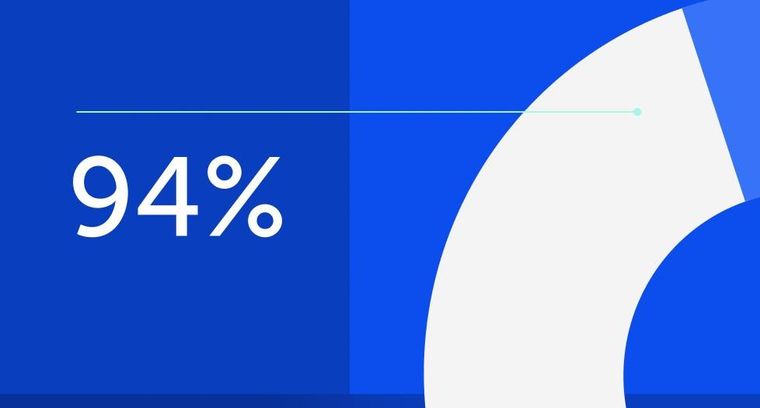
94% of researchers rate our articles as excellent or good
Learn more about the work of our research integrity team to safeguard the quality of each article we publish.
Find out more
ORIGINAL RESEARCH article
Front. Chem., 13 May 2022
Sec. Catalytic Reactions and Chemistry
Volume 10 - 2022 | https://doi.org/10.3389/fchem.2022.912550
This article is part of the Research TopicCatalysis Rising Stars in ChinaView all 10 articles
Metal-support interaction has been one of the main topics of research on supported catalysts all the time. However, many other factors including the particle size, shape and chemical composition can have significant influences on the catalytic performance when considering the role of metal-support interaction. Herein, we have designed a series of CuxO/ZnO catalysts as examples to quantitatively investigate how the metal-support interaction influences the catalytic performance. The electronic metal-support interactions between CuxO and ZnO were regulated successfully without altering the structure of CuxO/ZnO catalyst. Due to the lower work function of ZnO, electrons would transfer from ZnO to CuO, which is favorable for the formation of higher active Cu species. Combined experimental and theoretical calculations revealed that electron-rich interface result from interaction was favorable for the adsorption of oxygen and CO oxidation reaction. Such strategy represents a new direction to boost the catalytic activity of supported catalysts in various applications.
For decades, the Cu/ZnO system has attracted fundamental interest concerning its unique performance in CH3OH synthesis, CO2 conversion, steam reforming reactions, and oxidation reactions (Behrens et al., 2012; Kuld et al., 2016; Jia et al., 2017; Ye et al., 2018; Jiang et al., 2020). However, the role of the interaction between the two species on the catalytic performance is still not fully understood (Kniep et al., 2004; Liao et al., 2011; Bikaljevic et al., 2019; Zhang et al., 2021). The investigation of this key feature for the performance of Cu/ZnO catalysts has often led to different conclusions. For example, the interface in Cu/ZnO catalysts is crucial for CH3OH synthesis via CO2 hydrogenation reaction. Although the exact nature of the interfacial sites is still under debate, two possible active sites are generally proposed: Cu-ZnO synergistic sites at the interface and Cu-Zn surface alloy sites (Nakamura et al., 1996; Fujitani and Nakamura, 2000; Kattel et al., 2017a). In other reactions having different activation and reaction conditions, the reconstruction of Cu and Zn species during the reaction is nonnegligible as well. The active species and valence states at the interface are different. Due to the complexity of the catalytic reaction mechanism, there is no unified opinion on the interaction between the two components in Cu/ZnO catalysts (Whittle et al., 2002; Kattel et al., 2017b; Yang et al., 2017).
In fact, revealing the specific structure-activity relationship of supported catalysts is a challenging task because their performance is affected by numerous factors, including the composition, specific surface area, and pore structure of the support, particle size, crystal structure, and morphology of the active metal, and the interaction between the support and the active metal (Li et al., 2018a; Lou et al., 2020a; Lou et al., 2020b; Song et al., 2021). Moreover, these factors generally affect each other. Therefore, to study the effect of one of these parameters while maintaining the other factors constant is not an easy task. For example, to study the pore structure of Al2O3 on the performance in the Fischer-Tropsch synthesis reaction of a Co/Al2O3 catalyst, a series of Al2O3 materials with different pore structures need to be synthesized for the first. However, Al2O3 supports with different specific surface area or external exposed surface were obtained, which could affect the dispersion degree and size of the Co particles, as well as the interaction between Co having different particle sizes and the Al2O3 support (Xiong et al., 2005; Liu et al., 2017). Too many influencing factors were introduced during the research, leading to a controversial result. Thus, well-defined catalytic materials and well-designed experiments are essential to obtain a reliable result (Zhang et al., 2014; Yang et al., 2018).
One of the best studied catalytic reactions from a fundamental scientific viewpoint is the CO oxidation reaction, which constitutes an effective pathway to remove exhaust gas (Doherty et al., 2020; Jing et al., 2020). For this reaction, Cu-based materials have been preferentially employed as primary catalysts due to their variable valence state, low temperature reducibility, and low cost (Jernigan and Somorjai, 1994). Although pristine ZnO catalysts lack substantial oxygen vacancy defects to facilitate an efficient CO oxidation, its strong metal-support interaction (SMSI) with expensive noble metals can improve the reaction performance effectively (Liu et al., 2012; Liu et al., 2018). In this context, the combination of ZnO with Cu can be envisaged as a low-cost alternative to catalyze the CO oxidation reaction. However, the role of the interaction between both components in the catalytic performance is still unclear. Thermal decomposition is one of the most effective methods to obtain highly homogeneous nanomaterials (Park et al., 2004). In this paper, a well-defined CuxO/ZnO catalyst was synthesized via a thermal decomposition method. By performing simple efficient treatments, the interaction between CuxO and ZnO was regulated successfully without altering the structure of the catalyst. Using these well-defined catalysts and a series of advanced characterization methods, the role of the interaction between CuxO and ZnO on the CO oxidation performance was investigated thoroughly.
Cu(acac)2 (98%; acac = acetylacetonate), benzylamine (98%), and the ZnO support (99%, 100–500 nm) were purchased from Aladdin Chemical Reagent Co., Ltd. Ethanol was purchased from Sinopharm.
CO (99.999% purity), Ar (99.999% purity), O2 (99.999% purity), and 10% O2/90% Ar gas mixture were purchased from Sichuan Tianyi Science and Technology Co., Ltd.
The CuxO/ZnO catalysts were synthesized via a thermal decomposition method, according to which CuxO nanoparticles were allowed to nucleate and grow on the ZnO surface as presented in Scheme 1. In a typical synthesis, 0.626 g of Cu(acac)2 (2.4 mmol), 1.8 g of ZnO, and 100 g of benzylamine were suspended in a round-bottomed flask equipped with a reflux condenser. The mixture was ultrasonically dispersed under vigorous stirring for 0.5 h. Subsequently, the obtained solution was heated to 190°C with stirring in an oil bath and refluxed for 2 h. After cooling down to room temperature, the sample was centrifuged and washed with ethanol five times. The obtained catalyst was divided into four equal parts, followed by drying at 200°C for 12, 24, 144, and 324 h in a vacuum drying oven, respectively. The obtained catalyst samples were denoted as CuxO/ZnO-12, CuxO/ZnO-24, CuxO/ZnO-144, and CuxO/ZnO-324 depending on the drying time.
X-ray powder diffraction (XRD) was performed on a Bruker D8 powder diffractometer using Cu Kα radiation (1.5404 Å), operated at 40 kV and 40 mA and a Vantec −1 detector. The size and morphology of the catalysts was investigated using a Tecnai G2 20 S-TWIN transmission electron microscope (TEM) equipped with an energy dispersive X-ray (EDX) spectroscope operated at 200 kV. H2 Temperature-programmed reduction (TPR) was conducted using a Zeton Altamira AMI-300 instrument equipped with a thermal conductivity detector. Prior to the measurement, the catalyst (0.05 g) was flushed with high-purity Ar at 150°C for 1 h and then cooled to 50°C. Then, the temperature was raised to 800°C (ramp rate 10°C min−1) by applying a flow of 10% H2/Ar at a flow rate of 30 ml min−1. Finally, the temperature was held at 800°C for 30 min. X-ray photoelectron spectroscopy (XPS) measurements were performed on a Thermal Electron VG multilab 2000 with an Al K α X-ray source under vacuum at 2 × 10–6 Pa (the binding energies were corrected using the C 1 s peak at 284.6 eV of the surface adventitious carbon). Electron paramagnetic resonance (EPR) spectra were recorded on a Bruker EMX spectrometer. The EPR experiments were conducted with a center field of 3507.815 G and a frequency of 9.83 GHz using an Elexsys probe head with 15 mg of sample placed in a 4 mm tube. In situ diffuse reflectance infrared Fourier transform (DRIFT) spectra of catalysts were collected on a Nicolet Fourier transform infrared spectrometer (NEXUS 470). Before data collection, the catalyst was pretreated with a 10% O2/Ar flow rate of 100 ml min−1 at 300°C for 1 h to remove absorbed residues and then cooled to 30°C. Pure Ar was introduced to remove O2 before CO adsorption experiments.
Density functional theory calculations were performed by PBE (Perdew–Burke–Ernzerhof) method with PAW (projected-augmentation wave) potentials in Vienna ab-initio simulation package program. Plane-wave energy cutoff was set to 400 eV with Gaussian smearing scheme (sigma = 0.05 eV) suitable for semi-conduntor. Total energy and residual atomic force were converged to 10^-4 and 0.03 eV/A.
The CO oxidation reaction was conducted in an atmospheric pressure fixed-bed flow quartz reactor. To prevent temperature gradients, 30 mg of catalyst was diluted with inert SiC powder (0.3 g). The catalyst was pretreated with a 10% O2/Ar flow rate of 100 ml min−1 at 300 °C for 1 h to remove any absorbed residues and then cooled to room temperature. For the CO oxidation reaction, the reaction temperature was ramped up from 25 to 400°C with a heating rate of 2°C min−1. A total flow rate of 100 ml min−1 was used, and the space velocity was 200,000 ml (gcat h)−1. The outlet gas composition was measured using a gas chromatograph Agilent 7890 BGC equipped with a thermal conductivity detector. The CO conversion was calculated as follows:
The CuxO/ZnO-12 catalyst was synthesized via a previously reported thermal decomposition approach (Lyu et al., 2019) and dried in a vacuum drying oven at 200°C for 12 h. In the XRD pattern, characteristic diffraction peaks attributed to ZnO (JCPDS # 36-1451) were observed, whereas no peaks corresponding to CuxO appeared (Supplementary Figure S1). This indicates that CuxO particles were well dispersed on the ZnO support. Scanning transmission electron microscopy (STEM) and EDX mapping was used to investigate the morphology and element distribution of the catalysts. The high-angle annular dark-field STEM (HAADF-STEM) images displayed in Figures 1A,B show that CuxO particles with an irregular morphology and broad size distribution were distributed uniformly on the ZnO surface. The selected-area electron diffraction (SEAD) results show that the catalyst was composed of Cu2O and ZnO (Figure 1D). Thus, the lattice fringes with a d-spacing of 0.26 nm correspond to the (002) planes of ZnO, and the 0.25 nm lattice fringes can be ascribed to the (111) planes of Cu2O. Figure 1C also reveals a distinct oxide layer on the surface of the particles resulting from the formation of CuO after exposure to air and a distinct interface between CuxO and ZnO.
FIGURE 1. (A) High-angle annular dark-field scanning transmission electron microscopy images, (B) energy dispersive X-ray mapping, (C) high-resolution transmission electron microscopy, and (D) selected-area electron diffraction patterns of CuxO/ZnO-12 catalyst.
To modify the interaction between the CuxO and ZnO components without affecting the structure of the catalyst, other three CuxO/ZnO samples were prepared using the same procedure described for CuxO/ZnO-12 except that the heating in the vacuum drying oven at 200°C and 0.08 MPa was conducted for 24, 144, and 324 h, respectively. According to Fick’s second law, a concentration gradient due to the different degree of doping with time would cause diffusion of the atoms of the two components into the lattices of each other. The surface reconstruction of the catalyst would be minimized under vacuum. Besides, since the temperature is much lower than the Tammann temperature of CuxO, the agglomeration of CuxO particles would be reduced (Imtiaz et al., 2016). As confirmed by the STEM images of CuxO/ZnO-24, CuxO/ZnO-144, and CuxO/ZnO-324 presented in Supplementary Figure S2–4, the dispersion of CuxO particles was not affected by the treatment time. The change in Cu loading and pore structure was negligible under the present mild treatment conditions.
To confirm whether the interaction between the two components in the catalysts was tuned after treatment, the catalysts were characterized by H2-TPR. As shown in Figure 2, all the catalysts exhibited two distinct peaks at 220°C–300°C, which are related to the reduction of different CuxO species. The main TPR peak at about 260–300°C can be ascribed to the reduction of bulk CuxO, and a smaller shoulder peak at about 220°C–250°C is attributable to the reduction of CuxO interacting with ZnO, which confirms the existence of a CuxO-ZnO interaction that facilitates the reduction of CuxO species in all the catalysts (Jun et al., 1998; Kniep et al., 2005). Interestingly, the TPR profiles of the whole series of catalysts exhibit similar peak shapes, but the peak position shifts to lower temperature upon increasing the heat treatment time, indicating that the strong interaction with ZnO can promote the reduction of CuxO effectively. This suggests strongly that the CuxO-ZnO interaction was regulated successfully. Thus, a batch of well-defined CuxO/ZnO catalysts with different interactions between components was obtained. It should be pointed out that other structural differences between these catalysts were negligible.
The CO oxidation reaction was conducted on a fix-bed reactor under a gas hourly space velocity of 200,000 ml gcat−1 h−1 (gcat, grams of catalyst), which matches standard vehicle exhaust conditions. The light-off curves of the CO conversions are shown in Figure 3. Overall, although the activity of the CuxO/ZnO catalysts was limited, significant differences between the catalysts were observed. Thus, the temperature at which CO conversion is 100% (T100) was 230°C for CuxO/ZnO-12, whereas it increased gradually with increasing the heat treatment time. The Arrhenius plots of the CO oxidation rates and the activation energies (Ea) are presented in Figure 3B. The CuxO/ZnO-324 sample showed the highest Ea (81.8 kJ/mol), which was much higher than that of CuxO/ZnO-12 (68.6 kJ/mol). The Ea increased monotonously with the drying time.
FIGURE 3. (A) CO conversion over the catalyst series as a function of the reaction temperature; (B) Arrhenius plots of the different catalysts.
The different catalytic performance can be ascribed to the structural differences of the catalysts, which are exclusively due to the interaction between the two components, as described above. Although the “metal-support interaction” is a relatively broad concept because the interaction between the two components can be expressed in many forms, it is known to affect the catalytic performance. In a recent review, de Jong et al. (van Deelen et al., 2019) described that several catalyst characteristics such as nanoparticle morphology, charge transfer, interfacial perimeter, chemical composition, and SMSI have a profound effect on the catalytic performance in different reactions. These phenomena are often related to each other and influence the catalytic performance in different degrees, depending on the catalyst and the reaction.
The adhesion energy at the metal-support interface has been reported to affect the shape of the nanoparticles, which has a strong influence on their catalytic performance because different shapes expose certain facets (Bratlie et al., 2007; Xie et al., 2009; Zhou and Li, 2012). Recently, Zhang at al. explored the active sites of a Cu2O catalyst in CO oxidation using a series of Cu2O particles with regular morphology as model materials, since cubic Cu2O particles with different sizes possess different face sites and edge sites (Zhang et al., 2019). Herein, high-resolution TEM (HRTEM) was used to investigate the morphology of the CuxO particles in each catalyst. As shown in Figure 1, the CuxO particles in the CuxO/ZnO-12 catalyst exhibited no special morphology, and no morphological changes were observed upon extending the treatment time (Supplementary Figure S2–4). Therefore, the CuxO morphology is not the reason for the different performance of the catalysts. The STEM result also allows excluding the influence of the interface perimeter between CuxO and ZnO because the size of the CuxO particles is similar in the whole catalyst series. This was also confirmed by the surface element composition data obtained by XPS, according to which the surface Cu content and Cu/ZnO ratio of all the catalysts were almost the same (Supplementary Table S1).
The term strong metal-support interaction refers to the coverage of metal nanoparticles by suboxides, which are generated from the support under reducing conditions as a result of the surface reconstruction of the catalyst in a specific atmosphere (Liu et al., 2015; Tang et al., 2017; Dong et al., 2020). In the present study, HRTEM was used to examine whether the CuxO particles in the used catalyst were covered by ZnO (Figure 4). After the reaction, the structure of the CuxO-ZnO interface remained virtually unchanged, and no covering was observed. Recently, Luo et al. studied the surface reconstruction of a Cu catalyst during CO oxidation using in situ aberration-corrected environmental TEM under O2 atmosphere (Luo et al., 2020). However, this nonreducing atmosphere may not be conducive to the formation of SMSI in current case (Yang et al., 2021).
FIGURE 4. High-angle annular dark-field scanning transmission electron microscopy images of (A) used CuxO/ZnO-12 catalyst, (B) used CuxO/ZnO-24 catalyst, and (C) used CuxO/ZnO-144 catalyst; high-resolution transmission electron microscopy images of (D) used CuxO/ZnO-12 catalyst, (E) used CuxO/ZnO-24 catalyst, and (F) used CuxO/ZnO-144 catalyst.
A solid-state reaction occurring between metal nanoparticles and the support results in the formation of new phases (Chaika et al., 2020). For Cu/ZnO catalysts, the formation of a Cu-Zn compound induced by hydrogen or a reducing atmosphere often occurs in hydrogenation reactions (Grunwaldt et al., 2000). Meanwhile, the Cu-Zn alloy was reported to be the active center in CO2 hydrogenation. In this study, the CuxO/ZnO compound was fabricated according to Fick’s law (Van Milligen et al., 2005), and its formation was confirmed via EPR spectroscopy. As presented in Figure 5, the spectra of all the catalysts exhibited a resonance at g = 1.953, which is attributable to shallow donor centers caused by interstitial Zn and surface O vacancies (Wen et al., 2020). This peak of CuxO/ZnO catalysts was relatively weaker than that of pristine ZnO and decreased slightly with increasing the heat treatment time. Meanwhile, an anisotropic hyperfine structure was observed in all the catalysts. A resonance parameter g// of 2.15 indicates that Cu2+ replaced the cation sites of ZnO. Moreover, a broad peak at around g⊥ = 2.05 was detected for each sample, which can be ascribed to adsorbed oxygen radicals associated with oxygen vacancies, according to previous studies (Li et al., 2018b). Thus, the EPR results confirm that Cu2+ was successfully doped into the ZnO lattice. Notably, the signal assigned to the anisotropic hyperfine structure of Cu2+ decreased mildly with increasing the heating time due to the increase of the long-range dipolar interaction between Cu2+ ions as the Cu doping amount increased.
FIGURE 5. Electron paramagnetic resonance profiles of the as-synthesized CuxO/ZnO catalysts and pristine ZnO support.
To investigate the electronic states of the different catalysts, XPS measurements were performed. Figure 6A shows the Cu 2p spectra of the catalysts, in which some differences in the peak shape of Cu 2p2/3 can be observed. Thus, the Cu 2p2/3 peak for CuxO/ZnO-12, CuxO/ZnO-24, and CuxO/ZnO-144 was clearly split into two peaks: a peak at higher binding energy (933.4 eV) that can be assigned to Cu2+ and another peak at ca. 931.9 eV corresponding to Cu+. In contrast, only one peak at 933.4 eV was observed in the spectrum of CuxO/ZnO-344 (Svintsitskiy et al., 2013). Interestingly, the Cu+ peak shifted gradually toward the high binding energy region as the heat treatment time increased. For CuxO/ZnO-144, the Cu+ peak shifted to 932.6 eV. This is a strong indication of the change in the Cu electron state in the catalyst. The electronic state of Zn in the catalyst is also worthy of note.
FIGURE 6. X-ray photoelectron spectra of the catalysts: (A) Cu 2p spectra of the fresh catalyst; (B) Zn 2p spectra of the fresh catalyst; (C) Cu 2p spectra of the used catalyst; (D) Zn 2p spectra of the used catalyst.
As shown in Figures 6A,B peak at 1021.4 eV attributable to Zn2+ appeared in the spectra of CuxO/ZnO-12 (Zhu et al., 2018). This peak shifted gradually to higher binding energies with increasing the treatment time, indicating that the interaction between the two components changed and the electronic structure of the components was affected simultaneously.
Next, considering that the interaction between the active component and the support of a catalyst is sensitive to the atmosphere (Dong et al., 2018), the electronic property of the used catalysts was investigated by XPS to study the changes in the catalysts during the reaction. An interesting “reverse behavior” of the interaction was observed. As shown in Figure 6C, the surface Cu species of all the catalysts presented characteristic of Cu2+ after reaction, in line with the previous studies (Zhang et al., 2019). For the CuxO/ZnO-12 catalyst, the peak corresponding to Cu2+ shifted by 0.9 eV to higher binding energy (934.3 eV) compared with that of fresh CuxO/ZnO-12. For CuxO/ZnO-24, the peak shifted by 0.6 eV, for CuxO/ZnO-144 by 0.1 eV, and for CuxO/ZnO-144 by 0 eV. The Zn 2p spectra of the catalysts were also found to change dramatically after the reaction (Figure 6D). Thus, for CuxO/ZnO-12, the binding energy of the Zn 2p2/3 peak shifted from 1021.4 to 1022.8 eV. For CuxO/ZnO-24, this shift was 0.7 eV. Meanwhile, the Zn 2p2/3 peak shifted to lower binding energy after the reaction for CuxO/ZnO-144 and CuxO/ZnO-324. Taking the Zn 2p2/3 peak of pristine ZnO as a reference, the shift value to higher binding energy decreased with increasing the treatment time. In other words, the interaction between CuxO and ZnO in CuxO/ZnO-12 was the strongest among the catalysts after the reaction, whereas that of CuxO/ZnO-324 was the weakest, opposite to the trend for the fresh catalysts.
The used catalysts were further characterized by TPR to confirm the change in the interaction for all the catalysts. As shown in Figure 7, the main peak position of used CuxO/ZnO-12 shifted to lower temperature from 284 to 265°C. The reducibility of the catalysts decreased with the treatment time, following an opposite trend to that of the fresh catalysts. Considering that the strong interaction between CuxO and ZnO facilitated the reduction of CuxO, it can be concluded that the interaction between CuxO and ZnO in CuxO/ZnO-12 was the strongest in the series of catalysts after the reaction, which is in line with the XPS results. This suggests a dramatic reconstruction of the catalysts during the reaction. However, the initial strong interactions between CuxO and ZnO impeded the reconstruction process. Therefore, the CuxO/ZnO-12 sample, which had a weaker interaction at the beginning, underwent to a greater extent the reconstruction phenomenon compared with the other catalysts.
The electron transfer between the two components was predicted according to the work functions. The work functions calculated by the energy difference between the vacuum and Fermi levels are 5.51, 7.65, 4.57 eV for ZnO(100), CuO(100) and Cu2O(111) surfaces respectively, which indicates the shuttling charge transfer between ZnO and CuO/Cu2O. In other words, the electrons in Cu+ will be transferred to ZnO, which explains the shifting of the Cu+ peak in the XPS data toward higher binding energy (Figure 6A). Meanwhile, CuO accepts electrons from ZnO. For the used catalyst, since ZnO is a typical p-typ semiconductor, part of the electrons in the ZnO conduction band are trapped by the adsorbed surface oxygen to form oxygen species, and part of the electrons diffuse into the interface (Yin et al., 2016), which is consistent with the XPS results. After the reaction, the binding energy of the Zn 2p2/3 peak of CuxO/ZnO-12 shifted by 1.0 eV compared with that of pristine ZnO, indicating that the strong interaction causes ZnO to release a considerable amount of electrons. The shift value of the Cu 2p2/3 and Zn 2p2/3 peaks was negatively correlated with the T50 temperature (Figure 8). Electron transfer between metal and oxide support is a key step in CO oxidation reaction. In previous studies, White et al. (White et al., 2006) revealed that surface Cu2+ was an unlikely candidate for oxidation or O2 dissociation. The active catalyst state was confirmed to be Cu+ (in a Cu2O lattice) which is either present from the onset or formed by reduction of Cu2+ by adsorbed CO. Jernigan et al. (Jernigan and Somorjai, 1994) found that reaction rates for CO oxidation decreased with increasing copper oxidation state (Cu > Cu2O > CuO). Huang et al. (Huang and Tsai, 2003) also found that the activity of CuO will be significantly enhanced when non-stoichiometric copper oxides are formed. Thus, it is documented that electrons transfer could contribute to the formation of active Cu species with higher activity, revealing that the strong interaction between CuxO and ZnO is favorable for CO oxidation.
FIGURE 8. (A) Dependence of the T50 temperature and the difference between the Cu 2p2/3 peak position of the used catalyst and the peak position for Cu2+ (933.4 eV); (B) dependence of the T50 temperature and the difference between the Zn 2p2/3 peak position of the used catalyst and the peak position for Zn2+ (1021.3 eV).
It has been demonstrated that the electron-rich interface would promote the adsorption of oxygen on catalyst (Hayyan et al., 2016; Zeng et al., 2017), which was favorable for CO oxidation reaction. Figure 9 presented the O 1s spectra of activated CuxO/ZnO catalysts. It can be seen that three characteristic peaks could be fitted from the original curve. The peak at around 530.1 eV is assigned to lattice oxygen (OL) of catalysts, peak around 531.5 eV is attributed to the adsorbed oxygen (Oad) species including O2− and O2− on the surface, the weaker peak at 532.4 eV was the oxygen in hydroxyl groups on the surface. The relative Oad concentration [Oad/(OL + Oad)] was related to the oxygen storage capacity of catalysts. It can be seen that the activated CuxO/ZnO-12 possess a highest Oad concentration of 61.2% (Supplementary Table S2) and the Oad concentration of CuxO/ZnO catalysts decreased with the treatment time. Thus, the electron-rich interface was favorable for the adsorption of oxygen and CO oxidation reaction.
FIGURE 9. O 1s spectra of the activated CuxO/ZnO. Activated condition: 300 °C under 10% O2/Ar for 1 h.
To confirmed further the electronic metal-support interaction between two components. In situ DRIFTs experiment was conducted to revealed CO adsorbates behavior on surface of catalysts. Before data collection, the catalyst was pretreated with a 10% O2/Ar flow rate of 100 ml min−1 at 300 °C for 1 h to remove any absorbed residues and then cooled to 30°C. Supplementary Figure S8 present the spectrum evolution of CuxO/ZnO-12 catalyst at different stage. The peaks appear at 2120 and 2177 cm−1 after CO inlet are assigned to the vibrations of gaseous CO. After purging with Ar for 15 min, most gaseous CO in the cell was blew out, a surviving peak at ∼2113 cm−1 was assigned to the vibrations of linearly CO species adsorbed on Cu+ (Sarkodie et al., 2021). All the catalysts present a distinct CO absorption peak at ∼2113 cm−1, as shown in Figure 10A. Moreover, Figure 10B present the detailed absorption peak position of the catalysts, revealing that the absorption peak shift slightly to lower wavenumbers. As established in literature, strong M-C bonds are favored by electron-rich metal centers due to π back-bonding, high d-electron density leads to the formation of strong bond between Cu+ and CO via π-back bonding contributions (Li et al., 2017; Sarkodie et al., 2021). The ZnO support acts as an electronic donor to Cu species would increase the π* back-donation from Cu+ to adsorbed CO and hence decrease the carbonyl stretching frequency.
FIGURE 10. (A): In-situ DRIFTS spectra of different CuxO/ZnO catalyst. (B): Spectroscopic data between 2060 and 2160 cm−1.
Furthermore, in situ CO-DRIFTs date and relative Oad concentration data were correlated with activity data of catalyst. As shown in Figure 11A, the T50 temperature of catalyst decreased with position of CO adsorption peak, indicating that the activity of CuxO/ZnO catalyst decreases with the decreasing interfacial electron density. From Figure 11B, one can see that the catalytic activity increases with the relative Oad concentration of CuxO/ZnO catalyst.
FIGURE 11. (A) Dependence of the T50 temperature and the adsorption position of CO molecular; (B) dependence of the T50 temperature and relative Oad concentration.
On the basis of the experimental and theoretical results, the effect of the interaction between CuxO and ZnO on the oxidation performance of CO can be described as depicted in Figure 12. The active sites of the catalysts can be divided into two types: exposed CuxO particles (Figure 12A) and the heterojunction at the interface (Figure 12B). The particle size of the catalysts before and after the reaction was almost unchanged, and the difference in the contribution of exposed CuxO particles on the activity among all the catalysts was negligible. Thus, the electronic structure at the catalyst interface can be considered the main factor affecting the catalytic activity. The strong interaction between the two components promotes the enrichment of electrons at the interface and the activation and dissociation of oxygen molecules. Nevertheless, the catalyst reconstruction during the reaction is an important factor in the formation of a strong catalyst interaction.
FIGURE 12. Schematic of the interaction between CuxO and ZnO during the CO oxidation reaction. (A): Side view; (B) TOP view.
In this study, a well-defined CuxO/ZnO catalyst was synthesized via a typical thermal decomposition method. By adjusting the drying time of the catalyst under vacuum, the CuxO-ZnO interaction was regulated successfully. The interaction between CuxO and ZnO was altered by the reconstruction of the catalyst during the CO oxidation reaction. For catalysts with a weak interaction, the reconstruction process enhanced the interaction between the two components, which was conducive to the electron transfer and the overall activity. In contrast, for the catalysts with a strong interaction, the reconstruction process is blocked. After a restructuring procedure, the strong interaction between CuxO and ZnO is favorable for the CO oxidation reaction.
The raw data supporting the conclusions of this article will be made available by the authors, without undue reservation.
SL, YZ, and XL: validation, investigation, data curation, visualization, writing—original draft. ZL: DFT calculation. Z T: data curation. CL: writing—editing. JL: methodology, resources, supervision. LW: conceptualization, methodology, resources, supervision, writing—review and editing.
This work was supported by the National Natural Science Foundation of China (No. 22072184 and 22102220), the Young Top-notch Talent Cultivation Program of Hubei Province and Hubei Key Laboratory of Processing and Application of Catalytic materials (202023604).
The authors declare that the research was conducted in the absence of any commercial or financial relationships that could be construed as a potential conflict of interest.
All claims expressed in this article are solely those of the authors and do not necessarily represent those of their affiliated organizations, or those of the publisher, the editors and the reviewers. Any product that may be evaluated in this article, or claim that may be made by its manufacturer, is not guaranteed or endorsed by the publisher.
The Supplementary Material for this article can be found online at: https://www.frontiersin.org/articles/10.3389/fchem.2022.912550/full#supplementary-material
Behrens, M., Studt, F., Kasatkin, I., Kühl, S., Hävecker, M., Abild-Pedersen, F., et al. (2012). The Active Site of Methanol Synthesis over Cu/ZnO/Al 2 O 3 Industrial Catalysts. Science 336, 893–897. doi:10.1126/science.1219831
Bikaljevic, D., Rameshan, R., Köpfle, N., Götsch, T., Mühlegger, E., Schlögl, R., et al. (2019). Structural and Kinetic Aspects of CO Oxidation on ZnOx-Modified Cu Surfaces. Appl. Catal. A General 572, 151–157. doi:10.1016/j.apcata.2018.12.032
Bratlie, K. M., Lee, H., Komvopoulos, K., Yang, P., and Somorjai, G. A. (2007). Platinum Nanoparticle Shape Effects on Benzene Hydrogenation Selectivity. Nano Lett. 7, 3097–3101. doi:10.1021/nl0716000
Chaika, M. A., Mancardi, G., and Vovk, O. M. (2020). Influence of CaO and SiO2 Additives on the Sintering Behavior of Cr,Ca:YAG Ceramics Prepared by Solid-State Reaction Sintering. Ceram. Int. 46, 22781–22786. doi:10.1016/j.ceramint.2020.06.045
Doherty, F., Wang, H., Yang, M., and Goldsmith, B. R. (2020). Nanocluster and Single-Atom Catalysts for Thermocatalytic Conversion of CO and CO2. Catal. Sci. Technol. 10, 5772–5791. doi:10.1039/D0CY01316A
Dong, J., Fu, Q., Jiang, Z., Mei, B., and Bao, X. (2018). Carbide-Supported Au Catalysts for Water-Gas Shift Reactions: A New Territory for the Strong Metal-Support Interaction Effect. J. Am. Chem. Soc. 140, 13808–13816. doi:10.1021/jacs.8b08246
Dong, J., Fu, Q., Li, H., Xiao, J., Yang, B., Zhang, B., et al. (2020). Reaction-induced Strong Metal-Support Interactions between Metals and Inert Boron Nitride Nanosheets. J. Am. Chem. Soc. 142, 17167–17174. doi:10.1021/jacs.0c08139
Fujitani, T., and Nakamura, J. (2000). The Chemical Modification Seen in the Cu/ZnO Methanol Synthesis Catalysts. Appl. Catal. A General 191, 111–129. doi:10.1016/S0926-860X(99)00313-0
Grunwaldt, J.-D., Molenbroek, A. M., Topsøe, N.-Y., Topsøe, H., and Clausen, B. S. (2000). In Situ investigations of Structural Changes in Cu/ZnO Catalysts. J. Catal. 194, 452–460. doi:10.1006/jcat.2000.2930
Hayyan, M., Hashim, M. A., and AlNashef, I. M. (2016). Superoxide Ion: Generation and Chemical Implications. Chem. Rev. 116, 3029–3085. doi:10.1021/acs.chemrev.5b00407
Huang, T.-J., and Tsai, D. H. (2003). CO Oxidation Behavior of Copper and Copper Oxides. Catal. Lett. 87, 173–178. doi:10.1023/A:1023495223738
Imtiaz, Q., Abdala, P. M., Kierzkowska, A. M., Van Beek, W., Schweiger, S., Rupp, J. L. M., et al. (2016). Na+ Doping Induced Changes in the Reduction and Charge Transport Characteristics of Al2O3-Stabilized, CuO-Based Materials for CO2 Capture. Phys. Chem. Chem. Phys. 18, 12278–12288. doi:10.1039/C6CP00257A
Jernigan, G. G., and Somorjai, G. A. (1994). Carbon Monoxide Oxidation over Three Different Oxidation States of Copper: Metallic Copper, Copper (I) Oxide, and Copper (II) Oxide - A Surface Science and Kinetic Study. J. Catal. 147, 567–577. doi:10.1006/jcat.1994.1173
Jia, J., Qian, C., Dong, Y., Li, Y. F., Wang, H., Ghoussoub, M., et al. (2017). Heterogeneous Catalytic Hydrogenation of CO2by Metal Oxides: Defect Engineering - Perfecting Imperfection. Chem. Soc. Rev. 46, 4631–4644. doi:10.1039/C7CS00026J
Jiang, X., Nie, X., Guo, X., Song, C., and Chen, J. G. (2020). Recent Advances in Carbon Dioxide Hydrogenation to Methanol via Heterogeneous Catalysis. Chem. Rev. 120, 7984–8034. doi:10.1021/acs.chemrev.9b00723
Jing, P., Gong, X., Liu, B., and Zhang, J. (2020). Recent Advances in Synergistic Effect Promoted Catalysts for Preferential Oxidation of Carbon Monoxide. Catal. Sci. Technol. 10, 919–934. doi:10.1039/C9CY02073J
Jun, K.-W., Shen, W.-J., Rama Rao, K. S., and Lee, K.-W. (1998). Residual Sodium Effect on the Catalytic Activity of Cu/ZnO/Al2O3 in Methanol Synthesis from CO2 Hydrogenation. Appl. Catal. A General 174, 231–238. doi:10.1016/S0926-860X(98)00195-1
Kattel, S., Liu, P., and Chen, J. G. (2017a). Tuning Selectivity of CO2 Hydrogenation Reactions at the Metal/Oxide Interface. J. Am. Chem. Soc. 139, 9739–9754. doi:10.1021/jacs.7b05362
Kattel, S., Ramírez, P. J., Chen, J. G., Rodriguez, J. A., and Liu, P. (2017b). Active Sites for CO 2 Hydrogenation to Methanol on Cu/ZnO Catalysts. Science 355, 1296–1299. doi:10.1126/science.aal3573
Kniep, B., Girgsdies, F., and Ressler, T. (2005). Effect of Precipitate Aging on the Microstructural Characteristics of Cu/ZnO Catalysts for Methanol Steam Reforming. J. Catal. 236, 34–44. doi:10.1016/j.jcat.2005.09.001
Kniep, B. L., Ressler, T., Rabis, A., Girgsdies, F., Baenitz, M., Steglich, F., et al. (2004). Rational Design of Nanostructured Copper-Zinc Oxide Catalysts for the Steam Reforming of Methanol. Angew. Chem. Int. Ed. 43, 112–115. doi:10.1002/anie.200352148
Kuld, S., Thorhauge, M., Falsig, H., Elkjaer, C. F., Helveg, S., Chorkendorff, I., et al. (2016). Quantifying the Promotion of Cu Catalysts by ZnO for Methanol Synthesis. Science 352, 969–974. doi:10.1126/science.aaf0718
Li, W., Hu, Y., Jiang, H., Jiang, N., Bi, W., and Li, C. (2018b). Litchi-peel-like Hierarchical Hollow Copper-Ceria Microspheres: Aerosol-Assisted Synthesis and High Activity and Stability for Catalytic CO Oxidation. Nanoscale 10, 22775–22786. doi:10.1039/C8NR04642E
Li, W., Wang, H., Jiang, X., Zhu, J., Liu, Z., Guo, X., et al. (2018a). A Short Review of Recent Advances in CO2 Hydrogenation to Hydrocarbons over Heterogeneous Catalysts. RSC Adv. 8, 7651–7669. doi:10.1039/C7RA13546G
Li, Z., Zhong, L., Yu, F., An, Y., Dai, Y., Yang, Y., et al. (2017). Effects of Sodium on the Catalytic Performance of CoMn Catalysts for Fischer-Tropsch to Olefin Reactions. ACS Catal. 7, 3622–3631. doi:10.1021/acscatal.6b03478
Liao, F., Huang, Y., Ge, J., Zheng, W., Tedsree, K., Collier, P., et al. (2011). Morphology-Dependent Interactions of ZnO with Cu Nanoparticles at the Materials' Interface in Selective Hydrogenation of CO2 to CH3OH. Angew. Chem. Int. Ed. 50, 2162–2165. doi:10.1002/ange.20100710810.1002/anie.201007108
Liu, C., Zhang, Y., Zhao, Y., Wei, L., Hong, J., Wang, L., et al. (2017). The Effect of the Nanofibrous Al2O3aspect Ratio on Fischer-Tropsch Synthesis over Cobalt Catalysts. Nanoscale 9, 570–581. doi:10.1039/C6NR07529K
Liu, J., Qiao, B., Song, Y., Huang, Y., and Liu, J. (2015). Hetero-epitaxially Anchoring Au Nanoparticles onto ZnO Nanowires for CO Oxidation. Chem. Commun. 51, 15332–15335. doi:10.1039/C5CC03353E
Liu, M.-H., Chen, Y.-W., Lin, T.-S., and Mou, C.-Y. (2018). Defective Mesocrystal ZnO-Supported Gold Catalysts: Facilitating CO Oxidation via Vacancy Defects in ZnO. ACS Catal. 8, 6862–6869. doi:10.1021/acscatal.8b0491310.1021/acscatal.8b01282
Liu, X., Liu, M.-H., Luo, Y.-C., Mou, C.-Y., Lin, S. D., Cheng, H., et al. (20122012). Strong Metal-Support Interactions between Gold Nanoparticles and ZnO Nanorods in CO Oxidation. J. Am. Chem. Soc. 134 (24), 10251–10258. doi:10.1021/ja3033235
Lou, Y., Cai, Y., Hu, W., Wang, L., Dai, Q., Zhan, W., et al. (2020a). Identification of Active Area as Active Center for CO Oxidation over Single Au Atom Catalyst. ACS Catal. 10, 6094–6101. doi:10.1021/acscatal.0c01303
Lou, Y., Xu, J., Zhang, Y., Pan, C., Dong, Y., and Zhu, Y. (2020b). Metal-support Interaction for Heterogeneous Catalysis: from Nanoparticles to Single Atoms. Mater. Today Nano 12, 100093. doi:10.1016/j.mtnano.2020.100093
Luo, L., Nian, Y., Wang, S., Dong, Z., He, Y., Han, Y., et al. (2020). Real‐Time Atomic‐Scale Visualization of Reversible Copper Surface Activation during the CO Oxidation Reaction. Angew. Chem. Int. Ed. 59, 2505–2509. doi:10.1002/ange.20191502410.1002/anie.201915024
Lyu, S., Peng, B., Kuang, T., Rappé, K. G., Zhang, Y., Li, J., et al. (2019). Supported Cobalt Nanoparticles with a Single Active Phase for Fischer-Tropsch Synthesis. ACS Appl. Nano Mat. 2, 2266–2272. doi:10.1021/acsanm.9b00187
Milligen, B. P. v., Bons, P. D., Carreras, B. A., and Sánchez, R. (2005). On the Applicability of Fick's Law to Diffusion in Inhomogeneous Systems. Eur. J. Phys. 26, 913–925. doi:10.1088/0143-0807/26/5/023
Nakamura, J., Uchijima, T., Kanai, Y., and Fujitani, T. (1996). The Role of ZnO in Cu/ZnO Methanol Synthesis Catalysts. Catal. Today 28, 223–230. doi:10.1016/0920-5861(95)00240-5
Park, J., An, K., Hwang, Y., Park, J.-G., Noh, H.-J., Kim, J.-Y., et al. (2004). Ultra-large-scale Syntheses of Monodisperse Nanocrystals. Nat. Mater 3 (12), 891–895. doi:10.1038/nmat1251
Sarkodie, B., Hu, Y., Bi, W., Jiang, J., and Li, C. (2021). Promotional Effects of Cu O on the Activity of Cu/ZnO Catalyst toward Efficient CO Oxidation. Appl. Surf. Sci. 548, 149241. doi:10.1016/j.apsusc.2021.149241
Song, T., Dong, J., Li, R., Xu, X., Hiroaki, M., Yang, B., et al. (2021). Oxidative Strong Metal-Support Interactions between Metals and Inert Boron Nitride. J. Phys. Chem. Lett. 12, 4187–4194. doi:10.1021/acs.jpclett.1c00934
Svintsitskiy, D. A., Kardash, T. Y., Stonkus, O. A., Slavinskaya, E. M., Stadnichenko, A. I., Koscheev, S. V., et al. (2013). In Situ XRD, XPS, TEM, and TPR Study of Highly Active in CO Oxidation CuO Nanopowders. J. Phys. Chem. C 117, 14588–14599. doi:10.1021/jp403339r10.1021/jp403339r
Tang, H., Su, Y., Zhang, B., Lee, A. F., Isaacs, M. A., Wilson, K., et al. (2017). Classical Strong Metal-Support Interactions between Gold Nanoparticles and Titanium Dioxide. Sci. Adv. 3, e1700231. doi:10.1126/sciadv.1700231
van Deelen, T. W., Hernández Mejía, C., and de Jong, K. P. (2019). Control of Metal-Support Interactions in Heterogeneous Catalysts to Enhance Activity and Selectivity. Nat. Catal. 2, 955–970. doi:10.1038/s41929-019-0364-x
Wen, J., Huang, C., Sun, Y., Liang, L., Zhang, Y., Zhang, Y., et al. (2020). The Study of Reverse Water Gas Shift Reaction Activity over Different Interfaces: The Design of Cu-Plate ZnO Model Catalysts. Catalysts 10, 533. doi:10.3390/catal10050533
White, B., Yin, M., Hall, A., Le, D., Stolbov, S., Rahman, T., et al. (2006). Complete CO Oxidation over Cu2O Nanoparticles Supported on Silica Gel. Nano Lett. 6, 2095–2098. doi:10.1021/nl061457v
Whittle, D. M., Mirzaei, A. A., Hargreaves, J. S. J., Joyner, R. W., Kiely, C. J., Taylor, S. H., et al. (2002). Co-precipitated Copper Zinc Oxide Catalysts for Ambient Temperature Carbon Monoxide Oxidation: Effect of Precipitate Ageing on Catalyst Activity. Phys. Chem. Chem. Phys. 4, 5915–5920. doi:10.1039/B207691H
Xie, X., Li, Y., Liu, Z.-Q., Haruta, M., and Shen, W. (2009). Low-temperature Oxidation of CO Catalysed by Co3O4 Nanorods. Nature 458, 746–749. doi:10.1038/nature07877
Xiong, H., Zhang, Y., Wang, S., and Li, J. (2005). Fischer-Tropsch Synthesis: the Effect of Al2O3 Porosity on the Performance of Co/Al2O3 Catalyst. Catal. Commun. 6, 512–516. doi:10.1016/j.catcom.2005.04.018
Yang, F., Zhao, H., Wang, W., Wang, L., Zhang, L., Liu, T., et al. (2021). Atomic Origins of the Strong Metal-Support Interaction in Silica Supported Catalysts. Chem. Sci. 12, 12651–12660. doi:10.1039/D1SC03480D
Yang, X., Chen, H., Meng, Q., Zheng, H., Zhu, Y., and Li, Y. W. (2017). Insights into Influence of Nanoparticle Size and Metal-Support Interactions of Cu/ZnO Catalysts on Activity for Furfural Hydrogenation. Catal. Sci. Technol. 7, 5625–5634. doi:10.1039/C7CY01284E
Yang, Y., Luo, M., Zhang, W., Sun, Y., Chen, X., and Guo, S. (2018). Metal Surface and Interface Energy Electrocatalysis: Fundamentals, Performance Engineering, and Opportunities. Chem 4, 2054–2083. doi:10.1016/j.chempr.2018.05.019
Ye, R.-P., Lin, L., Li, Q., Zhou, Z., Wang, T., Russell, C. K., et al. (2018). Recent Progress in Improving the Stability of Copper-Based Catalysts for Hydrogenation of Carbon-Oxygen Bonds. Catal. Sci. Technol. 8, 3428–3449. doi:10.1039/C8CY00608C
Yin, M., Wang, F., Fan, H., Xu, L., and Liu, S. (2016). Heterojunction CuO@ZnO Microcubes for Superior P-type Gas Sensor Application. J. Alloys Compd. 672, 374–379. doi:10.1016/j.jallcom.2016.02.197
Zeng, Y., Wang, T., Zhang, S., Wang, Y., and Zhong, Q. (2017). Sol-gel Synthesis of CuO-TiO 2 Catalyst with High Dispersion CuO Species for Selective Catalytic Oxidation of NO. Appl. Surf. Sci. 411, 227–234. doi:10.1016/j.apsusc.2017.03.107
Zhang, Z.-c., Xu, B., and Wang, X. (2014). Engineering Nanointerfaces for Nanocatalysis. Chem. Soc. Rev. 43, 7870–7886. doi:10.1039/C3CS60389J
Zhang, Z., Chen, X., Kang, J., Yu, Z., Tian, J., Gong, Z., et al. (2021). The Active Sites of Cu-ZnO Catalysts for Water Gas Shift and CO Hydrogenation Reactions. Nat. Commun. 12, 4331–4339. doi:10.1038/s41467-021-24621-8
Zhang, Z., Wu, H., Yu, Z., Song, R., Qian, K., Chen, X., et al. (2019). Site‐Resolved Cu 2 O Catalysis in the Oxidation of CO. Angew. Chem. Int. Ed. 58, 4276–4280. doi:10.1002/anie.201814258
Zhou, K., and Li, Y. (2012). Catalysis Based on Nanocrystals with Well-Defined Facets. Angew. Chem. Int. Ed. 51, 602–613. doi:10.1002/anie.201102619
Keywords: metal-support interactions, Cu species, CO oxidation, ZnO, supported catalysts
Citation: Lyu S, Zhang Y, Li Z, Liu X, Tian Z, Liu C, Li J and Wang L (2022) Electronic Metal-Support Interactions Between CuxO and ZnO for CuxO/ZnO Catalysts With Enhanced CO Oxidation Activity. Front. Chem. 10:912550. doi: 10.3389/fchem.2022.912550
Received: 05 April 2022; Accepted: 25 April 2022;
Published: 13 May 2022.
Edited by:
Haifeng Xiong, Xiamen University, ChinaCopyright © 2022 Lyu, Zhang, Li, Liu, Tian, Liu, Li and Wang. This is an open-access article distributed under the terms of the Creative Commons Attribution License (CC BY). The use, distribution or reproduction in other forums is permitted, provided the original author(s) and the copyright owner(s) are credited and that the original publication in this journal is cited, in accordance with accepted academic practice. No use, distribution or reproduction is permitted which does not comply with these terms.
*Correspondence: Yuhua Zhang, cG9hbGVfemhhbmdAYWxpeXVuLmNvbQ==; Li Wang, bGkud2FuZ0BzY3VlYy5lZHUuY24=
†These authors have contributed equally to this work
Disclaimer: All claims expressed in this article are solely those of the authors and do not necessarily represent those of their affiliated organizations, or those of the publisher, the editors and the reviewers. Any product that may be evaluated in this article or claim that may be made by its manufacturer is not guaranteed or endorsed by the publisher.
Research integrity at Frontiers
Learn more about the work of our research integrity team to safeguard the quality of each article we publish.