- 1Medical College, Jinggangshan University, Jiʼan, China
- 2Jiʼan Key Laboratory of Personalized Drug Research of Neuropsychiatric Diseases, Jiʼan, China
- 3Center for Evidence Based Medical and Clinical Research, First Affiliated Hospital of Gannan Medical University, Ganzhou, China
Epilepsy, a severe brain disease affecting a large population, is treated mainly by antiepileptic drugs (AEDs). However, toxicity, intolerance, and low efficiency of the available AEDs have prompted the continual attempts in the discovery of new AEDs. In this study, we discovered a skeleton of triazolopyrimidine for the development of new AEDs. The design, synthesis, in vivo anticonvulsant activity evaluation of triazolopyrimidines (3a–3i and 6a–6e), and pyrazolopyrimidines (4a–4i) are reported. We found that most triazolopyrimidines showed anticonvulsive activity in the maximal electroshock (MES) and pentetrazol (PTZ)-induced seizure models. On the contrary, pyrazolopyrimidines (4a–4i) showed weak or no protective effects. Among the tested derivatives, compound 6d, holding a median effective dose (ED50) of 15.8 and 14.1 mg/kg against MES and PTZ-induced seizures, respectively, was found to be the most potent one. Moreover, the protection index (PI) value of 6d was significantly higher than that of the available AEDs such as valproate, carbamazepine, and diazepam. The antiepileptic efficacy of compound 6d was also observed in the 3-mercaptopropionic acid and bicuculline-induced seizure models. Antagonistic effects of flumazenil and 3-MP for the anticonvulsive activity of 6d and also the radioligand-binding assay confirmed the involvement of GABA receptors, at least benzodiazepine (BZD) receptor, in the anticonvulsant activity of compound 6d. The docking study of compounds 4e and 6d with GABAA receptor confirmed and explained their affinity to the BZD receptors.
1 Introduction
Epilepsy is a kind of common brain disease accompanied by repeated and unprovoked seizures. As one of the most common CNS disorders, it affects individuals of any age, any gender, and any ethnicity. About 3–4% of people in developed countries encountered this disease during their lifetime. For people in developing countries, this proportion is higher (Beghi and Hesdorffer, 2014). Epilepsy has a deleterious impact on society, economy, and specifically the physical and psychological well-being of patients (Perucca et al., 2018). According to the Global Burden of Disease Study (GBD) 2019, idiopathic epilepsy was ranked 20th in respect of disability-adjusted life years (DALYs) among 369 health conditions (GBD 2019 Diseases and Injuries Collaborators, 2020).
Antiepileptic drugs (AEDs) are the main method of epilepsy treatment. About two-thirds of patients with epilepsy can achieve seizure freedom. Since the 1990s, numbers of second-generation AEDs and third-generation AEDs (developed after 2000) have been introduced (Figure 1), expanding the opportunity for individual therapy for epilepsy patients. Some of them offer advantages in terms of favorable pharmacokinetics, fewer drug interactions, less adverse effects, and improved tolerability (Vidaurre and Herbst, 2019). Nevertheless, they did not considerably change the overall proportion of seizure-protected patients with epilepsy. Therefore, finding novel AEDs with high efficiency and low toxicity is still the focus of current AED therapy.
Triazole refers to a five-membered heterocycle with the molecular formula C2H3N3. It can be considered as an isostere of pyrazole, thiazole, imidazole, and oxazole. The electron richness and aromaticity of triazole enable it to freely bind with a wide range of biomacromolecules by interactions of pi–pi bonds, H bonds, and ion-dipole bonds. Therefore, it has been widely employed in various fields, especially in pharmaceutical research. Many drugs (such as triazolam, alprazolam, rufinamide, fluconazole, itraconazole, lamtidine, ribavirin, brassinazole, and sitagliptin) assembling the triazole moiety have already been used in clinics for the treatment of various diseases. Moreover, a terrific amount of triazole-contained derivatives have been designed and prepared to discover new anticonvulsants (Song and Deng, 2018), anti-Alzheimer (Xu et al., 2019), anticancer (Wen et al., 2020), antibacterial (Gao et al., 2019), antifungal (Peyton et al., 2015), and anti-inflammatory agents (Zhou and Wang, 2012).
Diazepam, a benzodiazepine drug, is often used to treat anxiety disorders, alcohol withdrawal syndrome, benzodiazepine withdrawal syndrome, seizures, and insomnia (Calcaterra and Barrow, 2014). The efficacy of diazepam comes from the activation of γ-aminobutyric acid (GABA) after it binds to benzodiazepine receptors (Riss et al., 2008). It is the top choice for the epileptic status to receive the intravenous or rectal administration of diazepam. Eighty percent of cases with different forms of epileptic status can be suppressed, and the effective proportion increases for generalized seizures (Loiseau, 1983). However, the side effects of diazepam including drowsiness, motor coordination disorders, and drug dependence limit its application in the clinics (Wu et al., 2016).
Based on these facts, a series of 7-substituted-[1,2,4]triazolo[1,5-α]pyrimidine derivatives (3a–3i) were designed and synthesized to develop new anticonvulsant agents in this study. The rationale behind this design refers to the similarity between the target compounds 3a–3i and diazepam (Figure 2). The target compounds have the requirements for binding to benzodiazepine (BZD) receptors 1) an aromatic ring A, 2) a coplanar proton-accepting group B, and 3) a non-coplanar aromatic ring C. With an intention, the designed compounds act as diazepam to display anticonvulsant activity through binding to BZD receptors. In addition, the triazole moiety in the compounds 3a–3i was replaced by pyrazole to give bioisosteric 4a–4i. These bioisosteres, without the proton-accepting group in a suitable position, were designed to enrich the structure–activity relationships (SARs) and verify the rationality of the aforementioned design. To obtain more SARs around the triazole derivatives, some triazole derivatives (6a–6e) with higher hydrophobicity were also prepared.
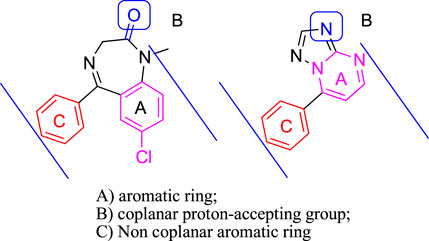
FIGURE 2. Pharmacodynamic characteristics of benzodiazepine site agonists (diazepam as a representative) and compounds reported herein (3a as a representative).
2 Materials and Methods
2.1 Chemical Part
2.1.1 Instruments and Reagents
Melting points were determined in open capillary tubes and were uncorrected. 1H NMR and 13C NMR spectra were measured using an AV-300 spectrometer (Bruker, Switzerland). High-resolution mass spectra were recorded using a MALDI-TOF/TOF mass spectrometer (Bruker Daltonik, Germany). The majority of chemicals were purchased from Aldrich Chemical Corporation (St Louis, United States).
2.1.2 Synthesis of (E)-3-(Dimethylamino)-1-Phenylprop-2-en-1-Ones (2a–2i)
Considering compound 2a as an example: acetophenone (1.00 g, 8 mmol) and DMF-DMA (1.50 g, 13 mmol) were mixed and stirred at 100°C for about 10 h. The reaction was monitored by TLC (30% ethyl acetate in petroleum ether). After the reaction was completed and cooled to about 40°C, 10 ml of petroleum ether was added and stirred until it was completely dissolved. The mixture was placed in a fridge to maintain at 4°C overnight. The solid precipitated was filtered and dried to obtain compound 2a. Mp 92°C–94°C, yield 77.4%. 1H-NMR (CDCl3, 400 MHz): δ 2.92 (s, 3H, NCH3), 3.13 (s, 3H, NCH3), 5.73 (d, 1H, J = 12.3, =CH), 7.81 (d, 1H, J = 12.3 Hz, =CH), 7.39–7.48 (m 3H, Ph-H), and 7.89–7.92 (m 2H, Ph-H). 13C-NMR (CDCl3, 101 MHz): δ 188.7, 154.3, 140.5, 130.9, 128.1, 127.5, 92.2, 45.1, and 37.3. ESI-HRMS calculated for C11H14NO+ ([M + H]+): 176.1070; found: 176.1074. Compounds 2b–2i were prepared from other substituted acetophenones under the same conditions.
2.1.3 Synthesis of 7-Substituted-[1,2,4]Triazolo[1,5-a]Pyrimidines (3a-3i)
Considering compound 3a as an example: compound 2a (0.7 g, 4 mmol), 3-amino-1,2,4-triazole (0.34 g, 4 mmol), and 4 ml of glacial acetic acid were mixed and stirred at 120°C for about 7 h. The reaction was monitored by TLC (50% ethyl acetate in petroleum ether). After the reaction was completed, 40 ml of ice water was added to precipitate, which was filtered and recrystallized with 50% ethanol to obtain compound 3a. Compounds 3b–3i were prepared under the same conditions.
2.1.4 Synthesis of 7-Phenylpyrazolo[1,5-a]Pyrimidines (4a-4i)
Considering compound 4a as an example: compound 2a (0.7 g, 4 mmol), 3-aminopyrazole (0.33 g, 4 mmol), and 4 ml of glacial acetic acid were mixed and stirred at 120°C for about 7 h. TLC (50% ethyl acetate in petroleum ether) was used to monitor the reaction process. After the reaction was completed, 40 ml of ice water was added to precipitate, which was filtered and recrystallized with 50% ethanol to obtain compound 4a. Compounds 4b–4i were prepared under the same conditions.
2.1.5 Synthesis of 4-([1,2,4]Triazolo[1,5-a]Pyrimidin-7-yl)Phenol (5)
Compound 3i (1.13 g, 5 mmol), BBr3 (5.00 g, 20 mmol), and 30 ml of dry CH2Cl2 were mixed and stirred in an ice bath for about 6 h. TLC (66% ethyl acetate in petroleum ether) was used to monitor the reaction process. After it was completed, the reaction was quenched by ice water. The organic solvent evaporated under reduced pressure to leave the product dispersed in the water, which was filtered to give compound 5. Mp 230–232°C, yield 89%. 1H-NMR (DMSO-d6, 400 MHz): δ 7.4 (d, 2H, J = 8.6 Hz, Ph-H), 7.61 (d, 1H, J = 4.8 Hz, Py-H), 8.19 (d, 2H, J = 8.6 Hz, Ph-H), 8.80 (s, 1H, Triazole-H), and 8.89 (d, 1H, J = 4.8 Hz, Py-H). 13C NMR (101 MHz, DMSO-d6): δ 161.6, 155.8, 155.5, 155.0, 148.0, 132.1, 120.0, 116.1, and 109.0. ESI-HRMS calculated for C11H9N4O+ ([M + H]+): 213.0771; found: 213.0777.
2.1.6 Synthesis of 7-(4-Alkoxyphenyl)-[1,2,4]Triazolo[1,5-a]Pyrimidines (6a-6e)
Considering compound 6a as an example: compound 5 (0.42 g, 2 mmol), K2CO3 (0.28 g, 2 mmol), bromopropane (0.30 g, 2.4 mmol), and 30 ml of acetonitrile were mixed and stirred at 90°C for about 8 h. TLC (50% ethyl acetate in petroleum ether) was used to monitor the reaction process. After the reaction was completed, the organic solvent was evaporated, and the residue was added to 30 ml of water and extracted by dichloromethane (3 ml × 30 ml). The combined dichloromethane was dried by MgSO4 and evaporated in a vacuum to give a residue, which was purified by column chromatography (silica gel, petroleum ether/EtOAc, 70:30) to provide compound 6a. Compounds 6b –6e were prepared under the same conditions using other haloalkanes.
The physical data of target compounds (3a–3i, 4a–4i, and 6a–6e) are listed as follows:
7-Phenyl-[1,2,4]triazolo[1,5-a]pyrimidine (3a):
Mp 142–143°C, yield 65.1%. 1H-NMR (DMSO-d6, 300 MHz): δ 7.61–8.21 (m, 6H, Ph-H, pyrimidine-H), 8.73 (s, 1H, triazole-H), and 8.96 (d, 1H, J = 4.5 Hz, pyrimidine-H). 13C-NMR (DMSO-d6, 75 MHz): δ 156.23, 156.00, 155.53, 147.78, 132.17, 130.07, 130.01, 129.15, and 110.16. ESI-HRMS calculated for C11H9N4+ ([M + H]+): 197.0822; found: 197.0826.
7-(4-Fluorophenyl)-[1,2,4]triazolo[1,5-a]pyrimidine (3b):
Mp 241–244°C, yield 74.7%. 1H-NMR (CDCl3, 300 MHz): δ 7.24 (d, 1H, J = 4.2 Hz, pyrimidine-H), 7.28–8.20 (m, 4H, Ph-H), 8.57 (s, 1H, triazole-H), and 8.88 (d, 1H, J = 4.2 Hz, pyrimidine-H). 13
C-NMR (CDCl3, 75 MHz): δ 164.78 (d, 1Jc-f = 251.3 Hz), 156.32, 155.89 (d, 4Jc-f = 3.6 Hz), 154.47, 147.28, 131.77 (d, 3Jc-f = 8.7 Hz), 125.73, 116.33 (d, 2Jc-f = 21.8 Hz), and 108.91. ESI-HRMS calculated for C11H8FN4+ ([M + H]+): 215.0728; found: 215.0730.
7-(2-Chlorophenyl)-[1,2,4]triazolo[1,5-a]pyrimidine (3c):
Mp 148–149°C, yield 58.4%. 1H-NMR (CDCl3, 300 MHz): δ 7.21–7.65 (m, 5H, Ph-H, pyrimidine-H), 8.54 (s, 1H, triazole-H), and 8.95 (d, 1H, J = 3.7 Hz, pyrimidine-H). 13C-NMR (CDCl3, 75 MHz): δ 155.89, 155.59, 154.36, 146.28, 133.38, 132.45, 131.01, 130.50, 129.08, 127.25, and 111.62. ESI-HRMS calculated for C11H8ClN4+ ([M + H]+): 231.0432; found: 231.0437.
7-(4-Chlorophenyl)-[1,2,4]triazolo[1,5-a]pyrimidine (3d):
Mp 232–233°C, yield 79.2%. 1H-NMR (CDCl3, 300 MHz): δ 7.26 (d, 1H, J = 3.9 Hz, pyrimidine-H), 7.60 (d, 2H, J = 8.4 Hz, Ph-H), 8.11 (d, 2H, J = 8.4 Hz, Ph-H), 8.57 (s, 1H, triazole-H), and 8.88 (d, 1H, J = 3.9 Hz, pyrimidine-H). 13C-NMR (CDCl3, 75 MHz): δ 156.17, 155.79, 154.52, 147.17, 138.42, 130.69, 129.35, 127.93, and 109.00. ESI-HRMS calculated for C11H8ClN4+ ([M + H]+): 231.0432; found: 231.0433.
7-(2,4-Dichlorophenyl)-[1,2,4]triazolo[1,5-a]pyrimidine (3e):
Mp 168–169°C, yield 47.4%. 1H-NMR (CDCl3, 300 MHz): δ 7.22 (d, 1H, J = 4.1 Hz, pyrimidine-H), 7.50–7.67 (m, 3H, Ph-H), 8.55 (s, 1H, triazole-H), 8.96 (d, 1H, J = 4.1 Hz, pyrimidine-H). 13C-NMR (CDCl3, 75 MHz): δ 155.71, 154.43, 145.23, 145.19, 138.27, 134.34, 131.87, 130.54, 127.75, 127.41, and 111.70. ESI-HRMS calculated for C11H7Cl2N4+ ([M + H]+): 265.0042; found: 265.0047.
7-(4-Bromophenyl)-[1,2,4]triazolo[1,5-a]pyrimidine (3f):
Mp 226–227°C, yield 50.1%. 1H-NMR (CDCl3, 300 MHz): δ 7.26 (d, 1H, J = 4.3 Hz, pyrimidine-H), 7.76 (d, 2H, J = 8.4 Hz, Ph-H), 8.03 (d, 2H, J = 8.4 Hz, Ph-H), 8.56 (s, 1H, triazole-H), and 8.89 (d, 1H, J = 4.3 Hz, pyrimidine-H). 13C-NMR (CDCl3, 75 MHz): δ 156.27, 155.93, 154.45, 147.20, 132.32, 130.80, 128.43, 126.85, and 108.91. ESI-HRMS calculated for C11H8BrN4+ ([M + H]+): 274.9927; found: 274.9930.
7-(4-Nitrophenyl)-[1,2,4]triazolo[1,5-a]pyrimidine (3g):
Mp 248–253°C, yield 65.5%. 1H-NMR (CDCl3, 300 MHz): δ 7.35 (d, 1H, J = 3.9 Hz, pyrimidine-H), 8.36 (d, 2H, J = 6.6 Hz, Ph-H), 8.49 (d, 2H, J = 6.6 Hz, Ph-H), 8.53 (s, 1H, triazole-H), and 8.99 (d, 1H, J = 3.9 Hz, pyrimidine-H). 13C-NMR (CDCl3, 75 MHz): δ 156.13, 156.11, 154.63, 149.57, 145.78, 135.35, 130.58, 124.08, and 109.76. ESI-HRMS calculated for C11H8N5O2+ ([M + H]+): 242.0673; found: 242.0676.
7-(P-tolyl)-[1,2,4]triazolo[1,5-a]pyrimidine (3h):
Mp 180–181°C, yield 47.6%. 1H-NMR (CDCl3, 300 MHz): δ 2.49 (s, 3H, Ph-CH3) 7.24 (d, 1H, J = 4.5 Hz, pyrimidine-H), 7.43 (d, 2H, J = 7.8 Hz, Ph-H), 8.04 (d, 2H, J = 7.8 Hz, Ph-H), 8.57 (s, 1H, triazole-H), and 8.88 (d, 1H, J = 4.5 Hz, Pyrimidine-H). 13C-NMR (CDCl3, 75 MHz): δ 156.13, 155.54, 154.49, 148.54, 142.84, 129.69, 129.30, 126.69, 108.84, and 21.64. ESI-HRMS calculated for C12H11N4+ ([M + H]+): 211.0978; found: 211.0984.
7-(4-Methoxyphenyl)-[1,2,4]triazolo[1,5-a]pyrimidine (3i):
Mp 188–189°C, yield 70.0%. 1H-NMR (CDCl3, 300 MHz): δ 3.94 (s, 3H, OCH3), 7.12 (d, 2H, J = 8.4 Hz, Ph-H), 7.26 (d, 1H, J = 4.4 Hz, pyrimidine-H), 8.18 (d, 2H, J = 8.4 Hz, Ph-H), 8.58 (s, 1H, triazole-H), and 8.85 (d, 1H, J = 4.4 Hz, pyrimidine-H). 13C-NMR (CDCl3, 75 MHz): δ 162.61, 156.21, 155.45, 154.37, 148.14, 131.25, 121.62, 114.43, 108.25, and 55.59. ESI-HRMS calculated for C12H11N4O+ ([M + H]+): 227.0927; found: 227.0935.
7-Phenylpyrazolo[1,5-a]pyrimidine (4a):
Mp 70–71°C, yield 60.0%. 1H-NMR (DMSO-d6, 300 MHz): δ 6.84 (d, 1H, J = 2.1 Hz, pyrazolo-H), 7.24 (d, 1H, J = 4.5 Hz, pyrimidine-H), 7.59–8.15 (m, 5H, Ph-H), 8.27 (d, 1H, J = 2.1 Hz, pyrazolo-H), and 8.62 (d, 1H, J = 4.5 Hz, pyrimidine-H). 13C-NMR (DMSO-d6, 75 MHz): δ 150.03, 149.84, 146.07, 144.94, 131.46, 131.13, 129.85, 128.94, 108.11, and 96.99. ESI-HRMS calculated for C12H10N3+ ([M + H]+): 196.0869; found: 196.0875.
7-(4-Fluorophenyl)pyrazolo[1,5-a]pyrimidine (4b):
Mp 133–134°C, yield 76.4%. 1H-NMR (CDCl3, 300 MHz): δ 6.83 (d, 1H, J = 2.1 Hz, pyrazolo-H), 6.91 (d, 1H, J = 4.3 Hz, pyrimidine-H), 7.22–8.13 (m, 4H, Ph-H), 8.20 (d, 1H, J = 2.1 Hz, pyrazolo-H), and 8.55 (d, 1H, J = 4.3 Hz, pyrimidine-H). 13C-NMR (CDCl3, 75 MHz): δ 164.28 (d, 1Jc-f = 251.1 Hz), 149.55, 148.75, 146.01, 144.87, 131.56 (d, 3Jc-f = 8.6 Hz), 126.99, 115.96 (d, 2Jc-f = 21.8 Hz), 107.02, and 97.16. ESI-HRMS calculated for C12H9FN3+ ([M + H]+): 214.0775; found: 214.0781.
7-(2-Chlorophenyl)pyrazolo[1,5-a]pyrimidine (4c):
Mp 113–114°C, yield 58.7%. 1H-NMR (CDCl3, 300 MHz): δ 6.85 (d, 1H, J = 2.3 Hz, pyrazolo-H), 6.88 (d, 1H, J = 4.1 Hz, pyrimidine-H), 7.47–7.63 (m, 4H, Ph-H), 8.18 (d, 1H, J = 2.3 Hz, pyrazolo-H), and 8.60 (d, 1H, J = 4.1 Hz, pyrimidine-H). 13C-NMR (CDCl3, 75 MHz): δ 148.85, 148.41, 145.11, 145.01, 133.50, 131.78, 130.94, 130.52, 130.29, 127.12, 108.99, and 97.22. ESI-HRMS calculated for C12H9ClN3+ ([M + H]+): 230.0480; found: 230.0483.
7-(4-Chlorophenyl)pyrazolo[1,5-a]pyrimidine (4d):
Mp 146–147°C, yield 69.1%. 1H-NMR (CDCl3, 300 MHz): δ 6.83 (d, 1H, J = 2.3 Hz, pyrazolo-H), 6.91 (d, 1H, J = 4.1 Hz, pyrimidine-H), 7.56 (d, 2H, J = 8.7 Hz, Ph-H), 8.03 (d, 2H, J = 8.7 Hz, Ph-H), 8.18 (d, 1H, J = 2.3 Hz, pyrazolo-H), and 8.55 (d, 1H, J = 4.1 Hz, pyrimidine-H). 13C-NMR (CDCl3, 75 MHz): δ 149.58, 148.77, 145.83, 144.86, 137.36, 130.62, 129.32, 129.05, 107.07, and 97.25. ESI-HRMS calculated for C12H9ClN3+ ([M + H]+): 230.0480; found: 230.0483.
7-(2,4-Dichlorophenyl)pyrazolo[1,5-a]pyrimidine (4e):
Mp 138–139°C, yield 55.5%. 1H-NMR (CDCl3, 300 MHz): δ 6.84 (d, 1H, J = 2.1 Hz, pyrazolo-H), 6.87 (d, 1H, J = 3.3 Hz, pyrimidine-H), 7.28–7.64 (m, 3H, Ph-H), 8.60 (d, 1H, J = 2.1 Hz, pyrazolo-H), and 8.59 (d, 1H, J = 3.3 Hz, pyrimidine-H). 13C-NMR (CDCl3, 75 MHz): δ 149.05, 148.48, 145.07, 143.69, 137.38, 134.46, 131.83, 130.31, 128.96, 127.59, 109.06, and 97.47. ESI-HRMS calculated for C12H8Cl2N3+ ([M + H]+): 264.0090; found: 264.0097.
7-(4-Bromophenyl)pyrazolo[1,5-a]pyrimidine (4f):
Mp 172–174°C, yield 50.3%. 1H-NMR (CDCl3, 300 MHz): δ 6.87 (s, 1H, pyrazolo-H), 6.95 (s, 1H, pyrimidine-H), 7.74 (d, 2H, J = 7.8 Hz, Ph-H), 7.98 (d, 2H, J = 7.8 Hz, Ph-H), 8.21 (s, 1H, pyrazolo-H), and 8.58 (s, 1H, pyrimidine-H). 13C-NMR (CDCl3, 75 MHz): δ 149.37, 148.70, 146.10, 144.96, 132.05, 130.82, 129.73, 125.86, 107.02, and 97.24. ESI-HRMS calculated for C12H9BrN3+ ([M + H]+): 273.9974; found: 273.9979.
7-(4-Nitrophenyl)pyrazolo[1,5-a]pyrimidine (4g):
Mp 252–253°C, yield 51.1%. 1H-NMR (DMSO-d6 + CDCl3, 300 MHz): δ 7.14 (d, 1H, J = 3.8 Hz, pyrimidine-H), 7.67 (s, 1H, pyrazolo-H), 8.22 (s, 1H, pyrazolo-H), 8.37 (d, 2H, J = 8.7 Hz, Ph-H), 8.46 (d, 2H, J = 8.7 Hz, Ph-H), and 8.66 (d, 1H, J = 3.8 Hz, pyrimidine-H). 13C-NMR (DMSO-d6 + CDCl3, 75 MHz): δ 156.93, 153.82, 149.54, 135.36, 130.65, 128.45, 124.37, 118.29, 112.88, and 102.30. ESI-HRMS calculated for C12H9N4O2+ ([M + H]+): 241.0720; found: 241.0726.
7-(P-tolyl)pyrazolo[1,5-a]pyrimidine (4h):
Mp 88–90°C, yield 46.5%. 1H-NMR (CDCl3, 300 MHz): δ 2.47 (s, 3H, Ph-CH3), 6.80 (d, 1H, J = 2.3 Hz, pyrazolo-H), 6.90 (d, 1H, J = 4.3 Hz, pyrimidine-H), 8.39 (d, 2H, J = 7.8 Hz, Ph-H), 7.96 (d, 2H, J = 7.8 Hz, Ph-H), 8.18 (d, 1H, J = 2.3 Hz, pyrazolo-H), and 8.52 (d, 1H, J = 4.3 Hz, pyrimidine-H). 13C-NMR (CDCl3, 75 MHz): δ 148.79, 144.72, 141.65, 129.71, 129.41, 129.18, 128.10, 127.19, 106.88, 96.88, and 21.58. ESI-HRMS calculated for C13H12N3+ ([M + H]+): 210.1026; found: 210.1031.
7-(4-Methoxyphenyl)pyrazolo[1,5-a]pyrimidine (4i):
Mp 119–121°C, yield 46.9%.1H-NMR (CDCl3, 300 MHz): δ 3.92 (s, 3H, Ph-OCH3), 6.80 (d, 1H, J = 2.3 Hz, pyrazolo-H), 6.91 (d, 1H, J = 4.4 Hz, pyrimidine-H), 7.10 (d, 2H, J = 8.7 Hz, Ph-H), 8.10 (d, 2H, J = 8.7 Hz, Ph-H), 8.20 (d, 1H, J = 2.3 Hz, pyrazolo-H), and 8.52 (d, 1H, J = 4.4 Hz, pyrimidine-H). 13C-NMR (CDCl3, 75 MHz): δ 161.95, 149.45, 148.57, 147.04, 144.76, 131.08, 123.04, 114.17, 106.37, 96.74, and 55.52. ESI-HRMS calculated for C13H12N3O+ ([M + H]+): 226.0975; found: 226.0980.
7-(4-Propoxyphenyl)-[1,2,4]triazolo[1,5-a]pyrimidine (6a):
Mp 120–121°C, yield 80%. 1H-NMR (CDCl3, 300 MHz): δ 1.08 (t, 3H, J = 7.4, CH3), 1.84–1.91 (t, 2H, CH2), 4.04 (t, 2H, J = 6.5 Hz, CH2), 7.08–7.11 (dd, 2H, J1 = 6.9 Hz, J2 = 2.1 Hz, Ph-H), 7.21 (d, 1H, J = 4.7 Hz, Ph-H), 8.14–8.17 (dd, 2H, J1 = 6.9 Hz, J2 = 2.1 Hz, Ph-H), 8.55 (s, 1H, triazole-H), and 8.82 (d, 1H, J = 4.7 Hz, N=C-H). 13C NMR (CDCl3, 75 MHz): δ 162.06, 156.30, 155.56, 154.09, 148.00, 131.07, 121.27, 114.73, 107.95, 69.71, 22.31, and 10.37. ESI-HRMS calculated for C14H15N4O+ ([M + H]+): 255.1240; found: 255.1228.
7-(4-Butoxyphenyl)-[1,2,4]triazolo[1,5-a]pyrimidine (6b):
Mp 95–97°C, yield 79%. 1H-NMR (CDCl3, 300 MHz): δ 1.00 (t, 3H, J = 7.3, CH3), 1.49–1.57 (m, 2H, CH2), 1.78–1.85 (m, 2H, CH2), 4.08 (t, 2H, J = 6.5 Hz, CH2), 7.08–7.11 (dd, 2H, J1 = 6.9 Hz, J2 = 2.1 Hz, Ph-H), 7.21 (d, 1H, J = 4.7 Hz, Ph-H), 8.14–8.17 (dd, 2H, J1 = 6.9 Hz, J2 = 2.0 Hz, Ph-H), 8.55 (s, 1H, triazole-H), and 8.82 (d, 1H, J = 4.7 Hz, N=C-H). 13C NMR (CDCl3, 75 MHz): δ 162.06, 156.31, 155.57, 154.08, 147.99, 131.06, 121.26, 114.73, 107.94, 67.93, 30.98, 19.07, and 13.70. ESI-HRMS calculated for C15H17N4O+ ([M + H]+): 269.1397; found: 269.1388.
7-(4-(Pentyloxy)phenyl)-[1,2,4]triazolo[1,5-a]pyrimidine (6c):
Mp 102–103°C, yield 75%. 1H-NMR (CDCl3, 300 MHz): δ 0.96 (t, 3H, J = 7.0, CH3), 1.35–1.53 (m, 2H, CH2CH2), 1.80–1.89 (m, 2H, CH2), 4.07 (t, 2H, J = 6.5 Hz, CH2), 7.08–7.11 (dd, 2H, J1 = 7.0 Hz, J2 = 2.0 Hz, Ph-H), 7.21 (d, 1H, J = 4.7 Hz, Ph-H), 8.14–8.17 (dd, 2H, J1 = 6.9 Hz, J2 = 2.0 Hz, Ph-H), 8.55 (s, 1H, triazole-H), and 8.82 (d, 1H, J = 4.7 Hz, N=C-H). 13C NMR (CDCl3, 75 MHz): δ 162.06, 156.30, 155.56, 154.08, 147.99, 131.06, 121.25, 114.72, 107.94, 68.23, 28.65, 28.00, 22.31, and 13.91. ESI-HRMS calculated for C16H19N4O+ ([M + H]+): 283.1553; found: 283.1549.
7-(4-(Hexyloxy)phenyl)-[1,2,4]triazolo[1,5-a]pyrimidine (6d):
Mp 96–97°C, yield 78%. 1H-NMR (CDCl3, 300 MHz): δ 0.92 (t, 3H, J = 7.0, CH3), 1.33–1.39 (m, 2H, CH2CH2), 1.44–1.54 (m, 2H, CH2), 1.79–1.89 (m, 2H, CH2), 4.07 (t, 2H, J = 6.5 Hz, CH2), 7.08–7.11 (dd, 2H, J1 = 6.9 Hz, J2 = 2.1 Hz, Ph-H), 7.21 (d, 1H, J = 4.7 Hz, Ph-H), 8.13–8.18 (dd, 2H, J1 = 6.9 Hz, J2 = 2.1 Hz, Ph-H), 8.55 (s, 1H, triazole-H), and 8.83 (d, 1H, J = 4.7 Hz, N=C-H). 13C NMR (CDCl3, 75 MHz): δ 162.07, 156.33, 155.58, 154.08, 148.01, 131.07, 121.26, 114.74, 107.94, 68.25, 31.41, 28.92, 25.54, 22.48, and 13.93. ESI-HRMS calculated for C17H21N4O+ ([M + H]+): 297.1710; found: 297.1702.
7-(4-(Benzyloxy)phenyl)-[1,2,4]triazolo[1,5-a]pyrimidine (6e):
Mp 120–122°C, yield 87%. 1H-NMR (CDCl3, 300 MHz): δ 5.19 (s, 2H, CH2), 7.17–7.21 (dd, 2H, J1 = 6.9 Hz, J2 = 2.0 Hz, Ph-H), 7.26 (s, 1H, C=C-H), 7.36–7.41 (m, 5H, Ph-H), 8.15–8.18 (dd, 2H, J1 = 6.9 Hz, J2 = 2.0 Hz, Ph-H), 8.56 (s, 1H, triazole-H), and 8.83 (d, 1H, J = 4.7 Hz, N=C-H). 13C NMR (CDCl3, 75 MHz): δ 161.64, 156.36, 155.60, 154.05, 147.88, 136.05, 131.13, 128.61, 128.17, 127.32, 121.88, 115.19, 107.98, and 70.20. ESI-HRMS calculated for C18H15N4O+ ([M + H]+): 303.1240; found: 303.1227.
2.2 Pharmacology
2.2.1 Animals
Male Kunming mice with weights from 18 to 22 g were used in all the pharmacological experiments. The mice were housed in the standard cages with 10 each, where a controlled temperature (25°C ± 2°C) with a 12 h light/dark cycle was maintained. All animals had free access to food and water. All experiments and procedures involving animals were carried out according to the Guide for the Care and Use of Laboratory Animals (National Academies Press, Washington, D.C., United States). Approval from the local medical ethical committee was obtained before the experiment.
2.2.2 Maximal Electroshock Seizure Test
In the MES test, mice were subjected to ear stimulation using a 0.2 s 60-Hz 50-mA alternating current to elicit seizures. Tonic hind limb extension was considered as the seizure occurring, and the vanishing of tonic hind limb extension indicates the protection against the MES-induced seizures. Mice were injected intraperitoneally with test compounds at doses of 300, 100, and 30 mg/kg to evaluate their general anticonvulsant activity. Half and 4 h intervals were chosen to test the anti-MES activity. Based on the rough effective dose obtained in the aforementioned assay, compounds effective at the dose of 30 mg/kg were subjected to the quantitative assay to determine their median effective dose (ED50). A series of doses of the tested compounds were injected into the mice with 10 in one group. When not less than three doses were obtained at which the mice were protected in 10–90%, the respective ED50, and 95% confidence intervals were calculated using these data by probit analysis.
2.2.3 Subcutaneous Pentylenetetrazole Test
In the PTZ test, mice were subcutaneously administered with PTZ at the dose of 85 mg/kg. At this dose, mice can be elicited a threshold seizure characterized by an episode of clonic spasms and tonic spasms. After being treated with test compounds at doses of 300, 100, and 30 mg/kg, the mice were considered protected if a single episode of clonic spasms of more than 5 s duration was not obtained. The quantitative anticonvulsant activity (ED50) in the PTZ model was determined and calculated with the same method described in the MES test.
2.2.4 Neurotoxicity Screening
The rotarod test was used to evaluate the neurotoxicity of the synthesized compounds. Before the formal experiments, mice were trained to climb on a rotating rod of diameter 3.2 cm with the rotation speed of 10 rpm. Test compounds in the three doses were intraperitoneally injected into the trained mice. After 30 min, they were put on the rotating rod to observe their ability to maintain equilibrium. The rough neurotoxic dose was obtained in which mice cannot maintain equilibrium on the rod for at least 1 min. The quantitative neurotoxic dose (TD50) of the test compounds was measured and calculated with the same method described in the MES test.
2.2.5 Bicuculline Test
In the BIC-induced seizure test, groups of 10 mice were administered with 30 mg/kg of test samples or vehicle. Then, 30 min later, the minimum dose of BIC (5.4 mg/kg) that could completely induce seizures for mice was subcutaneously injected into the test mice. The mice were placed into separate cages each, and the number of clonic seizures, tonic seizures, and mortality was recorded for 30 min.
2.2.6 3-Mercaptopropionic Acid Test
In 3-MP–induced seizure test, groups of 10 mice were administered with 30 mg/kg of test samples or vehicle. After 30 min, the minimum dose of 3-MP (60 mg/kg) that could completely induce seizures for mice was intraperitoneally injected into the test mice. The mice were placed into separate cages each, and the number of clonic seizures, tonic seizures, and mortality was recorded for 30 min.
2.2.7 Docking
Molecular docking was performed by Discovery Studio (release 2019). The three-dimensional (3D) GABAA receptor binding with diazepam was obtained from Richter’s publication (Richter et al., 2012). The hydrogen atoms of the GABAA receptor were added, and water and diazepam were removed. The 3D structures of test molecules were constructed by ChemDraw 16.0 software; then, they were transferred into the Discovery Studio platform to minimize energy. After the aforementioned preparation finished, the molecular docking was performed for the test molecules and GABAA. After the completion of molecular docking, the docking gave a batch of poses, which were ranked and selected by CDOCKER interaction energy. The lowest energy conformation of the ligand–receptor complex was evaluated, and the interaction type between GABAA receptor and test molecules was analyzed by Discovery Studio (release 2019).
2.2.8 Prediction of Drug Likeliness Parameters
Computational calculations were carried out to predict the drug likeliness properties of target compounds. The polar surface area (TPSA), number of rotatable bonds (n-ROTB), miLogP, number of hydrogen donor (HBD) and acceptor (HBA), and violations of Lipinski’s rule of five were calculated by using Molinspiration Property Calculator (Molinspiration Cheminformatics, 2021), which is a free online property calculating toolkit. Absorption (%ABS) was calculated using an equation % ABS = 109—(0.345 * PSA) (Zhao et al., 2002). LogBB, a ratio of the steady-state concentrations of a compound in the brain to that in the blood [i.e., log (Cbrain/Cblood)], was calculated using Clark’s equation as follows: logBB = −0.0148 × PSA + 0.152 × clogP + 0.139 (Clark, 1999).
2.2.9 Radioligand Receptor Binding Assay
The radioligand receptor binding studies were performed according to the procedure described by Ahmadi et al. (2014). Selected compounds were evaluated for their affinity to the GABAA BZD receptor by the radioligand receptor binding assay. Male SD rats with weights ranging from 220 to 260 g were sacrificed after a spinal dislocation. The cerebral tissues except the brainstem was immediately removed and homogenized for 30 s in 10 times the volume of ice-cold Tris–HCl buffer (50 mM, pH 7.4). The homogenates were centrifuged at 1,000 g for 10 min. The resulting supernatant was centrifuged at 30,000 g for 20 min. The precipitate was washed three times with ice-cold buffer by re-suspension and re-centrifugation. All of the centrifugation procedures were performed at 4°C. The obtained membrane preparation was maintained under −4 Fahrenheit until it was used in 20 days. The protein concentration was estimated by the Bradford method. After the membrane preparation, saturation and competition experiments were conducted to obtain the inhibition of radioligand-specific binding (IC50) and affinity (Ki) of the test compounds and diazepam. In the saturation experiment, 200 μg of membrane protein was incubated with nine different concentrations (0.25–25 nM) of [3H]- flumazenil at 30°C for 35 min. The total binding (TB) (receptor + radioligand), non-specific binding (NSB) (receptor + radioligand + excess diazepam), and specific binding (SB) (TB-NSB) were measured at the nine radioligand concentrations. The receptor-binding affinity (Kd) of [3H]-flumazenil and the maximum binding capacity of BZD receptors (Bmax) were calculated from the saturation experiment data. In the competition experiment, 200 μg of membrane protein in Tris–HCl buffer (50 mM, pH 7.4) was incubated with 0.5 nM [3H]-flumazenil and different concentrations (50 pm-5 mM) of test compounds at 30°C for 35 min. After incubation, the assay was terminated by an ice bath. The activity of each tube was measured by a liquid scintillation counter after centrifugation and wash treatment. The competition curve was achieved by plotting the %SB of radioligand vs. the concentrations of test compounds. %SB = 100 (BC-NSB)/(TB-NSB). BC represents the binding in the presence of the test compound. IC50 inhibiting the [3H]-flumazenil-specific binding and affinity for BZD receptors (Ki) of test compounds were calculated according to the Cheng–Prusoff equation. All the experiments were carried out in triplicates.
3 Results
3.1 Chemistry
The synthetical route of compounds 3a–3i was outlined in Scheme 1. The pathway started from the reaction of acetophenones (1a–1i) with N,N-dimethylformamide dimethyl acetal (DMF-DMA) at 100°C without solvent (Ma et al., 2018; Gado et al., 2020), which gave the intermediates (2a–2i). Next, compounds (2a–2i) were reacted with 3-amino-1,2,4-triazole and 3-aminopyrazole in AcOH to afford the target compounds 3a–3i and 4a–4i, respectively (Frizzo et al., 2014). Compounds (6a–6e) were prepared according to Scheme 2. Compound 3i was treated with BBr3 in CH2Cl2 to give the demethylated derivative 5, which was alkylated by haloalkanes to obtain the target compounds 6a–6e. 1H-NMR, 13C NMR, and mass spectrometry were used to characterize the chemical structures of targets. The physical/analysis data in detail and the yield of the targets are provided in Section 2.
3.2 Evaluation of the Anticonvulsant Activity and Neurotoxicity
The anticonvulsant activities of targets were evaluated using seizure models induced by MES and PTZ. The neurotoxicity was evaluated via the rotarod test. Structurally, target molecules could be categorized into three groups: 3a–3i (containing the triazole moiety), 4a–4i (containing the pyrazole moiety), and 6a–6e (containing the triazole moiety and higher hydrophobicity than the series of 3a–3i). In each series, substituents on the benzene ring at position 7 were altered to optimize the anticonvulsant activity.
Initially, all the target compounds were screened at three dosages (30, 100, and 300 mg/kg). As exhibited in Table 1, compounds 3a, 3b, 3c, 3e, 3h, and 3i from the first series of compounds displayed medium-to-weak anticonvulsant activity at 100 or 300 mg/kg at 0.5 h in the MES test. None in the second series of compounds (4a–4i) displayed anticonvulsant activity even at the highest dose of 300 mg/kg in the MES test. On the contrary, all compounds in the series of 6a–6e showed anticonvulsant activity in three dosages. Compounds 6c, 6d, and 6e displayed the best protective effect at the dosage of 30 mg/kg and remained active at 100 or 300 mg/kg after 4 h.
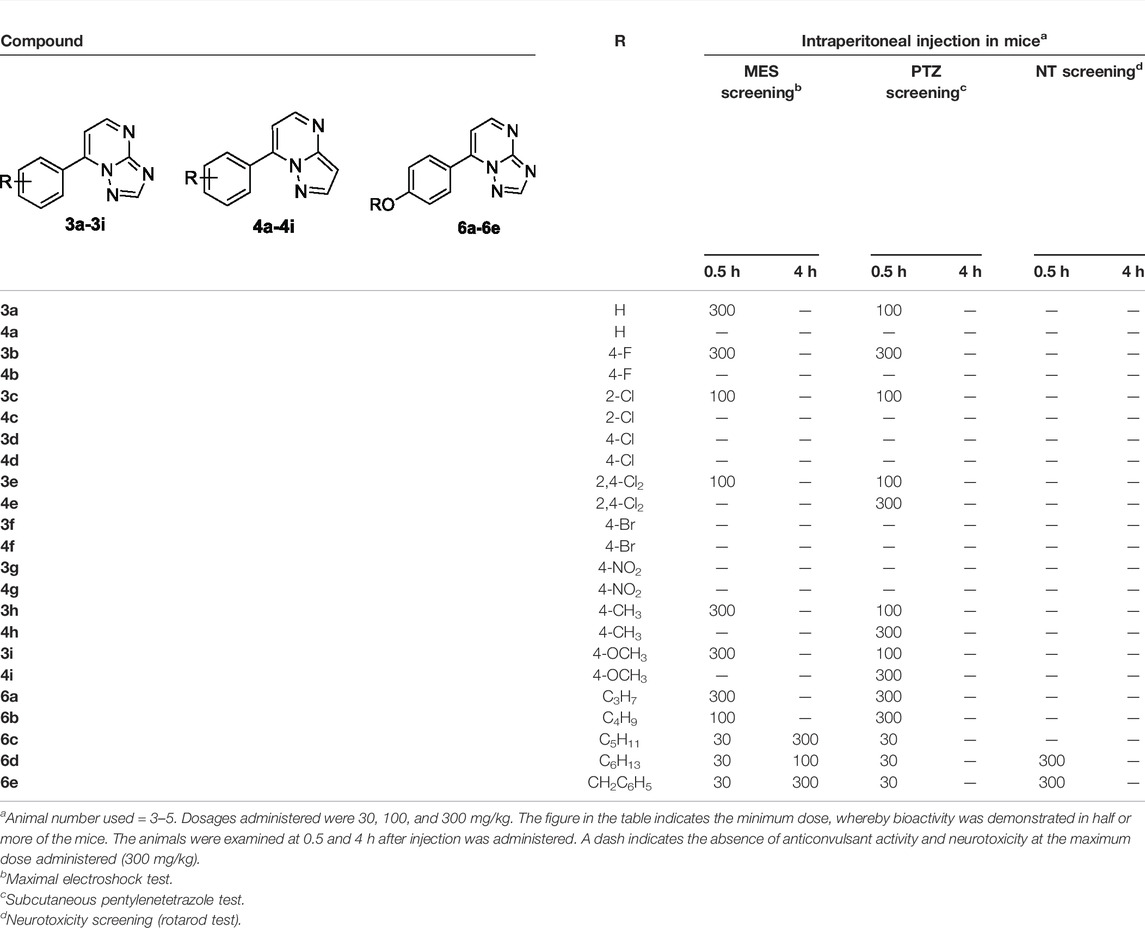
TABLE 1. Anticonvulsant activity and neurotoxicity of compounds 3a–3i, 4a–4i, and 6a–6e administered intraperitoneally in mice.
The performance of designed compounds in the PTZ model was generally similar to that in the MES test. Compounds 3a, 3b, 3c, 3e, 3h, and 3i from the first series of compounds displayed protection at the dosage of 100 or 300 mg/kg in the PTZ model at 0.5 h. Compounds 4e, 4h, and 4i in the second series exhibited anticonvulsant activity at the highest dose of 300 mg/kg at 0.5 h intervals. Compounds 6a and 6b protected the mice against PTZ-induced seizure at 300 mg/kg at 0.5 h interval. Compounds 6c, 6d, and 6e showed protection for mice at the dose of 30 mg/kg at 0.5 h interval.
None of the target molecules except 6d and 6e exhibited neurotoxicity at the maximum dosage of 300 mg/kg in the rotarod test. Some mice injected with 6d or 6e in 300 mg/kg could not maintain balance on a rotating rod. In addition, no compounds were observed to have side effects in common with antiepileptics, such as sedation, hypnosis, and anxiety (with features of bradykinesia or running) at the dosage of 100 mg/kg.
According to the visible antiepileptic activity of compounds 6c, 6d, and 6e indicated in the initial trials, the quantitative evaluation trials were conducted to obtain the ED50 and TD50 values. The quantitative data of compounds 6c, 6d, and 6e and those of the positive drugs valproate, carbamazepine, and diazepam, are listed in Table 2. Compound 6d with an ED50 value of 15.8 mg/kg was found to have the strongest effect against MES-induced seizures in mice. Meanwhile, compounds 6c and 6e displayed ED50 values of 25.3 and 38.0 mg/kg, respectively. All compounds tested were more potent than valproate but less active than that of carbamazepine and diazepam in the MES-induced seizure model. In the PTZ model, compounds 6c, 6d, and 6e gave ED50 values of 23.7, 14.1, and 28.4 mg/kg, respectively. They were more active than valproate and carbamazepine but less active than that of diazepam in the PTZ-induced seizure model.
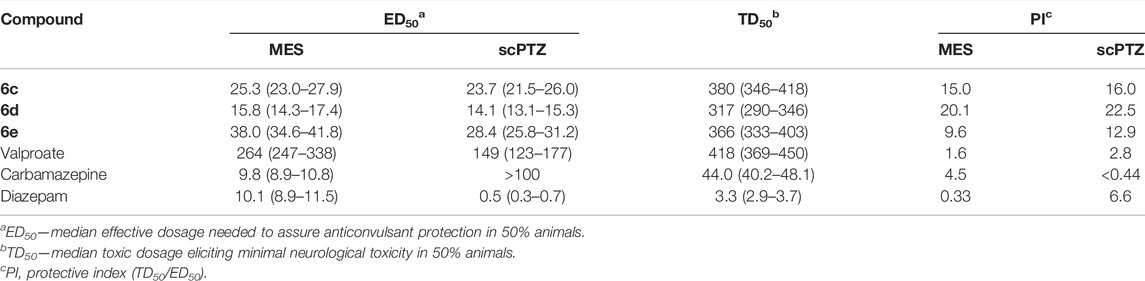
TABLE 2. Quantitative anticonvulsant date of 6c, 6d, and 6e (anti-MES and anti-PTZ) in mice administered intraperitoneally.
As for the neurotoxicity, all synthesized compounds, including the standard drug valproate, showed neurotoxicity at very high dosages (TD50 ≥ 317 mg/kg). However, the TD50 values for the positive controls carbamazepine and diazepam were 44.0 and 3.3 mg/kg, respectively, being remarkably less than those of the synthesized compounds. Based on the obtained results from MES, PTZ, and rotarod tests, the protective index (PI = TD50/ED50) was calculated and is presented in Table 2. The results revealed that the designed compounds were able to display good anticonvulsant activity with a high safety profile. Although the anti-MES and anti-PTZ activities of the compounds 6c–6e were lower than those of diazepam, their PI values were significantly higher than those of the latter.
3.3 Antiepileptic Activity Evaluation of Compound 6d in Other Seizure Models
To further confirm the antiepileptic activity, two seizure models induced by 3-MP and BIC were used. 3-MP, a competitive inhibitor of glutamate decarboxylase (GAD), can decrease the level of GABA in the brain by inhibiting its synthesis (Crick et al., 2014; Enrique et al., 2017). As shown in Table 3, 3-MP induced convulsions in 100% of the mice at 60 mg/kg. 3-MP and 6d combination therapy showed complete inhibition on mice’s clonic seizures, tonic seizures, and death in comparison with the results following treatment of 3-MP alone. BIC could induce seizures in mice as a competitive GABAA receptor antagonist (Velisek, 2006). BIC caused seizures in 100% of the mice at 5.4 mg/kg. As indicated in Table 4, carbamazepine significantly inhibited tonic seizure and death. Similarly, compound 6d inhibited tonic seizure, death (both from 100% to 0%, p < 0.001 for seizures and death), and clonic seizure (from 100% to 10%, p < 0.05) significantly. The superiority of compound 6d in inhibiting clonic seizures, tonic seizures, and death in seizure models caused by 3-MP and BIC, compared to carbamazepine, further identified the antiepileptic effect of 6d.
3.4 Study on the Possible Mechanism of Action
As is known, diazepam belongs to BZDs. The anticonvulsant activity of diazepam can be reversed by the BZD-receptor antagonist flumazenil (Kim et al., 2020). To understand the possible mechanism of the anticonvulsant activity of the most potent compound 6d, we checked if the protection activity of the compound 6d in the MES and PTZ tests could be antagonized by flumazenil. As shown in Table 5, administration of compound 6d at 30 mg/kg alone gave full protection to mice against the MES and PTZ-induced seizures, while pretreatment of flumazenil completely reversed the anti-MES and anti-PTZ activities of compound 6d. These results suggested that the anticonvulsant action of compound 6d might be related to BZD receptors.
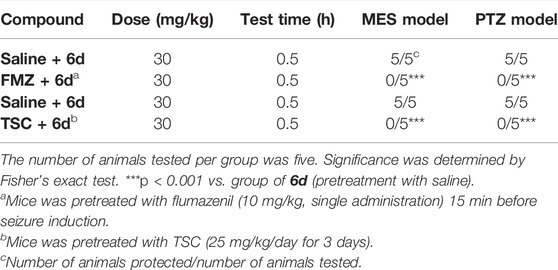
TABLE 5. Effects of flumazenil (FMZ) and thiosemicarbazide (TSC) on the anticonvulsive action of 6d against MES and PTZ-induced seizures in mice.
Thiosemicarbazide (TSC), a competitive inhibitor of the GABA synthesis enzyme, can decrease the GABA level in the brain by inhibiting the synthesis of GABA (Collins, 1973). The influence of TSC on the antiseizure activity of 6d was investigated to further confirm that the GABAergic system was involved in the antiseizure mechanism of 6d. As displayed in Table 5, the normal mice were completely protected in the MES model at 30 mg/kg, while the effects vanished in the treated mice. The anticonvulsant activity of 6d was completely reversed after the pretreatment with TSC at the dosage of 25 mg/kg for 3 days in the MES and PTZ models. Based on this finding, it could be concluded that compound 6d acts its antiepileptic activity by regulating the GABA function in the brain.
The radioligand receptor–binding study is a very powerful tool in the study of receptors and their ligands. To verify the hypothesis that these compounds are binding and affecting the GABAA receptor chloride ionophore complex via the BZP receptor, the affinity of compound 6d to the BZD receptor was evaluated using [3H] flumazenil as the specific ligand. The results showed that compound 6d and diazepam have an affinity to the BZD receptor in nanomolar concentrations (IC50 = 8.4 and 1.3 nM and Ki values = 3.9 and 0.61 nM, respectively). This result further confirmed that the anticonvulsant activity of 6d was mediated by BZD receptors (Table 6).

TABLE 6. Binding affinity of compound 6d and diazepam competing on 3H-flumazenil to the benzodiazepine receptor.
3.5 Molecular Docking Study
To explore the interaction pattern of the synthesized compounds to the BZD-binding site of the GABAA receptor, the docking of active compounds 3e and 6d with the BZD-binding site of the GABAA receptor (α1β2γ2) was conducted by Discovery Studio 4.0 Client. Interactions of the diazepam and compounds 3e and 6d with amino acids of BZD-binding pocket of the GABAA receptor are illustrated and shown in Figure 3. As shown in Figure 3, the most important residues in the binding mode of diazepam are Ser204, Thr206, Tyr209, His101, and Tyr159. These results are consistent with those reported by Ernst and Foroumadi (Richter et al., 2012; Mohammadi-Khanaposhtani et al., 2016). Interactions of compound 3e and the GABAA receptor were identical with those of diazepam. Critical amino acid residues SER204, Thr206, Tyr209, His101, and Tyr159 were all involved, but the amino acid residue Thr206 formed a hydrogen bonding with the N atom in position 3. The benzene ring in compound 3e was responsible for π–π interaction with His101 and Tyr159. The binding model of compound 6d was different from diazepam and compound 3e. Amino acid residues Phe77, His101, Arg132, and Tyr 209 were involved in the interactions between compound 6d and the GABAA receptor. It is worth mentioning that the N atom in position 3 was also a critical one that was involved in hydrogen-bonding interaction with His 101.
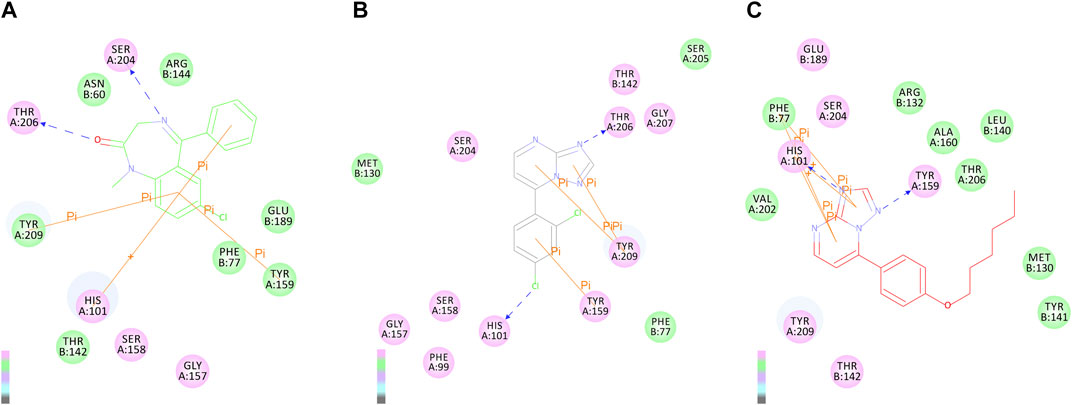
FIGURE 3. 2D binding modes of diazepam (A), compound 4e (B), and 6d (C) in the BZD-binding pocket of the GABAA receptor (α1β2γ2).
3.6 Prediction of Drug-Like Parameters
Drug-like property is an important basis for evaluating the potential of drug development. The drug-like properties of targeted compounds, such as molecular weight (MW), octanol/water partition coefficients (ClogP), the number of H-bond donors (HBD), the number of H-bond acceptors including (HBA), the number of rotatable bonds (ROTB), and the polar surface area (tPSA), were predicted by the computational study. The PSA of a molecule effectively represents the portion of its surface belonging to polar atoms, such as oxygen, nitrogen, and attached hydrogen, and is a descriptor related to the passive molecular transport of a molecule through membranes. From the PSA, intestinal absorption (%ABS) was calculated according to the algorithm described by Zhao et al. (2002). It has been proven by Palm et al. (1996) that drugs with a PSA value below 60 were completely absorbed in the intestine based on Caco-2 cell studies. The PSA values of most target compounds were all below 60 except for compounds 3g and 4g, and the level of intestinal absorption (%ABS) was bigger than 90% except compounds 3g and 4g. The following conclusions can be drawn that these compounds might display good transport properties in the intestines.
The “rule of five” is a set of simple molecular descriptors, which was established by Lipinski, which states that most “drug-like” molecules have common parameters, including LogP ≤ 5, Mw ≤ 500, HAB ≤ 10, HBD ≤ 5, and ROTB ≤ 10 (Lipinski et al., 2001). Molecules that violate one of the aforementioned rules may have bioavailability problems. It can be seen from Table 7 that all synthetic compounds meet all parameters of Lipinski and have good drug-likeliness.
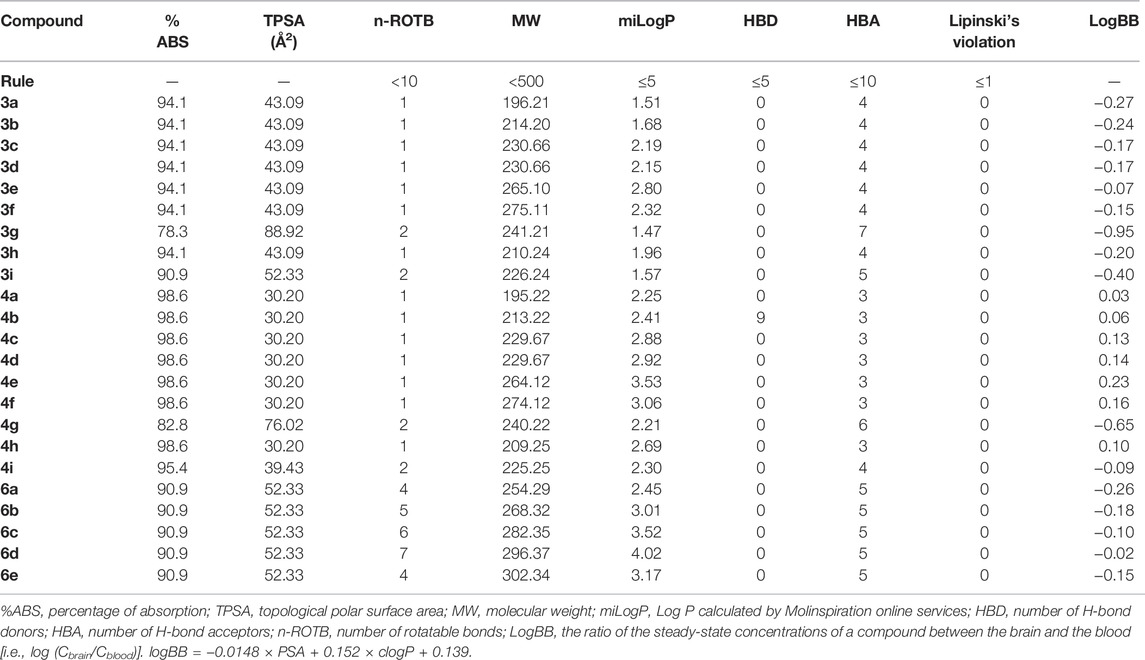
TABLE 7. Pharmacokinetic parameters important for good oral bioavailability and drug likeness of targets compounds 3a–3i, 4a–4i, and 6a–6e.
Good blood–brain barrier (BBB) permeability is necessary for CNS drugs (Fu, 2018) To predict the BBB penetration, logBB of the compounds was calculated according to the algorithm described by Clark (1999), which refers to the ratio of the steady-state concentrations of compounds in the brain and blood [i.e., log (Cbrain/Cblood)]. As reported, compounds with logBB < −1.0 are poorly distributed in the brain (Abraham et al., 1997). The logBB values of synthesized compounds are calculated and presented in Table 6. The measured logBB values for compounds 3a–3i, 4a–4i, and 6a–6e were ranging from −0.95 to 0.23. The most active compound 6d had logBB of −0.05, being the acceptable value for a CNS-active compound.
4 Discussion
Diazepam, a classic benzodiazepine drug, is widely used to treat epilepsy, especially the epileptic status (Calcaterra and Barrow, 2014). However, the side effects of diazepam including drowsiness, motor coordination disorders, and drug dependence limit its application in the clinics (Wu et al., 2016). To obtain new anticonvulsants, which are highly safe, triazole derivatives 3a–3i were designed and synthesized based on the pharmacophoric features of benzodiazepine agonists. The MES and PTZ models, as the most popular and widely used animal seizure models, were used in our anticonvulsant activity screen. As reported, the MES test is considered to be a predictor of the possible therapeutic effect on generalized tonic–clonic seizures, while the PTZ test represents an effective model for selecting candidates for human generalized myoclonic seizures (Loscher, 2011).
The preliminary study showed that some triazole derivatives synthesized displayed anticonvulsant activity in both seizure models. To verify whether the triazole moiety is necessary for the anticonvulsant activity, a series of pyrazole derivatives (4a–4i), as their bioisosterics, were prepared, and their anticonvulsant activity was screened. It was disappointing, but as expected, pyrazole derivatives (4a–4i) did not display any anticonvulsant activity in the MES test even at the highest dose. This suggested that triazole plays a critical role in anticonvulsant activity.
Considering that the BBB permeability is necessary for the compounds to work in vivo, we prepared additional compounds (6a–6e) with higher hydrophobicity. It is gratifying that these compounds (6a–6e) with higher hydrophobicity showed better performance in the seizure models. Compound 6d, whose ED50 values are 15.8 and 14.1 mg/kg against MES- and PTZ-induced seizures, respectively, in mice, was the most promising one in this study. Hydrophobicity is an important parameter for the anticonvulsant activity of triazole derivatives (Song and Deng, 2018; Aggarwal and Sumran, 2020). Many triazole derivatives holding a suitable aryl or alkyl group gave a better anticonvulsant activity in their analogs (Deng et al., 2014a; Deng et al., 2014b). From Table 1, it was noticeable that the hydrophobicity of compounds 6a–6e affects their anticonvulsant activity. For compounds 6a–6d, with the extension of the alkyl chain, the hydrophobicity of the compounds increased gradually, and the activity increased accordingly. The ClogP values of 6a, 6b, 6c, 6d, and 6e were calculated by ChemDraw 16.0 software as 1.56, 2.09, 2.62, 3.15, and 2.27, respectively. The bigger hydrophobicity of 6d gives it higher BBB permeability and higher in vivo anticonvulsant activity.
The comparison of the PTZ and MES test results obtained showed that all active molecules possessed better performance in the PTZ model than in the MES model. Some studies indicated that PTZ diminishes the GABAergic function (Macdonald and Barker, 1977), through competitive antagonism to the BZD receptor (Rehavi et al., 1982). Correspondingly, drugs that enhance GABAA receptor neurotransmission, such as BZDs (Shafie et al., 2020; Navidpour et al., 2021), can inhibit seizures induced by PTZ. PTZ, 3-MP, and BIC also induced seizures through GABAA receptor neurotransmission. The ability of compound 6d to block PTZ, 3-MP, and BIC-induced seizures may be attributed to its modulatory effect on GABAA receptor neurotransmission.
The anticonvulsant effect of compound 6d against MES- and PTZ-induced seizures was completely reversed by the BZD-receptor antagonist flumazenil, which suggested that BZD receptors might be involved in the anticonvulsant effect of compound 6d. The radioligand-binding assay further confirmed that the anticonvulsant activity of compound 6d can be attributed to the direct modulation of the BZD receptor. This result is consistent with our initial design and also supported by the docking study. Interactions of compound 3e and GABAA receptor were identical with those of diazepam. The amino acid residue Thr206 formed a hydrogen bond with the N atom in position 3, which was missed in the inactive derivatives 4a–4i. The binding model of compound 6d was different from the diazepam and compound 3e. But the N atom in position 3 was also a critical atom, which was involved in a hydrogen bonding interaction with His101 in the interactions of compound 6e and the GABAA receptor.
BZDs represent one of the important classes of anticonvulsant agents with excellent activity against generalized tonic–clonic and partial seizures. It has been clarified that it works by binding to the GABAA receptors. This binding increases the frequency of chloride ion channel opening, which facilitates the inhibitory effects of GABA. However, certain side effects are associated with the short- and long-term use of benzodiazepines, which includes sedation, confusion, drowsiness, ataxia, and memory impairment (Schmitz, 2016). Since chlordiazepoxide was found in 1960, a large number of BZD drugs have been found and approved, among which clobazam, clonazepam, nordazepam, nimetazepam, lorazepam, and diazepam are used in the treatment of epilepsy. All of them shared the common skeleton, that is, the benzodiazepine moiety, and gave the common SARs (Riss et al., 2008). In the last decade, new BZDs were designed and reported to find novel anticonvulsant agents with less adverse effects (Arora et al., 2020; Maramai et al., 2020). In 2017, Shao et al. (2018)reported a series of 7-(benzylamino)-1H-benzo[b] [1,4]diazepine-2,4(3H,5H)-dione derivatives. It was found that the most active compound was substituted by 4-fluoro, which holds an ED50 value of 36.5 mg/kg in the MES test and 68.2 mg/kg in the scPTZ test. Compared to that, compound 6d reported here exhibited better anti-MES and anti-PTZ activities. More important is that the structural skeleton jumped out of the bondage of benzodiazepine, which provides a new skeleton for the research of antiepileptic drugs based on the BZD-binding site of the GABA receptor.
5 Conclusion
In conclusion, the design, synthesis, and in vivo anticonvulsant activity of a series of triazole derivatives (3a–3i), pyrazole-contained bioisosterics (4a–4i), and analogs with higher hydrophobicity (6a–6e) were reported. More than half in the series of 3a–3i and all in the series of 6a–6e showed anticonvulsant activity in both MES- and PTZ-induced seizure models. On the contrary, pyrazole-containing bioisosterics (4a–4i) showed weak or no protective effects in both models. The triazole moiety played an important role in their anticonvulsant activity. This supposition was supported by the docking study. Among the synthesized compounds, the triazole derivative 6d was the most potent compound in the MES and PTZ tests. Although the anti-MES and anti-PTZ activities of the compound 6d were lower than those of diazepam, their PI values were significantly higher than those of the latter. Moreover, the experimental investigation of the compound 6d’s mechanism of action suggested that compound 6d works as an anticonvulsant agent via regulating the GABA function such as the activation of the BZD receptor. Prominent anticonvulsive performance and low neurotoxicity, combined with excellent drug-like properties of the triazolopyrimidines reported in this work, indicate their potential in the development of new anticonvulsant agents.
Data Availability Statement
The original contributions presented in the study are included in the article/Supplementary Material; further inquiries can be directed to the corresponding authors.
Ethics Statement
The animal study was reviewed and approved by the Jinggangshan University medical ethical committee.
Author Contributions
All authors listed have made a substantial, direct, and intellectual contribution to the work and approved it for publication.
Funding
This research was funded by the Jiangxi Provincial Natural Science Foundation (No. 20202BABL206154) and the National Natural Science Foundation of China (22167017 and 21602090).
Conflict of Interest
The authors declare that the research was conducted in the absence of any commercial or financial relationships that could be construed as a potential conflict of interest.
Publisher’s Note
All claims expressed in this article are solely those of the authors and do not necessarily represent those of their affiliated organizations, or those of the publisher, the editors, and the reviewers. Any product that may be evaluated in this article, or claim that may be made by its manufacturer, is not guaranteed or endorsed by the publisher.
Supplementary Material
The Supplementary Material for this article can be found online at: https://www.frontiersin.org/articles/10.3389/fchem.2022.925281/full#supplementary-material
References
Abraham, M. H., Takács-Novák, K., and Mitchell, R. C. (1997). On the Partition of Ampholytes: Application to Blood-Brain Distribution. J. Pharm. Sci. 86 (3), 310–315. doi:10.1021/js960328j
Aggarwal, R., and Sumran, G. (2020). An Insight on Medicinal Attributes of 1,2,4-triazoles. Eur. J. Med. Chem. 205, 112652. doi:10.1016/j.ejmech.2020.112652
Ahmadi, F., Dabirian, S., Faizi, M., Tabatabai, S. A., Beiki, D., and Shahhosseini, S. (2014). Optimum Conditions of Radioligand Receptor Binding Assay of Ligands of Benzodiazepine Receptors. Iran. J. Pharm. Res. 13 (Suppl. l), 79–86.
Arora, N., Dhiman, P., Kumar, S., Singh, G., and Monga, V. (2020). Recent Advances in Synthesis and Medicinal Chemistry of Benzodiazepines. Bioorg. Chem. 97, 103668. doi:10.1016/j.bioorg.2020.103668
Beghi, E., and Hesdorffer, D. (2014). Prevalence of Epilepsy-An Unknown Quantity. Epilepsia 55, 963–967. doi:10.1111/epi.12579
Calcaterra, N. E., and Barrow, J. C. (2014). Classics in Chemical Neuroscience: Diazepam (Valium). ACS Chem. Neurosci. 5 (4), 253–260. doi:10.1021/cn5000056
Clark, D. E. (1999). Rapid Calculation of Polar Molecular Surface Area and its Application to the Prediction of Transport Phenomena. 2. Prediction of Blood-Brain Barrier Penetration. J. Pharm. Sci. 88 (8), 815–821. doi:10.1021/js980402t
Collins, g. G. S. (1973). Effect of Aminooxyacetic Acid, Thiosemicarbazide and Haloperidol on the Metabolism and Half-Lives of Glutamate and GABA in Rat Brain. Biochem. Pharmacol. 22, 101–111. doi:10.1016/0006-2952(73)90259-1
Crick, E. W., Osorio, I., Frei, M., Mayer, A. P., and Lunte, C. E. (2014). Correlation of 3-mercaptopropionic Acid Induced Seizures and Changes in Striatal Neurotransmitters Monitored by Microdialysis. Eur. J. Pharm. Sci. 57, 25–33. doi:10.1016/j.ejps.2013.11.019
Deng, X.-Q., Song, M.-X., Wang, S.-B., and Quan, Z.-S. (2014a). Synthesis and Evaluation of the Anticonvulsant Activity of 8-Alkoxy-4,5-Dihydrobenzo[b][1,2,4]triazolo[4,3-D][1,4]thiazepine Derivatives. J. Enzyme Inhibition Med. Chem. 29 (2), 272–280. doi:10.3109/14756366.2013.776555
Deng, X.-Q., Song, M.-X., Zheng, Y., and Quan, Z.-S. (2014b). Design, Synthesis and Evaluation of the Antidepressant and Anticonvulsant Activities of Triazole-Containing Quinolinones. Eur. J. Med. Chem. 73, 217–224. doi:10.1016/j.ejmech.2013.12.014
Enrique, A., Goicoechea, S., Castaño, R., Taborda, F., Rocha, L., Orozco, S., et al. (2017). New Model of Pharmacoresistant Seizures Induced by 3-mercaptopropionic Acid in Mice. Epilepsy Res. 129, 8–16. doi:10.1016/j.eplepsyres.2016.10.012
Frizzo, C. P., Scapin, E., Marzari, M. R. B., München, T. S., Zanatta, N., Bonacorso, H. G., et al. (2014). Ultrasound Irradiation Promotes the Synthesis of New 1,2,4-Triazolo[1,5-A]pyrimidine. Ultrason. Sonochemistry 21 (3), 958–962. doi:10.1016/j.ultsonch.2013.12.007
Fu, B. M. (2018). Transport across the Blood-Brain Barrier. Adv. Exp. Med. Biol. 1097, 235–259. doi:10.1007/978-3-319-96445-4_13
Gado, F., Arena, C., Fauci, C. L., Reynoso-Moreno, I., Bertini, S., Digiacomo, M., et al. (2020). Modification on the 1,2-Dihydro-2-Oxo-Pyridine-3-Carboxamide Core to Obtain Multi-Target Modulators of Endocannabinoid System. Bioorg. Chem. 94, 103353. doi:10.1016/j.bioorg.2019.103353
Gao, F., Wang, T., Xiao, J., and Huang, G. (2019). Antibacterial Activity Study of 1,2,4-triazole Derivatives. Eur. J. Med. Chem. 173, 274–281. doi:10.1016/j.ejmech.2019.04.043
GBD 2019 Diseases and Injuries Collaborators (2020). Global Burden of 369 Diseases and Injuries in 204 Countries and Territories, 1990-2019: a Systematic Analysis for the Global Burden of Disease Study 2019. Lancet 396 (10258), 1204–1222. doi:10.1016/S0140-6736(20)30925-9
Kim, J. J., Gharpure, A., Teng, J., Zhuang, Y., Howard, R. J., Zhu, S., et al. (2020). Shared Structural Mechanisms of General Anaesthetics and Benzodiazepines. Nature 585 (7824), 303–308. doi:10.1038/s41586-020-2654-5
Lipinski, C. A., Lombardo, F., Dominy, B. W., and Feeney, P. J. (2001). Experimental and Computational Approaches to Estimate Solubility and Permeability in Drug Discovery and Development Settings. Adv. Drug Deliv. Rev. 46 (1-3), 3–26. doi:10.1016/s0169-409x(00)00129-0
Loiseau, P. (1983). Benzodiazepines in the Treatment of Epilepsy. Encephale 9 (4 Suppl. 2), 287B–292B.
Löscher, W. (2011). Critical Review of Current Animal Models of Seizures and Epilepsy Used in the Discovery and Development of New Antiepileptic Drugs. Seizure 20 (5), 359–368. doi:10.1016/j.seizure.2011.01.003
Ma, F., Liu, J., Zhou, T., Lei, M., Chen, J., Wang, X., et al. (2018). Discovery and Structure-Activity Relationships Study of Thieno[2,3-B]pyridine Analogues as Hepatic Gluconeogenesis Inhibitors. Eur. J. Med. Chem. 152, 307–317. doi:10.1016/j.ejmech.2018.04.028
Macdonald, R. L., and Barker, J. L. (1977). Pentylenetetrazol and Penicillin Are Selective Antagonists of GABA-Mediated Post-synaptic Inhibition in Cultured Mammalian Neurones. Nature 267 (56113), 720–721. doi:10.1038/267720a0
Maramai, S., Benchekroun, M., Ward, S. E., and Atack, J. R. (2020). Subtype Selective γ-Aminobutyric Acid Type A Receptor (GABAAR) Modulators Acting at the Benzodiazepine Binding Site: An Update. J. Med. Chem. 63 (7), 3425–3446. doi:10.1021/acs.jmedchem.9b01312
Mohammadi-Khanaposhtani, M., Shabani, M., Faizi, M., Aghaei, I., Jahani, R., Sharafi, Z., et al. (2016). Design, Synthesis, Pharmacological Evaluation, and Docking Study of New Acridone-Based 1,2,4-oxadiazoles as Potential Anticonvulsant Agents. Eur. J. Med. Chem. 112, 91–98. doi:10.1016/j.ejmech.2016.01.054
Molinspiration Cheminformatics (2021). Molinspiration. Available at: http://www.molinspiration.com/(Accessed on Dec 12, 2021).
Navidpour, L., Shabani, S., Heidari, A., Bashiri, M., Ebrahim-Habibi, A., Shahhosseini, S., et al. (2021). 5-[Aryloxypyridyl (Or Nitrophenyl)]-4h-1,2,4-Triazoles as Novel Flexible Benzodiazepine Analogues: Synthesis, Receptor Binding Affinity and Lipophilicity-dependent Anti-seizure Onset of Action. Bioorg. Chem. 106, 104504. doi:10.1016/j.bioorg.2020.104504
Palm, K., Luthman, K., Unge, A.-L., Strandlund, G., and Artursson, P. (1996). Correlation of Drug Absorption with Molecular Surface Properties. J. Pharm. Sci. 85 (1), 32–39. doi:10.1021/js950285r
Perucca, P., Scheffer, I. E., and Kiley, M. (2018). The Management of Epilepsy in Children and Adults. Med. J. Aust. 208 (5), 226–233. doi:10.5694/mja17.00951
Peyton, L. R., Gallagher, S., and Hashemzadeh, M. (2015). Triazole Antifungals: a Review. Drugs Today (Barc) 51 (12), 705–718. doi:10.1358/dot.2015.51.12.2421058
Rehavi, M., Skolnick, P., and Paul, S. M. (1982). Effects of Tetrazole Derivatives on [3H]diazepam Binding In Vitro: Correlation with Convulsant Potency. Eur. J. Pharmacol. 78 (3), 353–356. doi:10.1016/0014-2999(82)90037-1
Richter, L., de Graaf, C., Sieghart, W., Varagic, Z., Mörzinger, M., de Esch, I. J. P., et al. (2012). Diazepam-bound GABAA Receptor Models Identify New Benzodiazepine Binding-Site Ligands. Nat. Chem. Biol. 8 (5), 455–464. doi:10.1038/nchembio.917
Riss, J., Cloyd, J., Gates, J., and Collins, S. (2008). Benzodiazepines in Epilepsy: Pharmacology and Pharmacokinetics. Acta Neurol. Scand. 118 (2), 69–86. doi:10.1111/j.1600-0404.2008.01004.x
Schmitz, A. (2016). Benzodiazepine Use, Misuse, and Abuse: A Review. Ment. Health Clin. 6 (3), 120–126. doi:10.9740/mhc.2016.05.120
Shafie, A., Mohammadi-Khanaposhtani, M., Asadi, M., Rahimi, N., Ranjbar, P. R., Ghasemi, J. B., et al. (2020). Novel Fused 1,2,3-Triazolo-Benzodiazepine Derivatives as Potent Anticonvulsant Agents: Design, Synthesis, In Vivo, and In Silico Evaluations. Mol. Divers 24 (1), 179–189. doi:10.1007/s11030-019-09940-9
Shao, Y.-P., HanWu, R.-B. H. F., Wu, H.-F., and Piao, F.-Y. (2018). Synthesis and Anticonvulsant Activity of Some Novel 7-(benzylamino) -1h-Benzo[b][1,4]diazepine-2,4(3h, 5H)-Dione Derivatives. Med. Chem. Res. 27, 642–652. doi:10.1007/s00044-017-2089-1
Song, M.-X., and Deng, X.-Q. (2018). Recent Developments on Triazole Nucleus in Anticonvulsant Compounds: a Review. J. Enzyme Inhibition Med. Chem. 33 (1), 453–478. doi:10.1080/14756366.2017.1423068
Velisek, L. (2006). “Models of Seizures and Epilepsy,” in CHAPTER 11- Models of Chemically-Induced Acute Seizures (Academic Press), 127–152. 9780120885541.
Wen, X., Zhou, Y., Zeng, J., and Liu, X. (2020). Recent Development of 1,2,4-Triazole-Containing Compounds as Anticancer Agents. Ctmc 20 (16), 1441–1460. doi:10.2174/1568026620666200128143230
Wu, W., Zhang, L., and Xue, R. (2016). Lorazepam or Diazepam for Convulsive Status Epilepticus: A Meta-Analysis. J. Clin. Neurosci. 29, 133–138. doi:10.1016/j.jocn.2015.10.038
Xu, M., Peng, Y., Zhu, L., Wang, S., Ji, J., and Rakesh, K. P. (2019). Triazole Derivatives as Inhibitors of Alzheimer's Disease: Current Developments and Structure-Activity Relationships. Eur. J. Med. Chem. 180, 656–672. doi:10.1016/j.ejmech.2019.07.059
Zhou -H., C., and Wang, Y. (2012). Recent Researches in Triazole Compounds as Medicinal Drugs. Cmc 19 (2), 239–280. doi:10.2174/092986712803414213
Keywords: epilepsy, triazole, anticonvulsant, GABA, benzodiazepine (BZD) receptors, docking
Citation: Song M, Zhao W, Zhu Y, Liu W, Deng X and Huang Y (2022) Design, Synthesis, and Evaluation of Anticonvulsant Activities of New Triazolopyrimidine Derivatives. Front. Chem. 10:925281. doi: 10.3389/fchem.2022.925281
Received: 21 April 2022; Accepted: 16 May 2022;
Published: 23 June 2022.
Edited by:
Kaushik Chanda, VIT University, IndiaReviewed by:
Michal Szostak, Rutgers University, United StatesDaniel J. Canney, Temple University, United States
Copyright © 2022 Song, Zhao, Zhu, Liu, Deng and Huang. This is an open-access article distributed under the terms of the Creative Commons Attribution License (CC BY). The use, distribution or reproduction in other forums is permitted, provided the original author(s) and the copyright owner(s) are credited and that the original publication in this journal is cited, in accordance with accepted academic practice. No use, distribution or reproduction is permitted which does not comply with these terms.
*Correspondence: Xianqing Deng, dengxianqing1121@126.com; Yushan Huang, 3218680@qq.com