- National Metrology Institute of Japan (NMIJ), National Institute of Advanced Industrial Science and Technology (AIST), Tsukuba, Japan
Nitrous oxide (N2O) was investigated as the reaction gas for the determination of rare earth elements (REEs) by inductively coupled plasma–tandem quadrupole mass spectrometry (ICP-QMS/QMS). The use of N2O as the reaction gas apparently improved the yields of mM16O+ for Eu and Yb in the reaction cell. As a result, the sensitivities for measurement of Eu and Yb were apparently improved in comparison to those obtained with O2 as the reaction gas. A high sensitivity measurement of the whole set of REEs was achieved, providing a typical sensitivity of 300,000 CPS mL/ng for REEs measured with an isotope having isotopic abundance close to 100%. The use of N2O as the reaction gas helped suppress Ba-related spectral interferences with the measurement of Eu, permitting the measurement of Eu in a natural sample without mathematic correction of spectral interferences. The detection limits (unit, pg/mL) for 14 REEs (except for Pm) from La to Lu were 0.028, 0.018, 0.006, 0.026, 0.006, 0.010, 0.017, 0.006, 0.016, 0.010, 0.016, 0.004, 0.023, and 0.012, respectively. The validity of the present method was confirmed by determining REEs in river water-certified reference materials, namely, SLRS-3 and SLRS-4.
Introduction
Rare earth elements (REEs) are a group of 17 transition metals, including Sc, Y, and 15 lanthanides, in the periodic table of chemical elements (Balaram, 2019). Nevertheless, the lanthanides are more often referred to as REEs in many research works, while Pm is usually not investigated due to the lack of a natural and stable isotope. In the present work, REEs stand for the lanthanides except for Pm.
Due to the specific physicochemical properties of REEs, they are usually studied as a group of tracers for geological, hydrological, environmental, and fossil fuel research (Zuma et al., 2020; Wysocka, 2021). Acknowledging the capabilities for multi-elemental analysis, lower detection limits at ng/kg or even pg/kg levels, and commercial availability in common chemical analysis laboratories, inductively coupled plasma mass spectrometry (ICP-MS) is becoming a dominant approach for quantitative analysis of REEs in various samples (Bandura et al., 2006).
The extremely high temperature of the argon plasma provides an ionization efficiency for all REEs close to 100%, permitting the high sensitivity measurements by ICP-MS. At the same time, polyatomic spectral interferences such as oxide and hydroxide are usually problematic for accurate quantitation of REEs in natural samples, for example, 137Ba16O+ and 136Ba16O1H+ interfering with the measurement of 153Eu+, and 139La16O+ interfering with the measurement of 155Gd+.
There are majorly three ways to solve the problem caused by spectral interferences. One is the mathematic correction based on the ratio of the signal intensity of an interfering polyatomic ion (for example, 137Ba16O+) to that of an interferent ion (for example, 137Ba+) (Seregina et al., 2018; Zhao et al., 2019; Carvalho et al., 2020; Kunzetsova et al., 2021). Mathematic correction is a straightforward way of canceling spectral interferences in the measurements by ICP-MS. However, the accuracy and the precision of an analytical result may be greatly deteriorated in case of a relatively large portion (for example, over 30%) of spectral interference being corrected due to the variance in the signal intensity of the interfering spectrum. The second one is suppressing the formation of an interfering polyatomic ion by removing an interferent element (for example, Ba) from REEs through chemical separation (Watanabe et al., 2018; Ito et al., 2019; Barrat et al., 2020; Bradley et al., 2020) or by using a desolvating device to remove water contents introduced into the argon plasma (to suppress the formation of solvent related ions, for example, O+, H+, and OH+) (Giardi et al., 2018; Gatiboni et al., 2020; Shen et al., 2022). The third one is separating an interfering polyatomic ion from an REE of interest by high-resolution ICP-MS (Giardi et al., 2018; Barrat et al., 2020; Bradley et al., 2020; Kiruba et al., 2021), collision/reaction cell ICP-MS (Gueguen et al., 2009; Arslan et al., 2018; Rojano et al., 2019; Trommetter et al., 2020; Baghaliannejad et al., 2021), and tandem quadrupole ICP-MS with a reaction cell (ICP-QMS/QMS) (Galusha et al., 2018; Zhang et al., 2019; Zhu, 2020; Zhu et al., 2021).
A previous work by the present author showed that ICP-QMS/QMS provides the best separation of interfering polyatomic ion from an REE of interest by measuring at a so-called mass-shift mode with oxygen as the reaction gas (Zhu, 2020). In ICP-QMS/QMS, an octopole reaction cell is sandwiched by using two quadrupole analyzers. For the measurement of REEs at the mass-shift mode, the parameters for the first quadrupole (QMS1) were set to permit the pass of an ion of interest, for example, mass to charge ratio (m/z) = 139 for 139La+ (along with 138Ba1H+), and to block ions with m/z ≠ 139. After entering the reaction cell, 139La+ reacted with O2 to form 139La16O+, while 138Ba1H+ did not form 138Ba1H16O+. These product ions were introduced to the second quadrupole (QMS2) whose parameters were set to permit the pass of an ion with m/z = 155. As a result, the 139La16O+ ions passed through and arrived at the detector, while the product ions of 138Ba1H+ were blocked by the second quadrupole.
The mass-shift mode by ICP-QMS/QMS with oxygen as the reaction gas was effective for separating spectral interferences in the measurement of REEs. However, since reactions for the formation of EuO+ and YbO+ are endothermic, the transference ratio from M+ to MO+ for Eu+ and Yb+ was significantly lower than that for other REEs, resulting in relatively lower sensitivities. Optimization of the octopole bias voltage helped to improve the formation of EuO+ and YbO+. Nevertheless, the transference ratio from M+ to MO+ for Eu+ and Yb+ was still apparently lower than that for other REEs (Zhu et al., 2021).
In addition to the commonly equipped reaction cell gases, namely, He, H2, O2, and NH3, N2O had been used in collision/reaction cell ICP-MS and ICP-QMS/QMS to improve the formation of oxide ions (Bandura et al., 2006; Harouaka et al., 2021). In the present work, N2O was investigated as the reaction cell gas for ICP-QMS/QMS to improve the formation of EuO+ and YbO+ to achieve the highest sensitivities for the measurement of all REEs. The optimized conditions were applied to the measurement of REEs in two natural water-certified reference materials, namely, SLRS-3 and SLRS-4.
Materials and Methods
Instruments
Measurements of REEs in the present work were carried out with an ICP-QMS/QMS instrument (Agilent 8800, Agilent Technologies Japan, Ltd.), for which the typical operating conditions are summarized in Table 1. These operating conditions were optimized to obtain the best performance for measuring REEs with the highest sensitivity. A Millipore purification system (Nihon Millipore Kogyo) was used to provide deionized water for the present experiment. A chemical balance (model XS205DU) purchased from METTLER TOLEDO was used for making samples and calibrating solutions, while the chemical balance was calibrated yearly by the Japan Calibration Service System (JCSS).
Chemicals and Samples
The following chemicals were purchased from Kanto Chemical Co., Inc.: single-element standard solutions (1,000 mg/L) of REEs and barium; Ultrapur® grade HNO3 (60% in mass). Two river water CRMs (SLRS-3 and SLRS-4) were purchased from the National Research Council of Canada and analyzed to confirm the validity of the present method. The CRM samples were acidified to 0.3 mol/L of nitric acid by adding concentrated nitric acid, where this dilution factor was calculated and applied to the results to obtain the initial concentrations of REEs. Calibrating solutions for measurement by ICP-QMS/QMS were also prepared in 0.3 mol/L of nitric acid. Standard N2O gas (99.5%, 8 kg/m2, 5 L) was purchased from AS ONE Corp. and used as the reaction cell gas for ICP-QMS/QMS.
Calibrating Method
The concentrations of REEs in the present work were obtained based on a standard addition method (Zhu et al., 2018). Spiked and non-spiked sub-samples were made for each sample and subjected to the measurement by ICP-QMS/QMS. The natural content of yttrium in the sample was used as the internal standard. The concentrations of REEs in a spiked sub-sample for standard addition were over two folds of those in the initial sample to ensure the precision of calibration.
Results and Discussion
Dependence of Relative Signal Intensities of La, Eu, and Yb on the Octopole Bias of ICP-QMS/QMS
Measurement of REEs by ICP-QMS/QMS at the mass-shift mode (mM+ → mM16O+) with O2 as the reaction gas was effective for separating spectral interferences. However, the production ratios of EuO+ and YbO+ were significantly lower than those of other REEs because the reactions for the formation of EuO+ and YbO+ were endothermic, while those for other REEs were exothermic. A relatively stronger negative voltage applied to the octopole bias resulted in limited improvement of signal intensities for the measurement of Eu and Yb (Zhu, 2021). In the present work, N2O was used as the reaction gas for ICP-QMS/QMS to improve the production ratios of oxide ions of Eu+ and Yb+, while La+ was investigated as a representative of other REEs.
The dependence of signal intensities of La, Eu, and Yb on the octopole bias of ICP-QMS/QMS was investigated with O2 and N2O, respectively, as the reaction gases. The results are plotted in Figure 1. The default value of the octopole bias (suggested for mass-shift measurement with O2 as the reaction gas) was −5 V, and the range for octopole bias investigated in the present work was from −25 V to −5 V with a footstep of 5 V.
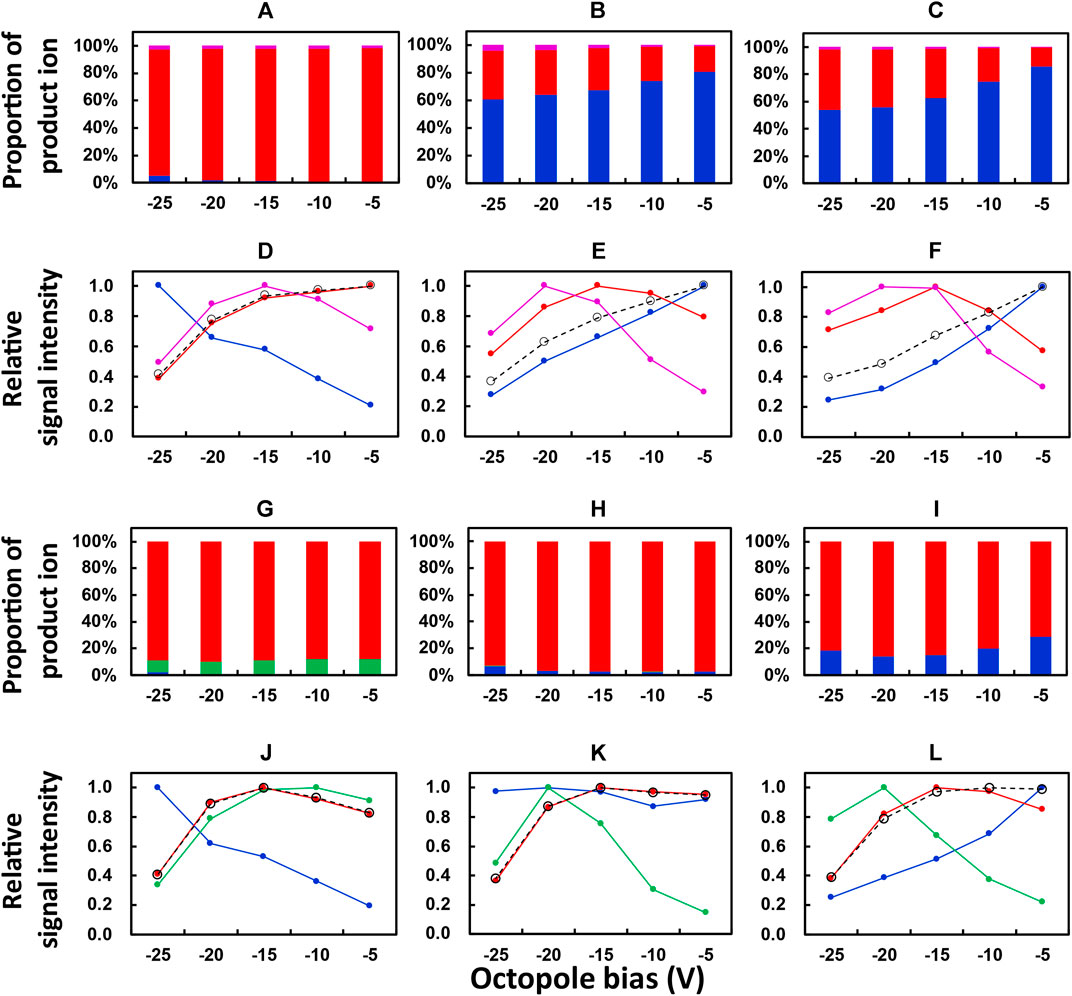
FIGURE 1. Dependence of the proportion of product ions and relative signal intensities on the octopole bias of ICP-QMS/QMS. Reaction gas: O2 for (A–F); N2O for G to L. Isotope monitored: 139La+ for (A,D,G,J); 153Eu+ for (B,E,H,K); 172Yb+ for (C,F,I,L). Color of the plot: blue, mM+; red, mM16O+; purple, mM16O2 +; green, mM14N+; black, the sum of (mM+, mM16O+, and mM16O2 +) or (mM+, mM16O+, and mM14N+). Reproducibility of the data, relative standard deviation under 2 %; n = 10.
A preliminary test in the present work was performed to check the major product ions from an REE ion mM+ when O2 and N2O were, respectively, used as the reaction gases. The results showed that the major product ions were mM+, mM16O+, and mM16O2+ and mM+, mM16O+, and mM14N+, respectively. Therefore, a study on the dependence of relative signal intensities on octopole bias was focused on these ions. Figures 1A–C and Figures 1G–I showed the proportion of product ions (for example, 139La+, 139La16O+, 139La16O2+) in the sum of those obtained from one precursor ion (for example, 139La+). Figures 1D–F and Figures 1J–L showed the relative signal intensity, which was calculated as the relative value of the signal intensity for an ion in comparison to its maximum value in the range of the octopole bias investigated. The results for Figures 1A to 1F and 1G to 1L were, respectively, obtained with O2 and N2O as the reaction gases.
As can be seen from Figures 1A–C, the dominating product ions from 139La+, 153Eu+, and 172Yb+ obtained at an octopole bias of −5 V were 139La16O+, 153Eu+, and 172Yb+, respectively. This can be attributed to the exothermic producing reaction of 139La16O+ and the endothermic ones for 153Eu16O+ and 172Yb16O+, when O2 was used as the reaction gas. When the octopole bias was changed to a stronger negative voltage until −25 V, the proportions of 153Eu16O+ and 172Yb16O+ increased from around 20% to around 40%, while that of 139La16O+ was not varied apparently. These results indicated that a stronger negative voltage of the octopole bias improved the relative yields of mM16O+ ions whose producing reactions are endothermic, which can be attributed to the increase of the velocity and the resulting collision energy of the precursor ion entering the reaction cell. However, such an increase in the velocity also results in a shorter retention time in the reaction cell and a lower reaction rate of an exothermic reaction. This contributed to the decrease in the relative signal intensity of 139La16O+ and the increase in that of 139La+ when the octopole bias changed from −5 V to a stronger negative voltage until −25 V, as shown in Figure 1D. By contrast, the maximum values of relative signal intensities of 153Eu16O+ and 172Yb16O+ (Figures 1E,F) were obtained at an octopole bias of −15 V, which can be attributed to the increased yields of these ions due to the elevated collision energy in comparison to the octopole bias of −5 V. Moreover, the decrease in the sum of relative signal intensities of product ions (black plots, Figures 1D–F) may indicate the decrease in transmittance of the ions when a stronger negative voltage was applied to the octopole bias. Based on these results, an octopole bias of −15 V was selected as the compromised optimum condition for measuring the whole set of REEs at relatively higher sensitivities.
The results obtained with N2O as the reaction gas are plotted in Figures 1G–L in a similar way to those in Figures 1A–F for the results obtained with O2. It is notable that mM14N+ ions are plotted in Figures 1G–L instead of mM16O2+ ions plotted in Figures 1A–F.
It can be seen from Figure 1G that the major product ion from 139La+ was 139La16O+ when N2O was used as the reaction gas, with a portion comparable to that obtained with O2 as the reaction gas (Figure 1A). By contrast, instead of the dominating production ions as 153Eu+ (Figure 1B) and 172Yb+ (Figure 1C) obtained with O2 as the reaction gas, 153Eu16O+ (Figure 1H) and 172Yb16O+ (Figure 1I) accounted for the dominating portions when N2O was used as the reaction gas. This can be attributed to the decrease in reaction energy for producing 153Eu16O+ and 172Yb16O+ when N2O was used instead of O2 (Harouaka et al., 2021).
As can be seen in Figures 1J–L, the highest relative signal intensities of 139La16O+, 153Eu16O+, and 172Yb16O+ were observed at an octopole bias of −15 V, which was selected as the optimum condition for measuring the whole set of REEs with N2O as the reaction gas.
Comparison of Normalized Sensitivities for the Measurement of REEs by ICP-QMS/QMS
The sensitivity for measuring an element by ICP-QMS/QMS depends on the sample uptake rate, the ionization rate, the abundance of the isotope selected for measurement, the transmittance in the ion lens system, and the yield of the product ion in the reaction cell. In the case of measuring REEs, the parameters for all REEs are quite similar, except for the abundance of the isotope and the yield of the product ion.
Therefore, normalized sensitivities of REEs were obtained to cancel the effect of the abundance of the isotope and to elucidate the contribution of the improved yield of the product ion by using N2O as the reaction gas. A normalized sensitivity (S*) was calculated from the initial sensitivity (S0) and the abundance of an isotope (A) based on Eq. 1. If the yields of product ions of interest were close to 100% for all REEs, the normalized sensitivities are expected to be close to one another.
The normalized sensitivities for REEs obtained in the present work are plotted in Figure 2, with O2 and N2O, respectively, used as the reaction gases. The octopole bias was set to -15 V for both O2 and N2O. As can be seen from Figure 2, the normalized sensitivities for all REEs were generally at a similar level when N2O was used as the reaction gas. The relatively lower normalized sensitivities of La and Ce might be attributed to the formation of mM14N+ ions (over 10% of the product ions), as indicated in Figure 1G.
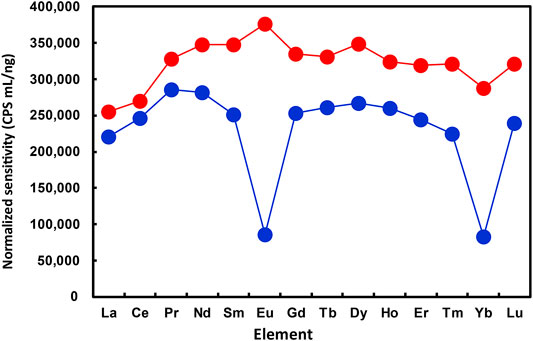
FIGURE 2. Comparison of normalized sensitivities for REEs obtained at different operating conditions of ICP-QMS/QMS. Reaction gas: O2 for blue plots; N2O for red plots. Reproducibility of the data, relative standard deviation under 2 %; n = 10.
By contrast, the normalized sensitivities of Eu and Yb were apparently lower than those of other REEs, when O2 was used as the reaction gas. Moreover, the relative sensitivities for all REEs obtained with N2O as the reaction gas were higher than those with O2 as the reaction gas. These results indicated that the optimized operating condition with N2O as the reaction gas provided higher yields of mM16O+ ions for all REEs and helped achieve higher sensitivities for measuring the whole set of REEs by ICP-QMS/QMS.
Ba-Related Spectral Interferences With the Measurement of Eu by ICP-QMS/QMS
Due to relatively smaller differences (one to two orders of magnitudes) among the concentrations of REEs in a natural sample, spectral interferences of oxide ions of lighter REEs (with a smaller atomic number) with the measurement of heavier REEs (with a larger atomic number) can be effectively suppressed by measuring at the mass-shift mode of ICP-QMS/QMS with O2 as the reaction gas. However, the concentrations of Ba in natural samples are usually much higher (for example, over four orders of magnitudes) than those of Eu, whose both stable isotopes (151Eu and 153Eu) suffer from spectral interferences from oxides and hydroxides of Ba. As a result, mathematical correction of Ba-related spectral interferences is still required even for the measurement by ICP-QMS/QMS with O2 as the reaction gas (Zhu and Itoh, 2021).
Ba-related spectral interferences with the measurement of 153Eu+ were further investigated in the present work, with O2 and N2O as the reaction gases, respectively. The investigation was carried out by introducing 10 mg/L Ba standard solution to the ICP-QMS/QMS and measuring the product ions of 153M+ (m/z = 153 for QMS1) at the m/z range of 160–180 for QMS2, covering the spectra of 153Eu16O+. The results are plotted in Figure 3.
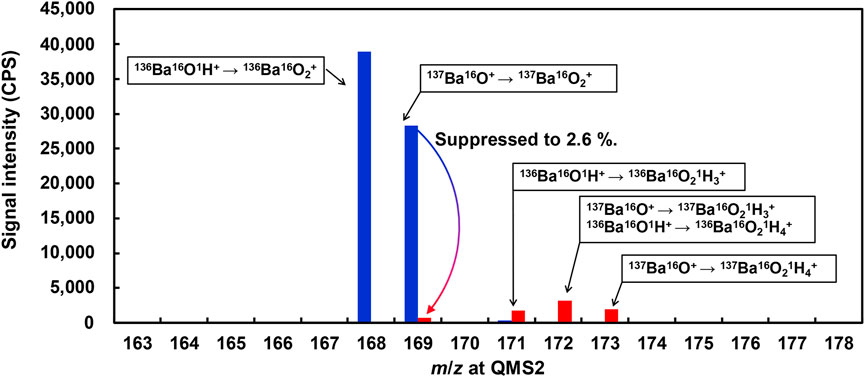
FIGURE 3. Comparison of Ba-related spectral interferences on the measurement of 153Eu+ by ICP-QMS/QMS. Reaction gas: O2 for blue plots; N2O for red plots. Octopole bias: −15 V. Seeing for QMS1, m/z = 153. Reproducibility of the data, relative standard deviation under 2 %; n = 10
As can be seen from Figure 3, the major product ions obtained with O2 as the reaction gas were 168M+ and 169M+, which can be attributed to 136Ba16O2+ and 137Ba16O2+, respectively. By contrast, the major product ions obtained with N2O as the reaction gas were 169M+, 171M+, and 172M+ and 173M+, which can be attributed to 137Ba16O2+, 136Ba16O2H3+ (137Ba16O2H3+ + 136Ba16O2H4+), and 137Ba16O2H4+, respectively. The source of hydrogen for product ions obtained with N2O as the reaction gas might be attributed to gaseous impurities.
It is notable that the signal intensity of 137Ba16O2+ was suppressed to 2.6% by using N2O as the reaction gas instead of O2, indicating much less spectral interferences with the measurement of 153Eu16O+.
Analytical Figures of Merits for Measurement of REEs by ICP-QMS/QMS
Sensitivity, blank equivalent concentration (BEC), and detection limit (DL) are usually provided as analytical figures of merits for the measurement of the elements by ICP-MS. These parameters are evaluated and summarized in Table 2, respectively, with O2 and N2O as the reaction gases.
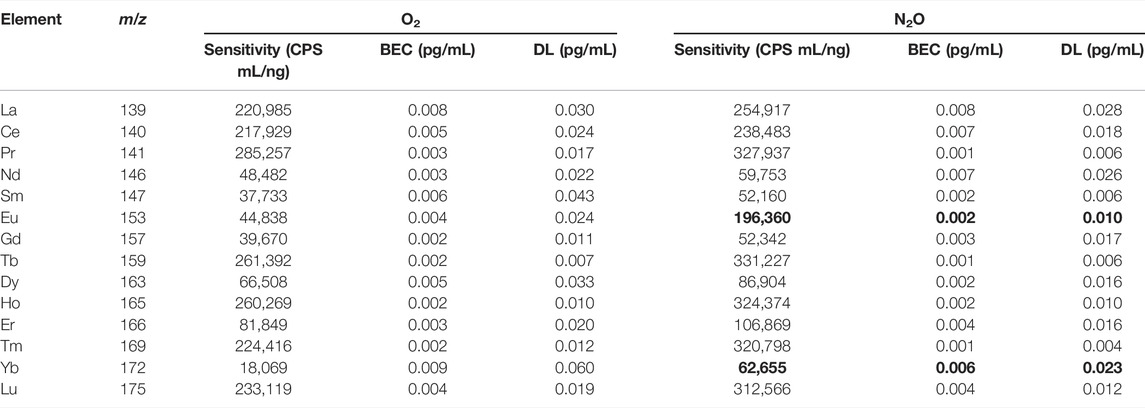
TABLE 2. Comparison of sensitivity, BEC, and DL values for REEs measured by ICP-QMS/QMS with O2 and N2O, respectively, as the reaction gases (octopole bias, -15 V).
The sensitivity was obtained as the signal intensity corresponding to each of 1.0 ng/mL REEs. A BEC value was obtained as the concentration equivalent to the average of five measurements of 0.3 mol/L HNO3 solution, where each measurement contains 10 repetitions (1 s/repetition) for each REE. A DL value was obtained as the concentration corresponding to 3-fold of the maximum standard deviation obtained in five measurements of the 0.3 mol/L HNO3 solution.
As can be seen from Table 2, the sensitivity, BEC, and DL values for each REE obtained with N2O as the reaction gas were generally superior to those obtained with O2, especially for Eu and Yb due to the improved yields of mM16O+ ions in the reaction cell. It is notable that the sensitivities summarized in Table 2 were the initial values without normalization to the isotopic abundances. As a result, the sensitivity depended on the isotopic abundance of an isotope measured. When N2O was used as the reaction gas and an REE was measured at an isotope with an isotopic abundance of 100%, the typical sensitivity was around 300,000 CPS mL/ng. The BEC and DL values for each REE with N2O as the reaction gas were, respectively, lower than 0.01 pg/mL and 0.03 pg/mL, sufficiently low for the direct measurement of REEs in most natural water samples. The data for Eu and Yb with N2O as the reaction gas are shown in bold fonts in Table 2 to highlight the improved analytical performance.
Results for the Recovery Test of Spiked REEs in River Water CRMs
Recovery tests of spiked REEs in river water CRMs were carried out by adding a mixed solution of REEs into river water CRMs, SLRS-3 and SLRS-4, respectively. The concentration of each REE in these spiked samples was elevated by 200 pg/mL. The concentrations of REEs in these spiked samples were determined along with those in the non-spiked SLRS-3 and SLRS-4 samples. A recovery value (R) was calculated based on Eq. 2, where,
The results of recovery tests are summarized in Table 3. It can be seen from Table 3 that each recovery value is close to 100%, indicating that the concentrations of REEs spiked to the river water CRMs can be accurately determined by ICP-QMS/QMS with O2 or N2O as the reaction gas. It is notable that the standard deviation values for La, Ce, Pr, Tb, Ho, Tm, and Lu were around 1.0%, which can be attributed to the excellent sensitivities due to the isotopes measured having high isotopic abundance. The standard deviation values for other REEs were generally in the range of 1–2%, except for those of Yb (roughly 3%) measured with O2 as the reaction gas. Such relatively lower standard deviation values of Yb were improved to a level similar to those of other REEs when N2O was used as the reaction gas, attributable to the improved yields of 172Yb16O+ in the reaction cell.
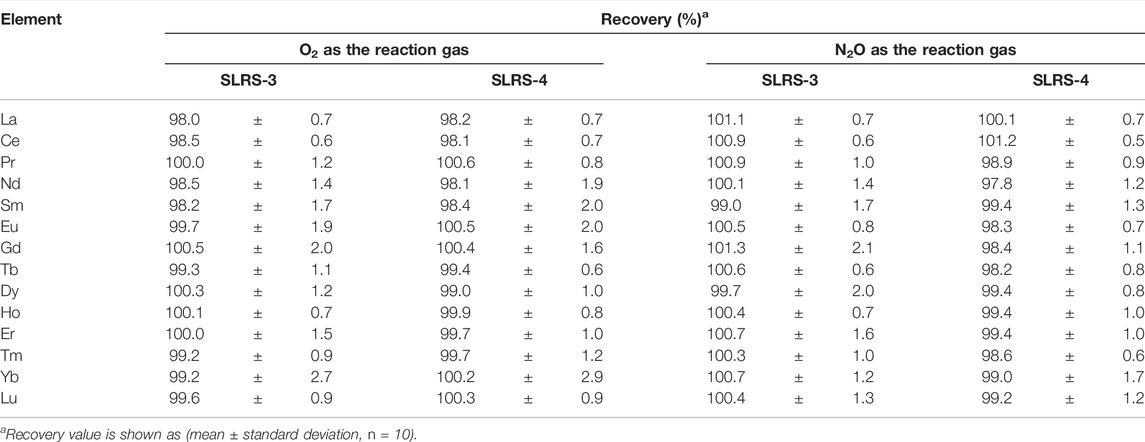
TABLE 3. Recoveries of spiked REEs in river water CRMs measured by ICP-QMS/QMS with O2 and N2O, respectively, as the reaction gases.
Analytical Results of REEs in River Water CRMs
The concentrations of REEs in two river water CRMs, namely, SLRS-3 and SLRS-4, were determined in the present work. The results are summarized in Table 4 along with reported data.
It can be seen from Table 4 that the results for each CRM obtained with O2 and N2O, respectively, as the reaction gas agreed with each other considering the standard deviation of each value. However, it is notable that the measurement of Eu with O2 as the reaction gas suffered approximately 10% of Ba-related spectral interference, which was mathematically corrected. By contrast, Ba-related spectral interference with the measurement of Eu was negligible when N2O was used as the reaction gas and did not require mathematic correction. The concentrations of Ba in both samples were approximately 15 ng/mL, roughly four orders of magnitude higher than the concentration of Eu.
The present results for REEs in SLRS-3 were generally close to those reported by Brenner et al. (1998), who applied mathematic corrections to the measurements of La, Ce, and Eu regarding Ba-related spectral interferences. The DLs for REEs achieved by Brenner et al. (1998) were in the range from 0.02 pg/mL for Sm to 0.2 pg/mL for Nd, roughly 10-fold higher than those obtained in the present work. By contrast, the results by Halicz et al. (1999) showed slightly higher values for most REEs except for Sm, Eu, Tm, and Lu, perhaps indicating some spectral interferences, even mathematic correction applied to the measurements of 151Eu+ regarding the spectral interference by 135Ba16O+. The DLs for REEs obtained by Halicz et al. (1999) were in the range from 0.005 pg/mL for Tm to 0.1 pg/mL for Nd. Brenner et al. (1998) and Halicz et al. (1999), respectively, used a Meinhard type pneumatic concentric nebulizer and an ultrasonic nebulization system to introduce the samples to their ICP-MS instruments. The present results for REEs in SLRS-4 obtained in the present work agreed with the compiled data based on multiple works (Zhu et al., 2021).
These results for REEs in SLRS-3 and SLRS-4 showed that the present method is effective for the determination of REEs in river water samples.
Conclusion
The application of N2O as the reaction gas for ICP-QMS/QMS provided excellent analytical figures of merits for the determination of REEs. In comparison to O2 that was usually used as the reaction gas, N2O improved the yields of mM16O+ ions for Eu and Yb in the reaction cell and permitted measuring them at much higher sensitivities.
The application of N2O as the reaction gas also contributed to the suppression of Ba-related spectral interferences with 153Eu+ to 2.6% of that observed with O2 as the reaction gas. This merit permitted the measurement of Eu without mathematic correction even with Ba concentrations over four orders of magnitude higher.
The analytical results for spiked REEs in SLRS-3 and SLRS-4 provided recovery values quite close to 100%. The concentrations of REEs in SLRS-3 and SLRS-4 determined by the present method were incidence with those reported, indicating the validity of the method.
Data Availability Statement
The original contributions presented in the study are included in the article, further inquiries can be directed to the corresponding author.
Author Contributions
YZ is the sole author of the present article.
Funding
The present work is partly supported by the Grant-in-Aid for Scientific Research (C) (No. 22K05181) provided by the Japan Society for the Promotion of Science (JSPS).
Conflict of Interest
The author declares that the research was conducted in the absence of any commercial or financial relationships that could be construed as a potential conflict of interest.
Publisher’s Note
All claims expressed in this article are solely those of the authors and do not necessarily represent those of their affiliated organizations, or those of the publisher, the editors, and the reviewers. Any product that may be evaluated in this article, or claim that may be made by its manufacturer, is not guaranteed or endorsed by the publisher.
Acknowledgments
The author appreciates the help from Mr. Yasuyuki Shikamori and Kazumi Nakano (both of Agilent Technologies, Japan) for their constructive discussion on the mechanism of ICP-QMS/QMS.
References
Arslan, Z., Oymak, T., and White, J. (2018). Triethylamine-assisted Mg(OH)2 Coprecipitation/preconcentration for Determination of Trace Metals and Rare Earth Elements in Seawater by Inductively Coupled Plasma Mass Spectrometry (ICP-MS). Anal. Chim. Acta 1008, 18–28. doi:10.1016/j.aca.2018.01.017
Baghaliannejad, R., Aghahoseini, M., and Amini, M. K. (2021). Determination of Rare Earth Elements in Uranium Materials by ICP-MS and ICP-OES after Matrix Separation by Solvent Extraction with TEHP. Talanta 222, 121509. doi:10.1016/j.talanta.2020.121509
Balaram, V. (2019). Rare Earth Elements: A Review of Applications, Occurrence, Exploration, Analysis, Recycling, and Environmental Impact. Geosci. Front. 10, 1285–1303. doi:10.1016/j.gsf.2018.12.005
Bandura, D. R., Baranov, V. I., Litherland, A. E., and Tanner, S. D. (2006). Gas-phase Ion-Molecule Reactions for Resolution of Atomic Isobars: AMS and ICP-MS Perspectives. Int. J. Mass Spectrom. 255-256, 312–327. doi:10.1016/j.ijms.2006.06.012
Barrat, J.-A., Bayon, G., Wang, X., Le Goff, S., Rouget, M.-L., Gueguen, B., et al. (2020). A New Chemical Separation Procedure for the Determination of Rare Earth Elements and Yttrium Abundances in Carbonates by ICP-MS. Talanta 219, 121244. doi:10.1016/j.talanta.2020.121244
Bradley, V. C., Manard, B. T., Roach, B. D., Metzger, S. C., Rogers, K. T., Ticknor, B. W., et al. (2020). Rare Earth Element Determination in Uranium Ore Concentrates Using Online and Offline Chromatography Coupled to ICP-MS. Minerals 10, 55. doi:10.3390/min10010055
Brenner, I. B., Liezers, M., Godfrey, J., Nelms, S., and Cantle, J. (1998). Analytical Characteristics of a High Efficiency Ion Transmission Interface (S Mode) Inductively Coupled Plasma Mass Spectrometer for Trace Element Determinations in Geological and Environmental Materials. Spectrochim. Acta Part B At. Spectrosc. 53, 1087–1107. doi:10.1016/S0584-8547(98)00173-6
Carvalho, L., Reis, A. T., Soares, E., Tavares, C., Monteiro, R. J. R., Figueira, P., et al. (2020). A Single Digestion Procedure for Determination of Major, Trace, and Rare Earth Elements in Sediments. Water Air Soil Pollut. 231, 541. doi:10.1007/s11270-020-04900-8
Galusha, A. L., Kruger, P. C., Howard, L. J., and Parsons, P. J. (2018). An Assessment of Exposure to Rare Earth Elements Among Patients Receiving Long-Term Parenteral Nutrition. J. Trace Elem. Med. Biol. 47, 156–163. doi:10.1016/j.jtemb.2018.02.013
Gatiboni, T. L., Iop, G. D., Diehl, L. O., Flores, E. M. M., Muller, E. I., and Mello, P. A. (2020). An Ultrasound‐assisted Sample Preparation Method of Carbonatite Rock for Determination of Rare Earth Elements by Inductively Coupled Plasma Mass Spectrometry. Rapid Commun. Mass Spectrom. 34, e8732. doi:10.1002/rcm.8732
Giardi, F., Traversi, R., Becagli, S., Severi, M., Caiazzo, L., Ancillotti, C., et al. (2018). Determination of Rare Earth Elements in Multi-Year High-Resolution Arctic Aerosol Record by Double Focusing Inductively Coupled Plasma Mass Spectrometry with Desolvation Nebulizer Inlet System. Sci. Total Environ. 613-614, 1284–1294. doi:10.1016/j.scitotenv.2017.09.247
Guéguen, F., Nonell, A., Granet, M., Favre, G., Isnard, H., and Chartier, F. (2009). Eu Isotopic Measurements with in situEu/Gd/Sm Separation Using O2as a Reactant Gas in Collision/reaction Cell Based MC-ICP-MS. J. Anal. At. Spectrom. 25, 201–205. doi:10.1039/b912605h
Halicz, L., Segal, I., and Yoffe, O. (1999). Direct REE Determination in Fresh Waters Using Ultrasonic Nebulization ICP-MS. J. Anal. At. Spectrom. 14, 1579–1581. doi:10.1039/A808387H
Harouaka, K., Allen, C., Bylaska, E., Cox, R. M., Eiden, G. C., di Vacri, M. L., et al. (2021). Gas-phase Ion-Molecule Interactions in a Collision Reaction Cell with Triple Quadrupole-Inductively Coupled Plasma Mass Spectrometry: Investigations with N2O as the Reaction Gas. Spectrochim. Acta Part B At. Spectrosc. 186, 106309. doi:10.1016/j.sab.2021.106309
Ito, S., Okada, T., Takaku, Y., Harada, M., Ikeda, M., Kishimoto, Y., et al. (2019). Development of a Method for Measuring Rare Earth Elements in the Environment for Future Experiments with Gadolinium-Loaded Detectors. Prog. Theor. Exp. Phys. 2019, 063H03. doi:10.1093/ptep/ptz060
Kiruba, K., Satyanarayanan, M., Sawant, S. S., Subramanyam, K. S. V., Dasaram, B., and Vamsi Krishna, G. (2021). New Soil Reference Material Validation for Trace and Rare-Earth Elements by High-Resolution Inductively Coupled Plasma Mass Spectrometry. Mapan 36, 147–156. doi:10.1007/s12647-020-00398-x
Kuznetsova, O. V., Dushenko, N. V., and Timerbaev, A. R. (2021). How Feasible Is Direct Determination of Rare Earth Elements in Seawater by ICP-MS? Anal. Sci. 37, 1633–1636. doi:10.2116/analsci.21N012
Sandoval Rojano, W. J., dos Anjos, T., Duyck, C. B., and Saint'Pierre, T. D. (2019). Determination of Rare Earth Elements in Environmental Samples with High Concentrations of Barium by Quadrupole Inductively Coupled Plasma Mass Spectrometry. Microchem. J. 149, 104026. doi:10.1016/j.microc.2019.104026
Seregina, I. F., Volkov, A. I., Ossipov, K., and Bolshov, M. A. (2018). Characterization of REE-Nb Ores by a Combination of Spectrochemical Techniques. Spectrochim. Acta Part B At. Spectrosc. 148, 172–182. doi:10.1016/j.sab.2018.06.018
Shen, S., Krogstad, E., Conte, E., and Brown, C. (2022). Rapid Unseparated Rare Earth Element Analyses by Isotope Dilution Multicollector Inductively Coupled Plasma Mass Spectrometry (ID-MC-ICP-MS). Int. J. Mass Spectrom. 471, 116726. doi:10.1016/j.ijms.2021.116726
Trommetter, G., Dumoulin, D., and Billon, G. (2020). Direct Determination of Rare Earth Elements in Natural Water and Digested Sediment Samples by Inductively Coupled Plasma Quadrupole Mass Spectrometry Using Collision Cell. Spectrochim. Acta Part B At. Spectrosc. 171, 105922. doi:10.1016/j.sab.2020.105922
Watanabe, T., Saito-Kokubu, Y., Murakami, H., and Iwatsuki, T. (2018). Onsite Chelate Resin Solid-phase Extraction of Rare Earth Elements in Natural Water Samples: its Implication for Studying Past Redox Changes by Inorganic Geochemistry. Limnology 19, 21–30. doi:10.1007/s10201-017-0513-3
Wysocka, I. (2021). Determination of Rare Earth Elements Concentrations in Natural Waters - A Review of ICP-MS Measurement Approaches. Talanta 221, 121636. doi:10.1016/j.talanta.2020.121636
Zhang, Y., Pan, Z., Jiao, P., Ju, J., He, T., Duan, T., et al. (2019). Solvent Extraction ICP-MS/MS Method for the Determination of REE Impurities in Ultra-high Purity Ce Chelates. At.Spectrosc. 40, 167–172. doi:10.46770/AS.2019.05.003
Zhao, W., Zong, K., Liu, Y., Hu, Z., Chen, H., and Li, M. (2019). An Effective Oxide Interference Correction on Sc and REE for Routine Analyses of Geological Samples by Inductively Coupled Plasma-Mass Spectrometry. J. Earth Sci. 30, 1302–1310. doi:10.1007/s12583-019-0898-5
Zhu, Y. (2020). Determination of Rare Earth Elements in Seawater Samples by Inductively Coupled Plasma Tandem Quadrupole Mass Spectrometry after Coprecipitation with Magnesium Hydroxide. Talanta 209, 120536. doi:10.1016/j.talanta.2019.120536
Zhu, Y., and Itoh, A. (2021). Pseudo Isotope Dilution (PID) as an Approach for Correcting Barium-Related Spectral Interferences on the Measurement of Europium by Inductively Coupled Plasma Mass Spectrometry (ICP-MS). Anal. Chim. Acta 1180, 338854. doi:10.1016/j.aca.2021.338854
Zhu, Y., Nakano, K., Shikamori, Y., and Itoh, A. (2021). Direct Determination of Rare Earth Elements in Natural Water Samples by Inductively Coupled Plasma Tandem Quadrupole Mass Spectrometry with Oxygen as the Reaction Gas for Separating Spectral Interferences. Spectrochim. Acta Part B At. Spectrosc. 179, 106100. doi:10.1016/j.sab.2021.106100
Zhu, Y., Nakano, K., Wang, Z., Shikamori, Y., Chiba, K., Kuroiwa, T., et al. (2018). Applications and Uncertainty Estimation of Single Level Standard Addition Method ICP-MS for Elemental Analysis in Various Matrix. Anal. Sci. 34, 701–710. doi:10.2116/analsci.18SBP09
Keywords: REEs, spectral interference, reaction cell, reaction gas, mass-shift, ICP-QMS/QMS
Citation: Zhu Y (2022) Determination of Rare Earth Elements by Inductively Coupled Plasma–Tandem Quadrupole Mass Spectrometry With Nitrous Oxide as the Reaction Gas. Front. Chem. 10:912938. doi: 10.3389/fchem.2022.912938
Received: 05 April 2022; Accepted: 29 April 2022;
Published: 29 June 2022.
Edited by:
Ottavia Giuffrè, University of Messina, ItalyReviewed by:
Paola Mello, Federal University of Santa Maria, BrazilFabio Mazzotti, University of Calabria Gargi, Italy
Copyright © 2022 Zhu. This is an open-access article distributed under the terms of the Creative Commons Attribution License (CC BY). The use, distribution or reproduction in other forums is permitted, provided the original author(s) and the copyright owner(s) are credited and that the original publication in this journal is cited, in accordance with accepted academic practice. No use, distribution or reproduction is permitted which does not comply with these terms.
*Correspondence: Yanbei Zhu, eWItemh1QGFpc3QuZ28uanA=