- State Grid Jiangsu Electric Power Co, Ltd. Research Institute, Nanjing, Jiangsu, China
Hydrogen energy is an excellent carrier for connecting various renewable energy sources and has many advantages. However, hydrogen is flammable and explosive, and its density is low and easy to escape, which brings inconvenience to the storage and transportation of hydrogen. Therefore, hydrogen storage technology has become one of the key steps in the application of hydrogen energy. Solid-state hydrogen storage method has a very high volumetric hydrogen density compared to the traditional compressed hydrogen method. The main issue of solid-state hydrogen storage method is the development of advanced hydrogen storage materials. Metal borohydrides have very high hydrogen density and have received much attention over the past two decades. However, high hydrogen sorption temperature, slow kinetics, and poor reversibility still severely restrict its practical applications. This paper mainly discusses the research progress and problems to be solved of metal borohydride hydrogen storage materials for solid-state hydrogen storage.
Introduction
Nowadays, with the shortage of fossil fuel energy and the increasingly serious environmental problems, people gradually realize the importance of finding new, efficient, environment-friendly and sustainable energy sources. In the global low-carbon energy system, hydrogen energy, as an efficient and clean new energy source, was initially used in hydrogen fuel cell vehicles fields, which then stimulated and promoted the development of hydrogen energy-related fields. The use of hydrogen energy generally includes three steps. The first is to use clean primary energy to produce hydrogen, then to store and transport the hydrogen, and finally to use the hydrogen for energy output equipment. Among them, hydrogen storage technology has become the key to the application and development of hydrogen energy due to the flammable, explosive features and low volumetric energy density of hydrogen. At present, there are three main ways of hydrogen storage: gaseous hydrogen storage, liquid hydrogen storage and solid hydrogen storage. Among them, solid-state hydrogen storage is a technology that stores hydrogen in solid hydride materials. As for the solid-state hydrogen storage mechanism, physical storage and chemical storage can be considered. The physical one is a method in which hydrogen is combined with the material in a molecular state, and hydrogen molecules are adsorbed on the surface of the material, while for the chemical one, hydrogen storage is based on a chemical absorption mechanism. In this chemical hydrogen storage material, hydrogen is combined with various elements or compounds by metal bonds, ionic bonds, or covalent bonds to form metal hydrides, coordination hydrides or chemical hydrides to achieve solid-state storage. High-capacity hydrogen storage materials composed of light elements include light metal hydrides (MgH2, AlH3), metal alanates [LiAlH4, NaAlH4, Mg(AlH4)2, etc.] metal borohydrides [LiBH4, NaBH4, Mg(BH4)2, etc.], metal nitrides [LiNH2, Mg(NH2)2, etc.], etc. (Schlapbach and Zuttel, 2001; Chen et al., 2002; Orimo et al., 2007; Jiang et al., 2021; Liu et al., 2021; Lu et al., 2021; Lin et al., 2022). Among these, the metal borohydrides (Figure 1) have a theoretical hydrogen storage capacity of more than 7.5 wt%, and have become a hot topic in the field of solid-state hydrogen storage research (Lv and Wu, 2021; Zhang et al., 2021; Li et al., 2022a; Wang et al., 2022a; Li et al., 2022b; Zhang et al., 2022).
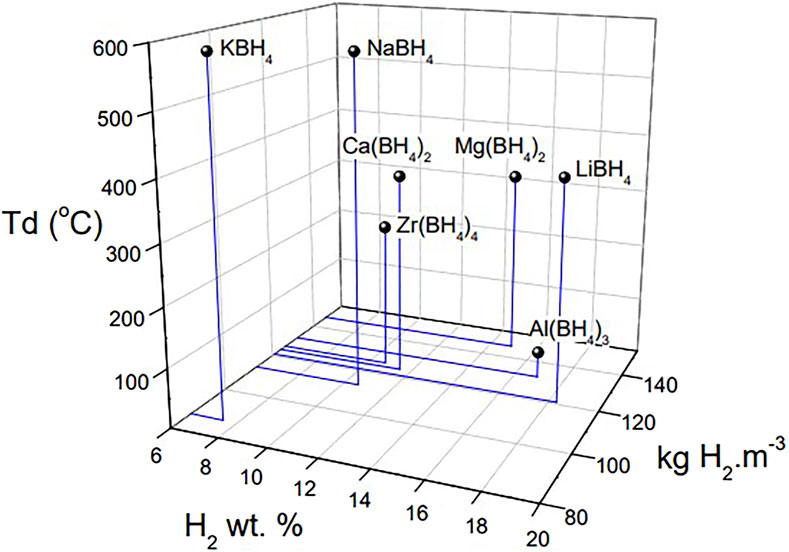
FIGURE 1. Hydrogen storage gravimetric capacity (wt.%), volume capacity (kg H2·m−3) and decomposition temperature of alkali, alkaline earth, Zr and Al borohydrides (Puszkiel et al., 2020).
However, metal borohydrides have high thermal stability, and generates highly inert elemental boron after hydrogen releasing, which affects its reverse reaction to absorb hydrogen again. Therefore, improving the reversible hydrogen storage properties of metal borohydrides has become one of the hotspots for solid-state hydrogen storage materials. This paper mainly discusses the modification methods, research progress and problems to be solved of metal borohydride hydrogen storage materials.
LiBH4
LiBH4 is a white powder at room temperature with a melting point of about 278°C. It is insoluble in hydrocarbons, but soluble in ether, tetrahydrofuran, and liquid nitrogen. LiBH4 is stable at room temperature and in dry air, but it is very sensitive to moisture and protic solvents. The oxidation of LiBH4 with water follows Eq. 1 (Xiong et al., 2017).
LiBH4 has an orthorhombic structure at room temperature with a space group of Pnma (a = 7.17858 Å, b = 4.43686 Å, c = 6.80321 Å), and transforms to hexagonal system (P63mc, a = 4.27631 Å, c = 6.94844 Å) at 108−112°C (Harris and Meibohm, 2002; Soulié et al., 2002; Orimo et al., 2007; Yu and Ross, 2011). When the temperature rises to 268−286°C, LiBH4 begins to melt and become liquid accompanied by the liberations of 2% of the hydrogen in compound. When further heated to 380°C, LiBH4 begins to release a large amount of hydrogen. When the temperature reaches 600°C, LiBH4 can practically release a total of 9 wt% hydrogen (Zuttel et al., 2003a; Zuttel et al., 2003b). The hydrogen release reaction formula is as Eqs 2, 3:
The theoretical hydrogen storage capacity of LiBH4 is 18.5 wt%, which is higher than all hydrogen storage alloys and general coordination hydrides. However, the hydrogen contained in LiBH4 is not completely available, and only 13.8 wt% hydrogen is released in the range of 380°C−680°C under one bar of H2 pressure (Stasinevich and Egorenko, 1968; Umegaki et al., 2009). In the process of hydrogen absorption, the reversible hydrogen absorption reaction of LiH and B can be completed at 600°C and 35 MPa for 12 h (Orimo et al., 2002).
Although pure LiBH4 is a high-capacity hydrogen storage material, it has high hydrogen absorption and desorption temperature, slow hydrogen releasing rate, and poor reversibility. There are two common methods for modifying LiBH4. The first method is to thermodynamically destabilize LiBH4 by adding metals, metal halides, oxides, amides or metal hydrides to form composite materials or alloys after dehydrogenation (Zhang and Tian, 2011; Zhou et al., 2012; Liu et al., 2016; Zhou et al., 2017; Cheng et al., 2018; Xian et al., 2019; Ding et al., 2020; Sulaiman et al., 2021); the second method kinetically improve LiBH4 by using catalysts (Sulaiman et al., 2021; Zheng et al., 2021; Li et al., 2022a; Wang et al., 2022a; Li et al., 2022b) or nanoconfinement, confining LiBH4 in mesoporous scaffolds or mixing LiBH4 with nanotubes or mesoporous gels etc. (Zhou et al., 2019; Wang et al., 2020a; Le et al., 2021; Xian et al., 2021; Ye et al., 2021; Wang et al., 2022b).
Thermodynamic destabilization
The first point that should be considered to enhance LiBH4 is thermodynamic destabilization. In 2006, Barkhordatian found that the 2LiBH4–MgH2 system has better hydrogen cycle thermodynamics than LiBH4 or MgH2 alone, which is believed to be because the formation of MgB2 “destroys” the decomposition of LiBH4 (Pinkerton et al., 2007).
Vajo et al. (2005) reported on the destabilization system of 2LiBH4–MgH2 and found that adding a destabilizer to LiBH4 to participate in hydrogen evolution can effectively reduce the reaction enthalpy change of LiBH4, and the enthalpy change of hydrogen evolution reaction was reduced to 46 kJ mol−1 H2 (Figure 2). Since then, researchers have carried out a lot of work around the destabilization of LiBH4, and have tried various destabilizing agents to improve the dehydrogenation performance and reversibility of LiBH4, including metal elements, metal hydrides, metal chlorides and metal oxides, etc. Among the destabilization systems, the LiBH4–MgH2 system, that is, the Li-RHC system (RHC, Reactive Hydride Complex), is highly studied. Barkohrdarian et al. patented the RHC concept in 2006. In the first published work on Li-RHC, the hydride mixture was doped with 2–3 mol% TiCl3 to improve the kinetic behavior, and isothermal measurements were performed in the range of 315°C–400°C, the enthalpy of hydrogen absorption down to 40.5 kJ mol−1. But the entropy of the Li-RHC system is different from that of the metal-hydrogen system, which is related to the [BH4]– cluster configuration after hydrogen interaction (Vajo et al., 2005).
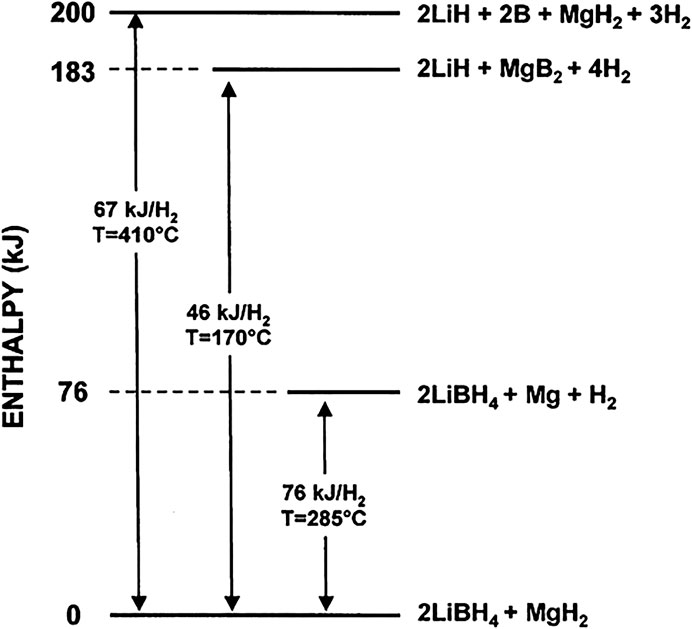
FIGURE 2. Enthalpy change of LiBH4-MgH2 after destabilization (Vajo et al., 2007).
Bosenberg et al. (2010) published a paper, which for the first time expounded the overall hydrogenation and dehydrogenation reaction mechanism of Li-RHC under dynamic conditions. The following two-step reaction (Eq. 4) was observed during hydrogen evolution:
In the first step, MgH2 is desorbed and Mg is formed. After that, LiBH4 desorb and LiH and MgB2 are formed, and they found that MgB2–LiH composites could start to absorb hydrogen at 50 bar and 250°C, and have a much lower decomposition temperature compared to pure LiBH4 (Bosenberg et al., 2007). The researchers further found that the formation of B at low hydrogen pressure during dehydrogenation prevented the rehydrogenation of Li-RHC. Barkhordarian et al. (2007), Bosenberg et al. (2007) proposed that the kinetic barriers for the formation of LiBH4, NaBH4 and Ca(BH4)2 were significantly reduced when B was replaced by MgB2, and the kinetics of these borohydrides were enhanced. The higher reactivity of B in MgB2 facilitate the formation of the [BH4]− complex. In addition, after replacing elemental B with MgB2, the reaction enthalpy decreased by about 10 W mol−1 H2.
The 2LiBH4–MgH2 system has different hydrogen evolution reaction paths under different hydrogen back pressures and temperatures. Only under suitable conditions (e.g., 350°C, 5.5 bar), LiBH4 will destabilize with MgH2 to form MgB2 and release hydrogen; otherwise, the dehydrogenation process of the system is the respective decomposition reactions of LiBH4 and MgH2 (Mao et al., 2013; Kim et al., 2015; Shao et al., 2015).
Nakagawa et al. (2007) found the effect of hydrogen back pressure on the dehydrogenation of Li-RHC, and studied the formation of MgB2 when dehydrogenation back pressure and inert gas were applied at 450°C, and found that only under hydrogen back pressure will MgB2 be formed, and the formation of MgB2 is considered to be a sign of a reversible reaction and is the key to the re-formation of LiBH4 in the reverse reaction. When heated at a back pressure below 3 bar, the LiBH4–MgH2 reaction product hardly has MgB2, but when the back pressure rises to 5 MPa, the product tends to be MgB2 (Shaw et al., 2010; Gosalawit-Utke et al., 2011; Zhou et al., 2012).
In addition to LiH–MgB2 composites, Li–Al–B–H is also a promising Li-RHC (Yang et al., 2007; Zhang et al., 2009; Ravnsbaek and Jensen, 2012; Ye et al., 2021). Liu et al. studied LiBH4–AlH3 composites and found that AlH3 can destabilize LiBH4. The LiBH4–AlH3 composite releases about 11.0 wt% hydrogen at 450°C for 6 h, and the kinetic performance is much better than that of pure LiBH4. In addition, AlH3 also improves the reversibility of LiBH4 in LiBH4–AlH3 composites, and the decomposition kinetics of LiBH4 is enhanced with the increase of AlH3 content. The 2LiBH4 + AlH3 composite can release 82% of the hydrogen capacity of LiBH4 within 29 min at 450°C (Liu et al., 2016; Liu et al., 2017).
Another destabilizing system that has received much attention is the Li–B–N–H composite system. The H atom in the [BH4]− group in LiBH4 tends to gain electrons and is negatively charged (Hδ−), and the H atom in NH3 or LiNH2 tends to lose electrons and is positively discharged (Hδ+). Hδ− and Hδ+ in the composite system will interact to generate hydrogen bonds, and the samples will interact during heating. It is easier to release hydrogen at low temperature than pristine LiBH4.
Johnson et al. (2009) found that at normal temperature and pressure, LiBH4 can react with NH3 to form LiBH4·NH3. When the temperature rises to about 40°C, NH3 will be released from LiBH4·NH3 and become pure LiBH4 instead of hydrogen. Pinkerton et al. (2005) studied the LiBH4–LiNH2 composite as hydrogen storage material for the first time. When LiBH4 and LiNH2 were subjected to high-energy ball milling at a molar ratio of 1:2 or heated to above 95°C, the mixture would in situ generate a new type of hydrogen storage material Li3BN2H8. The hydrogen storage capacity of the system is as high as 11.9 wt%, and the melting point is 190°C. The hydrogen releasing amount is 10 wt% at 250–350°C.
In a word, thermodynamic destabilization is an efficient method to tailor the hydrogen storage performances of LiBH4.
Kinetic improvement
Kinetics is another point that should be considered to improve the hydrogen storage properties of LiBH4. Catalysis and nanoconfinements are two common methods to improve the kinetics of LiBH4.
It was found that the effects of many metal elements, metal oxides and halides on LiBH4 were both destabilizing and catalysis. Fang et al. (2008) found that the Ni/Co/Fe borides produced by the reaction between the metal element and LiBH4 can catalyze the reaction. A composite of LiBH4 and single-wall nanotubes (SWNT) can release 11.4 wt% of hydrogen within 50 min at 450°C after ball milling. Wang et al. (Kang et al., 2013; Wang et al., 2013; Wang et al., 2014a; Wang et al., 2014b; Wang et al., 2014c) studied the effect of TiF3 and Nb2O5 doping on the LiBH4–MgH2 system by using a three-step preparation method of mixture pre-grinding, isothermal treatment and co-grinding with MgH2. The study found that NbB2 and TiB2 formed during hydrogen desorption can effectively improve the performances of LiBH4–MgH2 system. The reason for improving the cycling stability of the LiBH4–MgH2 system is that the reaction products of TiF3 and Nb2O5 with LiBH4–MgH2 are nucleating agents, which can promote the formation of MgB2, thereby promoting the cycling stability.
Cai et al. (2014) studied the catalytic effect of different forms of nanostructured CoB on the hydrogen absorption and desorption process of LiBH4, and found that the catalytic effect of CoB is roughly in the order of mulberry-like > bayberry-like > chain-like > sheet-like > rod-like, and it is proportional to the specific surface area. At 200°C, the mulberry-like and bayberry-like CoB catalyzed LiBH4 have the best hydrogen evolution properties, and the hydrogen releasing amounts of the two CoB-catalyzed LiBH4 were 4.6 wt% and 4.8 wt%, respectively. The mulberry-like CoB exhibited the best catalytic effect at 350°C, which obtained 10.4 wt% hydrogen from LiBH4 with almost complete reversibility at 400°C and 10 MPa. Moreover, at the fourth cycle 9.6% of hydrogen can still be released.
Among many metal halides, TiCl3 and TiF3 have the most significant catalytic effects and have been widely studied. Adding TiCl3 or TiF3 can reduce the initial dehydrogenation temperature of LiBH4 to about 100°C, and TiF3 has a more significant catalytic effect. Ti halides react with LiBH4 to form Ti hydrides and Li halides when heated, and the in-situ formation of Ti hydrides can effectively improve the cyclic dehydrogenation performance of LiBH4. Ming et al. (2008) studied the LiBH4 + 0.2MgCl2 + 0.1TiCl3 composite catalytic system, the material can desorb 5 wt% of hydrogen at 400°C, and can absorb 4.5 wt% of hydrogen at 600°C and 7 MPa after dehydrogenation.
Zhang et al. (2017) (Li et al., 2017) studied the effect of Co(OH)2, Co3O4 and CoO on the catalytic performance of LiBH4–2LiNH2. It was found that the three catalysts were in situ reduced to form metallic Co element during the heating process, and then catalyzed the molten LiBH4–2LiNH2 sample. The hydrogen desorption temperature was effectively lowered. The initial dehydrogenation temperature of the LiBH4–2LiNH2–0.05Co(OH)2 sample was reduced to 70°C, which is the lowest dehydrogenation temperature reported for this system so far.
Nanoconfinement is another method to improve the kinetics of LiBH4, which is to fill the material into the nanopores, and use the interaction between the material and the nanopores to promote the reaction or limit the phase separation during sorption process. Based on this, the confinement frame material must have a high specific surface area and porosity in order to improve the loading rate and avoid the collapse of the porous material during the hydrogen absorption and desorption cycle. At the same time, the material needs to have good chemical inertness to avoid reaction with the hydrogen storage material. Materials suitable for nanoconfinement include carbon-based materials (carbon aerogel/activated carbon/ordered mesoporous carbon), metal-organic frameworks (MOFs), and mesoporous silica (SBA-15), etc. The traditional method of grain refinement, such as high-energy ball milling, gradually refines the grain through the collision between the ball and the tank. But such method is still prone to lead to the agglomeration of nanoparticles and re-agglomeration into larger particles. Different from high-energy ball milling, the method of nano-confinement is to confine the hydride particles in the pores of the frame material, which can obtain finer particles than the ball milling method, and the particles does not agglomerate during the hydrogen absorption and desorption cycle, which increases the cycling stability. At the same time, it also shortens the distance of hydrogen diffusion, and increases the number of grain boundaries, which is conducive to the progress of hydrogen absorption and desorption reactions. The schematic diagram of preparing nanostructured metal coordination hydrides or metal hydrides by ball milling, solution impregnation and melt injection methods is shown in Figure 3.
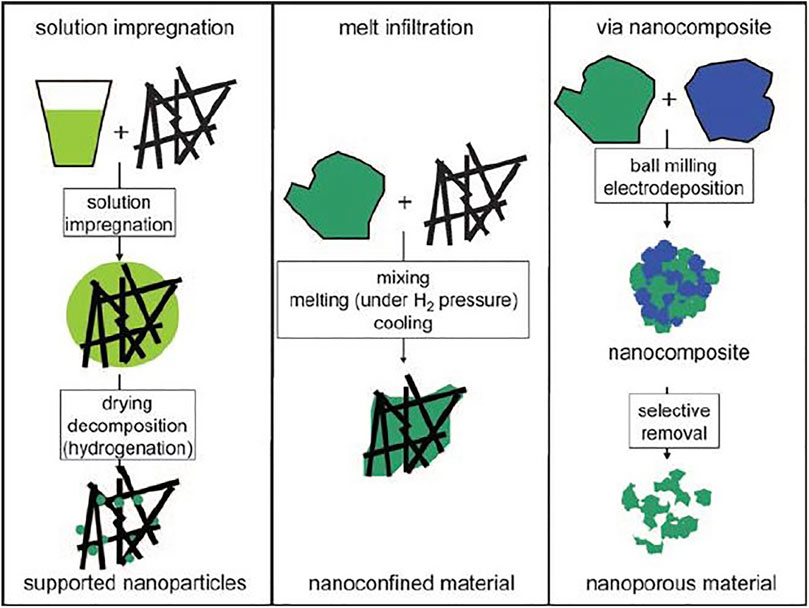
FIGURE 3. Schematic illustration of different ways to prepare nanostructured metal coordination hydrides or metal hydrides (Jongh and Adelhelm, 2010).
Liu et al. (2011) studied the confinement of LiBH4 in porous carbon with a pore size of more than 2 nm, which can effectively reduce the initial hydrogen desorption temperature of LiBH4 to 220°C. They further found that confining LiBH4 in highly ordered nanoporous carbon will lead to the disappearance of the diffraction peaks for phase transition and melting of LiBH4.
Xia et al. (2017) confined LiH in graphene, and then reacted LiH with B2H6 to generate nanoconfined LiBH4, as shown in Figure 4. The obtained material can dehydrogenate about 7.6 wt% at 280°C, and dehydrogenate about 9.7 wt.% within 60 min at 340°C. After absorbing hydrogen at 320°C and 100 bar, it still has 7.5 wt% of capacity after five cycles of hydrogen absorption/desorption.
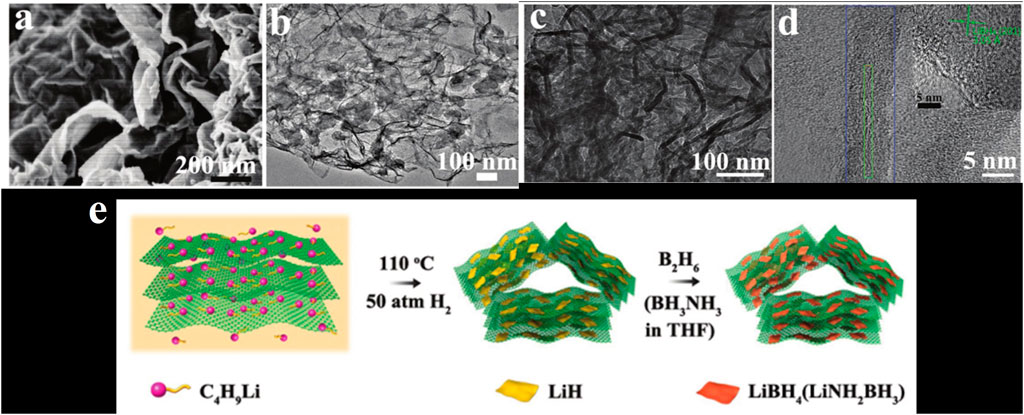
FIGURE 4. (A) SEM image of LiBH4@G. (B–D) TEM image of LiBH4@G. (E) Flow chart of preparation of LiH@G (Xia et al., 2017).
Gross et al. (2008) confined LiBH4 into the pores of carbon gels, and at 300°C, the nanostructured hydrides formed by filling LiBH4 in the bulk of porous carbon aerogels. The measured hydrogen evolution rate was higher than that of bulk materials. The rate of hydrogen evolution is 50 times faster than the bulk materials. At the same time, it was found that compared with the bulk LiBH4, the hydrogen desorption activation energy of the nanoconfined LiBH4 was reduced from 146 kJ mol−1 to 103 kJ mol−1, and the hydrogen desorption temperature was reduced by 75°C. The nanostructured LiBH4 also had a better hydrogen absorption and desorption cycle performance.
Jensen (Gosalawit-Utke et al., 2011; Gosalawitutke et al., 2012) et al. melted 2LiBH4–MgH2 into carbon aerogel scaffolds and found that the performance of 2LiBH4–MgH2 has been significantly improved, and 90% of the hydrogen can be released within 90 min. The non-confined material can only release 34% of the hydrogen. Through further research, the research group melted and infiltrated 2LiBH4–MgH2–0.13TiCl4 in the carbon aerogel scaffold, and then tested the hydrogen absorption and desorption performance. It was found that TiCl4 and LiBH4 were successfully nanoconfined in carbon aerogel scaffolds, while MgH2 was only partially confined. Heated at 25°C–500°C for 5 h, the nano-confined 2LiBH4–MgH2–0.13TiCl4 released up to 99% of the theoretical hydrogen storage capacity, while the nano-confined 2LiBH4–MgH2 was only 94%. At the same time, the kinetics are also greatly improved. To desorb 3.6 wt% H2 during the first dehydrogenation, the nanoconfined 2LiBH4–MgH2–0.13TiCl4 takes 1.5 h, while the nanoconfined 2LiBH4–MgH2 needs 3.5 h. Moreover, the TiCl4 doped material possesses a dehydrogenation rate twice of the undoped material. This indicates that combination of catalysis and nanoconfinement will lead to the further improvement of the LiBH4-based hydrogen storage material.
Verkuijlen et al. (2012) combined the nanoconfinement of LiBH4 in nanoporous carbon with the addition of nickel. Nickel nanoparticles of 5–6 nm were deposited in porous carbon and then melt infiltrated with LiBH4. The addition of nickel has only a slight effect on the hydrogen desorption of LiBH4, but significantly improves the cycling performance of LiBH4 under mild conditions.
Although the nanoconfinement method can effectively improve the thermodynamic and kinetic properties of hydrogen storage materials, there are still many key issues to be solved, such as how to confine a large number of hydrogen storage materials into nanopores and how to achieve a high filling efficiency.
To briefly summary, LiBH4 possesses a very high hydrogen capacity but suffers from high thermal stability and poor reversibility. Catalized LiBH4-based composite such as LiBH4–MgH2 composite with proper catalysts addition can reversibly absorb and desorb hydrogen with high capacity and favored kinetics.
NaBH4
NaBH4 is a common chemical reducing agent in the laboratory, with high thermal stability, and requires a decomposition temperature of 300°C in dry air. The theoretical hydrogen content of NaBH4 is 10.7 wt%, and the volumetric hydrogen storage density is 115 g L−1. The hydrogen desorption temperature of pure NaBH4 is relatively high and needs to be heated to 565°C. The hydrogen desorption reaction of NaBH4 is as Eq. 4. NaBH4 has a cubic structure at room temperature, which is the same as the crystal structure of NaCl (Urgnani et al., 2008; Liu and Li, 2009; Garroni et al., 2010; Martelli et al., 2010).
At present, the methods to improve the performance of NaBH4 include anion and cation substitution method, destabilization method, catalysis, and particle size nanometerization. It was found that adding MgH2 or YF3 can effectively improve the thermodynamic properties of NaBH4. The YB6 and MgB2 formed during the hydrogen evolution process are more stable than the metal elements Y and B, which are the key to the reversible release of NaBH4 (Garroni et al., 2009).
Ngene et al. (2011) used the liquid melting method to obtain nano-NaBH4 to achieve reversible hydrogen absorption and desorption performances. The initial hydrogen desorption temperature of nano-sized NaBH4 was reduced from 470°C of the matrix to below 250°C, and the reactant after dehydrogenation can be re-hydrogenated to NaBH4 under the condition of 60 bar H2 and 325°C. However, since the pores of this method are open, Na is volatile during the hydrogen evolution process, and only 43% of the hydrogen is stored during the cycle. Milanese et al. (2011) (Christian and Aguey-Zinsou, 2012) limited the size of NaBH4 particles to a few nanometers (<30 nm) by anti-solvent precipitation, which lowered its melting point and lead to hydrogen desorption beginning at 400°C. These nanoparticles can form a core after reacting with nickel chloride on their surface, which can achieve effective nanoconfinement for melting NaBH4 cores and their dehydrogenation products (Figure 5). Moreover, the reversibility and fast kinetics due to short diffusion lengths is also obtained.
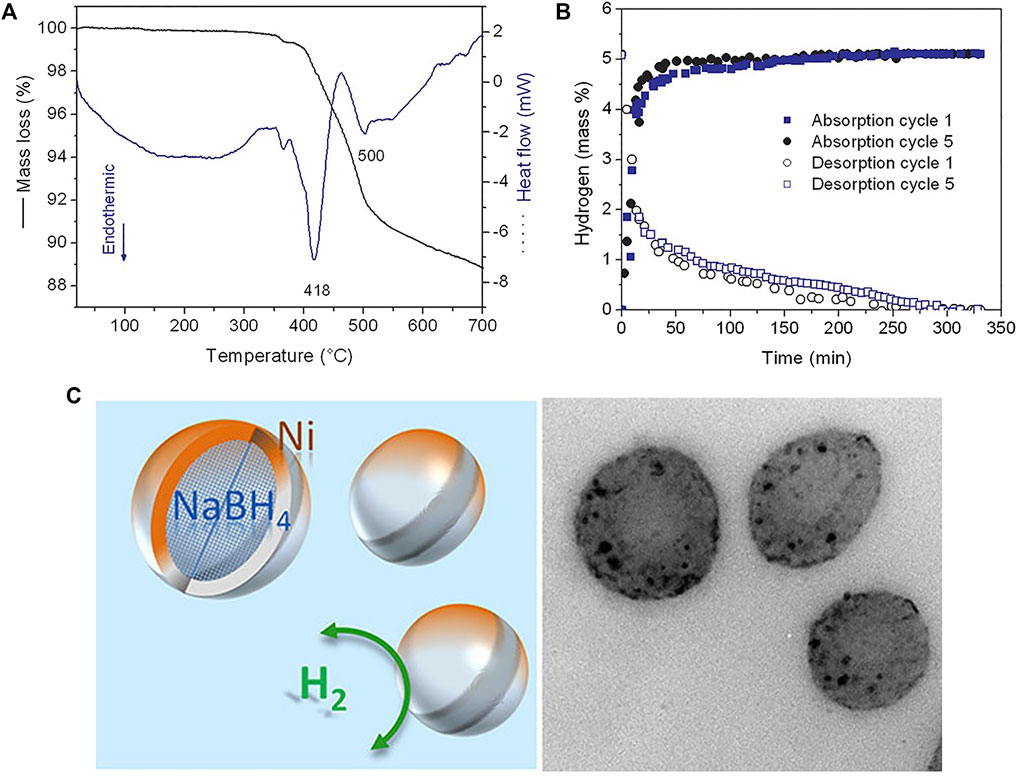
FIGURE 5. TGA/DSC curves (A) and isothermal hydrogen desorption curves at 350°C (B) of the nanoconfined NaBH4. (C) Models and TEM image of the Ni-doped nanoconfined NaBH4 (Christian and Aguey-Zinsou, 2012).
When the catalyst is added to the hydride through ball milling, the catalyst can be uniformly distributed on the surface and grain boundaries of the hydride, which is beneficial to the dissociation and recombination of hydrogen in the hydride. It was found that adding Ti, TiH2, TiF3 are beneficial to improve the thermodynamics of NaBH4, reducing the hydrogen desorption temperature of NaBH4, and among them, TiF3 has the best catalytic effect. TiF3 and NaBH4 will react with each other to form TiB2, and this formed TiB2 will catalyze the decomposition of the remaining NaBH4, and TiB2 will also catalyze the regeneration of NaBH4 to promote the stability of the cycle (Garroni et al., 2009).
In general, the research on NaBH4 mainly focus on its hydrolysis or methanolysis to generate hydrogen and limited papers on its dehydriding and rehydriding were reported (Santos and Sequeira, 2011). This is partially due to the high thermal stability and rather slow kinetics. Therefore, NaBH4 is more suitable for hydrogen generation by a hydrolysis or methanolysis process (Saka, 2021; Saka and Balbay, 2021; Saka, 2022a; Saka, 2022b; Saka, 2022c; Saka and Balbay, 2022).
Mg(BH4)2
The mass hydrogen storage density of Mg(BH4)2 is 14.8 wt%, and the volume hydrogen storage density is 112 g L−1. Mg(BH4)2 has a variety of crystal structures, and each crystal structures can transform into the other at different temperatures, which is determined by its own coordination of two [BH4]–, thus increasing the complexity of the molecular structure. There are about seven crystal structures reported for Mg(BH4)2, but although there are many crystal forms, they all transform to high-temperature stable β phase before hydrogen evolution, and this will lead to that different crystal forms have little effect on its hydrogen absorption and desorption properties.
The decomposition of α-Mg(BH4)2 first undergoes a phase transition at 190°C, and then decomposes into MgH2, Mg and MgB2 with the increase of temperature. The decomposition is divided into two steps (Chłopek et al., 2007; Li et al., 2007):
The desorption temperature of Mg(BH4)2 is lower than that of LiBH4, but a stable MgB12H12 is generated during the thermal decomposition of Mg(BH4)2, which makes Mg(BH4)2 have high thermodynamic stability and kinetic barrier (Yan et al., 2015). It can only be recovered under the conditions of 95 MPa and 400°C, so improving its hydrogen storage performance has become a research hotspot.
Adding transition metals and their compounds is a common method to improve hydrogen storage materials, and Ti-based compounds are one of the most commonly used additives to improve the performance of hydrogen storage materials. Bardaji et al. (2011) made TiCl3 and Mg(BH4)2 into nanocomposite materials, and found that the initial hydrogen desorption temperature was reduced to above 100°C, and further study shows that Mg(BH4)2 doped with NbCl5–TiCl3 nanocomposite could lead to that 5 wt% hydrogen could be released when the dehydrogenation temperature was lowered below 300°C, but the cycle performance of Mg(BH4)2 was not improved by doping.
Saldan et al. (2015) studied the effect of nickel-based additives such as nano-Ni, NiCl2, NiF2, and Ni3B on the hydrogen absorption and desorption performance of Mg(BH4)2. The Mg(BH4)2 was partially decomposed, and amorphous Ni3B appeared in the decomposition products. Ni3B did not participate in the reaction in the process of re-absorbing hydrogen. The addition of Ni3B can also effectively improve the hydrogen desorption kinetics of Mg(BH4)2, and the in-situ formed compound Ni3B is the main reason for improvement of the kinetic properties of Mg(BH4)2. Wang et al. (2020b) carried out ball milling of LiBH4 and MgCl2 to synthesize Mg(BH4)2 in situ. In fact, the temperature was reduced to 126.9°C, and the activation energy was reduced from 487.99 kJ mol−1 to 120.1 kJ mol−1 compared with the original Mg(BH4)2. Therefore, the hydrogen storage kinetics of Mg(BH4)2 is significantly improved. Further investigation showed that by adding NbF5 into the composites can also lead to better hydrogen storage properties than pristine and amorphous Mg(BH4)2. The catalyzed composite starts to release hydrogen at about 120°C with a total capacity of 10.04 wt%. The reversibility of the catalytic composite was also improved, and the catalytic composite could still release 4 wt% H2 in the third and fourth cycles.
Combining LiBH4 to form the LiBH4–Mg(BH4)2 composite system can also improve its hydrogen storage performance of Mg(BH4). Zhao-Karger et al. (2011) found that when LiBH4 and Mg(BH4)2 were mixed and ball-milled with molar ratio close to 1:1, the composite system would eutectic and release hydrogen at about 170°C, compared with pure Mg(BH4)2. The dehydrogenation temperature of the composite system decreased by about 100°C. Chen et al. (2012) used a Co-based catalyst to catalyze the hydrogen release of the LiBH4–Mg(BH4)2 system, which could make the composite system start to release hydrogen from 155°C, and at the same time, the hydrogen release rate of the system was increased by 1.6 times at 270°C.
Although researchers have tried various methods to improve the hydrogen storage performance of Mg(BH4)2, its initial hydrogen desorption temperature is still high, and the kinetic performance of Mg(BH4)2 at low temperature still needs to be improved.
Ca(BH4)2
Ca(BH4)2 is soluble in water but does not undergo hydrolysis reaction. It can exist stably in dry air, and begins to decompose at 360°C. The theoretical mass hydrogen storage density is 11.6 wt%, while 9.6 wt% of hydrogen can practically be released.
The decomposition of Ca(BH4)2 to release hydrogen is a multi-step reaction process accompanied by the formation of various possible intermediates. The reaction equation is as Eq. 6. At 347°C–387°C, Ca(BH4)2 decomposes to form CaH2 and some intermediate products, and at 397–497°C, the intermediate products decompose to form amorphous B and CaB6. Due to the complexity of the hydrogen absorption and desorption process of Ca(BH4)2, it is quite difficult to improve its hydrogen absorption and desorption kinetics.
Kim et al. (2008) studied the effect of transition metal halides on the dehydrogenation performance of Ca(BH4)2, and found that NbF5 would reduce the onset hydrogen desorption temperature of Ca(BH4)2 by 20°C. Under the conditions of 350°C and 90 bar H2, reversible uptake of 5 wt% H2 can be achieved. They believe that the catalytic effect of NbF5 may be because its melting point is only 77°C, which is melted during the ball milling process, so that it can be better distributed in Ca(BH4)2. In addition, NbF5 can make Ca(BH4)2 form CaH2−xFx solid solution phase in the process of dehydrogenation, which lead to the reverse reaction to generate CaH2 at 350°C and 90 bar. Minella’s further research (Bonatto Minella et al., 2011; Bonatto Minella et al., 2013; Bonatto Minella et al., 2015) found that when TiF4 and NbF5 catalysts were added, Ca(BH4)2 could generate CaB6 after dehydrogenation, and the formation of CaB6 was the key to the cyclic hydrogen absorption and desorption of Ca(BH4)2. The Ca(BH4)2 sample added with TiF4 and NbF5 catalysts can realize the reverse reaction at 350°C and 145 bar.
Chu et al. (2011) studied the composite system of Ca(BH4)2–2Mg(NH2)2 and Ca(BH4)2–2Ca(NH2)2, and found that the initial hydrogen desorption temperature of the composite system is 100°C lower than that of pure Ca(BH4)2. At 480°C, Ca(BH4)2–2Mg(NH2)2 can release 8.3 wt% hydrogen, and Ca(BH4)2–2Ca(NH2)2 can release 6.8 wt% hydrogen. Compared with pure Ca(BH4)2 samples, both have lower activation energy for dehydrogenation, and better hydrogen storage performance of Ca(BH4)2.
As a high-capacity hydrogen storage material, the hydrogen storage performance of Ca(BH4)2 can be improved by introducing catalysts and other means. But from the application point of view, there are still many issues need to be addressed for Ca(BH4)2. Many problems remain to be studied. For example, NbF5, which has the best catalytic effect among the catalysts, can only reduce the dehydrogenation temperature by 20°C. Although the forming composite of Ca(BH4)2 with other metal borides and amides can improve the performance of Ca(BH4)2 to a certain extent. However, the hydrogen desorption temperature of the composite system is still high, and the purity of hydrogen in the released gas is not high enough. It is still the research focus of Ca(BH4)2 hydrogen storage materials to find more effective methods to improve the hydrogen storage performance of Ca(BH4)2.
Conclusion
The metal borohydrides commonly found as LiBH4, NaBH4, Mg(BH4)2, and Ca(BH4)2 all have a high hydrogen capacity higher than 10 wt%, which is much higher than that of the materials that have been practically applied. Borohydrides also have cyclic hydrogen absorption and desorption properties, so borohydrides are one of the main research objects of solid-state hydrogen storage materials. In this paper, the common methods of borohydride modification, such as destabilization, catalysis, nanoconfinement, etc., are summarized. These methods have improved the hydrogen storage performance of borohydrides to a certain extent, but still cannot meet the comprehensive application requirements of fast kinetics, near room temperature operation, stable hydrogen absorption and desorption cycle performance, and long cycle life. Therefore, the research on borohydrides still needs to be done to find more effective methods to improve their hydrogen storage performance.
To tailor the hydrogen storage properties of metal borohydrides, the thermodynamic destabilization and kinetic improvement should be simultaneously considered by combination of various modification methods, which is the future research direction of metal borohydrides. In addition, prototype based on some metal borohydrides should be built to verify the practical hydrogen storage performances. Although the operating temperatures of metal borohydrides are relatively high compared with the traditional hydrogen storage alloys, the practical application is still possible when combined with high-temperature solid oxide fuel cell. Therefore, metal borohydrides are still promising materials for hydrogen storage.
Author contributions
JL, YM, and JY: Writing-Original draft preparation. LS and DG: Writing-Reviewing. PX: Funding and Editing.
Funding
We declare that the project of State Grid Jiangsu Electric Power Co., Ltd. (J2021175) and the National Natural Science of China (61904116) co-funded this research. The authors declare that State Grid Jiangsu Electric Power Co., Ltd. was not involved in the study design, collection, analysis, interpretation of data, the writing of this article or the decision to submit it for publication.
Acknowledgments
We acknowledge the project support from State Grid Jiangsu Electric Power Co., Ltd. (J2021175) and the National Natural Science Foundation of China (61904116).
Conflict of interest
JL, YM, JY, LS, DG, and PX were employed by State Grid Jiangsu Electric Power Co, Ltd. Research Institute.
Publisher’s note
All claims expressed in this article are solely those of the authors and do not necessarily represent those of their affiliated organizations, or those of the publisher, the editors and the reviewers. Any product that may be evaluated in this article, or claim that may be made by its manufacturer, is not guaranteed or endorsed by the publisher.
References
Bardaji, E. G., Hanada, N., Zabara, O., and Fichtner, M. (2011). Effect of several metal chlorides on the thermal decomposition behaviour of Mg(BH4)2. Int. J. Hydrogen Energy 36 (19), 12313–12318. doi:10.1016/j.ijhydene.2011.07.008
Barkhordarian, G., Klassen, T., Dornheim, M., and Bormann, R. (2007). Unexpected kinetic effect of MgB2 in reactive hydride composites containing complex borohydrides. J. Alloys Compd. 440 (1-2), L18–L21. doi:10.1016/j.jallcom.2006.09.048
Bonatto Minella, C., Garroni, S., Pistidda, C., Gosalawit-Utke, R., Barkhordarian, G., Rongeat, C., et al. (2011). Effect of transition metal fluorides on the sorption properties and reversible formation of Ca(BH4)2. J. Phys. Chem. C 115 (5), 2497–2504. doi:10.1021/jp107781m
Bonatto Minella, C., Pellicer, E., Rossinyol, E., Karimi, F., Pistidda, C., Garroni, S., et al. (2013). Chemical state, distribution, and role of Ti- and Nb-based additives on the Ca(BH4)2 system. J. Phys. Chem. C 117 (9), 4394–4403. doi:10.1021/jp3116275
Bonatto Minella, C., Garroni, S., Pistidda, C., Baró, M. D., Gutfleisch, O., Klassen, T., et al. (2015). Sorption properties and reversibility of Ti(IV) and Nb(V)-fluoride doped-Ca(BH4)2–MgH2 system. J. Alloys Compd. 622, 989–994. doi:10.1016/j.jallcom.2014.11.038
Bosenberg, U., Doppiu, S., Mosegaard, L., Barkhordarian, G., Eigen, N., Borgschulte, A., et al. (2007). Hydrogen sorption properties of MgH2–LiBH4 composites. Acta Mat. 55 (11), 3951–3958. doi:10.1016/j.actamat.2007.03.010
Bosenberg, U., Ravnsbaek, D. B., Hagemann, H., D'Anna, V., Minella, C. B., Pistidda, C., et al. (2010). Pressure and temperature influence on the desorption pathway of the LiBH4–MgH2 composite system. J. Phys. Chem. C 114 (35), 15212–15217. doi:10.1021/jp104814u
Cai, W. T., Wang, H., Liu, J. W., Jiao, L. F., Wang, Y. J., Ouyang, L. Z., et al. (2014). Towards easy reversible dehydrogenation of LiBH4 by catalyzing hierarchic nanostructured CoB. Nano Energy 10, 235–244. doi:10.1016/j.nanoen.2014.09.001
Chen, P., Xiong, Z. T., Luo, J. Z., Lin, J. Y., and Tan, K. L. (2002). Interaction of hydrogen with metal nitrides and imides. Nature 420 (6913), 302–304. doi:10.1038/nature01210
Chen, J., Yao, Z., Xiong, Z., Wu, G., Ping, C., He, T., et al. (2012). Enhanced hydrogen desorption from the Co-catalyzed LiBH4–Mg(BH4)2 eutectic composite. Int. J. Hydrogen Energy 37 (17), 12425–12431. doi:10.1016/j.ijhydene.2012.06.057
Cheng, C., Chen, M., Xiao, X., Huang, X., Zheng, J., and Chen, L. (2018). Superior reversible hydrogen storage properties and mechanism of LiBH4–MgH2–Al doped with NbF5 additive. J. Phys. Chem. C 122 (14), 7613–7620. doi:10.1021/acs.jpcc.8b00959
Chłopek, K., Frommen, C., Léon, A., Zabara, O., and Fichtner, M. (2007). Synthesis and properties of magnesium tetrahydroborate, Mg(BH4)2. J. Mat. Chem. 17 (33), 3496–3503. doi:10.1039/B702723K
Christian, M. L., and Aguey-Zinsou, K. F. (2012). Core-shell strategy leading to high reversible hydrogen storage capacity for NaBH4. ACS Nano 6 (9), 7739–7751. doi:10.1021/nn3030018
Chu, H., Wu, G., Zhang, Y., Xiong, Z., Guo, J., He, T., et al. (2011). Improved dehydrogenation properties of calcium borohydride combined with alkaline-earth metal amides. J. Phys. Chem. C 115 (36), 18035–18041. doi:10.1021/jp2052695
Ding, Z., Li, H., and Shaw, L. (2020). New insights into the solid-state hydrogen storage of nanostructured LiBH4–MgH2 system. Chem. Eng. J. 385, 123856. doi:10.1016/j.cej.2019.123856
Fang, Z. Z., Kang, X. D., Dai, H. B., Zhang, M. J., Wang, P., and Cheng, H. M. (2008). Reversible dehydrogenation of LiBH4 catalyzed by as-prepared single-walled carbon nanotubes. Scr. Mater. 58 (10), 922–925. doi:10.1016/j.scriptamat.2008.01.024
Garroni, S., Pistidda, C., Brunelli, M., Vaughan, G., Suri?Ach, S., and Baró, M. D. (2009). Hydrogen desorption mechanism of 2NaBH4 + MgH2 composite prepared by high-energy ball milling. Scr. Mat. 60 (12), 1129–1132. doi:10.1016/j.scriptamat.2009.02.059
Garroni, S., Olid, D., Teixidor, F., Suri? Ac H, S., Baró, M., and Caputo, R. (2010). Can Na2[B12H12] be a decomposition product of NaBH4? Phys. Chem. Chem. Phys. 12 (45), 15093–15100. doi:10.1039/C0CP00877J
Gosalawit-Utke, R., Nielsen, T. K., Saldan, I., Laipple, D., Cerenius, Y., Jensen, T. R., et al. (2011). Nanoconfined 2LiBH4–MgH2 prepared by direct melt Infiltration into nanoporous materials. J. Phys. Chem. C 115 (21), 10903–10910. doi:10.1021/jp2021903
Gosalawitutke, R., Nielsen, T. K., Pranzas, K., Saldan, I., Dornheim, M., Karimi, F., et al. (2012). 2LiBH4–MgH2 in a resorcinol furfural carbon aerogel scaffold for reversible hydrogen storage. J. Phys. Chem. C 116 (1), 1526–1534. doi:10.1021/jp2088127
Gross, A. F., Vajo, J. J., Vanatta, S. L., and Olson, G. L. (2008). Enhanced hydrogen storage kinetics of LiBH4 in nanoporous carbon scaffolds. J. Phys. Chem. C 112 (14), 5651–5657. doi:10.1021/jp711066t
Harris, P. M., and Meibohm, E. P. (2002). The crystal structure of lithium borohydride LiBH4. J. Am. Chem. Soc. 69 (5), 1231–1232. doi:10.1021/ja01197a524
Jiang, W., Wang, H., and Zhu, M. (2021). AlH3 as a hydrogen storage material: Recent advances, prospects and challenges. Rare Met. 40, 3337–3356. doi:10.1007/s12598-021-01769-2
Johnson, S. R., David, W. I. F., Royse, D. M., Sommariva, M., Tang, C. Y., Fabbiani, F. P. A., et al. (2009). The monoammoniate of lithium borohydride, Li(NH3)BH4: An effective ammonia storage compound. Chem. Asian J. 4, 849–854. doi:10.1002/asia.200900051
Jongh, P. E. d., and Adelhelm, P. (2010). Nanosizing and nanoconfinement: New strategies towards meeting hydrogen storage goals. ChemsusChem 3 (12), 1332–1348. doi:10.1002/cssc.201000248
Kang, X. D., Wang, K. K., Zhong, Y. J., Yang, B., and Wang, P. (2013). A novel three-step method for preparation of a TiB2-promoted LiBH4–MgH2 composite for reversible hydrogen storage. Phys. Chem. Chem. Phys. 15 (6), 2153–2158. doi:10.1039/c2cp43532b
Kim, J. H., Shim, J. H., and Cho, Y. W. (2008). On the reversibility of hydrogen storage in Ti- and Nb-catalyzed Ca(BH4)2. J. Power Sources 181 (1), 140–143. doi:10.1016/j.jpowsour.2008.02.094
Kim, K.-B., Shim, J.-H., Park, S.-H., Choi, I.-S., Oh, K. H., and Cho, Y. W. (2015). Dehydrogenation reaction pathway of the LiBH4–MgH2 composite under various pressure conditions. J. Phys. Chem. C 119 (18), 9714–9720. doi:10.1021/jp5123757
Le, T. T., Pistidda, C., Nguyen, V. H., Singh, P., Raizada, P., Klassen, T., et al. (2021). Nanoconfinement effects on hydrogen storage properties of MgH2 and LiBH4. Int. J. Hydrogen Energy 46 (46), 23723–23736. doi:10.1016/j.ijhydene.2021.04.150
Li, H.-W., Kikuchi, K., Nakamori, Y., Miwa, K., Towata, S., and Orimo, S. (2007). Effects of ball milling and additives on dehydriding behaviors of well-crystallized Mg(BH4)2. Scr. Mat. 57 (8), 679–682. doi:10.1016/j.scriptamat.2007.06.052
Li, Y., Zhang, Y., Gao, M., Pan, H., and Liu, Y. (2017). Preparation and catalytic effect of porous Co3O4 on the hydrogen storage properties of a Li-B-N-H system. Prog. Nat. Sci. Mater. Int. 27 (1), 132–138. doi:10.1016/j.pnsc.2016.12.010
Li, Z., Wang, S., Gao, M., Xian, K., Shen, Y., Yang, Y., et al. (2022). Catalyzed LiBH4 hydrogen storage system with in situ introduced Li3BO3 and V for enhanced dehydrogenation and hydrogenation kinetics as well as high cycling stability. ACS Appl. Energy Mat. 5 (1), 1226–1234. doi:10.1021/acsaem.1c03608
Li, Z., Gao, M., Wang, S., Zhang, X., Gao, P., Yang, Y., et al. (2022). In-situ introduction of highly active TiO for enhancing hydrogen storage performance of LiBH4. Chem. Eng. J. 433, 134485. doi:10.1016/j.cej.2021.134485
Lin, H.-J., Lu, Y.-S., Zhang, L.-T., Liu, H.-Z., Edalati, K., and Révész, Á. (2022). Recent advances in metastable alloys for hydrogen storage: A review. Rare Met. 41 (6), 1797–1817. doi:10.1007/s12598-021-01917-8
Liu, B. H., and Li, Z. P. (2009). Current status and progress of direct borohydride fuel cell technology development. J. Power Sources 187 (2), 291–297. doi:10.1016/j.jpowsour.2008.11.017
Liu, X., Peaslee, D., Jost, C. Z., Baumann, T. F., and Majzoub, E. H. (2011). Systematic pore-size effects of nanoconfinement of LiBH4: Elimination of diborane release and tunable behavior for hydrogen storage applications. Chem. Mat. 23 (5), 1331–1336. doi:10.1021/cm103546g
Liu, H. Z., Wang, X. H., Zhou, H., Gao, S. C., Ge, H. W., Li, S. Q., et al. (2016). Improved hydrogen desorption properties of LiBH4 by AlH3 addition. Int. J. Hydrogen Energy 41 (47), 22118–22127. doi:10.1016/j.ijhydene.2016.09.177
Liu, H. Z., Xu, L., Sheng, P., Liu, S. Y., Zhao, G. Y., Wang, B., et al. (2017). Hydrogen desorption kinetics of the destabilized LiBH4-AlH3 composites. Int. J. Hydrogen Energy 42 (35), 22358–22365. doi:10.1016/j.ijhydene.2016.12.083
Liu, X.-S., Liu, H.-Z., Qiu, N., Zhang, Y.-B., Zhao, G.-Y., Xu, L., et al. (2021). Cycling hydrogen desorption properties and microstructures of MgH2–AlH3–NbF5 hydrogen storage materials. Rare Met. 40 (4), 1003–1007. doi:10.1007/s12598-020-01425-1
Lu, Z.-Y., Yu, H.-J., Lu, X., Song, M.-C., Wu, F.-Y., Zheng, J.-G., et al. (2021). Two-dimensional vanadium nanosheets as a remarkably effective catalyst for hydrogen storage in MgH2. Rare Met. 40 (11), 3195–3204. doi:10.1007/s12598-021-01764-7
Lv, Y., and Wu, Y. (2021). Current research progress in magnesium borohydride for hydrogen storage (A review). Prog. Nat. Sci. Mater. Int. 31 (6), 809–820. doi:10.1016/j.pnsc.2021.11.001
Mao, J., Guo, Z., Yu, X., and Liu, H. (2013). Combined effects of hydrogen back-pressure and NbF5 addition on the dehydrogenation and rehydrogenation kinetics of the LiBH4–MgH2 composite system. Int. J. Hydrogen Energy 38 (9), 3650–3660. doi:10.1016/j.ijhydene.2012.12.106
Martelli, P., Caputo, R., Remhof, A., Mauron, P., Borgschulte, A., and Zuttel, A. (2010). Stability and decomposition of NaBH4. J. Phys. Chem. C 114 (15), 7173–7177. doi:10.1021/jp909341z
Milanese, C., Garroni, S., Girella, A., Mulas, G., Berbenni, V., Bruni, G., et al. (2011). Thermodynamic and kinetic investigations on pure and doped NaBH4-MgH2 system. J. Phys. Chem. C 115 (7), 3151–3162. doi:10.1021/jp109392e
Ming, A., Arthur, R., Jurgensen; William, ; A., Spencer, A, Donald, L., Hwang, S. J., et al. (2008). Stability and reversibility of lithium borohydrides doped by metal halides and hydrides. J. Phys. Chem. C 112 (47), 18661–18671. doi:10.1021/jp8024304
Nakagawa, T., Ichikawa, T., Hanada, N., Kojima, Y., and Fujii, H. (2007). Thermal analysis on the Li–Mg–B–H systems. J. Alloys Compd. 446, 306–309. doi:10.1016/j.jallcom.2007.02.097
Ngene, P., Roy, V., Verkuijlen, M., Jong, K. D., and Jongh, P. D. (2011). Reversibility of the hydrogen desorption from NaBH4 by confinement in nanoporous carbon. Energy Environ. Sci. 4 (10), 4108–4115. doi:10.1039/C1EE01481A
Orimo, S., Nakamori, Y., Matsushima, T., Ichikawa, T., Chen, D., Gottwald, J., et al. (2002). Nanostructured carbon-related materials for hydrogen storage. Columbus: International Symposium on Processing and Fabrication of Advanced Materials XI, 123–131.In
Orimo, S. I., Nakamori, Y., Eliseo, J. R., Züttel, A., and Jensen, C. M. (2007). Complex hydrides for hydrogen storage. Chem. Rev. 107 (10), 4111–4132. doi:10.1021/cr0501846
Pinkerton, F. E., Meisner, G. P., Meyer, M. S., Balogh, M. P., and Kundrat, M. D. (2005). Hydrogen desorption exceeding ten weight percent from the new quaternary hydride Li3BN2H8. J. Phys. Chem. B 109 (1), 6–8. doi:10.1021/jp0455475
Pinkerton, F. E., Meyer, M. S., Meisner, G. P., Balogh, M. P., and Vajo, J. J. (2007). Phase boundaries and reversibility of LiBH4/MgH2 hydrogen storage material. J. Phys. Chem. C 111 (35), 12881–12885. doi:10.1021/jp0742867
Puszkiel, J., Gasnier, A., Amica, G., and Gennari, F. (2020). Tuning LiBH4 for hydrogen storage: Destabilization, additive, and nanoconfinement approaches. Molecules 25 (1), 163. doi:10.3390/molecules25010163
Ravnsbaek, D. B., and Jensen, T. R. (2012). Mechanism for reversible hydrogen storage in LiBH4-Al. J. Appl. Phys. 111 (11), 112621. doi:10.1063/1.4726244
Saka, C., and Balbay, A. (2021). Oxygen and nitrogen-functionalized porous carbon particles derived from hazelnut shells for the efficient catalytic hydrogen production reaction. Biomass Bioenergy 149, 106072. doi:10.1016/j.biombioe.2021.106072
Saka, C., and Balbay, A. (2022). Metal-free catalyst fabrication by incorporating oxygen groups on the surface of the carbonaceous sample and efficient hydrogen production from NaBH4 methanolysis. Int. J. Hydrogen Energy 47 (11), 7242–7251. doi:10.1016/j.ijhydene.2021.12.070
Saka, C. (2021). Oxygen and nitrogen-doped metal-free microalgae carbon nanoparticles for efficient hydrogen production from sodium borohydride in methanol. Int. J. Hydrogen Energy 46 (52), 26298–26307. doi:10.1016/j.ijhydene.2021.05.111
Saka, C. (2022). Phosphorus decorated g-C3N4-TiO2 particles as efficient metal-free catalysts for hydrogen release by NaBH4 methanolysis. Fuel 322, 124196. doi:10.1016/j.fuel.2022.124196
Saka, C. (2022). Efficient and durable H2 production from NaBH4 methanolysis using N doped hybrid g-C3N4-SiO2 composites with ammonia as a nitrogen source. Fuel 324, 124594. doi:10.1016/j.fuel.2022.124594
Saka, C. (2022). Surface modification with oxygen doping of g-C3N4 nanoparticles by carbon vacancy for efficient dehydrogenation of sodium borohydride in methanol. Fuel 310, 122444. doi:10.1016/j.fuel.2021.122444
Saldan, I., Hino, S., Hamphries, T. D., Zavorotynska, O., Hauback, B. C., Jensen, C. M., et al. (2015). Structural changes observed during the reversible hydrogenation of Mg(BH4)2 with Ni-based additives. J. Phys. Chem. C 118 (40), 23376–23384. doi:10.1021/jp5066677
Santos, D. M. F., and Sequeira, C. A. C. (2011). Sodium borohydride as a fuel for the future. Renew. Sustain. Energy Rev. 15 (8), 3980–4001. doi:10.1016/j.rser.2011.07.018
Schlapbach, L., and Zuttel, A. (2001). Hydrogen-storage materials for mobile applications. Nature 414 (6861), 353–358. doi:10.1038/35104634
Shao, H., Felderhoff, M., and Weidenthaler, C. (2015). Kinetics enhancement, reaction pathway change, and mechanism clarification in LiBH4 with Ti-catalyzed nanocrystalline MgH2 composite. J. Phys. Chem. C 119 (5), 2341–2348. doi:10.1021/jp511479d
Shaw, L. L., Wan, X. F., Hu, J. Z., Kwak, J. H., and Yang, Z. G. (2010). Solid-state hydriding mechanism in the LiBH4 + MgH2 system. J. Phys. Chem. C 114 (17), 8089–8098. doi:10.1021/jp1003837
Soulié, J.-P., Renaudin, G., Černý, R., and Yvon, K. (2002). Lithium boro-hydride LiBH4: I. Crystal structure. J. Alloys Compd. 346 (1-2), 200–205. doi:10.1016/S0925-8388(02)00521-2
Stasinevich, D. S., and Egorenko, G. A. (1968). Thermographic investigation of alkali metal and magnesium tetrahydroborates at pressures up to 10 atm. Russ. J. Inorg. Chem. 13 (3), 341–343.
Sulaiman, N. N., Ismail, M., Timmiati, S. N., and Lim, K. L. (2021). Improved hydrogen storage performances of LiAlH4 + Mg(BH4)2 composite withTiF3 addition. Int. J. Energy Res. 45 (2), 2882–2898. doi:10.1002/er.5984
Umegaki, T., Yan, J.-M., Zhang, X.-B., Shioyama, H., Kuriyama, N., and Xu, Q. (2009). Boron- and nitrogen-based chemical hydrogen storage materials. Int. J. Hydrogen Energy 34 (5), 2303–2311. doi:10.1016/j.ijhydene.2009.01.002
Urgnani, J., Torres, F. J., Palumbo, M., and Baricco, M. (2008). Hydrogen release from solid state NaBH4. Int. J. Hydrogen Energy 33 (12), 3111–3115. doi:10.1016/j.ijhydene.2008.03.031
Vajo, J. J., Skeith, S. L., and Mertens, F. (2005). Reversible storage of hydrogen in destabilized LiBH4. J. Phys. Chem. B 109 (9), 3719–3722. doi:10.1021/jp040769o
Vajo, J. J., Salguero, T. T., Gross, A. E., Skeith, S. L., and Olson, G. L. (2007). Thermodynamic destabilization and reaction kinetics in light metal hydride systems. J. Alloys Compd. 446, 409–414. doi:10.1016/j.jallcom.2007.02.080
Verkuijlen, M., Ngene, P., Kort, D., Barré, C., Nale, A., Eck, E., et al. (2012). Nanoconfined LiBH4 and enhanced mobility of Li+ and BH4– studied by solid-state NMR. J. Phys. Chem. C 116 (42), 22169–22178. doi:10.1021/jp306175b
Wang, K. K., Kang, X. D., Luo, J. H., Hu, C. H., and Wang, P. (2013). Improved reversible dehydrogenation properties of LiBH4-MgH2 composite by tailoring nanophase structure using activated carbon. Int. J. Hydrogen Energy 38 (9), 3710–3716. doi:10.1016/j.ijhydene.2013.01.034
Wang, K. K., Kang, X. D., Kang, Q., Zhong, Y. J., Hu, C. H., and Wang, P. (2014). Improved reversible dehydrogenation of 2LiBH4-MgH2 composite by the controlled formation of transition metal boride. J. Mat. Chem. A 2 (7), 2146–2151. doi:10.1039/c3ta14176d
Wang, K. K., Kang, X. D., Zhong, Y. J., Hu, C. H., Ren, J. W., and Wang, P. (2014). Unexpected dehydrogenation behaviors of the 2LiBH4-MgH2 composite confined in a mesoporous carbon scaffold. J. Phys. Chem. C 118 (46), 26447–26453. doi:10.1021/jp505535h
Wang, K. K., Kang, X. D., Zhong, Y. J., Hu, C. H., and Wang, P. (2014). Improved reversible dehydrogenation properties of 2LiBH4-MgH2 composite by milling with graphitic carbon nitride. Int. J. Hydrogen Energy 39 (25), 13369–13374. doi:10.1016/j.ijhydene.2014.04.079
Wang, S., Gao, M. X., Xian, K. C., Li, Z. L., Shen, Y., Yao, Z. H., et al. (2020). LiBH4 nanoconfined in porous hollow carbon nanospheres with high loading, low dehydrogenation temperature, superior kinetics, and favorable reversibility. ACS Appl. Energy Mat. 3 (4), 3928–3938. doi:10.1021/acsaem.0c00325
Wang, X., Xiao, X., Zheng, J., Huang, X., Chen, M., Chen, L., et al. (2020). In-situ synthesis of amorphous Mg(BH4)2 and chloride composite modified by NbF5 for superior reversible hydrogen storage properties. Int. J. Hydrogen Energy 45 (3), 2044–2053. doi:10.1016/j.ijhydene.2019.11.023
Wang, X., Xiao, X., Liang, Z., Zhang, S., Qi, J., Lv, L., et al. (2022). Ultrahigh reversible hydrogen capacity and synergetic mechanism of 2LiBH4–MgH2 system catalyzed by dual-metal fluoride. Chem. Eng. J. 433, 134482. doi:10.1016/j.cej.2021.134482
Wang, S., Gao, M., Yao, Z., Liu, Y., Wu, M., Li, Z., et al. (2022). A nanoconfined-LiBH4 system using a unique multifunctional porous scaffold of carbon wrapped ultrafine Fe3O4 skeleton for reversible hydrogen storage with high capacity. Chem. Eng. J. 428, 131056. doi:10.1016/j.cej.2021.131056
Xia, G., Tan, Y., Chen, X., Fang, F., Sun, D., Li, X., et al. (2017). Oxygen-free layer-by-layer assembly of lithiated composites on graphene for advanced hydrogen storage. Adv. Sci. 4 (9), 1600257. doi:10.1002/advs.201600257
Xian, K., Gao, M., Li, Z., Gu, J., Shen, Y., Wang, S., et al. (2019). Superior kinetic and cyclic performance of a 2D titanium carbide incorporated 2LiH + MgB2 composite toward highly reversible hydrogen storage. ACS Appl. Energy Mat. 2 (7), 4853–4864. doi:10.1021/acsaem.9b00557
Xian, K., Nie, B., Li, Z., Gao, M., Li, Z., Shang, C., et al. (2021). TiO2 decorated porous carbonaceous network structures offer confinement, catalysis and thermal conductivity for effective hydrogen storage of LiBH4. Chem. Eng. J. 407, 127156. doi:10.1016/j.cej.2020.127156
Xiong, Z., Cao, L., Wang, J., and Mao, J. (2017). Hydrolysis behavior of LiBH4 films. J. Alloys Compd. 698, 495–500. doi:10.1016/j.jallcom.2016.12.256
Yan, Y., Remhof, A., Rentsch, D., and Züttel, A. (2015). The role of MgB12H12 in the hydrogen desorption process of Mg(BH4)2. Chem. Commun. 51 (4), 700–702. doi:10.1039/c4cc05266h
Yang, J., Sudik, A., and Wolverton, C. (2007). Destabilizing LiBH4 with a metal (M = Mg, Al, Ti, V, Cr, or Sc) or metal hydride (MH2, MgH2, TiH2, or CaH2). J. Phys. Chem. C 111 (51), 19134–19140. doi:10.1021/jp076434z
Ye, J., Xia, G., and Yu, X. (2021). In-situ constructed destabilization reaction of LiBH4 wrapped with graphene toward stable hydrogen storage reversibility. Mat. Today Energy 22, 100885. doi:10.1016/j.mtener.2021.100885
Yu, Y. G., and Ross, N. L. (2011). Vibrational and thermodynamic properties of Ni3S2 polymorphs from first-principles calculations. Phys. Chem. Min. 38 (3), 241–249. doi:10.1007/s00269-010-0399-7
Zhang, Y., and Tian, Q. (2011). The reactions in LiBH4–NaNH2 hydrogen storage system. Int. J. Hydrogen Energy 36 (16), 9733–9742. doi:10.1016/j.ijhydene.2011.05.035
Zhang, Y., Tian, Q. F., Zhang, J., Liu, S. S., and Sun, L. X. (2009). The dehydrogenation reactions and kinetics of 2LiBH4–Al composite. J. Phys. Chem. C 113 (42), 18424–18430. doi:10.1021/jp903967y
Zhang, Y., Liu, Y., Yang, Y., Li, Y., Hu, J., Gao, M., et al. (2017). Superior catalytic activity of in situ reduced metallic Co for hydrogen storage in a Co(OH)2-containing LiBH4/2LiNH2 composite. Mat. Res. Bull. 97, 544–552. doi:10.1016/j.materresbull.2017.09.037
Zhang, W., Zhang, X., Huang, Z., Li, H.-W., Gao, M., Pan, H., et al. (2021). Recent development of lithium borohydride-based materials for hydrogen storage. Adv. Energy Sustain. Res. 2 (10), 2100073. doi:10.1002/aesr.202100073
Zhang, X., Zhang, W., Zhang, L., Huang, Z., Hu, J., Gao, M., et al. (2022). Single-pot solvothermal strategy toward support-free nanostructured LiBH4 featuring 12 wt% reversible hydrogen storage at 400 °C. Chem. Eng. J. 428, 132566. doi:10.1016/j.cej.2021.132566
Zhao-Karger, Z., Boucharat, N., Nale, A., Fichtner, M., Lohstroh, W., et al. (2011). LiBH4−Mg(BH4)2: A physical mixture of metal borohydrides as hydrogen storage material. J. Phys. Chem. C 115 (13), 6095–6101. doi:10.1021/jp110518s
Zheng, J., Yao, Z., Xiao, X., Wang, X., He, J., Chen, M., et al. (2021). Enhanced hydrogen storage properties of high-loading nanoconfined LiBH4–Mg(BH4)2 composites with porous hollow carbon nanospheres. Int. J. Hydrogen Energy 46 (1), 852–864. doi:10.1016/j.ijhydene.2020.09.177
Zhou, Y. F., Liu, Y. F., Wu, W., Zhang, Y., Gao, M. X., and Pan, H. G. (2012). Improved hydrogen storage properties of LiBH4 destabilized by in situ formation of MgH2 and LaH3. J. Phys. Chem. C 116 (1), 1588–1595. doi:10.1021/jp2101573
Zhou, H., Liu, H.-Z., Xu, L., Gao, S.-C., Wang, X. H., and Yan, M. (2017). Hydrogen storage properties of Nb-compounds-catalyzed LiBH4–MgH2. Rare Met. 36 (9), 723–728. doi:10.1007/s12598-017-0929-2
Zhou, H., Wang, X.-H., Liu, H.-Z., Gao, S.-C., and Yan, M. (2019). Improved hydrogen storage properties of LiBH4 confined with activated charcoal by ball milling. Rare Mater. 38 (4), 321–326. doi:10.1007/s12598-018-1067-1
Zuttel, A., Wenger, P., Rentsch, S., Sudan, P., Mauron, P., and Emmenegger, C. (2003). LiBH4 a new hydrogen storage material. J. Power Sources 118 (1-2), 1–7. doi:10.1016/s0378-7753(03)00054-5
Keywords: hydrogen energy, hydrogen storage, metal borohydride, destabilization, catalysis, composite
Citation: Liu J, Ma Y, Yang J, Sun L, Guo D and Xiao P (2022) Recent advance of metal borohydrides for hydrogen storage. Front. Chem. 10:945208. doi: 10.3389/fchem.2022.945208
Received: 16 May 2022; Accepted: 20 July 2022;
Published: 17 August 2022.
Edited by:
Elisabeta I. Szerb, Institute of Chemistry “Coriolan Dragulescu” of Romanian Academy, RomaniaCopyright © 2022 Liu, Ma, Yang, Sun, Guo and Xiao. This is an open-access article distributed under the terms of the Creative Commons Attribution License (CC BY). The use, distribution or reproduction in other forums is permitted, provided the original author(s) and the copyright owner(s) are credited and that the original publication in this journal is cited, in accordance with accepted academic practice. No use, distribution or reproduction is permitted which does not comply with these terms.
*Correspondence: Peng Xiao, dm9kb2NvQGZveG1haWwuY29t