow rates. Therefore, IL/DES-based liquid absorbents still show their advantages, and different IL/DES-based technologies need to be developed for different cases.
Meanwhile, combining the features of ILs/DESs and IL/DES-based technologies, the key properties can be quite different. When ILs/DESs are used as a solvent, if it is a physical-based technology, CO2 solubility in weight (mass)-basis, selectivity, and viscosity need to be considered simultaneously, while for the chemical-based one, CO2 solubility in weight-basis, viscosity before and after CO2 absorption as well as desorption enthalpy should be concerned. For the advanced nano-confined ILs/DESs, CO2 solubility in weight-basis is the primary one, and desorption enthalpy may need to be included, while concerns on viscosity and selectivity can be mitigated.
3 Literature survey, data collection, properties perdition, and ionic liquids/deep eutectic solvents screening
Considering the importance of CO2 capture and separation, a lot of ILs and DESs have been reported and summarized in different review articles, while more are continuously reported individually. To collect ILs and DESs that have been developed together with their properties, firstly, the published review articles were summarized to accumulate the ILs and DESs that were summarized, and then the recently developed or reported ILs and DESs were surveyed. All the reviewed and surveyed ILs and DESs were collected together with the properties, including CO2 solubility, viscosity, selectivity/heat of absorption, and for those without providing such properties, COSMO-RS was used to predict for the promising ILs/DESs. Finally, top 10 ILs and DESs were suggested for different IL/DES-based technology developments.
During this procedure, the concern on selectivity depends on the gas streams for CO2 separation. Since selectivity is more essential for the pure physical-based ILs and DESs, which are preferable for the gas streams with relatively high CO2 content, such as biogas or natural gas (CH4+CO2), synthesis gas (H2+CO2, H2+CO2+CO), here CH4, CO, and H2, together with N2, were also concerned in order to estimate the ideal selectivity.
It should be pointed out that corrosion is an important property, while to the best of our knowledge, the relevant study on ILs/DESs is still limited, and thus such property was excluded in screening ILs/DESs.
3.1 Summary of previous review articles
Rashid (Rashid, 2021) reviewed the ILs developed in 2010–2020 to separate gases such as CO2, etc., where imidazolium-, amine-, phosphonium- and guanidium-based ILs were reviewed for CO2 separation, and [BMIM]+ was identified as the most-widely used cation. Shama et al. (2021) summarized the synthesis protocol and process, carbon capture capacity, absorption type (physical or chemical), as well as merits and demerits of ILs developed in 2006–2020. [Gly][ChLac], [Gly][EMIMCl], [BMIM][PF6], [EMIM][Tf2N], [HMIM][Tf2N], [ChCl][Urea], [ChCl][Gly], and [ChCl][EG] were included as physical absorption, and phosphonium-, amine-, imidazolium-, and choline-based ILs were collected as the chemical absorption. Sood et al. (2021) evaluated the recent advances in the IL-based CO2 capture in 2017–2020/2021, in which imidazolium-, phosphonium-, and amine-based ILs were reviewed, and [EMIM][NTF2] was identified as a profitable IL. Pellegrini et al. (2021) introduced ILs as the water-lean solvent for CO2 capture, and amine-, imidazolium-, and ammonium-based ILs were specifically discussed. Also, DESs, including ChCl:urea and polyamine hydrochloride:thymol, were introduced for CO2 capture.
Liu et al. (2021) summarized CO2 solubility in physical- and chemical-based ILs and DESs. In terms of physical-based ILs, ammonium-, phosphonium-, imidazolium-, pyrrolidinium-, and sulfonyl-based were reviewed, and [BMIM][BF4] (4.20 mol/kg, 273.15 K, 20.89 bar), [AMIM][Tf2N] (3.88 mol/kg, 313.2 K, 57.1 bar), and DEA][Bu] (3.71 mol/kg, 303 K, 99.35 bar) were selected as top three. For those chemical-based, imidazolium-, imide-, gunidinium-, phosphonium-, amino functionalized- and ammonium-based were collected, and [DETAH][Im] (11.91 mol/kg), [DETAH][Py] (11.39 mol/kg), and [DETAH][Gly] (10.15 mol/kg) were selected as the top three. The physical-based DESs were categorized into phosphonium- and ammonium-based as well as [ChCl][EA], [ChCl][U], [ChCl][1,2-propanediol], [L-Arg][Gly], [GuaCl][EA], [BHDECl][LA], and [BHDECl][acetic acid]. [N4444Cl][DECA] (1:2) showed the highest solubility of 1.52 mol/kg at 298.15 K and 19.9 bar. The chemical-based DESs were classified into amine-, imidazolium-, ammonium-based as well as DESs comprised of DBU and TBD combined with various alcohols including ethylene glycol, benzyl alcohol, 2-imidazolidone, dimethylolurea, 1,3-dimethylurea, and MDEA. [TBD][EG] (1:4) delineated the highest solubility of 12.9 mol/kg at 298.15 K and 1 bar.
Wibowo et al. (2021) summarized the application of DESs for CO2 capture from gas streams, specially syngas, where CO2 solubilities in the DESs developed in 2012–2020 were summarized. This review covered the DESs composed of ChCl as HBA mixed with various HBDs, including triethylene glycol, ethylene glycol, urea, glycerol, guiacol, and MEA. Besides, imidazolium-, phosphonium- and ammonium-based DES as well as [L-Arg][Gly] were included. Hansen et al. (2020) reviewed the fundamentals and applications of DES. Here, ammonium-, phosphonium-, and imidazolium-based DESs as well as the DESs formed by the combination of ChCl with urea, ethylene glycol, levulinic acid, alcium chloride hexahydrate, and furfuryl alcohol were collected.Wang et al. (2021b) reviewed functionalized and non-functionalized DES used for CO2, SO2, and NO capture. Choline-, amine-, and ammonium-based DESs in addition to [Gua][MEA], [DBN][DMLU], [DBN][DMU], [DBN][EU], [UEC][EDA], and [DBN][EU] were reported. In terms of physical-based DESs, [TPAC][MEA] exhibited the highest capacity of 1.71–3.53 mol/kg, and [MEAC][EDA] showed the highest capacity of 8.86 mol/kg within the functionalized DESs.
3.2 Review of ionic liquids and deep eutectic solvents developed since 2021
According to the survey, the developed ILs and DESs up to 2020 have been well collected and summarized, and thus, the newly published ILs and DESs since 2021 were surveyed and collected in this part based on the Web of Science (WOS) database.
3.2.1 Physical-based ionic liquids
11 studies have been focused on physical-based ILs since 2021. The studied ILs and corresponding properties and other information are summarized in Table 1. Here, Thapaliya et al. (2021) synthesized two tetracationic ILs (DABCO-T and DABCO-B) and four dicationic ILs (2OEt-Im, 3OEt-Im, C8-Im, and C10-Im), among which 3OEt-Im represented the highest capacity of CO2 (0.433 mol/kg at 298.15 K, 10 bar). Jalili et al. (2022) synthesized 1-benzyl-3-methylimidazolium bis (trifluoromethylsulfonyl) imide ([BZMIM][Tf2N]) IL. The CO2 solubility reached 1.919 ± 0.054 mol/kg at 303.15 K and 30.5 bar, and the selectivity over H2 reached 2.89.
Besides, the research work was also conducted for the reported ILs. For example, Wu et al. (2021) studied the CO2 solubility of three ILs ([EMIM][FAP], [HMIM][FAP], and [BMIM][FAP]) in a wide temperature (293.15–333.15 K) and pressure (0–50 bar) range. Safarov et al. (2021) chose 1-ethyl-3-methylimidazolium methanesulfonate [EMIM][CH3SO3] with a solubility of 3.3 mol/kg at 293.15 K and 45.77 bar. Peng et al. (2021). investigated [HMIM][Tf2N], [BMIM][Tf2N], [EMIM][Tf2N], and [OMIM][Tf2N] at 298.15–328.15 K and 20–50 bar. Yim et al. (2021) selected [BVIM][Tf2N], [N4222][Tf2N], and [P4441][Tf2N].
3.2.2 Chemical-based ionic liquids
16 studies have been focused on chemical-based ILs since 2021, which are listed in Table 2. During this period, Zema et al. (2021) prepared K+ chelated dual functional ILs using monoethanolamine (MEA), 2-amino-1-butanol (AMB), 2-amino-2-methyl-1-propanol (AMP), and DL-1- amino-2-propanol (DLAMP), and it was found that CO2 solubility (mol/kg) followed [K (AMP)2][Im] > [K (DLAMP)2][Im] > [K (AMB)2][Im] > [K (MEA)2][Im]. Qu et al. (2021) synthesized amino acid ILs (AAILs), including 1-methoxylbutyl-3-methylimidazolium lysine [MOBMIM][Lys], 1-methoxylbutyl-3-methylimidazolium arginine [MOBMIM][Arg], 1-methoxylbutyl-3-methylimidazolium glycine [MOBMIM][Gly], and 1-methoxylbutyl-3-methylimidazolium histidine [MOBMIM][His], and [MOBMIM][Gly] exhibited the highest mass absorption capacity (9.66 mol/kg) and the lowest viscosity. After the absorption, [MOBMIM][His] and [MOBMIM][Gly] stayed in the liquid phase, while [MOBMIM][Arg] and [MOBMIM][Gly] turned to gel. Recently, Zailani et al. (2022) synthesized ammonium based protic ILs using 2-ethylhexylammonium and bis-(2-ethylhexyl) ammonium coupled with pentanoate, hexanoate, and heptanoate ([EHA][C5], [EHA][C6], [EHA][C7], [BEHA][C5], [BEHA][C6], and [BEHA][C7]). The absorption capacity (mol/kg) decreased in the order of [C5] < [C6] < [C7], and [EHA][C7] showed the highest absorption capacity (∼3.0 mol/kg at 29 bar and room temperature). Taking the advantage of metal ion coordination, Suo et al. (2022) synthesized metal-ion-amino-based IL using metal salts {[M(OctNH2)4][NTf2]2, M = Co, Cu, Ni, Zn, and Mg}. The CO2 absorption capacity (mol/kg) followed the order of [Li(OctNH2)4][NTf2]2 > [Mg (DecNH2)4][NTf2]2 ≈ [Mg(OctNH2)4][NTf2]2 > [Ni(OctNH2)4][NTf2]2 > [Ni(DecNH2)4][NTf2]2 > [Co(OctNH2)4][NTf2]2 > [Zn(OctNH2)4][NTf2]2 > [CuMg(DecNH2)4][NTf2]2 > [Cu(OctNH2)4][NTf2]2 > [CuZnNiMg(DecNH2)4][NTf2]2 > [CuZn(DecNH2)4][NTf2]2. Additionally, it was stated that the addition of metal sites led to a significant enhancement of thermal stability. Keller et al. (2022) designed and synthesized aprotic N-heterocyclic anion ILs using triethyl (octyl)-phosphonium cations and different aprotic N-heterocyclic anions. [P2228][5MeBnIm], [P2228][3SH-4Triz], and [P2228][BnTriz] [P2228][5BrInda] pose high melting point before and after CO2 absorption (>333 K), while [P2228][4BrIm], [P2228][4Br3MePyra], [P2228][3ClInda], [P2228][4BrInda], [P2228][6BrInda], [P2228][6CNInda], and [P2228][3IPyra] were studied for CO2 absorption. It was observed that the indazolide-/pyrazolide-based ILs became solid after absorption, while [P2228][4BrIm] remained liquid. Among them, [P2228][4Br3MePyra] exhibited the highest solubility of 2.498 mol/kg at 321.8 K and 0.798 bar, and the uptake capacity (mol/kg) decreased in the order of [4Br3MePyra]− > [6BrInda]− > [3ClInda]− > [4BrInda]− > [3IPyra]− > [4BrIm]− > [6CNInda]−. Min et al. (2021) synthesized 1-ethyl-3- methylimidazolium glycinate ([EMIM][Gly]), and the presence of water enhanced the CO2 absorption capacity (8.645 mol/kg) at 25°C and 40 ml/min gas flow rate. Besides, Mei et al. (2022) restudied 1-ethyl-3-methylimidazolium acetate ([EMIM][OAc).
3.2.3 Physical-based deep eutectic solvents
12 studies have been focused on physical-based DESs since 2021, and their specific names, together with other information, are summarized in Table 3. Among them, two are newly synthesized (Deng et al., 2021; Pishro et al., 2021), while others are the previously synthesized DESs with additional efforts on e.g., high-pressure CO2 solubility (Haghbakhsh et al., 2021), the effects of water content (Liu et al., 2022), pressure/temperature/stirring rate/water content (Aboshatta and Magueijo, 2021), pressure/temperature/water content (Yan et al., 2022), HBA:HBD molar ratios (Luo et al., 2021; Qin et al., 2021), HBA and HBD nature (Rabhi et al., 2020; Haider et al., 2021; Luo et al., 2021; Mihăilă et al., 2021) on the CO2 solubility.
For the new DESs, Pishro et al. (2021) synthesized ammonium-based ones using ethanolamine hydrochloride (EAHC) and tetraethylenepentamine (TEPA) with various molar ratios and the one with 1:9 (molar ratio) exhibited the highest solubility of 9.671 mol/kg at 303.15 K and 16.03 bar.
Overall, choline chloride is widely used as HBA, while tetrabutylammonium bromide, methyltriphenylphosphonium bromide, imidazole, guanidine isothiocyanate, and ethanol amine hydrochloride were also used. In terms of HBD, various fatty acids (hexanoic, decanoic, dodecanoic acids, Levulinic-acid), alcohols (glycerol, ethylene glycol, diethylene glycol, triethylene glycol), amines (tetraethylenepenta amine, n-methyldiethanolamine, monoethanolamine), amides (urea, acetamide), and p-toluenesulfonic acid have been utilized.
3.2.4 Chemical-based deep eutectic solvents
Nine studies have been focused on chemical-based DESs since 2021, as summarized in Table 4. Among them, three DESs are newly synthesized (Fu et al., 2021a; Ahmad et al., 2021; Qian et al., 2022), while others are the previously synthesized ones for various purposes, including the discussion about the water content (Liu et al., 2022) and estimating the effects of HBA:HBD molar ration, type of anion, and the alkyl chain (Cichowska-Kopczyńska et al., 2021).
Ahmad et al. (2021) synthesized the MDEA-based DESs using [DEAHCl], [MDEAHCl], and [MEAHCl] as HBA and [MDEA] as HBD at 1:3 M ratio, and the CO2 solubility (mol/kg) followed the order of ∼2.63 > 2.46 > 1.35 at 298.15 K and 1 bar for the following DESs, [MEAHCl][MDEA], [DEAHCl][MDEA], and [MDEAHCl][MDEA]. The viscosity of the prepared DESs was in the range of 200–300 cP, while after CO2 absorption, it was increased up to ∼1,500 cP for the first two DES and ∼410 cP for the last one. Fu et al. (2021a) synthesized various types of DESs using 1,8-Diazabicyclo [5.4.0] undec-7-ene (DBU) combined with pyrrolidine, 2-pyrrolidinone, oxazolidinone, and ethyleneurea, and their solubilities (mol/kg) followed the order of [DBU][Pyr] (2:1) > [DBU][Eth] (2:1) > [DBU][Py] > (1:1) > [DNU][Oxa] (2:1), i.e., 8.2 > 7.5 > 6.5 > 6.3 mol/kg at 303 K and 1 bar. Qian et al. (2022) synthesized DESs using glycine and proline as HBA, and monoethanolamine and methyl diethanolamine as HBD with 1:4 M ratio for the post-combustion CO2 capture. The solubility (mol/kg) decreased in the following order: [Gly][MEA] > [Pro][MEA] > [Pro][MDEA], i.e., 5.2 > 4.6 > 1.1 mol/kg, at 298.15 K and 1.01 bar.
Apart from two-component DESs, Qian et al. (2022) synthesized three-component ones using amino acids as HBA, amines as HBDs, and 10–30 wt% of ethylene glycol. In this case, the CO2 solubility reached up to 5 mol/kg. Some DESs have been made by mixing ILs ethylene glycol and 4-methylimidazole (Fu et al., 2021b; Wang et al., 2021c; Lee et al., 2021; Wang et al., 2022a).
In summary, most of the work since 2021 is mainly on the synthesis of chemical-based ILs. Also, a great effort has been devoted to the imidazulim-, phosphonium- and ammonium-based ILs, which were previously synthesized. Among various physical-based ILs, [BVIM][Tf2N] showed the highest capacity of 6.3 mol/kg at 303.2 K and 71.1 bar. For the chemical-based ILs, [DETAH][Im] exhibited the highest solubility of 11.9 mol/kg at 313.15 K and 1 bar. For CO2 capture by DESs, choline-, ammonium-, phosphonium-, imidazolium-based solvents have been widely considered. In terms of the physical-based DESs, the highest solubility reached 9.7 mol/kg for [EAHC][TEPA] at 303.15 K and 16.03 bar [DBU][EG] captured CO2 with the highest capacity up to 12.5 mol/kg at 298.15 K and 1 bar as the chemical-based DES. Even though DESs show more promising compared to ILs, the research work is still relatively less. More focus needs to be on DESs.
3.3 Data collection, properties prediction, and ionic liquids/deep eutectic solvents screening
Based on the information from the previous reviews published in recent years as well as the new ILs and DESs that have been surveyed in this work, the available ILs and DESs that have been developed are summarized in Tables 1–4; Supplementary Table S1–S2. According to the survey and collection, the CO2 solubility is the main concern during the development, while the viscosity, selectivity and/or heat of absorption may also be covered, which are also essential for developing different IL/DES-based technologies.
Based on the collected ILs and DESs, promising ones can be suggested for different IL/DES-based technologies based on different criteria. In this work, the screening was conducted based on two options, according to the characterizations of different IL/DES-based technologies for CO2 separation.
3.3.1 Option 1: Top 10 ionic liquids/deep eutectic solvents based on the CO2 absorption capacity
For the technology of immobilization, i.e., nano-confined ILs/DESs for CO2 capture, the CO2 absorption capacity is essential, while the challenges related to viscosity and selectivity can be mitigated compared to the technologies with bulk ILs/DESs. Therefore, according to the results listed in Tables 1–4; Supplementary Table S1–S2, top 10 ILs/DESs based on CO2 absorption capacity were screened from all the studied ILs/DESs for each type, i.e., physical or chemical-based. The screened ILs/DESs are listed in Table 5.
3.3.2 Option 2: Top 10 ionic liquids/deep eutectic solvents based on a set of properties
In this option, top 10 ILs/DESs were screened based on a set of properties, including CO2 absorption capacity, viscosity, and selectivity/heat of absorption. For the solvent-based technologies with ILs/DESs, desirable properties more than CO2 absorption capacity are needed. However, not all the properties are available for the collected ILs/DESs. To achieve the screening, the work was conducted step by step. Step 1: top 20 ILs/DESs were screened based on the CO2 absorption capacity, and other properties of these top 20 ILs/DESs were combined or predicted with COSMO-RS; Step 2: top 10 ILs/DESs were further screened based on the different sets of parameters, depending on the feature of ILs/DESs (chemical-based or physical-based).
3.3.2.1 Properties for the top 20 ionic liquids/deep eutectic solvents
According to the CO2 absorption capacity of ILs and DESs collected in Tables 1–4; Supplementary Table S1–S2, top 20 ILs/DESs were selected. The chosen 20 ILs/DESs are listed in Supplementary Table S3–S6.
To conduct screening, as most important properties other than CO2 absorption capacity are unavailable, theoretical predictions can be desirable. The COSMO-RS model, as a prior method, has been widely used to predict a variety of thermodynamic properties, such as the phase equilibrium, saturated vapor pressure, infinite dilution activity coefficient of systems containing ILs and DESs, and identify molecular mechanisms in chemical separation and reaction processes (Klamt, 1995; Zhou et al., 2012; Klamt et al., 2016; Yu et al., 2022), and the previous research has evidenced the reliability of COSMO-RS in screening ILs and DESs (Palomar et al., 2011; Sistla and Khanna, 2011; Hadj-Kali et al., 2020). In this work, the COSMO-RS model was employed to evaluate the CO2 solubility, selectivity of CO2 to other gases (i.e., CO, H2, CH4, and N2) and CO2 excess enthalpy in ILs and DESs as well as the viscosity of ILs by means of the COSMOThermX19 software. The selectivity of different gases was obtained from the corresponding Henry’s constants.
In the COSMO-RS calculation, the molecular geometry structures of HBAs and HBDs in DESs as well as cations and anions in ILs were optimized based on the density functional theory (DFT) at the calculation level of B3LYP/6–31+G (d, p) (Curtiss et al., 1995) by using the Gaussian 09 program (Frisch et al., 2009). Then, the COSMO file was obtained based on the most stable geometrical configuration by carrying out the calculation of COSMO continuum solution models at the theoretical level of BP86/TZVP (Perdew, 1986; Schäfer et al., 1994).
Using COSMO-RS, the viscosity, selectivity, and CO2 excess enthalpy in ILs and DESs were estimated for the top 20 ILs/DESs. The results are summarized in Supplementary Table S7–S8, and depicted in Figures 1–3. Here, it should be mentioned that COSMO-RS cannot be used to predict the viscosities of DESs.
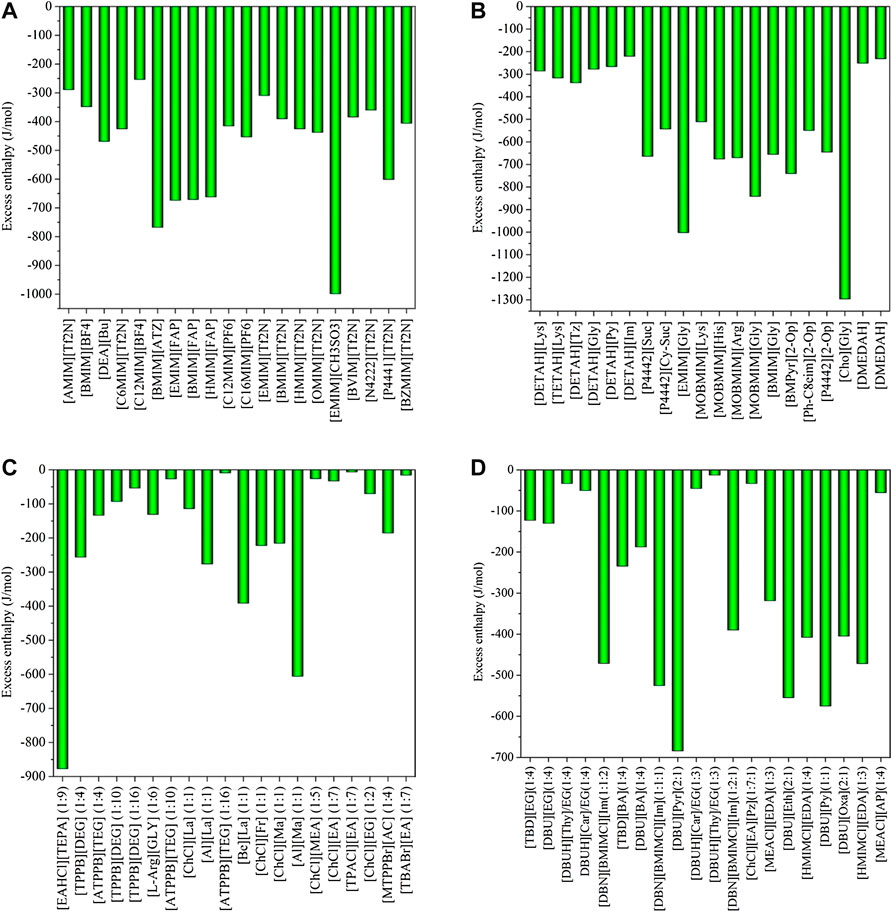
FIGURE 1. Excess enthalpies of equimolar CO2 in physical-based ILs (A), chemical-based ILs (B), physical-based DESs (C) and chemical-based DESs (D) predicted by the COSMO-RS model at 298.15 (K)
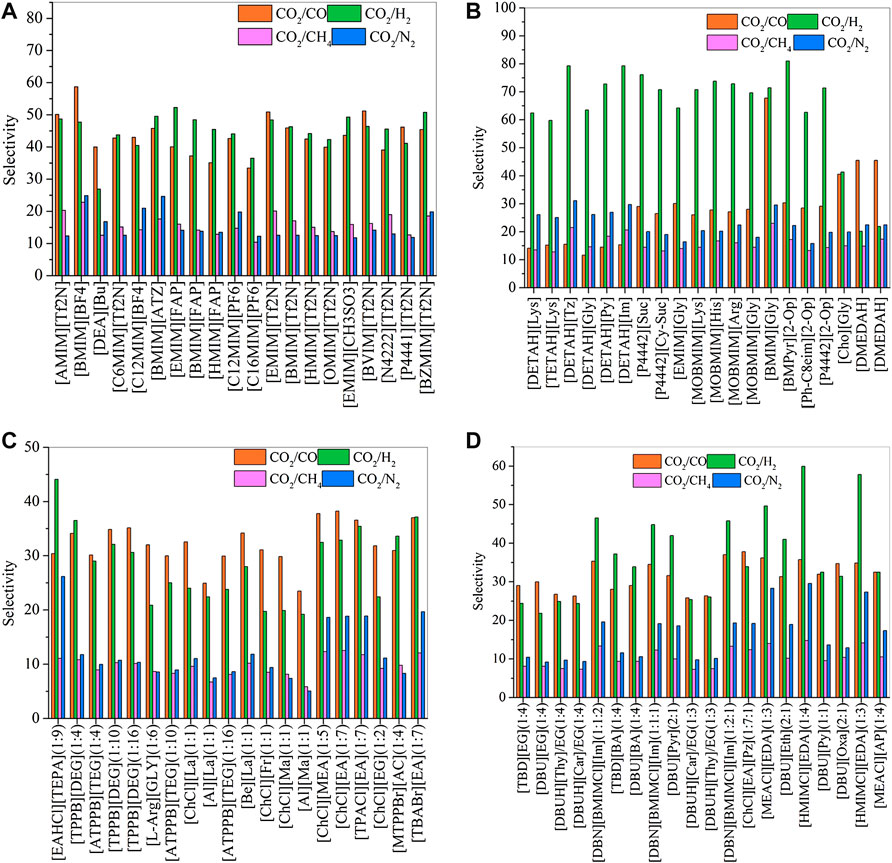
FIGURE 2. Selectivities of CO2/CO, CO2/H2, CO2/CH4, and CO2/N2 in physical-based ILs (A), chemical-based ILs (B), physical-based DESs (C) and chemical-based DESs (D) predicted by the COSMO-RS model at 298.15 (K)
Generally, CO2 excess enthalpy and selectivity for physical-based ILs/DESs should be lower than those of chemical-based. According to the prediction results of COSMO-RS (Figure 1), some physical-based ILs/DESs show even higher CO2 excess enthalpies than those of chemical-based, and the selectivity of chemical-based ILs/DESs is not as high as we expected (Figure 2), especially for CO2 over CO and CH4 in chemical-based ILs. These observations indicate that the qualitative prediction of COSMO-RS may need to be verified and further improved. However, no work has been conducted to study the selectively for the chemical-based ILs/DESs, and the reliability of COSMO-RS prediction needs to be further verified. As shown in Figure 3, the viscosity of the chemical-based ILs is in general higher than the physical-based, which is consistent with the observation from experiments. For almost all the top 20 ILs/DESs, the properties other than CO2 absorption capacity were not determined experimentally. In this work, COSMO-RS was thus used for a rough estimation for qualitative comparison. It should be mentioned here that, the molar ratios of some physical-based DESs were not mentioned in the literature, and thus the prediction with COSMO-RS cannot be performed. In this case, other DESs were added in the top 20 list to facilitate the utilization of COSMO-RS (see Supplementary Table S6).
3.3.2.2 Top 10 ionic liquids/deep eutectic solvents
As discussed in the previous section, the CO2 absorption capacity (mass basis), viscosity, and selectivity were used as the criteria to screen physical-based ILs/DESs, while the absorption capacity (mass basis), viscosity (before and after CO2 absorption), and CO2 absorption enthalpy were needed to screening chemical-based ILs/DESs. However, the viscosities of most DESs are not accessible, and it was excluded in the DES screening in this section. For the chemical-based ILs/DESs, the viscosity after CO2 absorption is also important, which again is inaccessible for most ILs/DESs. To address this, CO2 excess enthalpy in ILs and DESs was used as the index to reflect this. Also, CO2 absorption capacity is another important issue, which was also reflected with the CO2 excess enthalpy in ILs/DESs. Therefore, the top 20 ILs/DESs were further evaluated.
According to the results depicted in Figures 4–7, top 10 ILs/DESs were screened, and the results are summarized in Table 6.
4 Conclusion
Owing to the unique properties of ILs and DESs, intensive research has been conducted to develop such novel liquids for CO2 capture. In this work, different IL/DES-based technologies were summarized, and the important properties of each technology were identified to provide a guideline for screening the desirable ILs/DESs. Subsequently, the ILs and DESs studied in the recent 2 years were surveyed and then combined with those collected in the previous review articles to provide an extensive database. Furthermore, when the experimental data was not available, COSMO-RS was used to predict the properties that are needed for screening. Based on the established database, the identified important properties, and the predictions from the COSMO-RS, top 10 ILs/DESs were screened for different IL/DES-based technologies, being beneficial for further evaluation and development.
Author contributions
SF, GY, and XJ: Writing—Review & Editing by.
Acknowledgments
The authors thank the financial support from the Swedish Energy Agency.
Conflict of interest
The authors declare that the research was conducted in the absence of any commercial or financial relationships that could be construed as a potential conflict of interest.
Publisher’s note
All claims expressed in this article are solely those of the authors and do not necessarily represent those of their affiliated organizations, or those of the publisher, the editors and the reviewers. Any product that may be evaluated in this article, or claim that may be made by its manufacturer, is not guaranteed or endorsed by the publisher.
Supplementary material
The Supplementary Material for this article can be found online at: https://www.frontiersin.org/articles/10.3389/fchem.2022.951951/full#supplementary-material
References
Aboshatta, M., and Magueijo, V. (2021). A comprehensive study of CO2 absorption and desorption by choline-chloride/levulinic-acid-based deep eutectic solvents. Molecules 26, 5595. doi:10.3390/molecules26185595
Adhi, T. P., Situmorang, Y. A., Winoto, H. P., Ariono, D., Septiana, D., Imanuela, P., et al. (2021). H2S–CO2 gas separation with ionic liquids on low ratio of H2S/CO2. Heliyon 7, e08611. doi:10.1016/j.heliyon.2021.e08611
Ahmad, N., Lin, X., Wang, X., Xu, J., and Xu, X. (2021). Understanding the CO2 capture performance by MDEA-based deep eutectics solvents with excellent cyclic capacity. Fuel 293, 120466. doi:10.1016/j.fuel.2021.120466
Ahmad, N., Reddy, A. V. B., Saha, B. B., and Moniruzzaman, M. (2018). (Synthesis and characterization of ionic liquid polymer composite with zeolite and its application for carbon dioxide capture. IOP Conf. Ser. Mater. Sci. Eng. 458, 012009. doi:10.1088/1757-899X/458/1/012009
Ahmed, S. F., Mofijur, M., Tarannum, K., Chowdhury, A. T., Rafa, N., Nuzhat, S., et al. (2021). Biogas upgrading, economy and utilization: A review. Environ. Chem. Lett. 19, 4137–4164. doi:10.1007/s10311-021-01292-x
Alcantara, M. L., Santos, J. P., Loreno, M., Ferreira, P. I., Paredes, M. L., Cardozo-Filho, L., et al. (2018). Low viscosity protic ionic liquid for CO2/CH4 separation: Thermophysical and high-pressure phase equilibria for diethylammonium butanoate. Fluid Phase Equilibria 459, 30–43. doi:10.1016/j.fluid.2017.12.001
Alkhatib, I. I., Ferreira, M. L., Alba, C. G., Bahamon, D., Llovell, F. L., Pereiro, A. B., et al. (2020). Screening of ionic liquids and deep eutectic solvents for physical CO2 absorption by Soft-SAFT using key performance indicators. J. Chem. Eng. Data 65, 5844–5861. doi:10.1021/acs.jced.0c00750
Altamash, T., Amhamed, A., Aparicio, S., and Atilhan, M. (2020). Effect of hydrogen bond donors and acceptors on CO2 absorption by deep eutectic solvents. Processes 8, 1533. doi:10.3390/pr8121533
Amira, M., Hatim, M. I., Jullok, N., Rasidi, M. S., and Alamery, H. R. (2018). Synthesis and preparation of asymmetric PVDF-co-PTFE/DES supported membrane for CO2/N2 separation. IOP Conf. Ser. Mater. Sci. Eng. 429, 012067. doi:10.1088/1757-899X/429/1/012067
Amiri, N., Benyounes, H., Lounis, Z., and Shen, W. (2021). Design of absorption process for CO2 capture using cyano based anion ionic liquid. Chem. Eng. Res. Des. 169, 239–249. doi:10.1016/j.cherd.2021.03.014
Anthony, J. L., Anderson, J. L., Maginn, E. J., and Brennecke, J. F. (2005). Anion effects on gas solubility in ionic liquids. J. Phys. Chem. B 109, 6366–6374. doi:10.1021/jp046404l
Avila, J., Lepre, L. F., Goloviznina, K., Guazzelli, L., Pomelli, C. S., Chiappe, C., et al. (2021). Improved carbon dioxide absorption in double-charged ionic liquids. Phys. Chem. Chem. Phys. 23, 23130–23140. doi:10.1039/d1cp02080c
Ban, Y., Li, Z., Li, Y., Peng, Y., Jin, H., Jiao, W., et al. (2015). Confinement of ionic liquids in nanocages: Tailoring the molecular sieving properties of ZIF‐8 for membrane‐based CO2 capture. Angew. Chem. Int. Ed. Engl. 54, 15703–15707. doi:10.1002/ange.201505508
Bara, J. E., Hatakeyama, E. S., Gin, D. L., and Noble, R. D. (2008). Improving CO2 permeability in polymerized room‐temperature ionic liquid gas separation membranes through the formation of a solid composite with a room‐temperature ionic liquid. Polym. Adv. Technol. 19, 1415–1420. doi:10.1002/pat.1209
Bates, E. D., Mayton, R. D., Ntai, I., and Davis, J. H. (2002). CO2 capture by a task-specific ionic liquid. J. Am. Chem. Soc. 124, 926–927. doi:10.1021/ja017593d
Blanchard, L. A., Gu, Z., and Brennecke, J. F. (2001). High-pressure phase behavior of ionic liquid/CO2 systems. J. Phys. Chem. B 105, 2437–2444. doi:10.1021/jp003309d
Chen, F. F., Huang, K., Zhou, Y., Tian, Z. Q., Zhu, X., Tao, D. J., et al. (2016). Multi‐molar absorption of CO2 by the activation of carboxylate groups in amino acid ionic liquids. Angew. Chem. Int. Ed. Engl. 128, 7282–7286. doi:10.1002/ange.201602919
Cichowska-Kopczyńska, I., Warmińska, D., and Nowosielski, B. (2021). Solubility of carbon dioxide in deep eutectic solvents based on 3-amino-1-propanol and tetraalkylammonium salts at low pressure. Materials 14, 594. doi:10.3390/ma14030594
Curtiss, L. A., Mcgrath, M. P., Blaudeau, J. P., Davis, N. E., Binning, R. C., Radom, L., et al. (1995). Extension of Gaussian‐2 theory to molecules containing third‐row atoms Ga–Kr. J. Chem. Phys. 103, 6104–6113. doi:10.1063/1.470438
Dai, C., Lei, Z., and Chen, B. (2017). Gas solubility in long‐chain imidazolium‐based ionic liquids. AIChE J. 63, 1792–1798. doi:10.1002/aic.15711
De Riva, J., Ferro, V., Moya, C., Stadtherr, M., Brennecke, J., Palomar, J., et al. (2018). Aspen Plus supported analysis of the post-combustion CO2 capture by chemical absorption using the [P2228] [CNPyr] and [P66614] [CNPyr] AHA Ionic Liquids. Int. J. Greenh. Gas Control 78, 94–102. doi:10.1016/j.ijggc.2018.07.016
Deng, D., Deng, X., Duan, X., and Gong, L. (2021). Protic guanidine isothiocyanate plus acetamide deep eutectic solvents with low viscosity for efficient NH3 capture and NH3/CO2 separation. J. Mol. Liq. 324, 114719. doi:10.1016/j.molliq.2020.114719
Eike, D. M., Brennecke, J. F., and Maginn, E. J. (2004). Predicting infinite-dilution activity coefficients of organic solutes in ionic liquids. Ind. Eng. Chem. Res. 43, 1039–1048. doi:10.1021/ie034152p
Farahipour, R., Mehrkesh, A., and Karunanithi, A. T. (2016). A systematic screening methodology towards exploration of ionic liquids for CO2 capture processes. Chem. Eng. Sci. 145, 126–132. doi:10.1016/j.ces.2015.12.015
Frisch, M., Trucks, G., Schlegel, H., Scuseria, G., Robb, M., Cheeseman, J., et al. (2009). Gaussian 09 revision D. 01, 2009. Wallingford CT: Gaussian Inc.
Fu, H., Hou, Y., Sang, H., Mu, T., Lin, X., Peng, Z., et al. (2021a). Carbon dioxide capture by new DBU‐based DES: The relationship between ionicity and absorptive capacity. AIChE J. 67, e17244. doi:10.1002/aic.17244
Fu, H., Wang, X., Sang, H., Liu, J., Lin, X., Zhang, L., et al. (2021b). Highly efficient absorption of carbon dioxide by EG-assisted DBU-based deep eutectic solvents. J. CO2 Util. 43, 101372. doi:10.1016/j.jcou.2020.101372
García-Argüelles, S., Ferrer, M. L., Iglesias, M., Del Monte, F., and Gutiérrez, M. C. (2017). Study of superbase-based deep eutectic solvents as the catalyst in the chemical fixation of CO2 into cyclic carbonates under mild conditions. Materials 10, 759. doi:10.3390/ma10070759
García-GutiéRrez, P., Jacquemin, J., Mccrellis, C., Dimitriou, I., Taylor, S. R., Hardacre, C., et al. (2016). Techno-economic feasibility of selective CO2 capture processes from biogas streams using ionic liquids as physical absorbents. Energy fuels. 30, 5052–5064. doi:10.1021/acs.energyfuels.6b00364
Ghaedi, H., Ayoub, M., Sufian, S., Shariff, A. M., Hailegiorgis, S. M., Khan, S. N., et al. (2017). CO2 capture with the help of Phosphonium-based deep eutectic solvents. J. Mol. Liq. 243, 564–571. doi:10.1016/j.molliq.2017.08.046
Ghanbari, T., Abnisa, F., and Daud, W. M. a. W. (2020). A review on production of metal organic frameworks (MOF) for CO2 adsorption. Sci. Total Environ. 707, 135090. doi:10.1016/j.scitotenv.2019.135090
Gohndrone, T. R., Song, T., Desilva, M. A., and Brennecke, J. F. (2021). Quantification of ylide formation in phosphonium-based ionic liquids reacted with CO2. J. Phys. Chem. B 125, 6649–6657. doi:10.1021/acs.jpcb.1c03546
Greer, A. J., Taylor, S. R., Daly, H., Quesne, M. G., De Leeuw, N. H., Catlow, C. R. A., et al. (2021). Combined experimental and theoretical study of the competitive absorption of CO2 and NO2 by a superbase ionic liquid. ACS Sustain. Chem. Eng. 9, 7578–7586. doi:10.1021/acssuschemeng.1c01451
Gurkan, B. E., De La Fuente, J. C., Mindrup, E. M., Ficke, L. E., Goodrich, B. F., Price, E. A., et al. (2010). Equimolar CO2 absorption by anion-functionalized ionic liquids. J. Am. Chem. Soc. 132, 2116–2117. doi:10.1021/ja909305t
Hadj-Kali, M. K., Althuluth, M., Mokraoui, S., Wazeer, I., Ali, E., Richon, D., et al. (2020). Screening of ionic liquids for gas separation using COSMO-RS and comparison between performances of ionic liquids and aqueous alkanolamine solutions. Chem. Eng. Commun. 207, 1264–1277. doi:10.1080/00986445.2019.1680363
Haghbakhsh, R., Keshtkar, M., Shariati, A., and Raeissi, S. (2021). Experimental investigation of carbon dioxide solubility in the deep eutectic solvent (1 ChCl+ 3 triethylene glycol) and modeling by the CPA EoS. J. Mol. Liq. 330, 115647. doi:10.1016/j.molliq.2021.115647
Haider, M. B., Maheshwari, P., and Kumar, R. (2021). CO2 capture from flue gas using phosphonium based deep eutectic solvents: Modeling and simulation approach. J. Environ. Chem. Eng. 9, 106727. doi:10.1016/j.jece.2021.106727
Han, G., Yu, N., Liu, D., Yu, G., Chen, X., Zhong, C., et al. (2021). Stepped enhancement of CO2 adsorption and separation in IL‐ZIF‐IL composites with shell‐interlayer‐core structure. AIChE J. 67, e17112. doi:10.1002/aic.17112
Hansen, B. B., Spittle, S., Chen, B., Poe, D., Zhang, Y., Klein, J. M., et al. (2020). Deep eutectic solvents: A review of fundamentals and applications. Chem. Rev. 121, 1232–1285. doi:10.1021/acs.chemrev.0c00385
Hao, L., Li, P., Yang, T., and Chung, T.-S. (2013). Room temperature ionic liquid/ZIF-8 mixed-matrix membranes for natural gas sweetening and post-combustion CO2 capture. J. Membr. Sci. 436, 221–231. doi:10.1016/j.memsci.2013.02.034
Hospital-Benito, D., Lemus, J., Moya, C., Santiago, R., Ferro, V., Palomar, J., et al. (2021). Techno-economic feasibility of ionic liquids-based CO2 chemical capture processes. Chem. Eng. J. 407, 127196. doi:10.1016/j.cej.2020.127196
Hudiono, Y. C., Carlisle, T. K., Bara, J. E., Zhang, Y., Gin, D. L., Noble, R. D., et al. (2010). A three-component mixed-matrix membrane with enhanced CO2 separation properties based on zeolites and ionic liquid materials. J. Membr. Sci. 350, 117–123. doi:10.1016/j.memsci.2009.12.018
Hudiono, Y. C., Carlisle, T. K., Lafrate, A. L., Gin, D. L., and Noble, R. D. (2011). Novel mixed matrix membranes based on polymerizable room-temperature ionic liquids and SAPO-34 particles to improve CO2 separation. J. Membr. Sci. 370, 141–148. doi:10.1016/j.memsci.2011.01.012
Jalili, A. H., Mehdizadeh, A., Ahmadi, A. N., Zoghi, A. T., and Shokouhi, M. (2022). Solubility behavior of CO2 and H2S in 1-benzyl-3-methylimidazolium bis (trifluoromethylsulfonyl) imide ionic liquid. J. Chem. Thermodyn. 167, 106721. doi:10.1016/j.jct.2021.106721
Ji, X., Held, C., and Sadowski, G. (2012). Modeling imidazolium-based ionic liquids with ePC-SAFT. Fluid Phase Equilibria 335, 64–73. doi:10.1016/j.fluid.2012.05.029
Ji, X., Held, C., and Sadowski, G. (2014). Modeling imidazolium-based ionic liquids with ePC-SAFT. Part II. Application to H2S and synthesis-gas components. Fluid Phase Equilibria 363, 59–65. doi:10.1016/j.fluid.2013.11.019
Ji, X., and Zhu, C. (2013). A SAFT Equation of State for the H2S-CO2-H2O-NaCl system and applications for CO2-H2S transportation and geological storage. Energy Procedia 37, 3780–3791. doi:10.1016/j.egypro.2013.06.274
Jiang, S., Hu, Y., Wang, Y., and Wang, X. (2019a). Viscosity of typical room-temperature ionic liquids: A critical review. J. Phys. Chem. Reference Data 48, 033101. doi:10.1063/1.5090486
Jiang, Y., Taheri, M., Yu, G., Zhu, J., and Lei, Z. (2019b). Experiments, modeling, and simulation of CO2 dehydration by ionic liquid, triethylene glycol, and their binary mixtures. Ind. Eng. Chem. Res. 58, 15588–15597. doi:10.1021/acs.iecr.9b02540
Jing, G., Qian, Y., Zhou, X., Lv, B., and Zhou, Z. (2018). Designing and screening of multi-amino-functionalized ionic liquid solution for CO2 capture by quantum chemical simulation. ACS Sustain. Chem. Eng. 6, 1182–1191. doi:10.1021/acssuschemeng.7b03467
Keller, A. N., Bentley, C. L., Morales-Collazo, O., and Brennecke, J. F. (2022). Design and characterization of aprotic N-heterocyclic anion ionic liquids for carbon capture. J. Chem. Eng. Data 67, 375–384. doi:10.1021/acs.jced.1c00827
Kerlé, D., Ludwig, R., Geiger, A., and Paschek, D. (2009). Temperature dependence of the solubility of carbon dioxide in imidazolium-based ionic liquids. J. Phys. Chem. B 113, 12727–12735. doi:10.1021/jp9055285
Kilaru, P. K., Condemarin, R. A., and Scovazzo, P. (2008). Correlations of low-pressure carbon dioxide and hydrocarbon solubilities in imidazolium-phosphonium-and ammonium-based room-temperature ionic liquids. Part 1. Using surface tension. Ind. Eng. Chem. Res. 47, 900–909. doi:10.1021/ie070834r
Kim, Y., Choi, W., Jang, J., Yoo, K.-P., and Lee, C. (2005). Solubility measurement and prediction of carbon dioxide in ionic liquids. Fluid Phase Equilibria 228, 439–445. doi:10.1016/j.fluid.2004.09.006
Klamt, A. (1995). Conductor-like screening model for real solvents: A new approach to the quantitative calculation of solvation phenomena. J. Phys. Chem. 99, 2224–2235. doi:10.1021/j100007a062
Klamt, A., Eckert, F., Reinisch, J., and Wichmann, K. (2016). Prediction of cyclohexane-water distribution coefficients with COSMO-RS on the SAMPL5 data set. J. Comput. Aided. Mol. Des. 30, 959–967. doi:10.1007/s10822-016-9927-y
Lee, Y.-Y., Penley, D., Klemm, A., Dean, W., and Gurkan, B. (2021). Deep eutectic solvent formed by imidazolium cyanopyrrolide and ethylene glycol for reactive CO2 separations. ACS Sustain. Chem. Eng. 9, 1090–1098. doi:10.1021/acssuschemeng.0c07217
Lin, H., Gong, K., Hykys, P., Chen, D., Ying, W., Sofer, Z., et al. (2021). Nanoconfined deep eutectic solvent in laminated MXene for efficient CO2 separation. Chem. Eng. J. 405, 126961. doi:10.1016/j.cej.2020.126961
Lin, H., Gong, K., Ying, W., Chen, D., Zhang, J., Yan, Y., et al. (2019a). CO2‐Philic separation membrane: Deep eutectic solvent filled graphene oxide nanoslits. Small 15, 1904145. doi:10.1002/smll.201904145
Lin, W., Cai, Z., Lv, X., Xiao, Q., Chen, K., Li, H., et al. (2019b). Significantly enhanced carbon dioxide capture by anion-functionalized liquid pillar [5] arene through multiple-site interactions. Ind. Eng. Chem. Res. 58, 16894–16900. doi:10.1021/acs.iecr.9b02872
Liu, F., Chen, W., Mi, J., Zhang, J. Y., Kan, X., Zhong, F. Y., et al. (2019). Thermodynamic and molecular insights into the absorption of H2S, CO2, and CH4 in choline chloride plus urea mixtures. AIChE J. 65, e16574. doi:10.1002/aic.16574
Liu, X., Ao, Q., Shi, S., and Li, S. (2022). CO2 capture by alcohol ammonia based deep eutectic solvents with different water content. Mater. Res. Express 9, 015504.
Liu, X., Zhou, G., Zhang, S., and Yao, X. (2009). Molecular dynamics simulation of dual amino-functionalized imidazolium-based ionic liquids. Fluid phase equilibria 284, 44–49. doi:10.1016/j.fluid.2009.06.004
Liu, Y., Dai, Z., Zhang, Z., Zeng, S., Li, F., Zhang, X., et al. (2021). Ionic liquids/deep eutectic solvents for CO2 capture: Reviewing and evaluating. Green Energy & Environ. 6, 314–328. doi:10.1016/j.gee.2020.11.024
Luo, F., Liu, X., Chen, S., Song, Y., Yi, X., Xue, C., et al. (2021). Comprehensive evaluation of a deep eutectic solvent based CO2 capture process through experiment and simulation. ACS Sustain. Chem. Eng. 9, 10250–10265. doi:10.1021/acssuschemeng.1c02722
Luo, X., Guo, Y., Ding, F., Zhao, H., Cui, G., Li, H., et al. (2014). Significant improvements in CO2 capture by pyridine‐containing anion‐functionalized ionic liquids through multiple‐site cooperative interactions. Angew. Chem. Int. Ed. Engl. 126, 7173–7177. doi:10.1002/ange.201400957
Ma, C., Liu, C., Lu, X., and Ji, X. (2018). Techno-economic analysis and performance comparison of aqueous deep eutectic solvent and other physical absorbents for biogas upgrading. Appl. Energy 225, 437–447. doi:10.1016/j.apenergy.2018.04.112
Ma, C., Shukla, S. K., Samikannu, R., Mikkola, J.-P., and Ji, X. (2019). CO2 separation by a series of aqueous morpholinium-based ionic liquids with acetate anions. ACS Sustain. Chem. Eng. 8, 415–426. doi:10.1021/acssuschemeng.9b05686
Ma, C., Wang, N., Ye, N., and Ji, X. (2021). CO2 capture using ionic liquid-based hybrid solvents from experiment to process evaluation. Appl. Energy 304, 117767. doi:10.1016/j.apenergy.2021.117767
Mei, M., Hu, X., Song, Z., Chen, L., Deng, L., Qi, Z., et al. (2022). CO2 capture by 1-ethyl-3-methylimidazolium acetate: Solubility at low pressure and quantification of chemisorption and physisorption. J. Mol. Liq. 348, 118036. doi:10.1016/j.molliq.2021.118036
Mihăilă, E. G., Aruxandei, D. C., Doncea, S., Oancea, F., and Dincă, C. (2021). Deep eutectic solventsfor CO2 capture in post-combustion processes. Romania, Balkans: Studia Universitatis Babes-Bolyai.
Min, Z., Li, Z., Wang, H., Xuan, X., Zhao, Y., Wang, J., et al. (2021). How does the moisture affect CO2 absorption by a glycinate ionic liquid? ACS Sustain. Chem. Eng. 9, 853–862. doi:10.1021/acssuschemeng.0c07578
Mondal, M. K., Balsora, H. K., and Varshney, P. (2012). Progress and trends in CO2 capture/separation technologies: A review. Energy 46, 431–441. doi:10.1016/j.energy.2012.08.006
Moya, C., Hospital-Benito, D., Santiago, R., Lemus, J., and Palomar, J. (2020). Prediction of CO2 chemical absorption isotherms for ionic liquid design by DFT/COSMO-RS calculations. Chem. Eng. J. Adv. 4, 100038. doi:10.1016/j.ceja.2020.100038
Noorani, N., Mehrdad, A., and Ahadzadeh, I. (2021). CO2 absorption in amino acid-based ionic liquids: Experimental and theoretical studies. Fluid Phase Equilibria 547, 113185. doi:10.1016/j.fluid.2021.113185
Noorani, N., and Mehrdad, A. (2021a). CO2 adsorption onto 1-butyl-3-vinylimidazolium based poly (ionic liquid) s: Experimental and theoretical studies. J. Polym. Res. 28, 346. doi:10.1007/s10965-021-02695-8
Noorani, N., and Mehrdad, A. (2021b). Experimental and theoretical study of CO2 sorption in biocompatible and biodegradable cholinium-based ionic liquids. Sep. Purif. Technol. 254, 117609. doi:10.1016/j.seppur.2020.117609
Palomar, J., Gonzalez-Miquel, M., Polo, A., and Rodriguez, F. (2011). Understanding the physical absorption of CO2 in ionic liquids using the COSMO-RS method. Ind. Eng. Chem. Res. 50, 3452–3463. doi:10.1021/ie101572m
Pan, L., Shi, W., Li, B., and Wei, X. (2021). Experimental investigation on the performance of [APMIm] [Tf2N] for capturing CO2 from flue gas of the cement kiln tail. J. Therm. Sci. 30, 1780–1788. doi:10.1007/s11630-021-1504-6
Pellegrini, L. A., Gilardi, M., Giudici, F., and Spatolisano, E. (2021). New solvents for CO2 and H2S removal from gaseous streams. Energies 14, 6687. doi:10.3390/en14206687
Pena, C. A., Soto, A., and Rodríguez, H. (2021). Tetrabutylphosphonium acetate and its eutectic mixtures with common-cation halides as solvents for carbon dioxide capture. Chem. Eng. J. 409, 128191. doi:10.1016/j.cej.2020.128191
Peng, L., Wu, W., Hou, Y., Li, K., and Zhang, H. (2021). Experimental investigation on solubility characteristics of CO2–ionic liquids as new working pairs for absorption refrigeration systems. J. Engin. Thermophys. 30, 330–339. doi:10.1134/s1810232821020144
Perdew, J. P. (1986). Density-functional approximation for the correlation energy of the inhomogeneous electron gas. Phys. Rev. B 33, 8822–8824. doi:10.1103/physrevb.33.8822
Pishro, K. A., Murshid, G., Mjalli, F. S., and Naser, J. (2021). Carbon dioxide solubility in amine-based deep eutectic solvents: Experimental and theoretical investigation. J. Mol. Liq. 325, 115133. doi:10.1016/j.molliq.2020.115133
Qian, W., Hao, J., Zhu, M., Sun, P., Zhang, K., Wang, X., et al. (2022). Development of green solvents for efficient post-combustion CO2 capture with good regeneration performance. J. CO2 Util. 59, 101955. doi:10.1016/j.jcou.2022.101955
Qin, H., Song, Z., Cheng, H., Deng, L., and Qi, Z. (2021). Physical absorption of carbon dioxide in imidazole-PTSA based deep eutectic solvents. J. Mol. Liq. 326, 115292. doi:10.1016/j.molliq.2021.115292
Qu, Y., Lan, J., Chen, Y., and Sun, J. (2021). Amino acid ionic liquids as efficient catalysts for CO2 capture and chemical conversion with epoxides under metal/halogen/cocatalyst/solvent-free conditions. Sustain. Energy Fuels 5, 2494–2503. doi:10.1039/d1se00060h
Rabhi, F., Mutelet, F., and Sifaoui, H. (2020). Solubility of carbon dioxide in carboxylic acid-based deep eutectic solvents. J. Chem. Eng. Data 66, 702–711. doi:10.1021/acs.jced.0c00844
Ramdin, M., De Loos, T. W., and Vlugt, T. J. (2012). State-of-the-art of CO2 capture with ionic liquids. Ind. Eng. Chem. Res. 51, 8149–8177. doi:10.1021/ie3003705
Rashid, T. U. (2021). Ionic liquids: Innovative fluids for sustainable gas separation from industrial waste stream. J. Mol. Liq. 321, 114916. doi:10.1016/j.molliq.2020.114916
Ren, H., Lian, S., Wang, X., Zhang, Y., and Duan, E. (2018). Exploiting the hydrophilic role of natural deep eutectic solvents for greening CO2 capture. J. Clean. Prod. 193, 802–810. doi:10.1016/j.jclepro.2018.05.051
Ren, J., Wu, L., and Li, B.-G. (2012). Preparation and CO2 sorption/desorption of N-(3-aminopropyl) aminoethyl tributylphosphonium amino acid salt ionic liquids supported into porous silica particles. Ind. Eng. Chem. Res. 51, 7901–7909. doi:10.1021/ie2028415
Ruckart, K. N., O’brien, R. A., Woodard, S. M., West, K. N., and Glover, T. G. (2015). Porous solids impregnated with task-specific ionic liquids as composite sorbents. J. Phys. Chem. C 119, 20681–20697. doi:10.1021/acs.jpcc.5b04646
Safarov, J., Abdullayeva, G., Bashirov, M., Tuma, D., and Bashirov, R. (2021). The ionic liquid 1-ethyl-3-methylimidazolium methanesulfonate revisited: Solubility of carbon dioxide over an extended range of temperature and pressure. J. Mol. Liq. 333, 115920. doi:10.1016/j.molliq.2021.115920
Sanz-Perez, E. S., Murdock, C. R., Didas, S. A., and Jones, C. W. (2016). Direct capture of CO2 from ambient air. Chem. Rev. 116, 11840–11876. doi:10.1021/acs.chemrev.6b00173
Sarmad, S., Mikkola, J. P., and Ji, X. (2017a). Carbon dioxide capture with ionic liquids and deep eutectic solvents: A new generation of sorbents. ChemSusChem 10, 324–352. doi:10.1002/cssc.201600987
Sarmad, S., Xie, Y., Mikkola, J.-P., and Ji, X. (2017b). Screening of deep eutectic solvents (DESs) as green CO2 sorbents: From solubility to viscosity. New J. Chem. 41, 290–301. doi:10.1039/c6nj03140d
Schäfer, A., Huber, C., and Ahlrichs, R. (1994). Fully optimized contracted Gaussian basis sets of triple zeta valence quality for atoms Li to Kr. J. Chem. Phys. 100, 5829–5835. doi:10.1063/1.467146
Shah, J. K., and Maginn, E. J. (2005). Monte Carlo simulations of gas solubility in the ionic liquid 1-n-butyl-3-methylimidazolium hexafluorophosphate. J. Phys. Chem. B 109, 10395–10405. doi:10.1021/jp0442089
Shahbaz, M., Alnouss, A., Ghiat, I., Mckay, G., Mackey, H., Elkhalifa, S., et al. (2021). A comprehensive review of biomass based thermochemical conversion technologies integrated with CO2 capture and utilisation within BECCS networks. Resour. Conservation Recycl. 173, 105734. doi:10.1016/j.resconrec.2021.105734
Shama, V. M., Swami, A. R., Aniruddha, R., Sreedhar, I., and Reddy, B. M. (2021). Process and engineering aspects of carbon capture by ionic liquids. J. CO2 Util. 48, 101507. doi:10.1016/j.jcou.2021.101507
Shen, G., Held, C., Lu, X., and Ji, X. (2015). Modeling thermodynamic derivative properties of ionic liquids with ePC-SAFT. Fluid Phase Equilibria 405, 73–82. doi:10.1016/j.fluid.2015.07.018
Shi, W., and Luebke, D. R. (2013). Enhanced gas absorption in the ionic liquid 1-n-hexyl-3-methylimidazolium bis (trifluoromethylsulfonyl) amide ([HMIM] [Tf2N]) confined in silica slit pores: A molecular simulation study. Langmuir 29, 5563–5572. doi:10.1021/la400226g
Sistla, Y. S., and Khanna, A. (2015). CO2 absorption studies in amino acid-anion based ionic liquids. Chem. Eng. J. 273, 268–276. doi:10.1016/j.cej.2014.09.043
Sistla, Y. S., and Khanna, A. (2011). Validation and prediction of the temperature-dependent Henry's constant for CO2–ionic liquid systems using the conductor-like screening model for realistic solvation (COSMO-RS). J. Chem. Eng. Data 56, 4045–4060. doi:10.1021/je200486c
Smith, E. L., Abbott, A. P., and Ryder, K. S. (2014). Deep eutectic solvents (DESs) and their applications. Chem. Rev. 114, 11060–11082. doi:10.1021/cr300162p
Song, Z., Shi, H., Zhang, X., and Zhou, T. (2020). Prediction of CO2 solubility in ionic liquids using machine learning methods. Chem. Eng. Sci. 223, 115752. doi:10.1016/j.ces.2020.115752
Sood, A., Thakur, A., and Ahuja, S. M. (2021). Recent advancements in ionic liquid based carbon capture technologies. Chem. Eng. Commun. 2021, 1–22. doi:10.1080/00986445.2021.1990886
Sumon, K. Z., and Henni, A. (2011). Ionic liquids for CO2 capture using COSMO-RS: Effect of structure, properties and molecular interactions on solubility and selectivity. Fluid Phase Equilibria 310, 39–55. doi:10.1016/j.fluid.2011.06.038
Sun, Y. (2020). Modeling ionic liquids with ePC-SAFT─ properties and gas solubilities. Luleå, Sweden: Luleå University of Technology.
Sun, Y., Schemann, A., Held, C., Lu, X., Shen, G., Ji, X., et al. (2019). Modeling thermodynamic derivative properties and gas solubility of ionic liquids with ePC-SAFT. Ind. Eng. Chem. Res. 58, 8401–8417. doi:10.1021/acs.iecr.9b00254
Suo, X., Yang, Z., Fu, Y., Do‐Thanh, C. L., Maltsev, D., Luo, H., et al. (2022). New‐generation carbon‐capture ionic liquids regulated by metal‐ion coordination. ChemSusChem 15, e202102136. doi:10.1002/cssc.202102136
Taheri, M., Dai, C., and Lei, Z. (2018). CO2 capture by methanol, ionic liquid, and their binary mixtures: Experiments, modeling, and process simulation. AIChE J. 64, 2168–2180. doi:10.1002/aic.16070
Thapaliya, B. P., Puskar, N. G., Slaymaker, S., Feider, N. O., Do-Thanh, C.-L., Schott, J. A., et al. (2021). Synthesis and characterization of macrocyclic ionic liquids for CO2 separation. Ind. Eng. Chem. Res. 60, 8218–8226. doi:10.1021/acs.iecr.1c00673
Uma Maheswari, A., and Palanivelu, K. (2015). Carbon dioxide capture and utilization by alkanolamines in deep eutectic solvent medium. Ind. Eng. Chem. Res. 54, 11383–11392. doi:10.1021/acs.iecr.5b01818
Vicent-Luna, J. M., Gutiérrez-Sevillano, J. J., Anta, J. A., and Calero, S. (2013). Effect of room-temperature ionic liquids on CO2 separation by a Cu-BTC metal–organic framework. J. Phys. Chem. C 117, 20762–20768. doi:10.1021/jp407176j
Wang, C., Luo, H., Luo, X., Li, H., and Dai, S. (2010a). Equimolar CO2 capture by imidazolium-based ionic liquids and superbase systems. Green Chem. 12, 2019. doi:10.1039/c0gc00070a
Wang, C., Luo, X., Luo, H., Jiang, D. E., Li, H., Dai, S., et al. (2011). Tuning the basicity of ionic liquids for equimolar CO2 capture. Angew. Chem. Int. Ed. Engl. 50, 5020–5024. doi:10.1002/ange.201008151
Wang, C., Mahurin, S. M., Luo, H., Baker, G. A., Li, H., Dai, S., et al. (2010b). Reversible and robust CO2 capture by equimolar task-specific ionic liquid–superbase mixtures. Green Chem. 12, 870. doi:10.1039/b927514b
Wang, L., Xie, H., Huang, X., Xu, Y., Chu, T., Wu, Z., et al. (2020). CO2 and CH4 sorption by solid-state ammonium and imidazolium ionic liquids. Energy fuels. 35, 599–609. doi:10.1021/acs.energyfuels.0c02849
Wang, X., and Song, C. (2020). Carbon capture from flue gas and the atmosphere: A perspective. Front. Energy Res. 265. doi:10.3389/fenrg.2020.560849
Wang, X., Wu, C., and Yang, D. (2021a). CO2 absorption mechanism by diamino protic ionic liquids (DPILs) containing azolide anions. Processes 9, 1023. doi:10.3390/pr9061023
Wang, Y., Ren, S., Hou, Y., and Wu, W. (2021b). Capture of acidic gases from flue gas by deep eutectic solvents. Processes 9, 1268. doi:10.3390/pr9081268
Wang, Z., Wang, Z., Chen, J., Wu, C., and Yang, D. (2021c). The influence of hydrogen bond donors on the CO2 absorption mechanism by the bio-phenol-based deep eutectic solvents. Molecules 26, 7167. doi:10.3390/molecules26237167
Wang, Z., Wang, Z., Huang, X., Yang, D., Wu, C., Chen, J., et al. (2022a). Deep eutectic solvents composed of bio-phenol-derived superbase ionic liquids and ethylene glycol for CO2 capture. Chem. Commun. 58, 2160–2163. doi:10.1039/d1cc06856c
Wang, Z., Wang, Z., Xin, H., Yang, D., Wu, C., Chen, J., et al. (2022b). Deep eutectic solvents composed of bio-phenol-derived superbase ionic liquids and ethylene glycol for CO2 capture. Chem. Commun. 58, 2160–2163. doi:10.1039/d1cc06856c
Wibowo, H., Susanto, H., Grisdanurak, N., Hantoko, D., Yoshikawa, K., Qun, H., et al. (2021). Recent developments of deep eutectic solvent as absorbent for CO2 removal from syngas produced from gasification: Current status, challenges, and further research. J. Environ. Chem. Eng. 9, 105439. doi:10.1016/j.jece.2021.105439
Wu, J., Lv, B., Wu, X., Zhou, Z., and Jing, G. (2019). Aprotic heterocyclic anion-based dual-functionalized ionic liquid solutions for efficient CO2 uptake: Quantum chemistry calculation and experimental research. ACS Sustain. Chem. Eng. 7, 7312–7323. doi:10.1021/acssuschemeng.9b00420
Wu, W., Wang, L., Li, X., Liu, H., Zhang, H., Dou, B., et al. (2021). Phase equilibrium characteristics of CO2 and ionic liquids with [FAP]− anion used for absorption-compression refrigeration working pairs. J. Therm. Sci. 30, 165–176. doi:10.1007/s11630-020-1407-y
Xiong, W., Shi, M., Peng, L., Zhang, X., Hu, X., Wu, Y., et al. (2021). Low viscosity superbase protic ionic liquids for the highly efficient simultaneous removal of H2S and CO2 from CH4. Sep. Purif. Technol. 263, 118417. doi:10.1016/j.seppur.2021.118417
Xue, W., Li, Z., Huang, H., Yang, Q., Liu, D., Xu, Q., et al. (2016). Effects of ionic liquid dispersion in metal-organic frameworks and covalent organic frameworks on CO2 capture: A computational study. Chem. Eng. Sci. 140, 1–9. doi:10.1016/j.ces.2015.10.003
Xue, Z., Zhang, Z., Han, J., Chen, Y., and Mu, T. (2011). Carbon dioxide capture by a dual amino ionic liquid with amino-functionalized imidazolium cation and taurine anion. Int. J. Greenh. Gas Control 5, 628–633. doi:10.1016/j.ijggc.2011.05.014
Yan, M., Huan, Q., Zhang, Y., Fang, W., Chen, F., Pariatamby, A., et al. (2022). Effect of operating parameters on CO2 capture from biogas with choline chloride—Monoethanolamine deep eutectic solvent and its aqueous solution. Biomass Convers. biorefin. 2022, 1–15. doi:10.1007/s13399-021-02246-7
Yim, J.-H., Seo, W.-W., and Lim, J. S. (2021). CO2 solubility in bis (trifluoromethylsulfonyl) imide ([Tf2N]) anion-based ionic liquids:[BVIM][Tf2N], [P4441][tf2N], and [N4222][tf2N]. J. Chem. Eng. Data 67, 3–13. doi:10.1021/acs.jced.1c00433
Yu, G., Wei, Z., Chen, K., Guo, R., and Lei, Z. (2022). Predictive molecular thermodynamic models for ionic liquids. AIChE J. 68, e17575. doi:10.1002/aic.17575
Yu, Y., Mai, J., Huang, L., Wang, L., and Li, X. (2014). Ship in a bottle synthesis of ionic liquids in NaY supercages for CO2 capture. RSC Adv. 4, 12756. doi:10.1039/c3ra46971a
Zailani, N. H. Z. O., Yunus, N. M., Ab Rahim, A. H., and Bustam, M. A. (2022). Experimental investigation on thermophysical properties of ammonium-based protic ionic liquids and their potential ability towards CO2 capture. Molecules 27, 851. doi:10.3390/molecules27030851
Zema, Z. A., Chen, T., Shu, H., and Xu, Y. (2021). Tuning the CO2 absorption and physicochemical properties of K+ chelated dual functional ionic liquids by changing the structure of primary alkanolamine ligands. J. Mol. Liq. 344, 117983. doi:10.1016/j.molliq.2021.117983
Zeng, S., Wang, J., Bai, L., Wang, B., Gao, H., Shang, D., et al. (2015). Highly selective capture of CO2 by ether-functionalized pyridinium ionic liquids with low viscosity. Energy fuels. 29, 6039–6048. doi:10.1021/acs.energyfuels.5b01274
Zeng, S., Zhang, X., Bai, L., Zhang, X., Wang, H., Wang, J., et al. (2017). Ionic-liquid-based CO2 capture systems: Structure, interaction and process. Chem. Rev. 117, 9625–9673. doi:10.1021/acs.chemrev.7b00072
Zhang, J., Jia, C., Dong, H., Wang, J., Zhang, X., Zhang, S., et al. (2013). A novel dual amino-functionalized cation-tethered ionic liquid for CO2 capture. Ind. Eng. Chem. Res. 52, 5835–5841. doi:10.1021/ie4001629
Zhang, J., Zhang, S., Dong, K., Zhang, Y., Shen, Y., Lv, X., et al. (2006). Supported absorption of CO2 by tetrabutylphosphonium amino acid ionic liquids. Chem. Eur. J. 12, 4021–4026. doi:10.1002/chem.200501015
Zhang, N., Huang, Z., Zhang, H., Ma, J., Jiang, B., Zhang, L., et al. (2019). Highly efficient and reversible CO2 capture by task-specific deep eutectic solvents. Ind. Eng. Chem. Res. 58, 13321–13329. doi:10.1021/acs.iecr.9b02041
Zhang, Q., Vigier, K. D. O., Royer, S., and Jérôme, F. (2012). Deep eutectic solvents: Syntheses, properties and applications. Chem. Soc. Rev. 41, 7108. doi:10.1039/c2cs35178a
Zhang, S., Zhang, J., Zhang, Y., and Deng, Y. (2017). Nanoconfined ionic liquids. Chem. Rev. 117, 6755–6833. doi:10.1021/acs.chemrev.6b00509
Zhang, X., Ding, X., Song, Z., Zhou, T., and Sundmacher, K. (2021a). Integrated ionic liquid and rate‐based absorption process design for gas separation: Global optimization using hybrid models. AIChE J. 67, e17340. doi:10.1002/aic.17340
Zhang, X., Wang, J., Song, Z., and Zhou, T. (2021b). Data-driven ionic liquid design for CO2 capture: Molecular structure optimization and DFT verification. Ind. Eng. Chem. Res. 60, 9992–10000. doi:10.1021/acs.iecr.1c01384
Zhang, Y., Ji, X., Xie, Y., and Lu, X. (2016). Screening of conventional ionic liquids for carbon dioxide capture and separation. Appl. Energy 162, 1160–1170. doi:10.1016/j.apenergy.2015.03.071
Zhao, Y., Zhang, X., Zhen, Y., Dong, H., Zhao, G., Zeng, S., et al. (2011). Novel alcamines ionic liquids based solvents: Preparation, characterization and applications in carbon dioxide capture. Int. J. Greenh. Gas Control 5, 367–373. doi:10.1016/j.ijggc.2010.09.010
Zhou, T., Chen, L., Ye, Y., Chen, L., Qi, Z., Freund, H. R., et al. (2012). An overview of mutual solubility of ionic liquids and water predicted by COSMO-RS. Ind. Eng. Chem. Res. 51, 6256–6264. doi:10.1021/ie202719z
Keywords: ionic liquid, deep eutectic solvents, CO2 capture, COSMO-RS, green absorbents
Citation: Foorginezhad S, Yu G and Ji X (2022) Reviewing and screening ionic liquids and deep eutectic solvents for effective CO2 capture. Front. Chem. 10:951951. doi: 10.3389/fchem.2022.951951
Received: 24 May 2022; Accepted: 05 July 2022;
Published: 10 August 2022.
Edited by:
Tiancheng Mu, Renmin University of China, ChinaReviewed by:
Zhen Song, East China University of Science and Technology, ChinaJotheeswari Kothandaraman, Pacific Northwest National Laboratory (DOE), United States
Dezhong Yang, China University of Geosciences, China
Teng Zhou, The Hong Kong University of Science and Technology (Guangzhou), China
Copyright © 2022 Foorginezhad, Yu and Ji. This is an open-access article distributed under the terms of the Creative Commons Attribution License (CC BY). The use, distribution or reproduction in other forums is permitted, provided the original author(s) and the copyright owner(s) are credited and that the original publication in this journal is cited, in accordance with accepted academic practice. No use, distribution or reproduction is permitted which does not comply with these terms.
*Correspondence: Gangqiang Yu, eXVncUBianV0LmVkdS5jbg==; Xiaoyan Ji, WGlhb3lhbi5qaUBsdHUuc2U=