- 1College of Engineering and Technology, Southwest University, Chongqing, China
- 2Department of Quantum and Energy Materials, International Iberian Nanotechnology Laboratory (INL), Braga, Portugal
- 3School of Materials and Energy, Southwest University, Chongqing, China
- 4College of Materials Science and Engineering, Chongqing University, Chongqing, China
The detection of dissolved gases in oil is an important method for the analysis of transformer fault diagnosis. In this article, the potential-doped structure of the Ag3 cluster on the HfSe2 monolayer and adsorption behavior of CO and C2H4 upon Ag3–HfSe2 were studied theoretically. Herein, the binding energy, adsorption energy, band structure, density of state (DOS), partial density of state (PDOS), Mulliken charge analysis, and frontier molecular orbital were investigated. The results showed that the adsorption effect on C2H4 is stronger than that on CO. The electrical sensitivity and anti-interference were studied based on the bandgap and adsorption energy of gases. In particular, there is an increase of 55.49% in the electrical sensitivity of C2H4 after the adsorption. Compared to the adsorption energy of different gases, it was found that only the adsorption of the C2H4 system is chemisorption, while that of the others is physisorption. It illustrates the great anti-interference in the detection of C2H4. Therefore, the study explored the potential of HfSe2-modified materials for sensing and detecting CO and C2H4 to estimate the working state of power transformers.
Introduction
Two-dimensional transition metal dichalcogenides (TMDs) have recently emerged as a focus of the scientific community in virtue of their versatile and tunable physical properties (Xu et al., 2013; Duerloo et al., 2014; Si et al., 2020; Ju et al., 2021). Layered transition metal disulfides (LTMDs) own the properties of large specific surface value, high electronic activity, and sensitivity (Choi and Kim, 2018). The properties mentioned previously contribute to the great potential of the chemical sensors. Hafnium dichalcogenides (HfX2, X = S, Se, or Te) belong to middle-gap semiconductors and have a high chemical reactivity and various energy dispersions (Yue et al., 2015; Mirabelli et al., 2016; Mleczko et al., 2017; Cruzado et al., 2021). As a consequence, all the excellent properties mentioned previously embody their future applications in optoelectronics and electronics.
As the most important and valuable piece of equipment, the operation status of the oil-immersed power transformers affects the safety and stability of the electric power system operation directly (Gui et al., 2020). The malfunction that occurred in oil or the insulating paper is inevitable during the long-running process of the transformer. The malfunction mainly includes overheating of oil or the insulating paper, arc discharge, partial discharge, and spark discharge (Tang et al., 2020). Under the effect of electricity and heat, the transformer oil will undergo a chemical reaction and generate gases. The gases generated are mostly hydrocarbons and some other related gases, such as CH4, C2H4, CO2, and CO. Their composition and contents are closely linked to the type and severity of the transformer faults (Singh and Bandyopadhyay, 2010). Dissolved gas analysis (DGA) could discover the hidden faults sensitively. DGA mainly includes infrared spectroscopy, gas chromatography, Raman spectroscopy, and the gas sensor method (Tran et al., 2018). Due to its simple structure, high reaction sensitivity, low cost, and low power consumption, the gas sensor method is proposed to be applied for detecting gases by more and more scientists (Wei et al., 2020).
HfSe2 has the atomic stacking structure with a layer of Hf atoms stuck in the middle of two layers of Se atoms. Cui et al. explored the adsorption behavior of Pd-doped and Pt-doped HfSe2 monolayers upon several kinds of gases, such as NO2, SO2, and SOF2 (Cui et al., 2020a; Cui et al., 2020c). It is found that the Pd-doped monolayer behaves better for NO2, while the Pt-doped HfSe2 monolayer behaves better for SO2. Wang et al. studied the adsorption nature and behavior of Rh-doped HfSe2, and it shows a stronger interaction between the SO2 molecule and the monolayer than SO2F2 (Wang and Liao, 2019). Wu et al. studied the doping behavior of Pd atoms and the sensing character of the Pd-doped HfSe2 (Pd–HfSe2) monolayer upon CO and C2H2 (Wu et al., 2022). They found that the Pd-HfSe2 monolayer possesses a better adsorption behavior on CO. Yang et al. investigated the adsorption of CO, C2H2, and C2H4 based on the Cu-doped Se-vacant MoSe2 (Cu–MoSe2) monolayer (Yang et al., 2020). But Cu–MoSe2 is not selective for the detection of C2H4. Xu et al. explored four characteristic dissolved gases in transformer oil: H2, CH4, C2H2, and C2H4 (Xu et al., 2020). It was found that the adsorption effects on C2H2 and C2H4 are stronger than those of the others. Speaking of the methods of modifying the material monolayer, Asif et al. integrated positively charged semiconductive sheets of Zn-NiAl LDH and negatively charged layers of rGO to modify the glassy carbon electrode (Asif et al., 2019a). The modified electrode exhibited excellent electrocatalytic activity. The doping formation of the Ag3 cluster and the adsorption behavior of C2H4 and CO were not explored yet. In this article, the adsorption of C2H4 and CO of Ag3-doped HfSe2 monolayers was investigated. The doping formation adopted is the Ag3 cluster, and three atoms contribute to the formation of a stable triangle structure. The results showed that the Ag3-doped HfSe2 monolayer behaves better for C2H4 adsorption but is inactive for CO.
Computational Details
The first-principle calculations based on the DFT (density functional theory) framework emerged as an important and dominant method in quantum mechanics simulation (Delley, 2000). All the calculations in this article adopted the DMol3 package in Materials Studio software to establish the adsorption model of pristine and Ag3-doped HfSe2 upon C2H4 and CO. Moreover, Perdew–Burke–Ernzerhof (PBE) functional with a generalized gradient approximation (GGA) was used for the electron exchange and correlation function (Chen et al., 2019b). To better deal with the van der Waals interactions and the relativistic effect of doped atoms, Tkatchenko and Scheffler’s (TS) method and the DFT semi-core pseudopotential (DSSP) method were employed, respectively. The atomic orbital basis set was described by the double numerical plus polarization (DNP) method (Cui et al., 2020b). The supercell geometry optimizations were calculated under the Monkhorst-Pack k-point mesh of 5 × 5 × 1, while 7 × 7 × 1 was sampled for the more accurate electronic structure calculations. To ensure the precision of total energy, smearing was set as 0.005Ha, and the energy tolerance accuracy, maximum force, and displacement were set as 10−5 Ha, 2 × 10−3 Ha/Å, and 5 × 10−3 Å, respectively (Topsakal et al., 2009; Sharma et al., 2018). To mimic a free-standing graphene sheet of HfSe2, a periodic 4 × 4 × 1 HfSe2 supercell was established with a 20-Å vacuum region imposed in the direction where the sheet is not periodic, and the vacuum region is large enough to ensure the doping and gas adsorption processes, as well as eliminate the interaction between adjacent units (Yang et al., 2020).
The binding energy
The aforementioned equation represents the total energy of the Ag3-HfSe2 monolayer subtracting the total energies of the free-standing sheet of the HfSe2 and Ag3 cluster. Comparing the binding energy of different doping sites, the configuration with the lowest binding energy is defined as the most stable one (Asif et al., 2022).
The adsorption energy
Here, in the aforementioned equation,
Results and Discussion
Analysis of Pristine and the Ag3-Doped HfSe2 Monolayer
First, the geometric structure of the free-standing HfSe2 monolayer was optimized to obtain the most stable configuration, as shown in Figures 1A,B. It holds the atomic stacking structure with a layer of Hf atoms stuck in the middle of two layers of Se atoms (Wang and Liao, 2019). The Hf–Se bond in the intrinsic HfSe2 monolayer is measured as 2.70 Å, which is consistent with the articles published previously. Speaking of the performance of Ag3 doping on the HfSe2 monolayer, three doped sites were taken into account. They are named Tse1 (a tripod site right upside the lower-layer Se atoms), Tse2 (right upside the upper-layer Se atoms), and THf (a tripod site right upside the mid-layer Hf atoms) (Ambrusi et al., 2017; Ju et al., 2017; Mi et al., 2021). As far as the most stable doped configuration, the lower the binding energy (Eb), the more stable is the structure. Eb is calculated as shown in Eq. 2-1, and the lowest one was chosen as the most stable doping system in this article. The Ag3 cluster favored to be doped through the Tse1 site with the numerical value of −2.512 eV, of which the Eb is lower than −1.347 and −1.155 eV for Tse2 and THf, respectively.
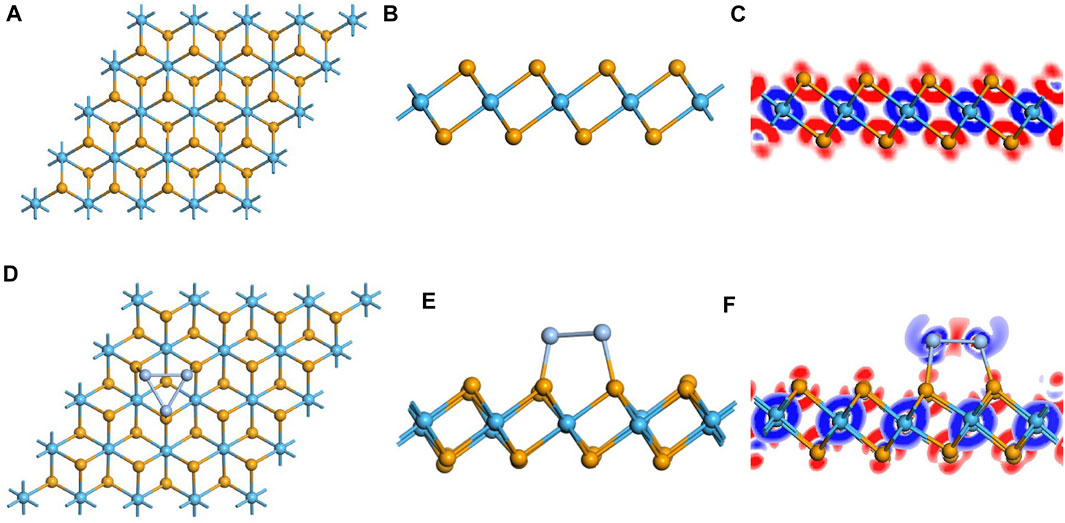
FIGURE 1. Optimized configuration and electronic structures of the pristine and doped monolayer. (A–C) pristine system and (D–F) doped system. In DCD, red and blue regions represent electron accumulation and deletion.
As is depicted in Figures 1D,E, there is a slight deformation after the doping of the Ag3 cluster in the HfSe2 monolayer, of which the Ag–Se bonds are 2.646, 2.628, and 2.648 Å, respectively. From the result of the Mulliken method (Chen et al., 2019a), it can be seen that the Ag3 cluster is positively charged by 0.267 e after doping. As is shown in Figure 1F, Ag atoms are surrounded by blue areas, in which the red and blue regions indicate electron accumulation and deletion independently (Cui et al., 2019). As a consequence, the analysis of the Mulliken method is in good accordance with the DCD, showing that the Ag3 cluster is an electron donator. Compared with the bandgap obtained as 0.539 eV of the pristine HfSe2 monolayer in Figure 2A, Ag3–HfSe2 is 0.553 eV with a decrease of 0.14 eV in Figure 2B. In comparison of the pristine and Ag3-doped HfSe2’s TDOS, it can be seen that there is a right shift, which is keeping with the result of the bandgap analysis.
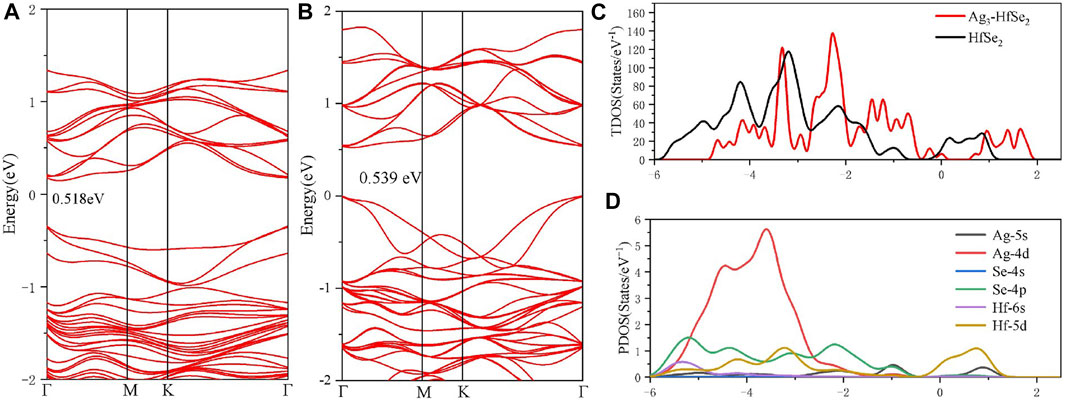
FIGURE 2. Band structure and the TDOS and PDOS of the pristine and doped monolayers. (A) Band structure of the pristine HfSe2 monolayer. (B–D) Band structure, TDOS, and PDOS of the Ag3–HfSe2 monolayer.
As illustrated in Figure 2D, it can be seen from the PDOS that there are several overlapping regions among the Ag-4d, Se-4p, and Hf-5d orbitals at −6 ∼ −1 eV, coincidently between the Hf-5d and Ag-5s orbitals at 0–1.5 eV. The PDOS verifies the conclusion that there are strong interactions existing among Ag, Se, and Hf atoms (Hu et al., 2019; Gao et al., 2020). As a consequence, due to the doping performance of the Ag3 cluster, it is among the Ag-4d, Hf-5d, and Se-4p orbitals that the main orbital hybridization occurs. In addition, compared with the TDOS and PDOS mentioned previously, it can be seen that it is on the Ag, Se, and Hf atoms that the bottom of the conduction band and the top of the valence band are localized, and it also verifies the charge transfers from the Ag3 cluster to the Se and Hf atoms (Yang et al., 2019).
Analysis of Gas Adsorption on the Pristine HfSe2 Monolayer
After investigating the doping performance of the Ag3 cluster, the adsorption behaviors of the pristine HfSe2 monolayer upon CO and C2H4 were investigated as follows. In terms of the adsorption site of different gases upon the pristine HfSe2 monolayer and Ag3–HfSe2 monolayer, all the possible adsorption sites were taken into account, but only the site with the lowest adsorption energy was adopted for further study (Zhang et al., 2009).
As for the adsorption of CO molecules on the pristine HfSe2 monolayer, three different adsorption directions were simulated. The molecule accessed the Se atoms via C and O atoms vertically and parallelly to the monolayer, respectively (Ma et al., 2016). As shown in Table 1, C atoms tend to be adsorbed by Se atoms in the upper layer of the pristine HfSe2 monolayer, with a distance of 4.055 Å between C and Se atoms. The length is significantly longer than the sum of the C and Se atoms’ covalent radii, verifying the inexistence of the C–Se bond (Zhou et al., 2018b). For the adsorption of CO on the pristine HfSe2 monolayer, the adsorption energy is 0.159 eV, which is less than 0.8 eV and to be defined as physisorption. The amount of charge transfer QT between the monolayer and CO molecule is very weak, with a value of 0.004 e. It includes 0.019 e of the C atom and −0.0105 e of the O atom (Late et al., 2014). Similarly, the DCD shown in Figure 3C reveals that the C atom and O atom are surrounded by blue and red areas, which is consistent with the Mulliken analysis. Furthermore, the bandgap, calculated as 0.691 eV, has an increase of 0.067 eV. As a consequence, based on the analysis mentioned previously, the adsorption effect of CO on the pristine monolayer is poor.
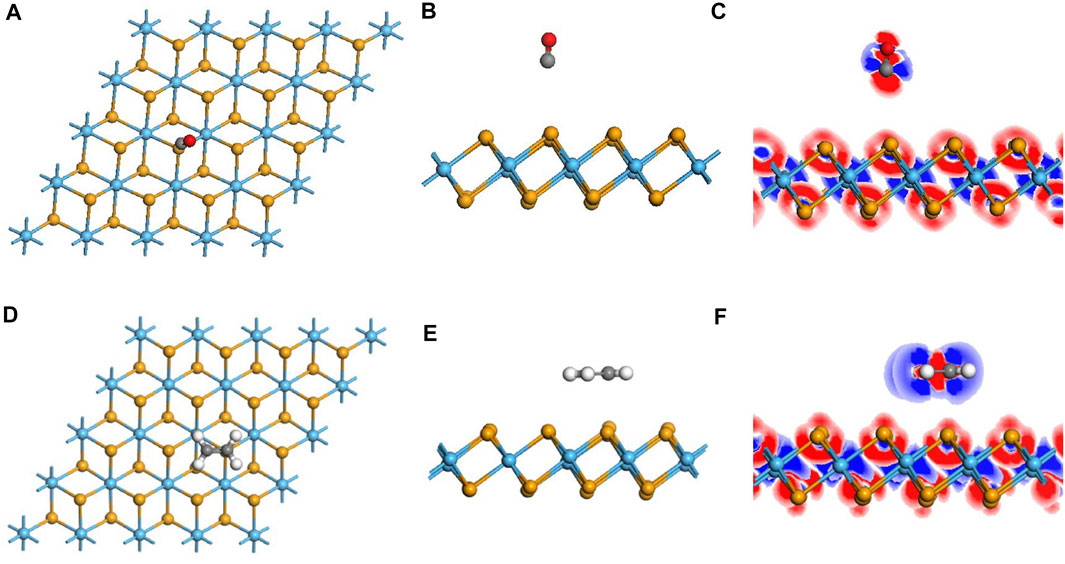
FIGURE 3. Geometric and electronic structures of the adsorption system of CO and C2H4 on the intrinsic monolayer. (A–C) Adsorption system of CO and related DCD. (D–F) Adsorption system of C2H4 and related DCD.
For the adsorption sites on the pristine monolayer of C2H4, two possible sites were investigated to simulate, with C2H4 being vertical and parallel to the upper layer (Qian et al., 2020). According to the calculated adsorption energy, it is the site that in a parallel direction holds the lowest energy. The C2H4 molecule is arrested by the Se atom, with a distance of 3.39 Å between C and Se atoms, which is longer than the sum of their covalent radii (Gao et al., 2019). The charge transfer QT generated by the CO molecule is −0.015 e, including −0.378 e of C atoms and 0.363 e of H atoms, which is consistent with the DCD shown in Figure 3F. Compared with the monolayer before the adsorption, there is an increase of 0.067 eV for the bandgap. In terms of the adsorption energy, it is 0.3437 eV that reveals the property of physisorption, which is below the standard of 0.8 eV (Zhou et al., 2018a). By reason of the foregoing analysis, the adsorption capacity of the pristine monolayer for C2H4 is not very well either.
Adsorption of the CO Molecule on the Ag3-Doped HfSe2 Monolayer
With regard to the adsorption of the doped monolayer, the adsorption of CO upon the Ag3-doped monolayer was investigated first. The most stable adsorption configuration is as same as the pristine one. As is depicted in Figure 4A, there is a slight deformation in the configuration after doping, manifesting the reduction of the distance between the C atom and dopant, elongated from that of 4.055 Å in the pristine system to 3.65 Å. But the distance measured as 3.65 Å is still similarly longer than the sum of the covalent radii, representing that there is no chemical effect (Cortés-Arriagada et al., 2018). Although Ead is raised to 0.388 eV, its property is still defined as physisorption.
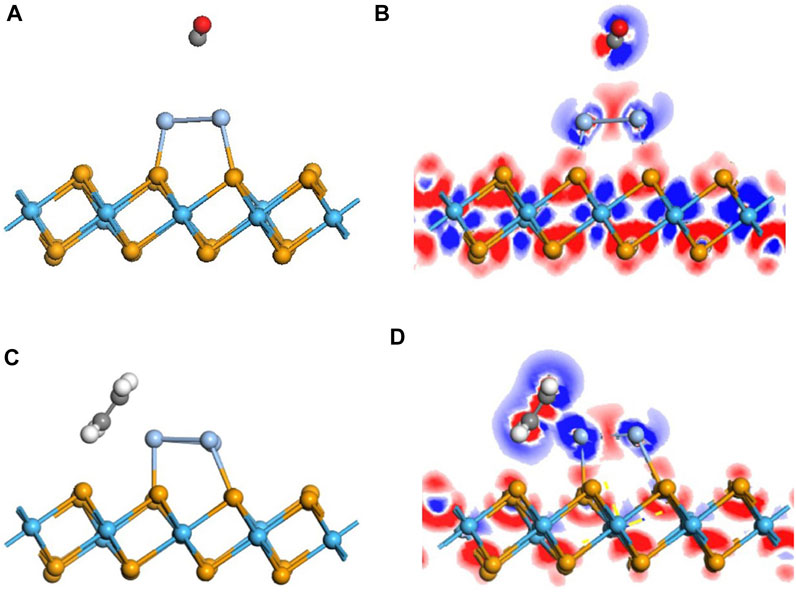
FIGURE 4. Geometric and electronic structures of the adsorption system of CO and C2H4 on the doped Ag3–HfSe2 monolayer. (A,B) Adsorption system of CO and related DCD. (C,D) Adsorption system of C2H4 and related DCD.
Figure 5B exhibits the total TDOS distribution of the adsorption system of CO, where a right shift is observed for the TDOS state of the CO adsorbed system after adsorption. By analyzing the band structure of the system after the adsorption of CO, it was found that the bandgap is 0.554 eV, which is shown in Figure 5A. Furthermore, as is shown in Table 2, there is 0.014 e transferred from the doped system to the CO molecule based on the analysis of Mulliken atomic charges (Allian et al., 2011). Combined with the Mulliken analysis, the demonstration of the DCD shown in Figure 5B is logical, in which the Ag atoms are surrounded by blue areas. In other words, the dopant Ag3 cluster plays an important role as an electron donator in the system. According to the PDOS in Figure 5C, it can be found that the Ag-4d orbital is highly hybrid with the C-2s and C-2p orbitals at −5 ∼ −4 eV, whereas the Ag-5s orbital is hybrid with the C-2p orbital at −1 ∼ −0.5 eV (Khan Musa et al., 2020). Also, after the adsorption of the CO molecule, the bandgap of the adsorbed system is raised to 0.554 eV, which has an increase of 0.015 eV.
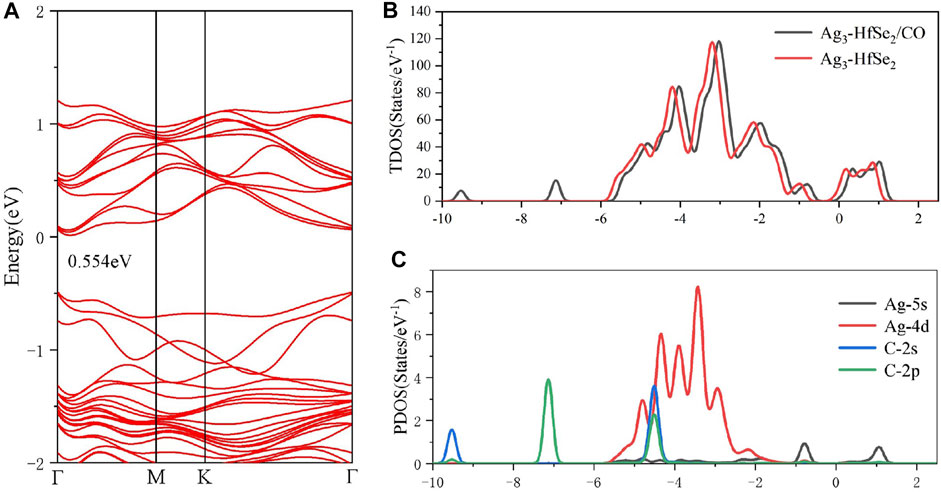
FIGURE 5. (A) Band structure, (B) TDOS and (C) PDOS of CO molecules’ adsorption on the Ag3-doped HfSe2 monolayer.
As a consequence, compared with the pristine system, there is a rise in the absolute value of the change in the bandgap. The change of the electronic parameters of the monolayer after the adsorption of CO molecules could be observed. Compared to the adsorption energy, adsorption distance, and charge transfer with those of pristine mentioned before, it can be seen that the adsorption performance of CO has been improved to a certain extent but is not ideal (Zhang et al., 2017). To draw a conclusion, it is not suitable for Ag3-doped HfSe2 either as a sensor material or as an adsorbent.
Adsorption of the C2H4 Molecule on the Ag3-Doped HfSe2 Monolayer
To better investigate the property of the adsorption of the C2H4 molecule upon the doped monolayer, three possible adsorption sites and directions were considered. They are as follows, approaching the Ag3 cluster in a parallel way and in a vertical way but in two different directions, respectively. Compared with the results of the adsorption energy for different sites, it is the parallel way that holds the lowest value, which is calculated as −0.911 eV. After adsorption, it can be seen that the gas molecule is arrested by the dopant Ag3 cluster. The detailed adsorption performance may be depicted, as in Figure 4C, in which the distance between the C atom and Ag atom is measured as 2.400 Å. It confirms that the interaction exists between the C and Ag atoms, and the structure has an obvious change. From the DCD in Figure 4D, it can be observed that the C atoms are surrounded by red areas, and the H atoms are surrounded by blue areas. It means that the H and Ag atoms act as electron donators (Wang et al., 2020). As is shown in Figure 6A, the band structure reveals that the bandgap of the adsorption system is 0.674 eV, which has an increase of 0.156 eV. Compared with the band structure before the adsorption, it can be found that it becomes denser and adds more impurity tracks. It widens the impurity band of the adsorption system, makes the transfer of electrons more conducive, and presents a better adsorption effect (Wu et al., 2017). The result obtained from the TDOS in Figure 6B demonstrates that there is a slight right shift, which is coincident with the analysis of the bandgap mentioned earlier. As demonstrated in Figure 6C, the PDOS reveals that there is a hybridization between the C-2p orbital and Ag-5s and Ag-4d orbitals at −6 ∼ −2 eV. On the basis of the analysis of Mulliken atomic charges, there is 0.221 e transferred from the C2H4 molecule to the doped system.
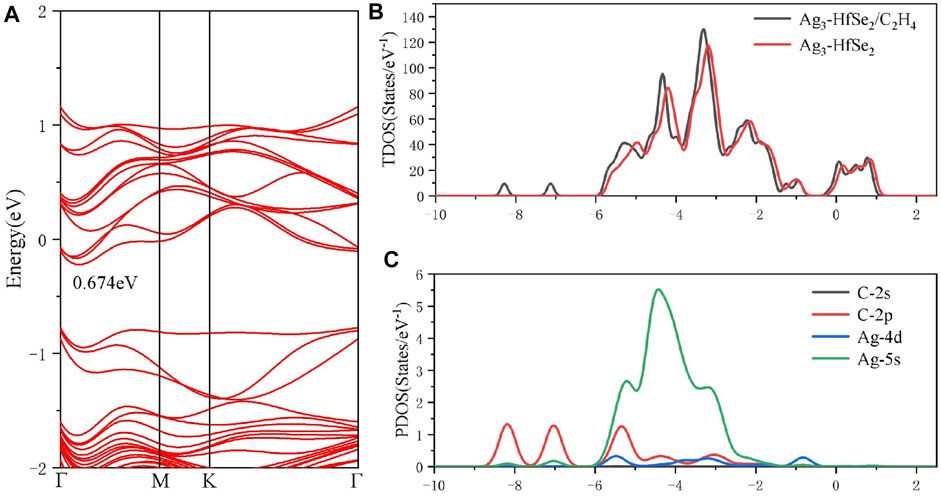
FIGURE 6. (A) Band structure, (B) TDOS and (C) PDOS of C2H4 molecules’ adsorption on the Ag3-doped HfSe2 monolayer.
Moreover, according to the adsorption of the two gases, it can be concluded that the doped Ag3–HfSe2 monolayer is more selective for C2H4 gas molecules. Compared to the electronic properties of the monolayer after the adsorption of CO and C2H4, it can be seen that the latter holds lower adsorption energy, a wider bandgap, and a higher charge transfer capacity. All the properties mentioned previously contribute to its better performance of adsorption. As a consequence, from the aspect of electron property, it is the C2H4 system that holds the stronger interaction compared with the CO system. This also indicates its strong potential for applying gas sensing for detecting C2H4 gas molecules (Chen et al., 2020).
Analysis of the Frontier Molecular Orbital Theory
On the basis of the electronic mechanism, the dopant changes the electrostatic potential on the HfSe2 monolayer because of its different electron affinities. It contributes to a change in the height of the surface potential barrier or a corresponding change in the resistance value of the semiconductor. Gas molecules are trapped on the monolayer by the Ag3 cluster, and the dopant could be defined as a catalyst during the adsorption process (Asif et al., 2019b). It means the dopant can enhance the adsorption nature of gas molecules and accelerate the sensing electron exchange. Based on the frontier molecular orbital theory, the reasons that affect the conductivity change can be reacted directly (Liao et al., 2021). As is known, the resistive gas sensor demonstrates the effect of adsorption on different gas molecules by detecting the resistance change of the material (Asif et al., 2018). As is depicted in Figure 7, it shows the highest occupied orbital (HOMO) and the lowest free orbital (LOMO) before and after the adsorption of C2H4 and CO molecules on pristine and doped HfSe2. Compared with the pristine substrate material, the difference value between the LOMO and HOMO has a slight increase, which is 0.11 and 0.10 eV, respectively. Figure 7 shows the frontier molecular orbital of the doped system, in which the corresponding bandgap between the LOMO and HOMO is 0.52 eV. Moreover, the LOMO and HOMO orbitals are mainly distributed on the side of the doped sites of the Ag3 cluster. However, the distribution of the LOMO and HOMO is stretched to the C2H4 and CO molecules obviously after the interaction between Ag3–HfSe2 and adsorbed gas molecules. During the adsorption process of the doped system, there have been incremental changes to various extents, which are 0.15 and 0.03 eV for C2H4 and CO systems, respectively.
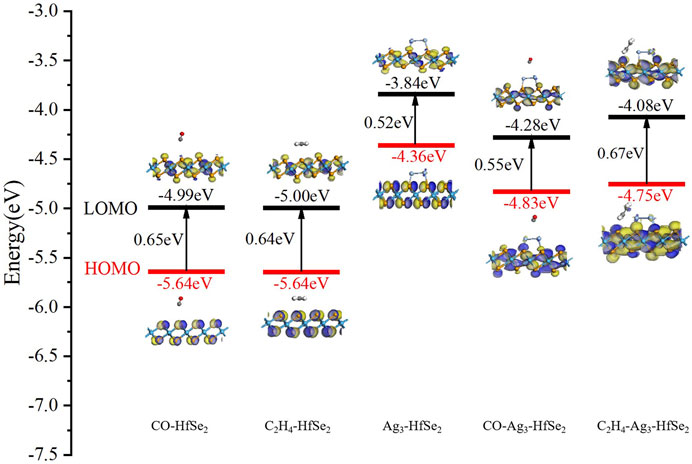
FIGURE 7. Frontier molecular orbital distributions of CO and C2H4 adsorption on pristine and Ag3–HfSe2 monolayers, respectively.
In accordance with the molecular orbital theory, the decrease in the bandgap means that the charge transfer of the system is harder. In other words, at a constant temperature, the wider the forbidden band, the harder it is for the electrons to be transferred from the valence band to the conduction band. As a result, from a macro point of view, it is demonstrated as an increase in the resistance but a decrease in the conductivity (Wu et al., 2022). The relation between resistivity and bandgap can be described as follows.
Here,
Analysis of Recovery Characteristics and Electrical Sensitivity
Last but not the least, it is the recovery properties that must be considered for the sensing materials, in which the recovery time is an important reference. Recovery time, also regarded as desorption time, refers to the desorption rate of the adsorbed gas molecules on a gas sensor. Generally speaking, the faster the recovery time, the better is the performance of the gas sensor. The desorption time is defined as formula (4-1).
Here,
In addition to the desorption time analyzed previously, it is the electrical sensitivity (ES) that must be considered, which is defined in the following equation.
Here,
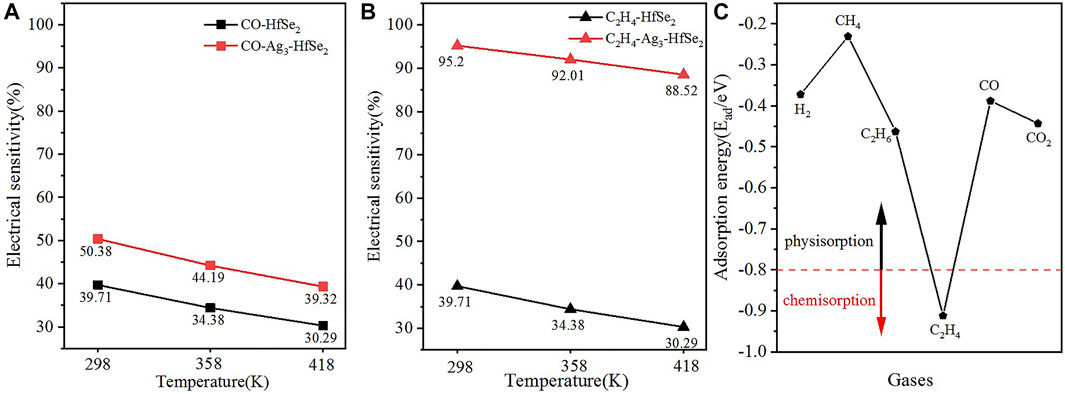
FIGURE 8. Electrical sensitivity of (A) CO and (B)C2H4 on pristine and doped adsorption systems. (C) Comparison of the adsorption energy of different gases.
To elucidate the anti-interference for the detection of the C2H4 molecule, the adsorption energy of different dissolved gases in oil was compared. The gases are H2, CH4, C2H6, C2H4, CO, and CO2 respectively. As is shown in Figure 8C, it can be seen that C2H4 is below the boundary at −0.8 eV, while other gases are above the boundary. In other words, only the adsorption of C2H4 is chemisorption while others are defined as physisorption. As a consequence, it can be concluded that the detection of C2H4 based on the Ag3–HfSe2 monolayer is of great anti-interference (Aziz et al., 2019).
Conclusion
In this article, the adsorption properties of the transformer oil decomposition gases (CO and C2H4) upon Ag3–HfSe2 are investigated theoretically on the basis of the first principle. The electronic behavior of Ag3–HfSe2 before and after the adsorption is analyzed and discussed by the band structure, DOS, DCD, and the Mulliken analysis. However, the desorption time is calculated through the adsorption energy, and the electrical sensitivity is analyzed through the bandgap and the frontier molecular orbital theory (Das and Shoji, 2011). After analyzing the adsorption behaviors and the response mechanisms of CO and C2H4, the main conclusions obtained are as follows. For the doping of the Ag3 cluster, the simulation results show that the Ag3 cluster is more inclined to be doped at the site Tse1. There are different adsorption interactions between the gases CO, C2H4, and the Ag3–HfSe2 monolayer, including the strong chemical adsorption of C2H4 and the weak physisorption of CO, respectively. In the adsorption process, C2H4 was attracted to transfer electrons to the monolayer of Ag3–HfSe2, whereas CO was just the reverse. After adsorption, the increase in the bandgap contributes to the increase in resistivity of Ag3–HfSe2, which corresponds to a decrease in conductivity. The resistivity relationship corresponding to the two adsorption systems is as follows: C2H4 > CO. After the doping of the Ag3 cluster, the electrical sensitivity has a great change for both the gases, including the change from 39.71 to 50.78% for CO and the change from 39.71 to 95.2% for C2H4, respectively. Speaking of the anti-interference of the detection for C2H4, the adsorption energy of different gases was compared. The results illustrate that the detection for C2H4 based on Ag3–HfSe2 is of great anti-interference. Our investigation highlights the high selectivity and better adsorption performance of C2H4 on the Ag3–HfSe2 monolayer. It provides guidance for expanding the application range of HfSe2 to the sensing materials and analyzes its feasibility to detect transformer oil decomposition theoretically.
Data Availability Statement
The original contributions presented in the study are included in the article/supplementary material; further inquiries can be directed to the corresponding authors.
Author Contributions
All authors listed have made a substantial, direct, and intellectual contribution to the work and approved it for publication.
Funding
This work has been supported in part by the National Natural Science Foundation of China (Nos. 52077177 and 51507144) and the Fundamental Research Funds for the Central Universities (No. XDJK 2019B021).
Conflict of Interest
The authors declare that the research was conducted in the absence of any commercial or financial relationships that could be construed as a potential conflict of interest.
Publisher’s Note
All claims expressed in this article are solely those of the authors and do not necessarily represent those of their affiliated organizations, or those of the publisher, the editors, and the reviewers. Any product that may be evaluated in this article, or claim that may be made by its manufacturer, is not guaranteed or endorsed by the publisher.
References
Allian, A. D., Takanabe, K., Fujdala, K. L., Hao, X., Truex, T. J., Cai, J., et al. (2011). Chemisorption of CO and Mechanism of CO Oxidation on Supported Platinum Nanoclusters. J. Am. Chem. Soc. 133, 4498–4517. doi:10.1021/ja110073u
Ambrusi, R. E., Luna, C. R., Sandoval, M. G., Bechthold, P., Pronsato, M. E., and Juan, A. (2017). Rhodium Clustering Process on Defective (8,0) SWCNT: Analysis of Chemical and Physical Properties Using Density Functional Theory. Appl. Surf. Sci. 425, 823–832. doi:10.1016/j.apsusc.2017.07.070
Asif, M., Aziz, A., Wang, H., Wang, Z., Wang, W., Ajmal, M., et al. (2019a). Superlattice Stacking by Hybridizing Layered Double Hydroxide Nanosheets with Layers of Reduced Graphene Oxide for Electrochemical Simultaneous Determination of Dopamine, Uric Acid and Ascorbic Acid. Mikrochim. Acta 186, 61. doi:10.1007/s00604-018-3158-y
Asif, M., Aziz, A., Ashraf, G., Iftikhar, T., Sun, Y. M., Xiao, F., et al. (2022). Unveiling Microbiologically Influenced Corrosion Engineering to Transfigure Damages into Benefits: A Textile Sensor for H2O2 Detection in Clinical Cancer Tissues. Chem. Eng. J. 427, 11. doi:10.1016/j.cej.2021.131398
Asif, M., Aziz, A., Ashraf, G., Wang, Z., Wang, J., Azeem, M., et al. (2018). Facet-Inspired Core-Shell Gold Nanoislands on Metal Oxide Octadecahedral Heterostructures: High Sensing Performance toward Sulfide in Biotic Fluids. ACS Appl. Mat. Interfaces 10, 36675–36685. doi:10.1021/acsami.8b12186
Asif, M., Aziz, A., Wang, Z., Ashraf, G., Wang, J., Luo, H., et al. (2019b). Hierarchical CNTs@CuMn Layered Double Hydroxide Nanohybrid with Enhanced Electrochemical Performance in H2S Detection from Live Cells. Anal. Chem. 91, 3912–3920. doi:10.1021/acs.analchem.8b04685
Aziz, A., Asif, M., Ashraf, G., Iftikhar, T., Hu, J. L., Xiao, F., et al. (2022). Boosting Electrocatalytic Activity of Carbon Fiber@fusiform-like Copper-Nickel LDHs: Sensing of Nitrate as Biomarker for NOB Detection. J. Hazard. Mater. 422, 11. doi:10.1016/j.jhazmat.2021.126907
Aziz, A., Asif, M., Azeem, M., Ashraf, G., Wang, Z., Xiao, F., et al. (2019). Self-stacking of Exfoliated Charged Nanosheets of LDHs and Graphene as Biosensor with Real-Time Tracking of Dopamine from Live Cells. Anal. Chim. Acta 1047, 197–207. doi:10.1016/j.aca.2018.10.008
Chen, D., Zhang, X., Tang, J., Cui, H., Li, Y., Zhang, G., et al. (2019a). Density Functional Theory Study of Small Ag Cluster Adsorbed on Graphyne. Appl. Surf. Sci. 465, 93–102. doi:10.1016/j.apsusc.2018.09.096
Chen, D., Zhang, X., Xiong, H., Li, Y., Tang, J., Xiao, S., et al. (2019b). A First-Principles Study of the SF6 Decomposed Products Adsorbed over Defective WS2 Monolayer as Promising Gas Sensing Device. IEEE Trans. Device Mat. Relib. 19, 473–483. doi:10.1109/tdmr.2019.2919773
Chen, W. L., Gui, Y. G., Li, T., Zeng, H., Xu, L. N., and Ding, Z. Y. (2020). Gas-sensing Properties and Mechanism of Pd-GaNNTs for Air Decomposition Products in Ring Main Unit. Appl. Surf. Sci. 531, 7. doi:10.1016/j.apsusc.2020.147293
Choi, S.-J., and Kim, I.-D. (2018). Recent Developments in 2D Nanomaterials for Chemiresistive-type Gas Sensors. Electron. Mat. Lett. 14, 221–260. doi:10.1007/s13391-018-0044-z
Cortés-Arriagada, D., Villegas-Escobar, N., and Ortega, D. E. (2018). Fe-doped Graphene Nanosheet as an Adsorption Platform of Harmful Gas Molecules (CO, CO2, SO2 and H2S), and the Co-adsorption in O2 Environments. Appl. Surf. Sci. 427, 227–236. doi:10.1016/j.apsusc.2017.08.216
Cruzado, H. N., Dizon, J. S. C., Macam, G. M., Villaos, R. A. B., Huynh, T. M. D., Feng, L.-Y., et al. (2021). Band Engineering and Van Hove Singularity on HfX2 Thin Films (X = S, Se, or Te). ACS Appl. Electron. Mat. 3, 1071–1079. doi:10.1021/acsaelm.0c00907
Cui, H., Jia, P. F., and Peng, X. Y. (2020a). Adsorption of SO2 and NO2 Molecule on Intrinsic and Pd-Doped HfSe2 Monolayer: A First-Principles Study. Appl. Surf. Sci. 513, 7. doi:10.1016/j.apsusc.2020.145863
Cui, H., Jia, P. F., Peng, X. Y., and Li, P. (2020b). Adsorption and Sensing of CO and C2H2 by S-Defected SnS2 Monolayer for DGA in Transformer Oil: A DFT Study. Mater. Chem. Phys. 249, 7. doi:10.1016/j.matchemphys.2020.123006
Cui, H., Zhang, X., Zhang, G., and Tang, J. (2019). Pd-doped MoS2 Monolayer: A Promising Candidate for DGA in Transformer Oil Based on DFT Method. Appl. Surf. Sci. 470, 1035–1042. doi:10.1016/j.apsusc.2018.11.230
Cui, H., Zhu, H. L., and Jia, P. F. (2020c). Adsorption and Sensing of SO2 and SOF2 Molecule by Pt-Doped HfSe2 Monolayer: A First-Principles Study. Appl. Surf. Sci. 530, 7. doi:10.1016/j.apsusc.2020.147242
Das, N. K., and Shoji, T. (2011). A Density Functional Study of Atomic Oxygen and Water Molecule Adsorption on Ni(111) and Chromium-Substituted Ni(111) Surfaces. Appl. Surf. Sci. 258, 442–447. doi:10.1016/j.apsusc.2011.08.107
Delley, B. (2000). From Molecules to Solids with the DMol3 Approach. J. Chem. Phys. 113, 7756–7764. doi:10.1063/1.1316015
Duerloo, K. A., Li, Y., and Reed, E. J. (2014). Structural Phase Transitions in Two-Dimensional Mo- and W-Dichalcogenide Monolayers. Nat. Commun. 5, 4214. doi:10.1038/ncomms5214
Gao, X., Zhou, Q., Wang, J., Xu, L., and Zeng, W. (2020). Performance of Intrinsic and Modified Graphene for the Adsorption of H2S and CH4: A DFT Study. Nanomater. (Basel) 10, 15. doi:10.3390/nano10020299
Gao, X., Zhou, Q., Lu, Z. R., Xu, L. N., Zhang, Q. Y., and Zeng, W. (2019). Synthesis of Cr2O3 Nanoparticle-Coated SnO2 Nanofibers and C2H2 Sensing Properties. Front. Mater. 6, 8. doi:10.3389/fmats.2019.00163
Gui, X. X., Zhou, Q., Peng, S. D., Xu, L. N., and Zeng, W. (2020). Dissolved Gas Analysis in Transformer Oil Using Sb-Doped Graphene: A DFT Study. Appl. Surf. Sci. 533, 11. doi:10.1016/j.apsusc.2020.147509
Hu, X. Y., Liu, M., Liu, X. Y., Ma, Y. X., Nan, H. S., Bi, D. M., et al. (2019). Sensing and Absorbing of Sulfur Mustard Using Pt-Decorated Graphene from First-Principles Calculations. Phys. E-Low-Dimensional Syst. Nanostructures 114, 6. doi:10.1016/j.physe.2019.113634
Ju, W., Li, T., Su, X., Li, H., Li, X., and Ma, D. (2017). Au Cluster Adsorption on Perfect and Defective MoS2 Monolayers: Structural and Electronic Properties. Phys. Chem. Chem. Phys. 19, 20735–20748. doi:10.1039/c7cp03062b
Ju, W. W., Zhang, Y., Li, T. W., Wang, D. H., Zhao, E. Q., Hu, G. X., et al. (2021). A type-II WSe2/HfSe2 van der Waals heterostructure with adjustable electronic and optical properties. Results Phys. 25, 10. doi:10.1016/j.rinp.2021.104250
Khan Musa, M. R., Zhang, C., Alruqui, A. B. A., Zhao, R., Jasinski, J. B., Sumanasekera, G., et al. (2020). Insight the Process of Hydrazine Gas Adsorption on Layered WS2: a First Principle Study. Nanotechnology 31, 495703. doi:10.1088/1361-6528/abb337
Late, D. J., Doneux, T., and Bougouma, M. (2014). Single-layer MoSe2 Based NH3 Gas Sensor. Appl. Phys. Lett. 105, 4. doi:10.1063/1.4903358
Liao, Y. M., Peng, R. C., Peng, S. D., Zeng, W., and Zhou, Q. (2021). The Adsorption of H2 and C2H2 on Ge-Doped and Cr-Doped Graphene Structures: A DFT Study. Nanomaterials 11, 13. doi:10.3390/nano11010231
Liu, Y. P., Zhou, Q., Mi, H. W., Wang, J. X., and Zeng, W. (2021). Gas-sensing Mechanism of Cr Doped SnP3 Monolayer to SF6 Partial Discharge Decomposition Components. Appl. Surf. Sci. 546, 9. doi:10.1016/j.apsusc.2021.149084
Ma, D., Wang, Q., Li, T., He, C., Ma, B., Tang, Y., et al. (2016). Repairing Sulfur Vacancies in the MoS2 Monolayer by Using CO, NO and NO2 Molecules. J. Mat. Chem. C 4, 7093–7101. doi:10.1039/c6tc01746k
Mi, H. W., Zhou, Q., and Zeng, W. (2021). A Density Functional Theory Study of the Adsorption of Cl2, NH3, and NO2 on Ag3-Doped WSe2 Monolayers. Appl. Surf. Sci. 563, 9. doi:10.1016/j.apsusc.2021.150329
Mirabelli, G., Mcgeough, C., Schmidt, M., Mccarthy, E. K., Monaghan, S., Povey, I. M., et al. (2016). Air Sensitivity of MoS2, MoSe2, MoTe2, HfS2, and HfSe2. J. Appl. Phys. 120, 9. doi:10.1063/1.4963290
Mleczko, M. J., Zhang, C., Lee, H. R., Kuo, H. H., Magyari-Köpe, B., Moore, R. G., et al. (2017). HfSe2 and ZrSe2: Two-Dimensional Semiconductors with Native High-κ Oxides. Sci. Adv. 3, e1700481. doi:10.1126/sciadv.1700481
Qian, G. C., Peng, Q. J., Zou, D. X., Wang, S., Yan, B., and Zhou, Q. (2020). First-Principles Insight into Au-Doped MoS2 for Sensing C2H6 and C2H4. Front. Mater. 7, 9. doi:10.3389/fmats.2020.00022
Sharma, A., AnuKHAN, M. S., Khan, M. S., Husain, M., Khan, M. S., and Srivastava, A. (2018). Sensing of CO and NO on Cu-Doped MoS2Monolayer-Based Single Electron Transistor: A First Principles Study. IEEE Sensors J. 18, 2853–2860. doi:10.1109/jsen.2018.2801865
Si, K. Y., Ma, J. Y., Lu, C. H., Zhou, Y. X., He, C., Yang, D., et al. (2020). A two-dimensional MoS2/WSe2 van der Waals heterostructure for enhanced photoelectric performance. Appl. Surf. Sci. 507, 11. doi:10.1016/j.apsusc.2019.145082
Singh, S., and Bandyopadhyay, M. (2010). Dissolved Gas Analysis Technique for Incipient Fault Diagnosis in Power Transformers: A Bibliographic Survey. IEEE Electr. Insul. Mag. 26, 41–46. doi:10.1109/mei.2010.5599978
Tang, S. R., Chen, W. G., Jin, L. F., Zhang, H., Li, Y. Q., Zhou, Q., et al. (2020). SWCNTs-based MEMS Gas Sensor Array and its Pattern Recognition Based on Deep Belief Networks of Gases Detection in Oil-Immersed Transformers. Sensors Actuators B-Chemical 312, 12. doi:10.1016/j.snb.2020.127998
Topsakal, M., Akturk, E., and Ciraci, S. (2009). First-principles Study of Two- and One-Dimensional Honeycomb Structures of Boron Nitride. Phys. Rev. B 79, 11. doi:10.1103/physrevb.79.115442
Tran, D., Mac, H., Tong, V., Tran, H. A., and Nguyen, L. G. (2018). A LSTM Based Framework for Handling Multiclass Imbalance in DGA Botnet Detection. Neurocomputing 275, 2401–2413. doi:10.1016/j.neucom.2017.11.018
Wang, J. X., Zhou, Q., Xu, L. N., Gao, X., and Zeng, W. (2020). Gas Sensing Mechanism of Dissolved Gases in Transformer Oil on Ag-MoS2 Monolayer: A DFT Study. Phys. E-Low-Dimensional Syst. Nanostructures 118, 9. doi:10.1016/j.physe.2019.113947
Wang, X., and Liao, Y. L. (2019). Selective Detection of SO2 in SF6 Insulation Devices by Rh-Doped HfSe2 Monolayer: a First-Principles Study. Appl. Phys. a-Materials Sci. Process. 125, 8. doi:10.1007/s00339-019-2759-6
Wei, Z., Xu, L., Peng, S., and Zhou, Q. (2020). Application of WO3 Hierarchical Structures for the Detection of Dissolved Gases in Transformer Oil: A Mini Review. Front. Chem. 8, 188. doi:10.3389/fchem.2020.00188
Wu, H. L., Zhang, B., Li, X. X., and Hu, X. X. (2022). First-principles Screening upon Pd-Doped HfSe2 Monolayer as an Outstanding Gas Sensor for DGA in Transformers. Comput. Theor. Chem. 1208, 7. doi:10.1016/j.comptc.2021.113553
Wu, P., Yin, N., Li, P., Cheng, W., and Huang, M. (2017). The Adsorption and Diffusion Behavior of Noble Metal Adatoms (Pd, Pt, Cu, Ag and Au) on a MoS2 Monolayer: a First-Principles Study. Phys. Chem. Chem. Phys. 19, 20713–20722. doi:10.1039/c7cp04021k
Xu, L., Zhu, H., Gui, Y., Long, Y., Wang, Q., and Yang, P. (2020). First-Principles Calculations of Gas-Sensing Properties of Pd Clusters Decorated AlNNTs to Dissolved Gases in Transformer Oil. Ieee Access 8, 162692–162700. doi:10.1109/access.2020.3020636
Xu, M., Liang, T., Shi, M., and Chen, H. (2013). Graphene-Like Two-Dimensional Materials. Chem. Rev. 113, 3766–3798. doi:10.1021/cr300263a
Yang, G. F., Yan, P. F., Zhu, C., Gu, Y., Lu, N. Y., Xue, J. J., et al. (2019). Selenium Vacancy-Enhanced Gas Adsorption of Monolayer Hafnium Diselenide (HfSe2) from a Theoretical Perspective. Adv. Theory Simulations 2, 8. doi:10.1002/adts.201900052
Yang, S., Chen, X., Gu, Z., Ling, T., Li, Y., and Ma, S. (2020). Cu-Doped MoSe2 Monolayer: A Novel Candidate for Dissolved Gas Analysis in Transformer Oil. Acs Omega 5, 30603–30609. doi:10.1021/acsomega.0c04572
Yue, R., Barton, A. T., Zhu, H., Azcatl, A., Pena, L. F., Wang, J., et al. (2015). HfSe2 Thin Films: 2D Transition Metal Dichalcogenides Grown by Molecular Beam Epitaxy. Acs Nano 9, 474–480. doi:10.1021/nn5056496
Zhang, D., Sun, Y. E., Jiang, C., Yao, Y., Wang, D., and Zhang, Y. (2017). Room-temperature Highly Sensitive CO Gas Sensor Based on Ag-Loaded Zinc Oxide/molybdenum Disulfide Ternary Nanocomposite and its Sensing Properties. Sensors Actuators B Chem. 253, 1120–1128. doi:10.1016/j.snb.2017.07.173
Zhang, Y. H., Chen, Y. B., Zhou, K. G., Liu, C. H., Zeng, J., Zhang, H. L., et al. (2009). Improving Gas Sensing Properties of Graphene by Introducing Dopants and Defects: a First-Principles Study. Nanotechnology 20, 185504. doi:10.1088/0957-4484/20/18/185504
Zhou, Q., Lu, Z., Wei, Z., Xu, L., Gui, Y., and Chen, W. (2018a). Hydrothermal Synthesis of Hierarchical Ultrathin NiO Nanoflakes for High-Performance CH4 Sensing. Front. Chem. 6, 194. doi:10.3389/fchem.2018.00194
Keywords: the first-principle study, HfSe2 monolayer, Ag3 doping, gas adsorption, transformer oil
Citation: Jia L, Chen J, Cui X, Wang Z, Zeng W and Zhou Q (2022) Gas Sensing Mechanism and Adsorption Properties of C2H4 and CO Molecules on the Ag3–HfSe2 Monolayer: A First-Principle Study. Front. Chem. 10:911170. doi: 10.3389/fchem.2022.911170
Received: 02 April 2022; Accepted: 13 April 2022;
Published: 12 May 2022.
Edited by:
Muhammad Asif, Wuhan Institute of Technology, ChinaReviewed by:
Shenqi Wang, Huazhong University of Science and Technology, ChinaAbdul Rahman Rahman, Zhejiang University, China
Copyright © 2022 Jia, Chen, Cui, Wang, Zeng and Zhou. This is an open-access article distributed under the terms of the Creative Commons Attribution License (CC BY). The use, distribution or reproduction in other forums is permitted, provided the original author(s) and the copyright owner(s) are credited and that the original publication in this journal is cited, in accordance with accepted academic practice. No use, distribution or reproduction is permitted which does not comply with these terms.
*Correspondence: Qu Zhou, emhvdXF1QHN3dS5lZHUuY24=; Wen Zeng, d2VuemVuZ0BjcXUuZWR1LmNu