- 1College of Biological and Pharmaceutical Engineering, West Anhui University, Lu’an, China
- 2College of Life and Health, Anhui Science and Technology University, Fengyang, China
- 3Institute of Biomedical Engineering, Chinese Academy of Medical Sciences and Peking Union Medical College, Tianjin Key Laboratory of Biomedical Material, Tianjin, China
- 4Mudanjiang Medical University, Mudanjiang, China
Ligustrazine (TMP) is a natural pyrazine alkaloid extracted from the roots of Ligusticum Chuanxiong Hort, which has the potential as an antitumor agent. A series of 33 ligustrazine–heterocycle (TMPH) derivatives were designed, synthesized, and investigated via antitumor screening assays, molecular docking analysis, and prediction of drug-like properties. TMP was attached to other heterocyclic derivatives by an 8–12 methylene alkyl chain as a linker to obtain 33 TMPH derivatives. The structures were confirmed by 1H-NMR, 13C-NMR, and high-resolution mass spectroscopy spectral (HR-MS) data. The antiproliferative activity against human breast cancer MCF-7, MDA-MB-231, mouse breast cancer 4T1, mouse fibroblast L929, and human umbilical vein endothelial HUVEC cell lines was evaluated by MTT assay. Compound 12–9 displayed significant inhibitory activity with IC50 values in the low micromolar range (0.84 ± 0.02 µM against the MDA-MB-231 cell line). The antitumor effects of compound 12–9 were further evaluated by plate cloning, Hoechst 33 342 staining, and annexin V-FITC/PI staining. The results indicated that compound 12–9 inhibited the proliferation and apoptosis of breast cancer cells. Furthermore, molecular docking of compound 12–9 into the active site of the Bcl-2, CASP-3, and PSMB5 target proteins was performed to explore the probable binding mode. The 33 newly synthesized compounds were predicted to have good drug-like properties in a theoretical study. Overall, these results indicated that compound 12–9 inhibited cell proliferation through PSMB5 and apoptosis through Bcl-2/CASP-3 apoptotic signaling pathways and had good drug-like properties. These results provided more information, and key precursor lead derivatives, in the search for effective bioactive components from Chinese natural medicines.
Introduction
Breast cancer is one of the most diagnosed malignant tumors in women worldwide, with high morbidity and mortality (Jaaks et al., 2022; Sammut et al., 2022). Triple-negative breast cancer (TNBC) is the most aggressive subtype of breast cancer (El-Arabey and Abdalla, 2022; Yang et al., 2022; Zhang S. et al., 2022) and is characterized by large tumor size, high content of positive lymph nodes, high histological grade with high mortality, and high recurrence and metastasis rates (Xiu et al., 2022). TNBC is one of the main causes of cancer death in women worldwide (Altei et al., 2022). The current strategies for the treatment of TNBC include radiotherapy (Raafat Elsayed et al., 2022), chemotherapy, and targeted therapy (Mao et al., 2021). The treatment of TNBC with natural medicines has also been investigated. Despite remarkable advances in the treatment of TNBC (Chen et al., 2022), the lack of efficacious anticancer drugs is still a problem (Zhang P. et al., 2022). In addition, the side effects of existing drugs are a major obstacle in the treatment of TNBC (Olivier and Prasad, 2022; Zhang D. et al., 2022). It is therefore desirable to develop new effective anticancer drugs for the treatment of TNBC.
Proteasomes play a critical role in nuclear and cytosolic proteolysis (Dwivedi et al., 2021; Jia et al., 2021), and the inhibition of proteasomes has been proposed as an effective strategy against TNBC (Ramdas et al., 2019). The proteasome 20S subunit beta 5 (PSMB5) (Liu et al., 2020), which is a key regulator of proteasome function (Wei et al., 2018), is a promising target protein for proteasome inhibition in the treatment of TNBC (Liu et al., 2020). Pathways related to PSMB5 include signaling by Hedgehog, mitotic G1-G1/S phases (Oerlemans et al., 2008), and down-regulation by IFN-gamma (at the protein level) (Rut and Drag, 2016). The high expression of PSMB5 has been shown to be indicative of poor prognosis in TNBC patients (Liu et al., 2020). Silencing of PSMB5 sensitized cells to apoptosis and ultimately enhanced the effect of chemotherapy (Wei et al., 2018; Adwal et al., 2020). PSMB5 has been associated with cancer cell proliferation in TNBC patients (Liu et al., 2020). As signaling pathways that regulate cell apoptosis and survival (Guan et al., 2022), Bcl-2 and CASP-3 apoptotic signaling pathways have also been implicated in TNBC by promoting, or inhibiting, apoptotic pathways triggered by mitochondrial dysfunction (Shen et al., 2020; An et al., 2021; Guan et al., 2022). The development of a potent anticancer agent that is capable of inhibiting proliferation through PSMB5 and apoptosis through Bcl-2/CASP-3 apoptotic signaling pathways has emerged as an effective strategy for TNBC treatment (Lang et al., 2019; Nabil et al., 2021). PSMB5, Bcl-2, and CASP-3 play key roles in the signaling pathway of TNBC, and these proteins are attractive targets in the development of ligand molecules as potential antitumor agents. Many compounds have been designed, synthesized, and evaluated as inhibitors of these target proteins. ABT-199 and derivatives with an acyl sulfonamide skeleton were shown to have antiproliferative effects targeting Bcl-2 (Wang et al., 2021). Bortezomib, carfilzomib, and syringolin analogs have demonstrated potential antitumor activity targeting PSMB5 (Yoshida et al., 2018; Allmeroth et al., 2021). Furthermore, compound 1,2,3-triazole-thiazolidinone-carvone hybrid derivatives showed moderate antiproliferative activity through the CASP-3 pathway (Oubella et al., 2021). Lead compounds, including curcumin, quercetin, and baicalin, from natural medicines, have also shown antitumor activity by regulating the signaling pathways of PSMB5, Bcl-2, and CASP-3 (Chen et al., 2018; Mortezaee et al., 2019).
Bioactive components from natural medicines, a key source of lead compounds for the development of innovative drugs (Kataura et al., 2021), have a wide range of biological activities (Jiang et al., 2021), including antitumor, anti-inflammatory, and anti-infection activity. Many antitumor agents, such as vinblastine, paclitaxel, and ligustrazine (TMP), have been isolated from natural products. Natural medicines can have additive or synergistic effects (Yang et al., 2021) by simultaneously acting on multiple targets of the TNBC pathway. Extracts of Rosmarinus officinalis L., Astragalus polysaccharides, and bioactive components of tetrandrine are useful in the treatment of TNBC because these compounds have the ability to improve the postoperative “tumor constitution” (Chen et al., 2021). TMP is a natural alkaloid monomer pyrazine derivative extracted and isolated from the roots of Ligusticum Chuanxiong Hort, which has been shown to be a potential antitumor agent (Xie et al., 2019). TMP has demonstrated multiple antitumor effects, including inhibiting proliferation (Luo et al., 2021), apoptosis, invasion, and metastasis of malignant tumors (Bian et al., 2021). The introduction of substitutions into the TMP nucleus may improve the antitumor effect (Xu et al., 2017a; Wang et al., 2019). A TMP dimer with an alkyl chain as the linker had significantly stronger antitumor activity than the TMP monomer (Li et al., 2014). TMP-chalcone hybrids demonstrated antiproliferative activity in an in vivo model of TNBC (Luo et al., 2021).
In the present study, flexible alkyl chains of different lengths (8–12 carbons) were used as linkers. TMP was structurally combined with other pharmaco-active heterocycles, according to the splicing method of active substructures, to synthesize new ligustrazine–heterocycle (TMPH) derivatives. A series of 33 TMPH derivatives were designed, synthesized, and investigated via antitumor screening assays, molecular docking with Bcl-2, CASP-3, and PSMB5 target proteins, and prediction of drug-like properties. The structures of the newly synthesized derivatives were confirmed by 1H-NMR, 13C-NMR, and HR-MS spectral data. The antiproliferative activity against five cell lines (human breast cancer MCF-7 and MDA-MB-231, mouse breast cancer 4T1, mouse fibroblast L929, and human umbilical vein endothelial HUVEC) was evaluated by MTT assay. In addition, one of the TMPH derivatives with potent antiproliferative activity was selected for further evaluation by plate cloning, Hoechst 33 342 staining, and annexin V-FITC/PI staining. Molecular docking into the active site of the Bcl-2, CASP-3, and PSMB5 target proteins was performed to explore the probable binding mode. Finally, the 33 newly synthesized compounds were predicted to have good drug-like properties in a theoretical study.
Materials and Methods
The experimental section contains seven main sections as follows:
• Synthesis and Structure Elucidation of the 33 TMPH Compounds
• In Vitro Antiproliferative Activity Evaluation by MTT Assay
• Clonal Formation Assay of MDA-MB-231 Cells
• Hoechst 33 342 Staining of MDA-MB-231 Cells
• Apoptosis Analysis by Annexin V-FITC/PI Staining
• Molecular Docking to Bcl-2, CASP-3, and PSMB5
• Prediction of In Silico Drug-Like Properties
Synthesis and Structure Elucidation of the 33 TMPH Compounds
All solvents and reagents used were obtained from commercial sources and used without further purification. TMP was provided by Aladdin® (Shanghai, China) with a purity of 98% as indicated by HPLC. The progress of the synthetic reactions was monitored by thin-layer chromatography (TLC) on silica gel GF254 plates, and the target products were purified by silica gel column chromatography using a mixture of petroleum ether and acetone as the eluent. The purity of the target compounds was >95% as determined by HPLC (Waters 2695 with a Kromasil C18 column eluted by methanol/water 85:15). The accurate mass was measured using a VG ZAB-HS double-focusing magnetic mass spectrometer. 1H-NMR and 13C-NMR spectra were collected using a Bruker 400 MHz superconducting Fourier transform liquid NMR spectrometer and Avance III 400 MHz liquid NMR spectrometer, using tetramethylsilane and DMSO-d6 as the internal reference and solvent, respectively. The chemical shifts of the protons were recorded in ppm (δ). The structure characterization data for the 33 new TMPH compounds are shown in detail in the Supplementary Material.
In Vitro Antiproliferative Activity Evaluation by MTT Assay
The in vitro antiproliferative activity of 33 TMPH compounds was evaluated by MTT assay (Medica et al., 2022). Five types of TNBC-related cell lines (human breast cancer MCF-7 and MDA-MB-231, mouse breast cancer 4T1, mouse fibroblast L929, and human umbilical vein endothelial HUVEC cell lines) were used. The cell lines were obtained from the Shanghai Institute of Biochemistry and Cell Biology of the Chinese Academy of Sciences (Shanghai, China). The cell lines were cultured in DMEM supplemented with 1% penicillin–streptomycin solution (100×) and 10% FBS at 37°C under a humid atmosphere containing 5% CO2. After cell passage, cryopreservation, and resuscitation, the growth-inhibitory effects against the five cell lines were determined by MTT assay. The 33 TMPH compounds and the reference control drug cisplatin were dissolved in 0.1% DMSO solution. The cell lines were maintained in 96-well plates and subjected to each test compound (0–50 μM) at 37°C for 48 h. After treatment with 1 mg/ml MTT solution for 4 h, the formazan crystals were dissolved in each well. A microplate reader (Varioskan Flash 3001, Thermo Scientific) was used to determine the cell viability at 490 nm, and each experiment was repeated four times. The collected data were expressed as the mean ± SEM. The inhibitory concentration of the 34 compounds at 50% (IC50) was calculated via concentration–inhibition relationship regression by SPSS software.
Clonal Formation Assay of MDA-MB-231 Cells
Human breast cancer MDA-MB-231 cells were cultured in a six-well plate with 1,500 cells per well and incubated for 24 h at 37°C under a humid atmosphere containing 5% CO2. Then, the MDA-MB-231 cells were incubated with compound 12–9 at final concentrations of 0, 0.5, 1.0, and 2.0 μM for 10 days. The treated cells were washed with PBS and fixed with 4% paraformaldehyde for 15 min at room temperature. Then, the end product of the cell colonies was visualized by staining with 0.1% crystal violet for 10 min. The images were photographed using a handy camera, and the number of cell colonies was assessed using open Image J software (National Institutes of Health). Each experiment was repeated three times. A group of >50 cells was defined as one colony formation.
Hoechst 33 342 Staining of MDA-MB-231 Cells
The induction of apoptosis by compound 12–9 was visually evaluated in MDA-MB-231 cells by Hoechst 33 342 staining. The test cells were transferred to six-well plates at a density of 1 × 105 cells. After incubation for 24 h, 2 ml of media containing 2 μM compound 12–9 was used to replace the culture medium. Then, the MDA-MB-231 cells were stained with Hoechst 33 342 solution (10 μg/ml in the culture medium, Beyotime Institute of Biotechnology, Shanghai, China) at 37°C in a dark room for 20 min after incubation with the test compound 12–9. The cells were then washed twice with a serum-free medium to remove the leftover dye. The apoptotic cells stained by Hoechst 33 342 were imaged using CLSM (Carl-Zeiss LSM 710).
Apoptosis Analysis by Annexin V-FITC/PI Staining
Apoptosis induced by TMPH compound 12–9 was evaluated by annexin V-FITC/PI staining. Briefly, MDA-MB-231 cells in the logarithmic growth phase were cultured in a six-well plate and incubated for 24 h at 37°C under a humid atmosphere containing 5% CO2. Then, the cultured cells were incubated with TMPH 12–9 at final concentrations of 0, 0.5, 1.0, and 2.0 μM for 24 h. The incubated cells were washed twice with PBS, digested by trypsin, and centrifuged at 1,000 rpm for 5 min. The collected MDA-MB-231 cells were transferred to a 1.5-ml tube and resuspended in a solution of 200 μl of annexin V-FITC and 5 μl of PI. After incubation in a dark environment (covered with aluminum foil) for 15 min at room temperature, the collected cells were evaluated by flow cytometry (CyFlow®Cube 6, Sysmex). The apoptosis analysis data were shown as a pseudo color map and analyzed by FlowJo software.
Molecular Docking to Bcl-2, CASP-3, and PSMB5
Molecular docking analysis is widely used to evaluate the potential binding affinity and interaction mechanism between a ligand and a target protein (Ma et al., 2021). To further elucidate the mechanism of action (Zhang et al., 2020), compound 12–9 was subjected to flexible receptor docking with the proteins, Bcl-2, CASP-3, and PSMB5, using the molecular docking protocols of the Schrodinger Maestro platform (https://www.schrodinger.com/). The crystal structures obtained by X-ray crystallography with the highest resolution in the form of the lowest value were selected for the docking study. The originated ligands for each target are given here as a reference. The crystal structures of the targets Bcl-2 Bcl-2 (PDB ID: 6GL8), CASP-3 (PDB ID: 1NME), and PSMB5 (PDB ID: 5LEY) were retrieved from RCSB PDB (https://www.pdbus.org/) and subjected to the protein preparation wizard. A glide grid box was then generated around the original ligand inhibitor in the binding pocket of each target protein (Ma et al., 2018). The glide ligand docking module was then used to conduct between the ligand compounds and the proteins Bcl-2, CASP-3, and PSMB5. The binding mode for a given ligand with each target protein was identified by the highest binding energy in the form of a docking score. Only flexibility was taken into account, and the protein was considered to form a semi-rigid pocket. The docking results for the ligand–protein complexes were obtained according to the docking scores, which were calculated using the Schrodinger Maestro scoring function.
Prediction of In Silico Drug-Like Properties
Poor drug-like properties can lead to the failure of a potential drug (Ji, 2021), and the prediction of drug-like properties is becoming more critical in the development of lead compounds (Gupta et al., 2021). Also, 12 mathematical models for the molecular descriptors nHA (number of hydrogen bond acceptors), nHD (number of hydrogen bond donors), TPSA (topological polar surface area), MW (molecular weight), logP (logarithm of the n-octanol/water distribution coefficient), PPB (plasma protein binding), VD (volume distribution), CL (clearance), T1/2 (half-life), and IGC50 (48 h Tetrahymena pyriformis IGC50 value), which characterize the physicochemical, medicinal chemistry, and ADMET properties, were calculated by Schrodinger Canvas and web-based applications of ADMETlab (https://admetmesh.scbdd.com/). The drug-like properties of the 33 TMPH compounds, as well as the TMP reference, were then evaluated.
Results and Discussion
Synthesis and Structure Elucidation of the 33 TMPH Compounds
The general synthetic procedures for the target products are outlined in Scheme 1. The intermediate TMP-COOH was first synthesized by the treatment of the precursor TMP with KMnO4 and HCl in water at 37°C for 24 h. The target compounds linked by an amide chain were synthesized by a reaction with 1-(3-dimethylaminopropyl)-3-ethylcarbodiimide hydrochloride (EDCl) and 4-dimethylaminopyridine (DMAP) in anhydrous CH2Cl2 at room temperature. To explore the effect of the length of the linker, TMP moieties with an 8–12 methylene alkyl chain as a linker were synthesized. All the target compounds were characterized by 1H-NMR, 13C-NMR, and HR ESI-MS. The details of the physical properties and the spectral data are provided in the Supplementary Material.
In Vitro Antiproliferative Activity Evaluation by MTT Assay
The antiproliferative activity of the compounds was investigated against five cell lines (human breast cancer MCF-7 and MDA-MB-231, mouse breast cancer 4T1, mouse fibroblast L929, and human umbilical vein endothelial HUVEC). The ability of the synthesized compounds to inhibit cell proliferation in the five cell lines was evaluated by MTT assay with cisplatin as a positive control. The IC50 values for the antiproliferative activity of the compounds are shown in Table 1. The biological activity of all the TMPH derivatives was higher than that of the original TMP against the tested five cell lines. All the compounds displayed moderate to good inhibition against MDA-MB-231 cells, compared with the reference drug, with IC50 values ranging from 0.84 ± 0.02 to 13.02 ± 0.27 µM. In addition, 42.42% (14/33) of the compounds (compounds 8–3, 8–10, 10–6, 10–9, 10–13, 12–1, 12–2, 12–3, 12–5, 12–6, 12–9, 12–10, 12–12, and 12–13) had broad-spectrum cytotoxic activity in all tested cell lines with IC50 values ranging from 1.61 ± 0.08 to 19.47 ± 0.45 µM. The antiproliferative activity increased with increasing carbon chain length in the flexible linker, and the activity was the highest with a 12-carbon alkyl chain linker. In addition, compounds 10–9, 12–3, and 12–9 had higher activity than cisplatin in the MCF-7, 4T1, and L929 cell lines, and the selective index (SI) values between L929 and MDA-MB-231 [IC50 (L929)/IC50 (MDA-MB-231)] were 1.23, 3.61, and 3.76, respectively. In contrast, the SI value for cisplatin was 2.64. Compound 12–9 with the 12-carbon alkyl chain, 1,12-diaminododecane, displayed notable antiproliferative activity compared with the reference drug cisplatin and the other TMPH compounds, which had IC50 values ranging from 0.84 ± 0.02 to 12.37 ± 0.09 µM against the five cell lines. These results encouraged us to further investigate the possible antitumor mechanisms of TMPH 12–9. Structure–activity relationship analysis suggested that the introduction of a heterocyclic ring increased the antiproliferative activity of the TMPH compounds to a certain extent. The in vitro antiproliferative activity increased with the increasing carbon chain length in the linker. In the derivatives with a 12-carbon chain, the introduction of a chlorine group had no obvious effect (12–10 vs. 12–1). The cytotoxicity toward the MDA-MB-231 cell lines increased with an increasing number of methoxy groups (12–12 vs. 12–11 with three and two methoxy groups, respectively). In addition, the SI value increased with the introduction of a benzimidazole group replacing the benzene group (12–4 vs. 12–1). Compared with compound 12–4, compound 12–9 showed much promising antiproliferative activity in most of the tested cell lines. The structures of 12–4 and 12–9 are very similar, but the position of the carboxylic acid substituent plays a key role in the antiproliferative activity. No obvious differences in the activity in most of the tested cell lines were found between the three compounds with two fused six-membered rings (12–5, 12–6, and 12–13). These results indicated that the introduction of a nitrogen atom into the fused six-membered rings had no appreciable effect on MTT activity. Many TMP derivatives have been synthesized and evaluated with potent antitumor activity, including monocarbonyl TMP–curcumin hybrids (Ai et al., 2016), TMP–betulinic acid hybrids (Xu et al., 2017b), and TMP–rhein derivatives. A TMP dimer linked by decane-1,10-diamine exhibited cytotoxicity in FaDu cells with an IC50 value of 1.36 nM (Wang et al., 2019), and a TMP–vanillic acid amide derivative had displayed high potency with an EC50 value of 17.39 μM (Xu et al., 2017a). In the present study, TMPH derivatives with a flexible alkyl chain were developed with IC50 values in the low micromolar range.
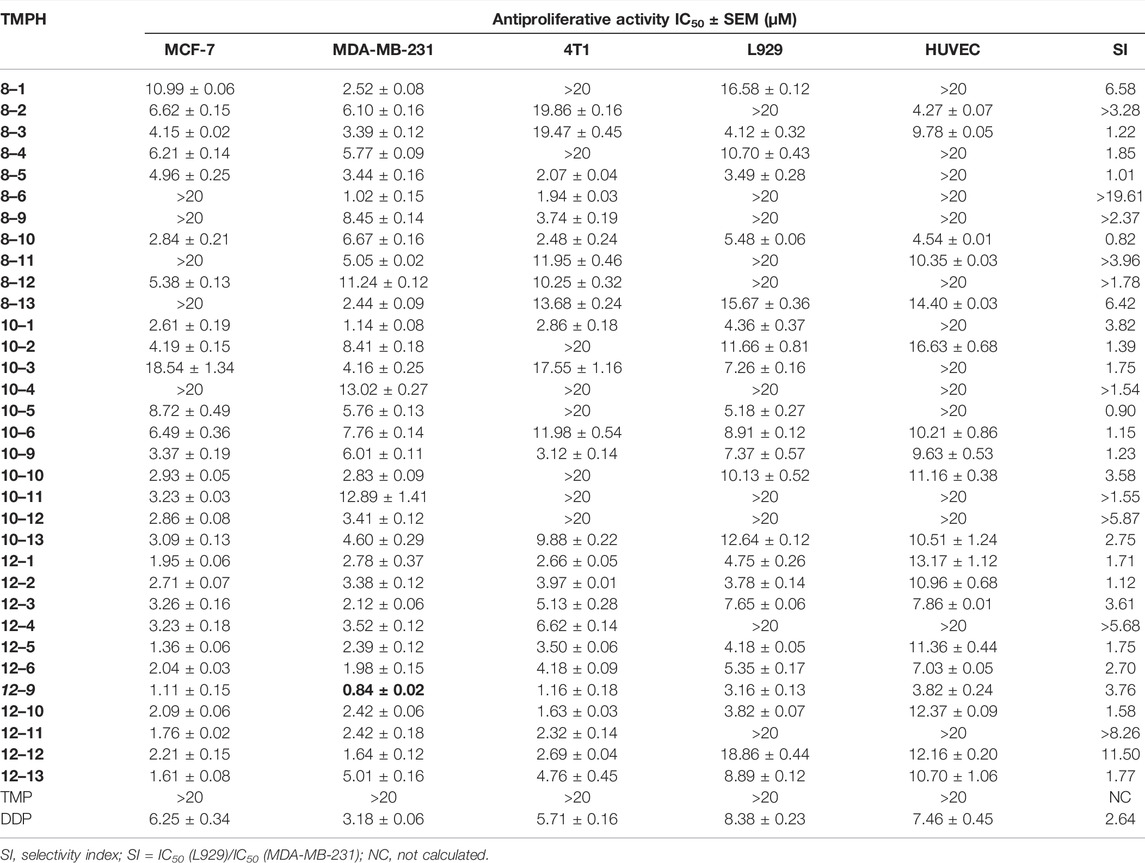
TABLE 1. Cytotoxicity of ligustrazine–heterocyclic derivatives (TMPH) to five kinds of cells by MTT assay.
Clonal Formation Assay of MDA-MB-231 Cells
According to the MTT assay results shown in Table 1, compound 12–9 had the highest antiproliferative activity in vitro, especially in the human breast cancer MDA-MB-231 cell line (IC50 = 0.84 ± 0.02 µM) of all the tested compounds. Thus, compound 12–9 was selected for further investigation in the colony formation assay in MDA-MB-231 cells. As shown in Figure 1, in MDA-MB-231 cells treated with compound 12–9 at concentrations of 0.5, 1.0, and 2.0 μM for 10 days, the colonies formed by cloning were significantly fewer and smaller than the untreated control group. Compound 12–9 also exhibited dose-dependent inhibition of the proliferation of MDA-MB-231 cells. These results indicated that TMPH 12–9 reduced the clonogenic ability of MDA-MB-231 cells and inhibited the proliferation of tumor cells.
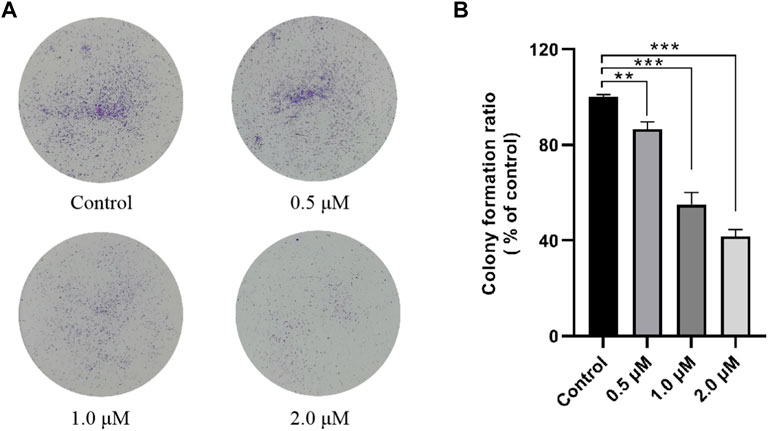
FIGURE 1. Effect of TMPH 12–9 on the colony formation in MDA-MB-231 cells. (A) Representative images of MDA-MB-231 colonies; (B) percentage of colonies compared with that of the control group, n = 3. ∗∗: p < 0.05; ∗∗∗: p < 0.001 versus control.
Hoechst 33 342 Staining of MDA-MB-231 Cells
The ability of compound 12–9 to induce apoptosis in MDA-MB-231 cells was investigated by Hoechst 33 342 staining. MDA-MB-231 cells were allowed to grow in the presence (1.0 μM) or absence of TMPH 12–9 for 48 h. As shown in Figure 2, the untreated MDA-MB-231 cells as the control group exhibited almost negligible apoptotic characteristics with uniform weak blue fluorescence. In contrast, most of the MDA-MB-231 cells in the TMPH 12–9 treatment group appeared shrunken and showed strong blue fluorescence. The aforementioned results suggested that the induction of apoptosis in MDA-MB-231 cells might be the cause of the cell growth inhibition observed for compound 12–9.
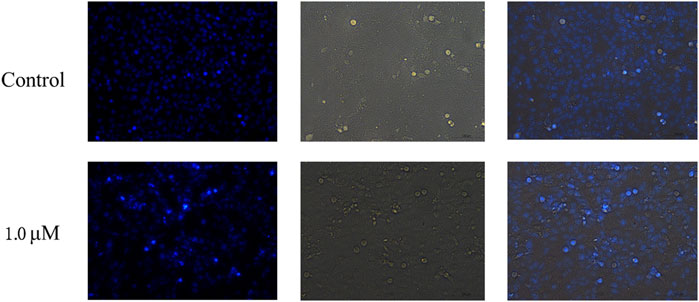
FIGURE 2. Fluorescence microscopy images of MDA-MB-231 cells with Hoechst staining: the control group and treatment group with 1.0 μM TMPH 12–9.
Apoptosis Analysis by Annexin V-FITC/PI Staining
The ability of compound 12–9 to induce apoptosis was evaluated by annexin V-FITC/PI flow cytometry. Human breast cancer MDA-MB-231 cells were allowed to grow in the presence (0.5, 1.0, and 2.0 μM) or absence of 12–9 for 48 h and then were co-stained with PI and annexin-V/FITC chromogenic agents. The quadrant images in Figure 3 represent four different cell states: Q1, necrotic cells (annexin V−/PI+); Q2, late apoptotic or necrotic cells (annexin V+/PI+); Q3, early apoptotic cells (annexin V+/PI−); and Q4, living cells (annexin V−/PI−). As shown in Figure 3, after treatment with different concentrations of compound 12–9 for 48 h, the apoptotic ratio in MDA-MB-231 cells increased gradually from 13.7% for the reference control group to 23.96% (0.5 μM 12–9), 32.64% (1.0 μM 12–9), and 46.9% (2.0 μM 12–9). These data indicated that TMPH compound 12–9 effectively induced apoptosis in MDA-MB-231 cells in a dose-dependent manner, from 0.5 to 2.0 μM TMPH 12–9. This result was consistent with the Hoechst 33 342 staining results for apoptosis analysis.
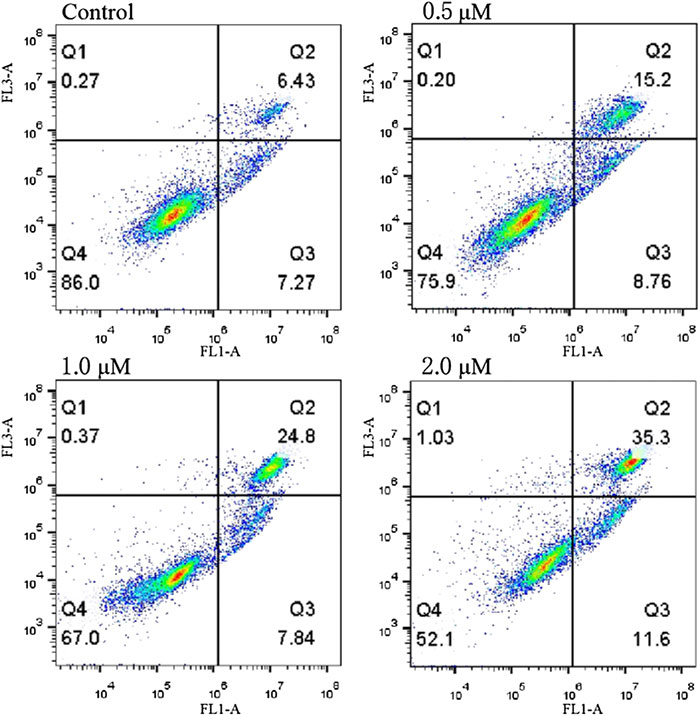
FIGURE 3. Annexin V-FITC and PI staining to evaluate apoptosis in MDA-MB-231 cells following compound 12–9 treatment. MDA-MB-231 cells were treated with MDA-MB-231 (0, 0.5, 1.0, and 2.0 μM, for 48 h), stained by annexin V-FITC and PI, and analyzed using flow cytometry.
Molecular Docking to Bcl-2, CASP-3, and PSMB5
Compound 12–9 and the reference control TMP were docked with the Bcl-2, CASP-3, and PSMB5 receptors using molecular docking protocols, and the binding modes between the ligand and protein were analyzed visually. The crystal structures of Bcl-2 (PDB ID: 6GL8), CASP-3 (PDB ID: 1NME), and PSMB5 (PDB ID: 5LEY) were downloaded into the Schrodinger Maestro platform and subjected to the protein preparation wizard using default parameters. The binding mode of compound 12–9 is shown in Figure 4. The docking scores of 12–9 and TMP with Bcl-2, CASP-3, and PSMB5 were −4.495 and −4.226, −5.410 and −4.331, and −7.925 and −5.509, respectively. The scoring functions indicated that 12–9 had a greater affinity with the targets than TMP, which was used as a reference. Visual inspection of the results in Figure 4 indicated that compound 12–9 was embedded into the binding pocket of each antitumor target protein. TMPH 12–9 was bound firmly at the active site of each target receptor by two conventional hydrogen bonds. In addition to these hydrogen bonds, non-bonding amino acid residues were surrounded by the ligand 12–9 and each target complex surface. These results indicated that the synthesized ligustrazine derivative 12–9 may elicit anticancer effects by activating the Bcl-2, CASP-3 apoptotic pathway, and PSMB5 proliferation pathway. Moreover, the cell-specific response of compound 12–9 can be partly explained by the human MDA-MB-231 breast carcinoma cells completely.
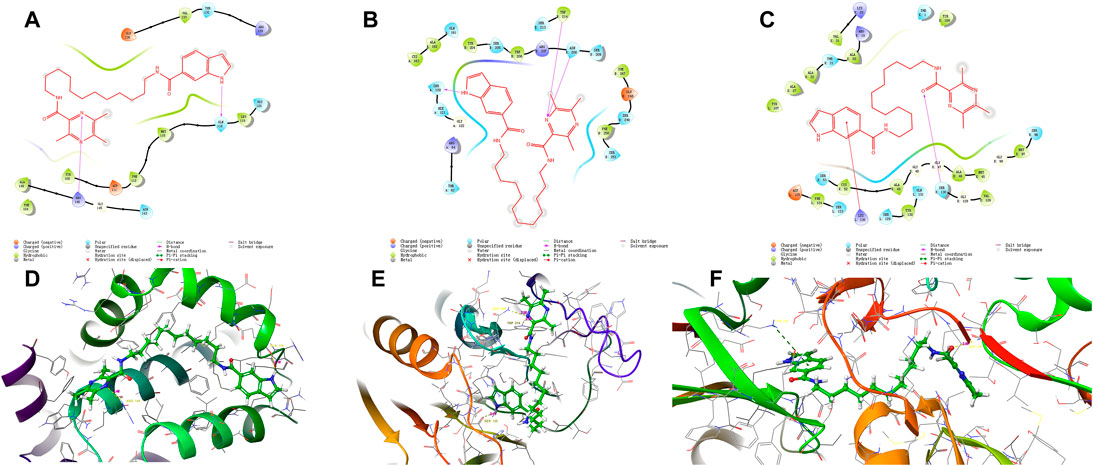
FIGURE 4. Visual presentation of the interaction of the crystal structure of compound 12–9 (shown as stick) with its docked pose of Bcl-2, CASP-3, and PSMB5 and (represented as a secondary structure in green) performed by the Schrodinger. [(A–C) represented as the ligand interaction diagram with Bcl-2, CASP-3, and PSMB5 respectively. (D–F) represented as binding interactions with Bcl-2, CASP-3, and PSMB5, respectively.].
Prediction of In Silico Drug-Like Properties
The prediction of drug-like properties is becoming highly desirable because most drugs in development fail in clinical trials. The drug-like properties of the 33 newly synthesized TMPH compounds were evaluated using the Schrodinger Canvas and ADMETlab 2.0 platforms. Also, 12 key molecular descriptors for the physicochemical, medicinal chemistry, and ADMET properties were calculated to evaluate the drug-like properties, as shown in Table 2. The MWs of most of the compounds were under 500 Da, except for six compounds (8–12, 12–3, 12–5, 12–6, 12–10, and 12–13). The logP values of 10 compounds (8–1, 8–2, 8–4, 8–5, 8–6, 8–9, 8–12, 10–6, 10–11, and 10–12) were <5. The 33 TMPH compounds had 2–9 hydrogen bond acceptors and 0–3 hydrogen bond donors. These results indicated that only 3 of the 33 TMPH compounds (12–3, 12–6, and 12–13) violated two rules out of Lipinski’s rule of five (nHA ≤ 10, nHD ≤ 5, MW ≤ 500, and logP ≤ 5). Except for the logP values, 78.79% (26/33) of the TMPH compounds met the other requirements in Lipinski’s rule of five. Therefore, the structural modification should be conducted to optimize the logP value. According to the Pfizer rule, compounds with a high logP value (>3) and low TPSA (<75) are likely to be toxic. None of the 33 TMPH compounds had logP values > 3 indicating acceptable toxicity. In addition, none of the 33 TMPH compounds conformed to the GSK rule (MW ≤ 400, logP ≤4). The T1/2 and CL values were in the range of 0.02–0.5 h and 3.51–4.92, respectively, suggesting that the compounds would be rapidly metabolized. The IGC50 values of the compounds were in the range of 4.39–5.4 and 4.22–5.91, implying low toxicity. These results suggested that the synthesized compounds had good drug-likeness, as shown in Table 2. This information might be useful for designing new drugs with favorable drug-like properties for the treatment of TNBC.
Conclusion
A series of 33 TMPH compounds consisting of a TMP derivative linked to a pharmaco-active heterocycle by a flexible alkyl chain were rationally designed and synthesized as anticancer agents that targeted PSMB5 with good IC50 values in the low micromolar range. Cell-based antiproliferative assays led to the identification of compound 12–9, which had IC50 values ranging from 0.84 ± 0.02 to 12.37 ± 0.09 µM in the five tested cell lines. The antitumor effects of TMPH 12–9 were further evaluated by plate cloning, Hoechst 333 42 staining, and annexin V-FITC/PI staining. TMPH 12–9 inhibited the proliferation and apoptosis of breast cancer cells. Molecular docking of compound 12–9 into the active site of Bcl-2, CASP-3, and PSMB5 proteins was performed to explore the probable binding mode. Finally, the 33 newly synthesized compounds were predicted to have good drug-like properties in a theoretical study.
In conclusion, in the present study, TMPH derivatives were systematically designed and synthesized, and the antitumor proliferation and apoptotic activities, molecular docking, and prediction of drug-like properties were analyzed. The results showed that compound 12–9 had good antiproliferative and apoptosis-inducing effects in the human breast cancer MDA-MB-231 cell line and had good drug-like properties in a theoretical study. This study provides a foundation for the research and development of effective bioactive components from Chinese natural medicines as candidate antitumor drugs.
Data Availability Statement
The original contributions presented in the study are included in the article/Supplementary Material; further inquiries can be directed to the corresponding authors.
Author Contributions
SM and GH designed and guided the whole experiment. NZ, JW, JH, BL, FZ, PW, and SL performed this experiment and wrote the draft. SM, GH, and JH implemented the modification of this manuscript to improve its quality. All authors read and approved the final manuscript.
Funding
This study was financially supported by the Education Bureau of Anhui Province (KJ2020ZD011 and gxbjZD2020081), the CAMS Innovation Fund for Medical Sciences (2021-I2M-1-052), the Capital Health Research and Development of Special Fund Program (2022-1-4041), and the Tianjin Key Technology R&D Program (20YFZCSY00570).
Conflict of Interest
The authors declare that the research was conducted in the absence of any commercial or financial relationships that could be construed as a potential conflict of interest.
The handling editor CF declared a past collaboration with the author SM.
Publisher’s Note
All claims expressed in this article are solely those of the authors and do not necessarily represent those of their affiliated organizations, or those of the publisher, the editors, and the reviewers. Any product that may be evaluated in this article, or claim that may be made by its manufacturer, is not guaranteed or endorsed by the publisher.
Supplementary Material
The Supplementary Material for this article can be found online at: https://www.frontiersin.org/articles/10.3389/fchem.2022.941367/full#supplementary-material
References
Adwal, A., Kalita-De Croft, P., Shakya, R., Lim, M., Kalaw, E., Taege, L. D., et al. (2020). Tradeoff Between Metabolic I-Proteasome Addiction and Immune Evasion in Triple-Negative Breast Cancer. Life Sci. Alliance 3, e201900562. doi:10.26508/lsa.201900562
Ai, Y., Zhu, B., Ren, C., Kang, F., Li, J., Huang, Z., et al. (2016). Discovery of New Monocarbonyl Ligustrazine-Curcumin Hybrids for Intervention of Drug-Sensitive and Drug-Resistant Lung Cancer. J. Med. Chem. 59, 1747–1760. doi:10.1021/acs.jmedchem.5b01203
Allmeroth, K., Horn, M., Kroef, V., Miethe, S., Muller, R. U., and Denzel, M. S. (2021). Bortezomib Resistance Mutations in PSMB5 Determine Response to Second-Generation Proteasome Inhibitors in Multiple Myeloma. Leukemia 35, 887–892. doi:10.1038/s41375-020-0989-4
Altei, W. F., Pachane, B. C., Souza, C., Marques, M. M. C., and Selistre-De-Araujo, H. (2022). New Insights into the Discovery of Drugs for Triple-Negative Breast Cancer Metastasis. Expert Opin. Drug Discov. 17, 365–376. doi:10.1080/17460441.2022.2039619
An, H., Heo, J. S., Kim, P., Lian, Z., Lee, S., Park, J., et al. (2021). Tetraarsenic Hexoxide Enhances Generation of Mitochondrial ROS to Promote Pyroptosis by Inducing the Activation of Caspase-3/GSDME in Triple-Negative Breast Cancer Cells. Cell Death Dis. 12, 159. doi:10.1038/s41419-021-03454-9
Bian, Y., Yang, L., Sheng, W., Li, Z., Xu, Y., Li, W., et al. (2021). Ligustrazine Induces the Colorectal Cancer Cells Apoptosis via P53-Dependent Mitochondrial Pathway and Cell Cycle Arrest at the G0/G1 Phase. Ann. Palliat. Med. 10, 1578–1588. doi:10.21037/apm-20-288
Chen, K., Rekep, M., Wei, W., Wu, Q., Xue, Q., Li, S., et al. (2018). Quercetin Prevents In Vivo and In Vitro Myocardial Hypertrophy Through the Proteasome-GSK-3 Pathway. Cardiovasc Drugs Ther. 32, 5–21. doi:10.1007/s10557-018-6771-4
Chen, H., Yang, J., Yang, Y., Zhang, J., Xu, Y., and Lu, X. (2021). The Natural Products and Extracts: Anti-Triple-Negative Breast Cancer In Vitro. Chem. Biodivers. 18, e2001047. doi:10.1002/cbdv.202001047
Chen, C. H., Liu, Y. H., Eskandari, A., Ghimire, J., Lin, L. C., Fang, Z. S., et al. (2022). Integrated Design of a Membrane-Lytic Peptide-Based Intravenous Nanotherapeutic Suppresses Triple-Negative Breast Cancer. Adv. Sci. (Weinh) 9, e2105506. doi:10.1002/advs.202105506
Dwivedi, V., Yaniv, K., and Sharon, M. (2021). Beyond Cells: The Extracellular Circulating 20S Proteasomes. Biochim. Biophys. Acta Mol. Basis Dis. 1867, 166041. doi:10.1016/j.bbadis.2020.166041
El-Arabey, A. A., and Abdalla, M. (2022). The Role of GATA3 in the Metastasis of Triple-Negative Breast Cancer and High-Grade Serous Ovarian Cancer. Hum. Cell 35, 1298–1300. doi:10.1007/s13577-022-00706-4
Guan, X., Meng, X., Zhu, K., Kai, J., Liu, Y., Ma, Q., et al. (2022). MYSM1 Induces Apoptosis and Sensitizes TNBC Cells to Cisplatin via RSK3-Phospho-BAD Pathway. Cell Death Discov. 8, 84. doi:10.1038/s41420-022-00881-1
Gupta, K., Chawla, P. A., and Sharma, D. (2021). Synthetic Strategies Towards Safer NSAIDs Through Prodrug Approach: A Review. Mini Rev. Med. Chem. 21, 2065–2102. doi:10.2174/1389557521666201231140554
Jaaks, P., Coker, E. A., Vis, D. J., Edwards, O., Carpenter, E. F., Leto, S. M., et al. (2022). Effective Drug Combinations in Breast, Colon and Pancreatic Cancer Cells. Nature 603, 166–173. doi:10.1038/s41586-022-04437-2
Ji, X. (2021). Prodrug Strategies for Critical Drug Developability Issues: Part I. Curr. Top. Med. Chem. 21, 2155–2156. doi:10.2174/156802662124211104100228
Jia, H., Couto-Rodriguez, R. L., Gal, D., Mondragon, P., Wassel, P. C., Yu, D., et al. (2021). Expression and Tandem Affinity Purification of 20S Proteasomes and Other Multisubunit Complexes in Haloferax Volcanii. Methods Enzymol. 659, 315–326. doi:10.1016/bs.mie.2021.08.002
Jiang, M., Cui, B. W., Wu, Y. L., Nan, J. X., and Lian, L. H. (2021). Genus Gentiana: A Review on Phytochemistry, Pharmacology and Molecular Mechanism. J. Ethnopharmacol. 264, 113391. doi:10.1016/j.jep.2020.113391
Kataura, T., Tashiro, E., Nishikawa, S., Shibahara, K., Muraoka, Y., Miura, M., et al. (2021). A Chemical Genomics-Aggrephagy Integrated Method Studying Functional Analysis of Autophagy Inducers. Autophagy 17, 1856–1872. doi:10.1080/15548627.2020.1794590
Lang, S. J., Schmiech, M., Hafner, S., Paetz, C., Steinborn, C., Huber, R., et al. (2019). Antitumor Activity of an Artemisia Annua Herbal Preparation and Identification of Active Ingredients. Phytomedicine 62, 152962. doi:10.1016/j.phymed.2019.152962
Li, G. L., Wang, P. L., Xu, X., Lin, J. X., Chu, F. H., Song, J. X., et al. (2014). Synthesis and Protective Effect of Ligustrazine Intermediates Against CoCl2-Induced Neurotoxicity in Differentiated PC12 Cell. Zhongguo Zhong Yao Za Zhi 39, 2679–2683.
Liu, L., Fu, Y., Zheng, Y., Ma, M., and Wang, C. (2020). Curcumin Inhibits Proteasome Activity in Triple-Negative Breast Cancer Cells Through Regulating p300/miR-142-3p/PSMB5 Axis. Phytomedicine 78, 153312. doi:10.1016/j.phymed.2020.153312
Luo, Y., Wu, W., Zha, D., Zhou, W., Wang, C., Huang, J., et al. (2021). Synthesis and Biological Evaluation of Novel Ligustrazine-Chalcone Derivatives as Potential Anti-Triple Negative Breast Cancer Agents. Bioorg Med. Chem. Lett. 47, 128230. doi:10.1016/j.bmcl.2021.128230
Ma, S. T., Feng, C. T., Xiong, Y. X., Zhang, X. L., Miao, C. G., and Yu, H. (2018). In Silico System Pharmacology for the Potential Bioactive Ingredients Contained in Xingnaojing Injection () and its Material Basis for Sepsis Treatment. Chin. J. Integr. Med. 24, 944–949. doi:10.1007/s11655-017-2421-0
Ma, S. T., Zhang, N., Hong, G., Feng, C. T., Hong, S. W., and Dai, G. L. (2021). Unraveling the Action Mechanism of Buyang Huanwu Tang (BYHWT) for Cerebral Ischemia by Systematic Pharmacological Methodology. Comb. Chem. High. Throughput Screen 24, 1114–1125. doi:10.2174/1386207323666200901100529
Mao, Q., Liu, S., Lv, M., Sun, Y., Zhang, C., and Li, L. (2021). Nomogram for Predicting Overall Survival and Assessing the Survival Benefit of Adjuvant Treatment in pT1-2N0M0 Triple-Negative Breast Cancer: A Surveillance, Epidemiology, and End Results-Based Study. Front. Oncol. 11, 663621. doi:10.3389/fonc.2021.663621
Medica, A. J., Gibb, Z., Sheridan, A., Harrison, N., and Aitken, R. J. (2022). Causative Mechanisms and Functional Correlates of MTT Reduction in Stallion Spermatozoa. Reproduction 163, 341–350. doi:10.1530/REP-21-0464
Mortezaee, K., Salehi, E., Mirtavoos-Mahyari, H., Motevaseli, E., Najafi, M., Farhood, B., et al. (2019). Mechanisms of Apoptosis Modulation by Curcumin: Implications for Cancer Therapy. J. Cell Physiol. 234, 12537–12550. doi:10.1002/jcp.28122
Nabil, G., Alzhrani, R., Alsaab, H. O., Atef, M., Sau, S., Iyer, A. K., et al. (2021). CD44 Targeted Nanomaterials for Treatment of Triple-Negative Breast Cancer. Cancers (Basel) 13, 898. doi:10.3390/cancers13040898
Oerlemans, R., Franke, N. E., Assaraf, Y. G., Cloos, J., Van Zantwijk, I., Berkers, C. R., et al. (2008). Molecular Basis of Bortezomib Resistance: Proteasome Subunit Beta5 (PSMB5) Gene Mutation and Overexpression of PSMB5 Protein. Blood 112, 2489–2499. doi:10.1182/blood-2007-08-104950
Olivier, T., and Prasad, V. (2022). Sacituzumab Govitecan in Metastatic Triple Negative Breast Cancer (TNBC): Four Design Features in the ASCENT Trial Potentially Favored the Experimental Arm. Transl. Oncol. 15, 101248. doi:10.1016/j.tranon.2021.101248
Oubella, A., El Mansouri, A. E., Fawzi, M., Bimoussa, A., Laamari, Y., Auhmani, A., et al. (2021). Thiazolidinone-Linked1,2,3-triazoles with Monoterpenic Skeleton as New Potential Anticancer Agents: Design, Synthesis and Molecular Docking Studies. Bioorg. Chem. 115, 105184. doi:10.1016/j.bioorg.2021.105184
Raafat Elsayed, A. A., Al-Marsoummi, S., Vomhof-Dekrey, E. E., and Basson, M. D. (2022). SLFN12 Over-Expression Sensitizes Triple Negative Breast Cancer Cells to Chemotherapy Drugs and Radiotherapy. Cancer Genomics Proteomics 19, 328–338. doi:10.21873/cgp.20323
Ramdas, P., Radhakrishnan, A. K., Abdu Sani, A. A., Kumari, M., Anandha Rao, J. S., and Abdul-Rahman, P. S. (2019). Advancing the Role of Gamma-Tocotrienol as Proteasomes Inhibitor: A Quantitative Proteomic Analysis of MDA-MB-231 Human Breast Cancer Cells. Biomolecules 10, 19. doi:10.3390/biom10010019
Rut, W., and Drag, M. (2016). Human 20S Proteasome Activity Towards Fluorogenic Peptides of Various Chain Lengths. Biol. Chem. 397, 921–926. doi:10.1515/hsz-2016-0176
Sammut, S. J., Crispin-Ortuzar, M., Chin, S. F., Provenzano, E., Bardwell, H. A., Ma, W., et al. (2022). Multi-Omic Machine Learning Predictor of Breast Cancer Therapy Response. Nature 601, 623–629. doi:10.1038/s41586-021-04278-5
Shen, X., Lei, J., and Du, L. (2020). miR-31-5p May Enhance the Efficacy of Chemotherapy with Taxol and Cisplatin in TNBC. Exp. Ther. Med. 19, 375–383. doi:10.3892/etm.2019.8191
Wang, J., Hong, G., Li, G., Wang, W., and Liu, T. (2019). Novel Homo-Bivalent and Polyvalent Compounds Based on Ligustrazine and Heterocyclic Ring as Anticancer Agents. Molecules 24, 4505. doi:10.3390/molecules24244505
Wang, B., Feng, W., Wang, J., Dong, Y., Liu, Y., Yao, Y., et al. (2021). Discovery of Potent and Selective Bcl-2 Inhibitors with Acyl Sulfonamide Skeleton. Bioorg. Med. Chem. 47, 116350. doi:10.1016/j.bmc.2021.116350
Wei, W., Zou, Y., Jiang, Q., Zhou, Z., Ding, H., Yan, L., et al. (2018). PSMB5 Is Associated with Proliferation and Drug Resistance in Triple-Negative Breast Cancer. Int. J. Biol. Markers 33, 102–108. doi:10.5301/ijbm.5000283
Xie, H. J., Zhao, J., Zhuo-Ma, D., Zhan-Dui, N., Er-Bu, A., and Tsering, T. (2019). Inhibiting Tumour Metastasis by DQA Modified Paclitaxel Plus Ligustrazine Micelles in Treatment of Non-Small-Cell Lung Cancer. Artif. Cells Nanomed. Biotechnol. 47, 3465–3477. doi:10.1080/21691401.2019.1653900
Xiu, M., Zhang, P., Wang, X., Fan, Y., Li, Q., Li, Q., et al. (2022). Survival Outcomes for Dose-Dense Paclitaxel Plus Carboplatin Neoadjuvant vs Standard Adjuvant Chemotherapy in Stage II to III Triple-Negative Breast Cancer: A Prospective Cohort Study with Propensity-Matched Analysis. Int. J. Cancer 151, 578–589. doi:10.1002/ijc.34022
Xu, B., Xu, X., Zhang, C., Zhang, Y., Wu, G., Yan, M., et al. (2017a). Synthesis and Protective Effect of New Ligustrazine-Vanillic Acid Derivatives Against CoCl2-Induced Neurotoxicity in Differentiated PC12 Cells. Chem. Cent. J. 11, 20. doi:10.1186/s13065-017-0250-z
Xu, B., Yan, W. Q., Xu, X., Wu, G. R., Zhang, C. Z., Han, Y. T., et al. (2017b). Combination of Amino Acid/Dipeptide with Ligustrazine-Betulinic Acid as Antitumor Agents. Eur. J. Med. Chem. 130, 26–38. doi:10.1016/j.ejmech.2017.02.036
Yang, Z., Zhang, Q., Yu, L., Zhu, J., Cao, Y., and Gao, X. (2021). The Signaling Pathways and Targets of Traditional Chinese Medicine and Natural Medicine in Triple-Negative Breast Cancer. J. Ethnopharmacol. 264, 113249. doi:10.1016/j.jep.2020.113249
Yang, X., Cao, D., Ma, W., Gao, S., Wen, G., and Zhong, J. (2022). Wnt Signaling in Triple-Negative Breast Cancers: Its Roles in Molecular Subtyping and Cancer Cell Stemness and its Crosstalk with Non-Coding RNAs. Life Sci. 300, 120565. doi:10.1016/j.lfs.2022.120565
Yoshida, T., Ri, M., Kanamori, T., Aoki, S., Ashour, R., Kinoshita, S., et al. (2018). Potent Antitumor Activity of a Syringolin Analog in Multiple Myeloma: A Dual Inhibitor of Proteasome Activity Targeting Beta2 and Beta5 Subunits. Oncotarget 9, 9975–9991. doi:10.18632/oncotarget.24160
Zhang, N., Wang, J., Sheng, A., Huang, S., Tang, Y., Ma, S., et al. (2020). Emodin Inhibits the Proliferation of MCF-7 Human Breast Cancer Cells Through Activation of Aryl Hydrocarbon Receptor (AhR). Front. Pharmacol. 11, 622046. doi:10.3389/fphar.2020.622046
Zhang, D., You, Y., Xu, Y., Cheng, Q., Xiao, Z., Chen, T., et al. (2022a). Facile Synthesis of Near-Infrared Responsive On-Demand Oxygen Releasing Nanoplatform for Precise MRI-Guided Theranostics of Hypoxia-Induced Tumor Chemoresistance and Metastasis in Triple Negative Breast Cancer. J. Nanobiotechnol. 20, 104. doi:10.1186/s12951-022-01294-z
Zhang, P., Qin, C., Liu, N., Zhou, X., Chu, X., Lv, F., et al. (2022b). The Programmed Site-Specific Delivery of LY3200882 and PD-L1 siRNA Boosts Immunotherapy for Triple-Negative Breast Cancer by Remodeling Tumor Microenvironment. Biomaterials 284, 121518. doi:10.1016/j.biomaterials.2022.121518
Keywords: ligustrazine–heterocyclic (TMPH) derivatives, triple-negative breast cancer, antitumor, proliferation, apoptosis
Citation: Ma S, Zhang N, Hou J, Liu S, Wang J, Lu B, Zhu F, Wei P, Hong G and Liu T (2022) Synthesis and Discovery of Ligustrazine–Heterocycle Derivatives as Antitumor Agents. Front. Chem. 10:941367. doi: 10.3389/fchem.2022.941367
Received: 11 May 2022; Accepted: 07 June 2022;
Published: 25 July 2022.
Edited by:
Sheng-xiang Yang, Zhejiang Agriculture and Forestry University, ChinaReviewed by:
Lifeng Han, Tianjin University of Traditional Chinese Medicine, ChinaDianlei Wang, Anhui University of Chinese Medicine, China
Lin Li, Northwestern Polytechnical University, China
Copyright © 2022 Ma, Zhang, Hou, Liu, Wang, Lu, Zhu, Wei, Hong and Liu. This is an open-access article distributed under the terms of the Creative Commons Attribution License (CC BY). The use, distribution or reproduction in other forums is permitted, provided the original author(s) and the copyright owner(s) are credited and that the original publication in this journal is cited, in accordance with accepted academic practice. No use, distribution or reproduction is permitted which does not comply with these terms.
*Correspondence: Ge Hong, aG9uZ2dlQGJtZS5wdW1jLmVkdS5jbg==; Tianjun Liu, bGl1dGpAYm1lLnB1bWMuZWR1LmNu
†These authors have contributed equally to this work