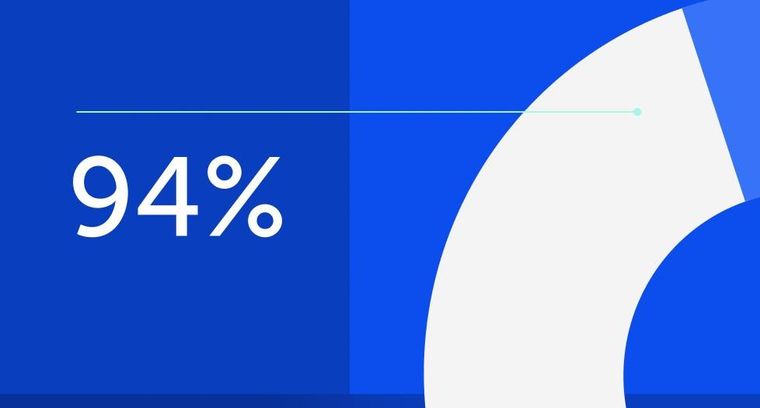
94% of researchers rate our articles as excellent or good
Learn more about the work of our research integrity team to safeguard the quality of each article we publish.
Find out more
ORIGINAL RESEARCH article
Front. Chem., 19 May 2022
Sec. Polymer Chemistry
Volume 10 - 2022 | https://doi.org/10.3389/fchem.2022.902487
This article is part of the Research TopicPolymers for High Electric Field ApplicationsView all 9 articles
Dielectrics with improved energy density have long-standing demand for miniature and lightweight energy storage capacitors for electrical and electronic systems. Recently, polyvinylidene fluoride (PVDF)-based ferroelectric polymers have shown attractive energy storage performance, such as high dielectric permittivity and high breakdown strength, and are regarded as one of the most promising candidates. However, the non-negligible energy loss and inferior temperature stability of PVDF-based polymers deteriorated the energy storage performance or even the thermal runaway, which could be ascribed to vulnerable amorphous regions at elevated temperatures. Herein, a new strategy was proposed to achieve high energy density and high temperature stability simultaneously of PVDF/PMMA blends by in situ polymerization. The rigidity of the amorphous region was ideally strengthened by in situ polymerization of methyl methacrylate (MMA) monomers in a PVDF matrix to obtain PVDF/PMMA blends. The atomic force microscopic study of the microstructure of etched films showed the ultra-homogenous distribution of PMMA with high glass transition temperature in the PVDF matrix. Consequently, the temperature variation was remarkably decreased, while the high polarization response was maintained. Accordingly, the high energy density of ∼8 J/cm3 with ∼80% efficiency was achieved between 30 and 90 °C in PVDF/PMMA films with 39–62% PMMA content, outperforming most of the dielectric polymers. Our work could provide a general solution to substantially optimize the temperature stability of dielectric polymers for energy storage applications and other associated functions.
Energy storage devices with dielectric material play a critical role in electronics and electrical power systems such as hybrid vehicles, renewable energy systems, and electromagnetic systems (Tan, 2020; Wu et al., 2022). The storage of electrostatic energy depends on electric-field–induced polarization of dielectric materials, requiring high dielectric permittivity, high breakdown strength, and low loss. Polymers are one of the most widely used dielectric materials due to their advantages of high breakdown strength and scalability. Nowadays, the demand for higher energy and energy storage capacitors with higher power is rapidly growing. Taking hybrid vehicles as an example, the energy storage capacitors are required to be lightweight and should be able to withstand high temperatures of the nearby heat source like engines (Li et al., 2021; Li et al., 2016). This makes it highly challenging to prepare next generation dielectric polymers with high energy density and high temperature stability.
The energy density (ue) stored in dielectric materials can be presented by the following equation:
where E is the electric field, and D is the electrical displacement (Chu et al., 2006). It is evident that energy density mainly depends on the breakdown strength (Eb) and polarization response (P) of dielectric materials. At present, biaxially oriented polypropylene (PP) is the most widely used dielectric material for energy storage, owing to its high breakdown strength, low dielectric loss, and ease of manufacturing (Zheng et al., 2018). However, the shortcomings of polypropylene are its inherent low permittivity (εr ≈ 2.2) and consequently low energy density (∼2 J/cm3). Recently, poly (vinylidene fluoride) (PVDF) and its copolymers have gained a lot of attention, owing to their high permittivity (εr ≈ 10–50), which enables them to achieve a high energy density (4−30 J/cm3) (Prateek et al., 2016; Baer and Zhu, 2017; Zha et al., 2021). However, at elevated temperature, the working voltage rate for PVDF-based dielectrics must be reduced without extra cooling devices due to their poor temperature stability (Li et al., 2015; Li et al., 2021).
Such a dilemma arises due to the contrasting features between the high energy density and robust temperature stability required for the mobility of molecular chains. To achieve high energy density, the mobility of activated molecular chains favors easy switching of polarization under external electric fields and consequently high polarization response. On the other hand, to maintain robust temperature stability, freezing of molecular motion is preferred to prevent an upsurge of current leakage and free volume (Rajib et al., 2015; Zhou et al., 2018; Zhou et al., 2020; Liu et al., 2022). Therefore, the energy storage performance of PVDF-based dielectrics is usually exciting at room temperature. However, the elevation of working temperature up to 70°C, results in a drop of energy density by 22–80% (Khanchaitit et al., 2013; Rajib et al., 2015; Li et al., 2016; Liu et al., 2017). Research works on enhancing temperature stability of polymer dielectric focus on the rigidity of chains through either chemical means or physical approach involving the spatial confinement effect. For example, Li et al. crosslinked poly (chlorotrifluoroethylene-co-vinylidene fluoride), which showed improvement in the energy density and efficiency from 0.57 J/cm3 and 37.9% to 4.33 J/cm3 and 70% at 150°C, respectively (Li et al., 2020). A variety of polymers with aromatic groups or fused-ring heterocyclic configurations on backbones, such as polyetherimide (PEI), polyether ether ketone (PEEK), and polytetrafluoroethylene (PTFE), have been investigated at higher temperatures (above 150°C) (Li et al., 2019; Zhang et al., 2021; Wu et al., 2022). The chemical modifications of polymer chains can significantly reinforce thermal stability but at the cost of polarization response. Using the physical approach, Zhu et al. laminated PVDF with polycarbonate (PC), thus enabling the working of layered materials at 170°C (Yin et al., 2019). In our previous work, blending of PVDF with high glass transition temperature (Tg) polymethyl methacrylate (PMMA) introduced a spatial confinement effect on the structure upon heating, which brought about the stabilization of energy density around 7.8−9.8 J/cm3 between 30 and 70°C (Liu et al., 2019). However, the confinement provided by van der Waals forces of physical modification is inferior to that achieved through chemical approaches. Consequently, the realization of high energy density and robust temperature stability in polymer dielectrics remains a big challenge.
In this work, an in situ polymerization approach of PMMA in the PVDF matrix was proposed to achieve ultra-homogeneous distribution and thereby enhance its confinement effect on temperature stability. Blending by in situ polymerization was realized through the polymerization of MMA in the PVDF solution and confirmed by nuclear magnetic resonance spectroscopy and thermogravimetric analysis. It was evident that the enhanced homogeneous dispersion significantly improved the structural stability upon heating and thus improved the energy storage performance at elevated temperature. Our finding provides a general approach for developing high-performance polymer blends to achieve high energy density and robust temperature stability simultaneously.
PVDF powder was purchased from Alfa Aesar, whereas MMA monomers were purchased from TCI. Azodiisobutyronitrile (AIBN), methanol, and N,N-dimethylformamide (DMF) were purchased from Aladdin, and acetic acid was purchased from Macklin.
PVDF/PMMA blends were prepared by in situ polymerization. First, PVDF powder was dissolved in DMF in a 10% weight ratio. MMA monomer was added into the solution, according to its weight ratio in the blend (20 wt% to 80 wt%, 10 wt% per step, and 100%). After the solution was stirred to homogenize, it was cooled down to about -70°C and protected from light, followed by the addition of AIBN (1% of MMA). Second, the solution was polymerized at 75°C for 2 h under the nitrogen atmosphere. Finally, the samples were obtained as sediments from methanol and dried (Hussein et al., 2016; Hussein et al., 2018). PVDF/PMMA films (20 mm × 20 mm) were prepared by solution casting, followed by heat treatment at 200°C for 10 min, and then quenched with ice water.
The characteristic groups of PMMA were measured by nuclear magnetic resonance to confirm the successful polymerization of PMMA after the fabrication process. The successful polymerization of PMMA was verified using the 1H spectrum of nuclear magnetic resonance (NMR, JEOL 400 MHz). Atomic force microscopy (AFM, Bruker Icon) was employed to study the morphology of polymer films. Prior to observation, the blend films were pre-treated (etched) with glacial acetic acid for 1 h to remove the PMMA component, which helped to determine the distribution of PVDF and PMMA. The thermodynamic properties of the specimens were measured by thermogravimetric analysis (TGA, METTLER TOLEDO) and differential scanning calorimetry (DSC, Discover DSC250) at a heating rate of 10 °C/min. The crystalline structures of the specimens were analyzed by Fourier transform infrared spectrometry (FTIR, NICOLET6700) and X-ray diffractometry (XRD, Bruker Advance D8). To evaluate the variation of the structure with temperature rise, the in situ heating small-angle X-ray scattering (SAXS, Anton-Paar) measurement was conducted on the specimens with two-slit–collimated Cu–Kα radiation (λ = 1.54 Å). Dielectric permittivity and loss tangent were determined at 1 kHz using an LCR impedance analyzer (HIOKI IM3536), with circular gold electrode diameter = 10 mm. The electrical displacement (D)–electric field (E) hysteresis loops were obtained using a ferroelectric workstation (PolyK Technologies) with a 1,000 Hz unipolar triangular signal to determine the energy density and its associated efficiency with temperature changes, with circular gold electrode diameter = 3 mm.
The in situ polymerization process of PMMA in the PVDF solution is depicted in Figure 1. The polymerization of MMA was initiated by AIBN at 75°C, and sufficient dispersion of PMMA was formed in the PVDF matrix. To confirm the successful polymerization of PMMA in the PVDF matrix, the PVDF/PMMA solutions are estimated by nuclear magnetic resonance which indicates the existence of characteristic -O-CH3 groups of PMMA, as shown in Supplementary Figure S1 (Mccord et al., 1994). Considering the possible variations during polymerization, the final weight ratios of the two phases in the blends were measured by TGA.
FIGURE 1. Schematic representation of the preparation process of PVDF/PMMA blends. (A) Synthesis process of PMMA and (B) the preparation process of PVDF/PMMA blends.
As shown in Figure 2, PVDF/PMMA with a designed ratio of 70/30 was chosen as an example to determine the final weight ratio. PVDF/PPMMA blends underwent the four stages of thermal decomposition, which corresponded sequentially to the volatilization of residual DMF molecules, end-initiated scission of the unstable end groups of PMMA chains, random scission of PMMA chains, and decomposition of PVDF (Hirata et al., 1985; Botelho et al., 2008). Thereafter, PMMA lost 100% of its weight, whereas PVDF lost 62.5% (Nguyen, 1985). Accordingly, the proportion of PMMA (M(PMMA)) in the blends could be calculated by the following equation:
where TG (PMMA) and TG (PVDF) are mass fractions of PMMA and PVDF, respectively. Thus, the weight ratio of PMMA was found to be 24.7%, slightly lower than the designed value of 30%. The TGA results of other samples are shown in Supplementary Figure S4, wherein the realized ratios of the blends were 0, 6.0, 19.5, 24.7, 39.0, 56.8, 62.1, and 74.3%. The PMMA ratio was evidently lower than its designed value, which could be ascribed to dilution of MMA by the PVDF solution.
DSC and FTIR analyses were conducted to investigate the influence of in situ polymerization on the microstructure and crystallinity of the blend. The DSC profiles in Figure 3A indicate that the semi-crystalline feature of the blends gradually turned amorphous with the increase in the PMMA content. The crystallinity, derived from the melting enthalpy in the endothermic heat flow curve, decreased from 51% for neat PVDF to 4% for PVDF/PMMA (44/56), and the peak for melting enthalpy disappeared when the PMMA content was more than 56.8%. These results of crystallinity were further corroborated by FTIR spectral analysis, as displayed in Figure 3B. The characteristic absorption peaks for the α-phase of PVDF around 532, 614, 763, 795, 854, 975, 1,149, 1,209, and 1,383 cm−1 blurred when the PMMA content was above 39%, and those corresponding to the β-phase of PVDF around 840 and 881 cm−1 blurred when the PMMA content was above 74% (Cai et al., 2017). Therefore, the phase structure of PVDF turns from the α phase to the β phase gradually with PMMA addition, which is also evidenced by XRD results, as shown in Supplementary Figure S3. Also, both DSC and FTIR results suggested that the PVDF/PMMA blends experienced a drop in crystallinity and turned amorphous when the PMMA contents reached 56–74%. Furthermore, the melting temperature (Tm) of the blends on the DSC profiles decreases with PMMA addition from 160.9°C for pristine PVDF to 151.8°C for PVDF/PMMA (43.2/56.8), suggesting the suppression of PVDF crystallization and imperfection of the PVDF crystalline structure. The amorphous regions were characterized by glass transition temperature, which manifests itself as a step in DSC profiles. Glass transition temperatures of PVDF and PMMA were about −40 and 105°C, respectively. In the DSC profiles of the blends, they merged into one step, which revealed exceedingly homogenous distribution of PVDF and PMMA in the amorphous regions due to in situ polymerization.
AFM height profiles further helped to evaluate the distribution of PVDF and PMMA in the blends. Prior to AFM analysis, the blend films were pre-treated (etched) with glacial acetic acid for 1 h to remove the PMMA component. This procedure specifically dissolved PMMA and thus helped to determine the distribution of PVDF and PMMA. The bright yellow region in the AFM image indicated PVDF, and the dark yellow region indicated the cavity caused by the elimination of PMMA, as shown in Figure 4. The morphology revealed that the distribution of PVDF and PMMA was ultra-fine (∼μm) across the entire range of ratio, illustrating highly homogenous dispersion caused by in situ polymerization (Kang et al., 2009; Dong et al., 2019).
FIGURE 4. AFM height images of the blends with PMMA contents of (A) 39%, (B) 56.8%, (C) 62.1 and (D) 74.3% after etching.
Homogenous dispersion could gently stabilize the structure upon heating by its adequate molecular contact (Wunderlich, 2003; Wei et al., 2015; Huang et al., 2018). To evaluate the confinement effect of in situ polymerization, the long period of PVDF/PMMA was assessed through one-dimensional correlation function (K(z)) of SAXS (Hu and Tashiro, 2016). The long period refers to the thickness of each lamella and amorphous layer in the crystalline stacks. Since the lamellar volume remains stable on heating below melting temperature, the variation over the long period mainly comes from the amorphous regions. The SAXS spectra of the PVDF/PMMA blends are shown in Supplementary Figure S5, in which the scattering peak of crystalline stacks diminished when the PMMA content reached 39%. Therefore, the one-dimensional fitting was only performed for the blends exhibiting an obvious scattering peak; the K(z) values of PVDF/PMMA with PMMA contents of 0%, 6%, 19.5, and 24.7% in the temperature range of 20–100°C are shown in Figure 5. The percentage of expansion for a long period was calculated by comparing its variations and is denoted in the figures.
FIGURE 5. K(z) values from SAXS of the blends with PMMA contents of (A) 0%, (B) 6%, (C)19.5, and (D) 24.7%.
Upon heating, the expansion of long period decreased from 9.5% for PVDF to 2.9% for PVDF/PMMA (75/25), demonstrating the enhanced rigidity of amorphous regions due to in situ polymerization (Fatnassi et al., 2010; Gumyusenge et al., 2018).
Figure 6 shows variations in dielectric permittivity (εr) and dielectric loss (tgδ) with temperature ranging from -100 to 90°C at different frequencies (60 Hz, 100 Hz, 1 kHz, 10 kHz, and 100 kHz). Although a decrease in permittivity on the addition of PMMA was inevitable, the dielectric loss at high temperature was significantly reduced. For pristine PVDF and PVDF/PMMA (94/6), dielectric loss increased rapidly when heated to above 60°C. With further addition of PMMA, the dielectric loss of the blends remained stable and low at elevated temperature. Such reduction certified the increase in rigidity by in situ polymerization of PMMA and was essential for the stabilization of energy storage performance upon heating.
FIGURE 6. Temperature dependence of dielectric permittivity for PVDF/PMMA with PMMA contents of (A) 0%, (B) 6%, (C) 19.5%, (D) 24.7%, (E) 39%, (F) 56.8%, (G) 62.1%, (H) 74.3%, and (I) 100%.
The energy storage performance of the blends from 30 to 90°C is shown in Figures 7A–D, whereas variations in maximum energy density and efficiency with temperature are compared in Figures 7E,F. With an increase in the PMMA content, in the same electric field, energy density decreased slightly due to decrease in polarization. However, in order to enhance the temperature stability of energy storage, it is more important to maintain stable breakdown strength and energy loss at elevated temperature. The blends with light PMMA (0–24%) showed an intense reduction in efficiency with an increase in the electric field and temperature, which could serve the energy dissipation and deteriorate the energy storage performance. As anticipated, efficiency remained stable with further increase in the PMMA content at elevated temperature and electric field. Consequently, the energy density of the blends with the PMMA ratio of 39–62% sustained around 8 J/cm3, and the efficiency of around 70–83% could be sustained within the testing temperature range.
FIGURE 7. Energy densities and efficiencies of PVDF/PMMA blends at (A) 30°C, (B) 50°C, (C) 70°C, and (D) 90°C and the comparison of variations of (E) maximum energy density and (F) efficiency with temperature.
It is noteworthy that the energy storage performance at elevated temperature was outstanding, compared with other dielectric polymers, as shown in Table 1. This verified the validity of our proposed in situ polymerization strategy for the blends.
TABLE 1. Comparison of energy densities of the blend prepared in this study with other dielectric polymers between 70 and 90°C.
In conclusion, an in situ polymerization blending strategy for the PVDF/PMMA blends was proposed with a view to improve the temperature stability of energy storage performance and unveil its influence on the structure and dielectric properties upon heating. Through in situ polymerization of high-Tg PMMA in the PVDF matrix, the blends displayed the ultra-homogenous distribution of the two components, leading to a robust amorphous structure upon heating, as characterized by SAXS. Accordingly, the energy density and efficiency were remarkably enhanced between 30 and 90°C. At elevated temperature, the energy density and efficiency of the blends with 39–62% PMMA were ∼8 J/cm3 and around 70–83%, respectively, which outperformed most relevant dielectric polymers at an elevated temperature. This work provides a general approach to synthesizing polar polymers with high energy density and robust temperature stability.
The original contributions presented in the study are included in the article/Supplementary Material, further inquiries can be directed to the corresponding authors.
YL: formal analysis and writing—original draft. ZL: investigation, visualization, and writing—original draft. JG: conceptualization, writing—review, and editing. MW: fabrication. XL: methodology, writing—review and editing. YH: characterization, review and editing. YL: review and editing. LZ: supervision.
The authors warmly acknowledge the support from the National Key R&D Program of China (Grant No. 2020YFA0710500), the Fundamental Research Funds of Xian Jiaotong University (Grant No. xzy012021022), the Fundamental Research Funds for the Central Universities of China (Grant No. xtr042019002), the Natural Science Foundation of Ordos (Grant No. 2021EEDSCXQDFZ014), and the Natural Science Basic Research Program of Shaanxi Province (No. 2021JQ-042).
The authors declare that the research was conducted in the absence of any commercial or financial relationships that could be construed as a potential conflict of interest.
All claims expressed in this article are solely those of the authors and do not necessarily represent those of their affiliated organizations, or those of the publisher, the editors, and the reviewers. Any product that may be evaluated in this article, or claim that may be made by its manufacturer, is not guaranteed or endorsed by the publisher.
The authors are thankful to Yang Feng for kindly helping with polymerization and the assistance from Chang Huang and Chao Feng at the Instrumental Analysis Center of Xi’an Jiaotong University for SAXS and NMR measurements.
The Supplementary Material for this article can be found online at: https://www.frontiersin.org/articles/10.3389/fchem.2022.902487/full#supplementary-material
Baer, E., and Zhu, L. (2017). 50th Anniversary Perspective: Dielectric Phenomena in Polymers and Multilayered Dielectric Films. Macromolecules 50, 2239–2256. doi:10.1021/acs.macromol.6b02669
Botelho, G., Lanceros-Mendez, S., Gonçalves, A. M., Sencadas, V., and Rocha, J. G. (2008). Relationship between Processing Conditions, Defects and Thermal Degradation of Poly(vinylidene Fluoride) in the β-phase. J. Non-Crystalline Solids 354, 72–78. doi:10.1016/j.jnoncrysol.2007.07.012
Cai, X., Lei, T., Sun, D., and Lin, L. (2017). A Critical Analysis of the α, β and γ Phases in Poly(vinylidene Fluoride) Using FTIR. RSC Adv. 7, 15382–15389. doi:10.1039/C7RA01267E
Chu, B., Zhou, X., Ren, K., Neese, B., Lin, M., Wang, Q., et al. (2006). A Dielectric Polymer with High Electric Energy Density and Fast Discharge Speed. Science 313, 334–336. doi:10.1126/science.1127798
Dong, W., Hakukawa, H., Yamahira, N., Li, Y., and Horiuchi, S. (2019). Mechanism of Reactive Compatibilization of PLLA/PVDF Blends Investigated by Scanning Transmission Electron Microscopy with Energy-Dispersive X-Ray Spectrometry and Electron Energy Loss Spectroscopy. ACS Appl. Polym. Mat. 1, 815–824. doi:10.1021/acsapm.9b00061
Fatnassi, M., Ben Cheikh Larbi, F., and Halary, J. L. (2010). Quantitative Analysis of Semicrystalline Blends SAXS Data: Theoretical Modeling versus Linear Correlation Function. Int. J. Polym. Sci. 2010, 1–6. doi:10.1155/2010/829752
Gumyusenge, A., Tran, D. T., Luo, X., Pitch, G. M., Zhao, Y., Jenkins, K. A., et al. (2018). Semiconducting Polymer Blends that Exhibit Stable Charge Transport at High Temperatures. Science 362, 1131–1134. doi:10.1126/science.aau0759
Hirata, T., Kashiwagi, T., and Brown, J. E. (1985). Thermal and Oxidative Degradation of Poly(methyl Methacrylate): Weight Loss Macromolecules, 18, 1410–1418. doi:10.1021/ma00149a010
Hu, J., and Tashiro, K. (2016). Relation between Higher-Order Structure and Crystalline Phase Transition of Oriented Isotactic Polybutene-1 Investigated by Temperature-dependent Time-Resolved Simultaneous WAXD/SAXS Measurements. Polymer 90, 165–177. doi:10.1016/j.polymer.2016.03.004
Huang, H., Chen, X., Yin, K., Treufeld, I., Schuele, D. E., Ponting, M., et al. (2018). Reduction of Ionic Conduction Loss in Multilayer Dielectric Films by Immobilizing Impurity Ions in High Glass Transition Temperature Polymer Layers. ACS Appl. Energy Mat. 1, 775–782. doi:10.1021/acsaem.7b00211
Hussein, M. A., Albeladi, H. K., El-Shishtawy, R. M., and Osman, O. I. (2018). Cross-linked PMMA-Based Bifunctional Amino Derivatives. J. Therm. Anal. Calorim. 134, 1715–1728. doi:10.1007/s10973-018-7764-z
Hussein, M. A., El-Shishtawy, R. M., Abu-Zied, B. M., and Asiri, A. M. (2016). The Impact of Cross-Linking Degree on the Thermal and Texture Behavior of Poly(methyl Methacrylate). J. Therm. Anal. Calorim. 124, 709–717. doi:10.1007/s10973-016-5240-1
Kang, S. J., Park, Y. J., Bae, I., Kim, K. J., Kim, H.-C., Bauer, S., et al. (2009). Printable Ferroelectric PVDF/PMMA Blend Films with Ultralow Roughness for Low Voltage Non-volatile Polymer Memory. Adv. Funct. Mat. 19, 2812–2818. doi:10.1002/adfm.200900589
Khanchaitit, P., Han, K., Gadinski, M. R., Li, Q., and Wang, Q. (2013). Ferroelectric Polymer Networks with High Energy Density and Improved Discharged Efficiency for Dielectric Energy Storage. Nat. Commun. 4, 1–7. doi:10.1038/ncomms3845
Li, Q., Chen, L., Gadinski, M. R., Zhang, S., Zhang, G., Li, H. U., et al. (2015). Flexible High-Temperature Dielectric Materials from Polymer Nanocomposites. Nature 523, 576–579. doi:10.1038/nature14647
Li, H., Gadinski, M. R., Huang, Y., Ren, L., Zhou, Y., Ai, D., et al. (2020). Crosslinked Fluoropolymers Exhibiting Superior High-Temperature Energy Density and Charge-Discharge Efficiency. Energy Environ. Sci. 13, 1279–1286. doi:10.1039/C9EE03603B
Li, H., Xie, Z., Liu, L., Peng, Z., Ding, Q., Ren, L., et al. (2019). High-performance Insulation Materials from Poly(ether Imide)/boron Nitride Nanosheets with Enhanced DC Breakdown Strength and Thermal Stability. IEEE Trans. Dielect. Electr. Insul. 26, 722–729. doi:10.1109/TDEI.2018.00763710.1109/tdei.2019.8726017
Li, H., Zhou, Y., Liu, Y., Li, L., Liu, Y., and Wang, Q. (2021). Dielectric Polymers for High-Temperature Capacitive Energy Storage. Chem. Soc. Rev. 50, 6369–6400. doi:10.1039/D0CS00765J
Li, Q., Liu, F., Yang, T., Gadinski, M. R., Zhang, G., Chen, L.-Q., et al. (2016). Sandwich-structured Polymer Nanocomposites with High Energy Density and Great Charge-Discharge Efficiency at Elevated Temperatures. Proc. Natl. Acad. Sci. U.S.A. 113, 9995–10000. doi:10.1073/pnas.1603792113
Liu, F., Li, Q., Li, Z., Liu, Y., Dong, L., Xiong, C., et al. (2017). Poly(methyl Methacrylate)/boron Nitride Nanocomposites with Enhanced Energy Density as High Temperature Dielectrics. Compos. Sci. Technol. 142, 139–144. doi:10.1016/j.compscitech.2017.02.006
Liu, X.-J., Zheng, M.-S., Chen, G., Dang, Z.-M., and Zha, J.-W. (2022). High-temperature Polyimide Dielectric Materials for Energy Storage: Theory, Design, Preparation and Properties. Energy Environ. Sci. 15, 56–81. doi:10.1039/D1EE03186D
Liu, Y., Gao, J., Wang, Y., Zhou, J., Cao, L., He, Z., et al. (2019). Enhanced Temperature Stability of High Energy Density Ferroelectric Polymer Blends: The Spatial Confinement Effect. Macromol. Rapid Commun. 40, 1900406. doi:10.1002/marc.201900406
Long, H., Jing, W., Zhong, Y., Kongjun, Z., and Deng, C. (2022). Dielectric and Energy Storage Properties of PVDF/Nd-BaTiO3@Al2O3 Composite Films | Functional Materials Letters. Available at: https://www.worldscientific.com/doi/abs/10.1142/S1793604719500346 (accessed March 19, 2022).
Mccord, E. F., Anton, W. L., Wilczek, L., Ittel, S. D., Nelson, L. T. J., Raffell, K. D., et al. (1994). 1 H and 13 C NMR of PMMA Macromonomers and Oligomers - End Groups and Tacticity. Macromol. Symp 47–64. doi:10.1002/masy.19940860106
Nguyen, T. (1985). Degradation of Poly[vinyl Fluoride] and Poly[vinylidene Fluoride]. J. Macromol. Sci. Part C 252, 227–275. doi:10.1080/15583728509412823
Prateek, , Thakur, V. K., and Gupta, R. K. (2016). Recent Progress on Ferroelectric Polymer-Based Nanocomposites for High Energy Density Capacitors: Synthesis, Dielectric Properties, and Future Aspects. Chem. Rev. 116, 4260–4317. doi:10.1021/acs.chemrev.5b00495
Rajib, M., Arif Ishtiaque Shuvo, M., Karim, H., Delfin, D., Afrin, S., and Lin, Y. (2015). Temperature Influence on Dielectric Energy Storage of Nanocomposites. Ceram. Int. 41, 1807–1813. doi:10.1016/j.ceramint.2014.09.127
Tan, D. Q. (2020). Review of Polymer‐Based Nanodielectric Exploration and Film Scale‐Up for Advanced Capacitors. Adv. Funct. Mat. 30, 1808567. doi:10.1002/adfm.201808567
Wang, Y., Li, Z., Wu, C., and Cao, Y. (2020). High-temperature Dielectric Polymer Nanocomposites with Interposed Montmorillonite Nanosheets, Chem. Eng. J. 401, 126093. doi:10.1016/j.cej.2020.126093
Wei, J., Zhang, Z., Tseng, J.-K., Treufeld, I., Liu, X., Litt, M. H., et al. (2015). Achieving High Dielectric Constant and Low Loss Property in a Dipolar Glass Polymer Containing Strongly Dipolar and Small-Sized Sulfone Groups. ACS Appl. Mat. Interfaces 7, 5248–5257. doi:10.1021/am508488w
Wen, F., Zhang, L., Wang, P., Li, L., Chen, J., Chen, C., et al. (2020). A High-Temperature Dielectric Polymer Poly(acrylonitrile Butadiene Styrene) with Enhanced Energy Density and Efficiency Due to a Cyano Group. J. Mat. Chem. A 8, 15122–15129. doi:10.1039/D0TA03540H
Wu, X., Chen, X., Zhang, Q. M., and Tan, D. Q. (2022). Advanced Dielectric Polymers for Energy Storage. Energy Storage Mater. 44, 29–47. doi:10.1016/j.ensm.2021.10.010
Wunderlich, B. (2003). Reversible Crystallization and the Rigid-Amorphous Phase in Semicrystalline Macromolecules. Prog. Polym. Sci. 28, 383–450. doi:10.1016/S0079-6700(02)00085-0
Yin, K., Zhang, J., Li, Z., Feng, J., Zhang, C., Chen, X., et al. (2019). Polymer Multilayer Films for High Temperature Capacitor Application. J. Appl. Polym. Sci. 136, 47535. doi:10.1002/app.47535
Zha, J.-W., Zheng, M.-S., Fan, B.-H., and Dang, Z.-M. (2021). Polymer-based Dielectrics with High Permittivity for Electric Energy Storage: A Review. Nano Energy 89, 106438. doi:10.1016/j.nanoen.2021.106438
Zhang, Q., Chen, X., Zhang, B., Zhang, T., Lu, W., Chen, Z., et al. (2021). High-temperature Polymers with Record-High Breakdown Strength Enabled by Rationally Designed Chain-Packing Behavior in Blends. Matter 4, 2448–2459. doi:10.1016/j.matt.2021.04.026
Zhang, X., Yifei, Z., Zhicheng, Z., and Yuhao, W. (2017). Poly(tetrafluoroethylene-hexafluoropropylene) Films with High Energy Density and Low Loss for High-Temperature Pulse Capacitors. Polymer 114, 311. doi:10.1016/j.polymer.2017.03.022
Zheng, M.-S., Zheng, Y.-T., Zha, J.-W., Yang, Y., Han, P., Wen, Y.-Q., et al. (2018). Improved Dielectric, Tensile and Energy Storage Properties of Surface Rubberized BaTiO3/polypropylene Nanocomposites. Nano Energy 48, 144–151. doi:10.1016/j.nanoen.2018.03.049
Zhou, L., Zhou, Y., Shi, Y., Chen, T., Zou, T., Zhou, D., et al. (2020). Enhancing Thermal Stability of P(VDF-HFP) Based Nanocomposites with Core-Shell Fillers for Energy Storage Applications. Compos. Sci. Technol. 186, 107934. doi:10.1016/j.compscitech.2019.107934
Keywords: energy density, energy storage dielectrics, temperature stability, in situ polymerization, polyvinylidene fluoride
Citation: Liu Y, Liu Z, Gao J, Wu M, Lou X, Hu Y, Li Y and Zhong L (2022) High Energy Density and Temperature Stability in PVDF/PMMA via In Situ Polymerization Blending. Front. Chem. 10:902487. doi: 10.3389/fchem.2022.902487
Received: 23 March 2022; Accepted: 19 April 2022;
Published: 19 May 2022.
Edited by:
Jun-Wei Zha, University of Science and Technology Beijing, ChinaReviewed by:
Tiandong Zhang, Harbin University of Science and Technology, ChinaCopyright © 2022 Liu, Liu, Gao, Wu, Lou, Hu, Li and Zhong. This is an open-access article distributed under the terms of the Creative Commons Attribution License (CC BY). The use, distribution or reproduction in other forums is permitted, provided the original author(s) and the copyright owner(s) are credited and that the original publication in this journal is cited, in accordance with accepted academic practice. No use, distribution or reproduction is permitted which does not comply with these terms.
*Correspondence: Jinghui Gao, Z2FvamluZ2h1aUB4anR1LmVkdS5jbg==; Lisheng Zhong, bHN6aG9uZ0B4anR1LmVkdS5jbg==
†These authors have contributed equally to this work
Disclaimer: All claims expressed in this article are solely those of the authors and do not necessarily represent those of their affiliated organizations, or those of the publisher, the editors and the reviewers. Any product that may be evaluated in this article or claim that may be made by its manufacturer is not guaranteed or endorsed by the publisher.
Research integrity at Frontiers
Learn more about the work of our research integrity team to safeguard the quality of each article we publish.