- 1School of Life and Environmental Science, Wenzhou University, Wenzhou, China
- 2State and Local Joint Engineering Research Center for Ecological Treatment Technology of Urban Water Pollution, Wenzhou, China
- 3Zhejiang Provincial Key Lab for Water Environment and Marine Biological Resources Protection, Wenzhou, China
- 4School of Environmental Science and Engineering, Shanghai Jiao Tong University, Shanghai, China
Surfactants, especially non-ionic surfactants, play an important role in the preparation of nanocarriers and can also promote the enzymatic hydrolysis of lignocellulose. A broad overview of the current status of surfactants on the immobilization of cellulase is provided in this review. In addition, the restricting factors in cellulase immobilization in the complex multiphase hydrolysis system are discussed, including the carrier structure characteristics, solid-solid contact obstacles, external diffusion resistance, limited recycling frequency, and nonproductive combination of enzyme active centers. Furthermore, promising prospects of cellulase-oriented immobilization are proposed, including the hydrophilic-hydrophobic interaction of surfactants and cellulase in the oil-water reaction system, the reversed micelle system of surfactants, and the possible oriented immobilization mechanism.
Introduction
Bioethanol, as a renewable, economically affordable, and environmentally safe energy material, will gradually become a substitute for fossil fuels. It has far-reaching research significance and application value for the development of a sustainable energy strategy (Karimi et al., 2021; Zeng et al., 2021; Ziaei-Rad et al., 2021; Suhartini et al., 2022). Due to competition with food supply in the first generation of bioethanol production, lignocellulose, a non-starch material, has become an important raw material for bioethanol production (Alonso et al., 2019; Maia et al., 2020; Winarni et al., 2020). Adsorption of cellulases onto lignin has been considered as the major factor in retarding enzymatic cellulose degradation of lignocellulosic biomass (Djajadi et al., 2018). Hydrophobic interaction, electrostatic interaction and hydrogen bonding have been regarded as the cause of the nonproductive binding of cellulases to lignin (Djajadi et al., 2018; Li et al., 2020; Song et al., 2020). A natural “biodegradable barrier” of lignin cell walls which are connected in a strong, yet resilient network under the action of covalent and non-covalent bonds render the cellulose inaccessible (Mnich et al., 2020; Chu et al., 2021). Therefore, to reduce the recalcitrance of lignocellulosic biomass to biochemical degradation, pretreatment methods have been developed to break down the lignin-hemicellulose-cellulose matrix and increase the enzyme accessibility of the cellulose scaffold (Jiang et al., 2017a; Jia et al., 2018; Rocha-Martin et al., 2018).
In general, lignin-derived inhibition is the major physical obstacle restricting the enzymatic hydrolysis of cell wall polysaccharides (Leonidas et al., 2019; Zheng et al., 2021). More importantly, the non-specific binding of free cellulase on lignocellulosic substrates may account for the low rate of hydrolysis at the action mechanism level during enzymatic hydrolysis. Some enzymes remain free after the enzymatic hydrolysis of lignocellulosic substrates, while non-specific binding to the residual substrates also prevents the efficient recycling of cellulase (Rahikainen et al., 2011; Kellock et al., 2017; Bhawna et al., 2020). Moreover, the utility of cellulases has been limited due to their low operational stability, high costs, and poor reutilization when used in the native form (Yang et al., 2017).
To overcome these barriers, immobilization is usually used to improve enzyme stability and even activity or selectivity when properly designed, which can also facilitate the reuse of enzymes and effective cost of catalytic processes (Mita and Eldin, 2014; Li et al., 2016; Mehta et al., 2016; Xu et al., 2016; Zhang et al., 2016). The characteristics of various immobilization methods of enzymes is summarized in Table 1. Cellulases represent a large group of enzymes from various organism and with different substrate specificity, biophysical properties, etc. The immobilization behavior is different depending on the enzyme or enzyme mixture investigated. During the immobilization process of cellulase, the structure and properties of carrier materials have significant effects on the performance of the immobilized enzyme (Kalantari et al., 2013; Li et al., 2018). The size of the carriers plays an important role in determining the activity of the immobilized enzyme owing to the inverse relationship between the carrier size and enzyme loading. Thus, large carrier size decreases enzyme activity in general (Valencia et al., 2010), and a reduction in the size of the carriers results in a higher surface area for enzyme binding (Malar et al., 2018; Malar et al., 2020). For the immobilization of cellulase, the smaller size of the surface pore should be kept lower than that of the cellulase macromolecule (6–20 nm), which can further reduce the internal and external diffusion resistance in the heterogeneous system (DiCosimo et al., 2013; Santos et al., 2015). Therefore, nanocarriers are widely used in the immobilization of enzymes because of their unique properties, such as large specific surface area to volume ratio (Cao et al., 2016; Roth et al., 2016; Malar et al., 2020).
Moreover, the immobilization of cellulase has been achieved based on physical adsorption, covalent binding, or affinity interactions (Zang et al., 2014; Hosseini et al., 2018; Zhang and Hay, 2019), including carrier-binding, microemulsion-based organo-gels (MBGs), ultrasonic encapsulation, crosslinking, entrapment, glutathione-labeling, and chelation (Mroczkiewicz et al., 2012; Nicoletti et al., 2015). However, enzymes often display drastically lower activity in organic solvents than in water, and the water layer on the molecular surface of enzymes determines their activity in organic media (Zhang et al., 2012). Therefore, among several approaches to resolve the challenges, one of the most effective methods is immobilization of the enzymes within an aqueous microenvironment in the organic solvents. Microemulsions formed by amphiphilic surfactants have been widely reviewed as effective media for the immobilization of enzymes in hydrophobic solvents (Itabaiana et al., 2014; Rajnish et al., 2021; Savic et al., 2021). The MBGs method based on microemulsions has been used to form matrices for enzyme immobilization to achieve enzymatic catalysis in nonconventional medium as they appear to be rigid and stable for a long time, even within the reaction solution (Zhang et al., 2012). Therefore, the MBGs method has unique advantages of improving the chemical stability of immobilized enzymes and maintaining high catalytic activity (Pavlidis et al., 2010; Itabaiana et al., 2014). It is clearly that the surfactants play an important role in the preparation of nanomaterials (Lou et al., 2017; Bao et al., 2019; Ortiz-Martínez et al., 2019; Alexander et al., 2020).
The surfactants have been widely used for the preparation of nanocarriers as shown in Table 2, forming the nano-template by micelles and emulsions of surfactants is a common method that can greatly reduce the surface tension of the solvent and change the interface composition and structure (Carter and Puig-Sellart, 2016; Bao et al., 2019). Desirable nanostructured materials can be produced because of the special nanoreactors formed by surfactant micelles and the oriented alignment characteristics of surfactants in solution, such as the Langmuir-Blodgett (LB) membranes and liposomes (Lok Kumar et al., 2014; Gutierrez et al., 2016). Furthermore, the non-ionic surfactants can significantly enhance cellulose hydrolysis, thus reducing enzyme loading (Lou et al., 2017; Bao et al., 2019). However, inhibitory effects have been observed with the addition of amphoteric, anionic, and cationic surfactants (Lou et al., 2017; Bao et al., 2019). Moreover, the loss of enzyme activity during immobilization is a notable problem; the structural distortion caused by the strong enzyme-support interactions may produce steric hindrances and catalytic cleft blockage (Carlsson et al., 2014; Suárez et al., 2018). Although a large dose of original cellulase is added for a higher load of immobilized enzyme to improve the activities of the immobilized enzyme, no significant improvement in enzymatic activity has been observed due to the random and inhomogeneous combination of the nanocarriers and cellulase molecules (Nakayama et al., 2009). Oriented immobilization, as a specific binding method, can effectively prevent the nonproductive combination of enzymes and nanocarriers, which further improves the immobilization and hydrolysis efficiency. The reversed micelles formed by surfactants have been successfully used in the preparation of oriented-immobilized lipase when their concentration exceeds the critical micelle concentration (CMC) (Fan et al., 2016). To date, few studies have reported the oriented immobilization of cellulase. Therefore, this review mainly focuses on the important roles of surfactants in the immobilization of cellulase, mainly including the preparation of nanocarriers and cellulase hydrolysis. Moreover, a novel insight into the oriented immobilization of cellulase in a surfactant reversed micelle (SRM) system was discussed and found to have promising prospects.
Effects of Surfactants on Nanocarriers
Preparation of Nanocarriers Based on Surfactants
The basic physical and chemical properties of surfactants, such as micelle formation, dispersing, emulsifying, and solubilizing, have made them widely useful in the field of nanotechnology (Yang et al., 2017). Several ordered aggregations formed by the surfactants are used as nano-templates for the preparation of nanocarriers, such as micelles and reversed micelles. The process can greatly reduce the surface tension of the solvent and change the interface composition and structure (Bao et al., 2019). For the preparation of nanocarriers, surfactant micelles are the microreactors of nanocarriers during the preparation process, and the morphology of microreactors is controllable because of the amphiphilic characteristics of surfactants, which have been used for the preparation of desirable nanostructured carriers (Yiamsawas et al., 2017). For instance, hydrophilic surfactants are often used for the preparation of spherical nanocarriers because of their dispersibility in water (Luan and Ramos, 2010). Similarly, the reversed micelles of surfactants can effectively define the particle size and reaction microenvironment in the water, providing a nanoscale reaction space. It has been widely used because the aggregates self-assembled by surfactant molecules can be used to synthesize ordered mesoporous materials with a simpler operation and more uniform channel distribution (Bao et al., 2017; Bao et al., 2019).
Surface Modification of Nanocarriers in the Surfactant System
Surfactants can also change the surface properties of nanocarriers, such as their morphology, magnetic properties, dispersion, and catalytic performances (Asghar et al., 2016; Wei et al., 2018; Lopes et al., 2019; Alexander et al., 2020). This modification may result in a new structure with new surface activity due to the combination of hydrophilic groups of surfactants and surface groups of nanocarriers. For example, the use of surfactants of decylamine and cetyltrimethylammonium bromide can provide an easy and effective way to change the functionality of cellulose nanocrystals with a hydrophobic polylactic acid matrix and to evaluate the effects of surface chemistry on the reinforcement mechanisms (Orellana et al., 2018). Meanwhile, the presence of surfactants can make nanocarriers more difficult to re-agglomerate by reducing the surface energy and form a steric hindrance effect (Wang M. et al., 2013; Tan et al., 2019), the surfactants are coated on the surface of the nanocarriers to form a space barrier layer, the hydrophilic group faces outward and the hydrophobic group faces inward, so that the agglomeration of the particles is avoided.
Effects of Nanocarriers on Immobilization of Cellulase
The structure and properties of carrier materials have great influence on the properties of immobilized cellulase, such as internal geometry (e.g., flat surfaces or thin fibers), specific surface area, superficial activation degree, mechanical resistance, and pore diameter (Santos et al., 2015; Begum et al., 2019; Malar et al., 2020). Meanwhile, partitioning and mass transport limitations may yield spatial variations in local reaction rates in porous materials (Neira and Herr, 2017). Therefore, to improve the stability and catalytic activity of immobilized cellulase, various materials, such as chitin, chitosan, nylon, and polyvinyl alcohol, have been widely used as carriers (Cherian et al., 2015; Priydarshani et al., 2018).
The physical effects of nanocarriers on immobilized cellulase are as follows: 1) The pore size and effective surface area of the nanocarriers. Not all porous carriers can be used for immobilization of cellulase due to the limitation of pore size, which should be larger than or equal to that of the cellulase to reduce steric hindrance. The effective surface area occupied by the enzyme determines the maximum load of the immobilized cellulase (Santos et al., 2015). When a stable surface area is maintained, the amount of immobilized or absorbed cellulases is related to the pore size because the pore diameter determines the size of the protein that can be immobilized on that carrier (Teresa et al., 2009; Webster et al., 2015); 2) the number of carrier-bound active groups (CAGs) is another key factor controlling the enzyme-carrier multi-interaction (Cristina et al., 2011; Santos et al., 2015); 3) the size of carriers plays a very important role in the preparation of immobilized cellulase, in that a smaller carrier size with larger specific surface area will be better for the cellulase immobilization load, and the higher surface porosity of the carriers providing numerous binding sites for cellulase is one of the most important factors influencing the activity of immobilized cellulase (Chen et al., 2010; Santos et al., 2015; Malar et al., 2020); 4) the mechanical properties of the carriers need to be controlled considering the final configuration of the reactor. If the reactor is a fixed-bed reactor, such as inorganic supports like porous glass, silicates, it should possess very high rigidity to withstand high pressures without pressure problems, but the situation is different if a stirred-tank reactor is used (Cristina et al., 2011; Santos et al., 2015); 5) after the cellulase penetrates the carriers, the internal morphology of carriers will determine the possibility of obtaining a very intense or very limited enzyme-carrier interaction (Santos et al., 2015). When the diameter of the carriers is smaller than that of the enzyme, it is difficult to obtain an intense enzyme-carrier interaction (Cristina et al., 2011), but if the carriers have sufficiently large internal surfaces, it is possible to get an intense interaction with a similar flat surface (e.g., agarose beads, porous glass, or silicates) (Malar et al., 2018).
In particular, the special superparamagnetism of magnetic nanocarriers has attracted increasing interest as they allow easy recycling and separation of catalysts and biomolecules from high-viscosity liqueurs and high-solid-content broths. This unique characteristic has been well-applied to immobilization of cellulase, and a better hydrolysis efficiency and recycling feasibility have been observed (Alftrén et al., 2014; Cao et al., 2016; Cipolatti et al., 2014; Xing et al., 2015). During immobilization of cellulase, magnetic chitosan microspheres (C-MNPs) are frequently used as carriers because of their significant biological (i.e., biodegradable, biocompatible, bioactive) and chemical properties (polycationic, hydrogel, contains reactive groups, such as -OH and -NH2). Moreover, the hydrophilic properties of the C-MNPs play an important role in the preparation of oriented-immobilized cellulase based on the SRM system. The conventional immobilization of cellulase molecules on a single magnetic nanocarrier is simple, the chitosan was usually first coated on the magnetic nanocarriers for further combination with cellulase (Figure 1). Subsequently, the combined material based on Fe3O4 nanocarriers have received extensive attention in cellulase immobilization to improve enzyme activity, loading, and stability because of their low toxicity, biocompatibility, and easy synthesis (Jordan et al., 2011). Magnetite nanocarriers coated with silica and modified by organic-silanes, biocompatible, and with hydrophilic properties, are promising for cellulase immobilization.
The binding sites of enzymes on the surfaces of carriers depend on the chemical properties of the carriers. For non-covalent immobilization, the chemical structure of the skeleton and surface determines the applicability of carriers. The functional groups play a key role in the activity, stability, and selectivity of the enzyme, and the size, charge, polarity, and hydrophilicity/hydrophobicity of groups can affect their binding functions (Watanabe et al., 2010). Different properties of the ionic groups on the surfaces of carriers may result in different enzyme activities and further determine the structure of immobilized cellulase (Santos et al., 2015; Berlin et al., 2016; Frančič et al., 2016; Hui et al., 2016; Zhou et al., 2018). The chemistry properties of enzyme and carrier cause the oriented distribution of catalytic domain of enzyme from dispersion layer to diffusion layer during the immobilization process is shown in Figure 2. In this process, the CAGs directly participate in binding with enzyme molecules, but the carrier-bound inert groups (CIGs) are not directly involved. This interaction inevitably disturbs the maintenance of the natural conformation of the enzyme, leading to structural and functional changes in the enzyme molecules. No obvious stability change has been observed when the newly formed conformation is similar to that of the natural enzyme.
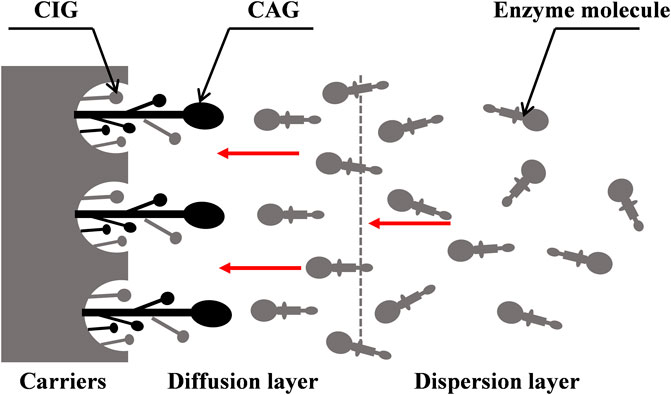
FIGURE 2. Binding schematic diagram of enzyme and carrier caused by the chemistry properties during the immobilization process. CIGs means the carrier-bound inert groups and CAGs means the carrier-bound active groups; The larger end of enzyme molecule stands for the catalytic domain and the other end stands for the adsorption domain.
The covalent binding between carriers and catalytic cleft of the enzyme not only causes pore plugging of the surface, but also leads to the drag increment of in-diffusion. Although an initial high dosage of cellulase is added, the inhomogeneous distribution of the carrier surface structure results in the uncontrollable immobilization sites, and ineffective immobilization may lead to a significant loss of enzymatic activity and reduce the accessibility of the substrate to the functional sites. Moreover, the partition and mass transport limitations of nanocarriers may cause spatial variation in local reaction rates and further affect enzymatic hydrolysis (Du et al., 2017; Zeng et al., 2019). The chitosan molecules are mostly used because of the large number of -OH and amino groups (-NH3), which are easier to co-precipitate with cellulase (Bindhu and Abraham, 2010; Urrutia et al., 2018; Saha et al., 2019; Mo et al., 2020). Moreover, surface modification is an important strategy for tuning the properties of nanocarriers. Surface modification can either alter the existing property or introduce new properties onto nanoparticles using various agents, such as organ siloxane, N-(3-dimethylaminopropyl)-N′-ethylcarbodiimide hydrochloride (EDC), and carbodiimide, as well as amino silanes, such as 3-aminopropyltriethoxysilane, aminoethyl aminopropyl polydimethylsiloxane, and silica (Chang et al., 2011; Gokhale et al., 2013; Riedel et al., 2017; Malar et al., 2018; Malar et al., 2020).
Roles of Surfactants on Cellulase Hydrolysis
In most reports about hydrophobic ionic liquids, the enzymes are not dissolved but merely in a dispersed state and therefore regarded as a heterogeneous catalyst. Some hydrophilic ionic liquids can accelerate the dissolution of enzyme molecules, but cause the destruction of the protein secondary structure, leading to the inactivation of the enzyme (Fujita and Ohno, 2010; Moniruzzaman et al., 2010). In pure hydrophilic ionic liquids the enzymes can be dispersed at the monomolecular level. The hydrophilic proteins in almost anhydrous nonpolar solvents form suspensions, whereas proteins with extended hydrophobic surface segments form microemulsions in the same media, greatly reducing the catalytic efficiency of the enzyme (Zuev et al., 2003; Predvoditelev et al., 2010). Nonpolar hydrophobic solvents, such as heptane, octane, and benzene, do not cause the dehydration of biocatalysts. Therefore, the enzyme can maintain its catalytic activity (Muginova et al., 2010). Similarly, the catalytic activity of enzymes can be retained in the surfactant micelle system due to the water-oil amphiphilicity of surfactants (Muginova et al., 2010). Non-ionic surfactants can significantly accelerate the enzymatic hydrolysis of lignocellulose (Qing et al., 2010; Seo et al., 2011b; Eckard et al., 2012; Yiamsawas et al., 2017). For instance, Tween-20 can enhance the specific adsorption of cellulase, and the conversion efficiency of cellulose increased from 9 to 21% within 72 h when a high lignocellulosic substrate was added (Seo et al., 2011a). The prevention of non-productive enzyme adsorption onto lignin is the most widely investigated mechanism for this enhancement (Sipos et al., 2010; Lou et al., 2017). Recently, Djajadi et al. (2018) has proved that the adsorption of cellulases onto lignin substrates is reversible by nature, the reversible adsorption-desorption is existing in the process. But the non-productive adsorption caused by the ineffective combination will occupy large number of catalytic clefts of enzyme molecule which greatly hinder the enzymatic hydrolysis. Non-ionic surfactants can render lignin surfaces more hydrophilic by increasing their polar surface energy component, which can reduce the non-productive adsorption of cellulases onto lignocellulosic substrates caused by the ineffective combination between catalytic clefts of enzymes and lignin substrates (Jiang et al., 2017b), thereby promoting the enzymatic hydrolysis of lignocellulose. However, for the anionic surfactant-cellulase system, the adsorbed surfactants on the surface of cellulase cause a lower negative charge area, which further leads to negative catalytic activity due to the presence of sulfonic acid groups with a higher ionization degree (Yu and Zhang, 2016).
Furthermore, the effect of surfactants on cellulase hydrolysis is related to the concentration of surfactants (Zhou et al., 2015). In the enzymatic hydrolysis process, cellulose molecules are specifically adsorbed by the cellulose-binding domain (carbohydrate-binding module, CBM) and exert a driving force on the enzyme during the hydrolysis of cellulose (Liu et al., 2011; Tomme et al., 2015; Arslan et al., 2016). The adsorption of CBM can increase the cellulase concentration of the substrate surface by promoting the association of enzymes and substrates, but the non-covalent interactions (e.g., hydrogen bonds, electrostatic, and hydrophobic interactions) may lead to a nonproductive combination, because the random combination will occupy the active center of enzyme, resulting in the loss of catalytic activity. Ineffective adsorption can be reduced in the presence of surfactants due to the hydrophobic structure of surfactants, which can interact with the hydrophobic lignocellulosic substrates and form a coating (Kumar and Wyman, 2010; Li et al., 2012). However, contrasting results were obtained when different concentrations of surfactants were added to the enzymatic hydrolysis system. Some studies have suggested that a high concentration of surfactants can inhibit cellulase activity because strong hydrophobic interaction between the surfactant and cellulase can further reduce the effective adsorption of enzymes on cellulose (Wang Z. et al., 2013; Bao et al., 2019). However, the promotion effect of surfactant in enzymatic saccharification was observed in low concentration of lignosulfonate with low molecular weight and good sulfonation, which can be explained that the lignosulfonate can prevent the nonproductive binding of cellulase to lignin substrate, and the formed lignosulfonate-cellulase aggregate can also stabilize and enhance the binding of cellulase to lignin substrate (Lou et al., 2014).
The Oriented Immobilization of Cellulase in the SRM System
The oriented immobilization of proteins on a solid support can effectively avoid its denaturation and keep its catalytic clefts fully exposed to solution, thus maximally preserving the bioaffinity or bioactivity. Liu and Yu (2016) has summarized the recent advances in oriented immobilization of proteins with a particular focus on antibodies and enzymes. However, the orientated immobilization of enzymes at the solvent interface is never involved. Thereby, the follow-up content will propose a novel method to achieve the oriented immobilization of cellulase in the SRM system.
Construction of the SRM System
The SRM system has been widely used in the preparation of immobilized enzymes (Dong et al., 2010; Marhuendaegea et al., 2015). The special structure of surfactant molecules caused a water-oil amphipathy with a hydrophobic nonpolar hydrocarbon chain (alkyl) and a hydrophilic polar group (such as -OH, -COOH, -NH2, and -SO3H) distributed at different ends. In the water-oil (W/O) system, the surfactants are dissolved in the nonpolar organic solvent when a trace of water is provided, and the reversed micelles are formed when the concentration exceeds the CMC (Takagi et al., 2019; Chi et al., 2018). In reversed micelles, the nonpolar groups of the surfactants are exposed to the nonpolar organic solvents, while the polar groups are arranged inside. Therefore, a polar core with the ability to dissolve polar substances in the microreactors is formed. The SRMs are nanoscale aggregates that are formed spontaneously, and the W/O microemulsion with low water content provides a stable thermodynamic system (Tao et al., 2013). According to the hydrophilic-hydrophobic interaction of surfactants and cellulase in the oil-water reaction system, the large number of oil-water interfaces in the system provides a good environment for the catalytic reaction of enzyme molecules (Brady and Jordaan, 2009).
Mechanism of Oriented-Immobilized Cellulase in the SRM System
Multipoint covalent attachment is likely the most effective strategy for immobilization, but it is difficult to allow the immobilization of enzymes at a well-defined position since the proteins are usually attached to the solid surface by uncontrolled chemical bonds (Barbosa et al., 2015; Hernandez and Fernandez-Lafuente, 2011; Li et al., 2016). The uncontrolled conformational changes were caused by random immobilization, which may lead to a significant loss of enzyme activity, and the disordered enzyme orientation may also reduce the accessibility of the substrate to functional sites (Orellana et al., 2018; Steen Redeker et al., 2013; Yu et al., 2012). However, the hydrophilic cellulase will be dissolved in the SRM system due to the existence of surfactants, which can maintain the activity of the enzyme and prevent the toxic effects of organic solvents (Tao et al., 2013). The active centers of cellulase molecules are usually clefts, which provide a different microenvironment (Zhang et al., 2015) because the structures of cellulase active centers are mainly composed of eight kinds of amino acids (tryptophan, tyrosine, histidine, phenylalanine, aspartic acid, glutamic acid, and arginine). Aromatic amino acids and some polar amino acids appeared more frequently, such as tryptophan, tyrosine, histidine, aspartate, asparagine and arginine, most of which are hydrophobic tryptophan and phenylalanine residues, especially the tryptophan which has the highest content and plays an important role in the recognition and binding of enzyme molecules and substrate (Zhang et al., 2015). Hydrophobic active centers are conducive to the combination of catalyzed groups of cellulase and substrates. When the specific substrate is close to the active centers, a change in the conformation of the cellulase molecule can be induced so that the reaction groups of the enzyme active centers and substrate are aligned correctly. Meanwhile, the molecular orbitals between the reaction groups of the active centers are strictly located in the right direction for easier enzymatic reactions. Therefore, cellulase is distributed in the W/O interface, and the catalytic active center is toward the organic solvent and the other side toward the “pool”. Moreover, the addition of surfactants can enhance the aggregation effect of cellulase on the W/O interface, and the existence of a crosslinking agent promotes the covalent crosslinking of enzyme molecules. The catalytic activity centers of the cross-linked microspheres are distributed uniformly and toward the outside, which solves the challenge of the uncontrollable attachment sites of the cellulase molecules in the immobilization process (Li et al., 2016; Steen Redeker et al., 2013; Yu et al., 2012). In the SRM system, the hydrophobic active molecules are exposed to the outside, which is beneficial for the further combination of immobilized cellulase and lignocellulosic substrates. However, the immobilized sites of cellulase molecules remain stochastic and heterogeneous, which may lead to covalent binding between the carriers and the active center of the enzyme and further cause ineffective immobilization and enzymatic reactions (Li et al., 2016). Therefore, to achieve oriented immobilization and improve the recycling times of cellulase, C-MNPs can be used as carriers as shown in Figure 3. This method can effectively prevent the ineffectiveness of cellulase immobilization. In this process, glutaraldehyde is used as the crosslinking agent, and EDC and N-hydroxysuccinimide are the coupling agents (Figure 4). In the W/O system, the free carboxyl group (-COOH) in the adsorption zone of the cellulase molecules can realize covalent binding with a large number of amino terminal catalytic residues of chitosan molecules (Fan et al., 2016). The process cannot destroy the catalytic center of cellulase, and the exposed catalytic clefts increase the effective attachment of immobilized cellulase to solid substrates, which further promotes enzymatic hydrolysis. Therefore, the oriented immobilization of enzymes is obtained in the SRM system, which can prevent nonproductive combinations effectively and further promote enzymatic hydrolysis.
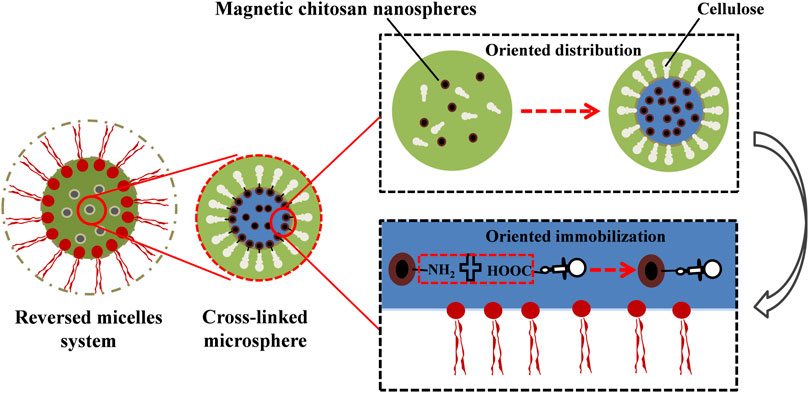
FIGURE 3. The oriented immobilization diagrammatic sketch of single-layer cellulase in the surfactant reversed micelles system, the “green” represents the internal “pool” of SRM system, “black” represents the magnetic chitosan microspheres (C-MNPs), “brown” represents the cross-linked microsphere.
Conclusion
Cellulase plays an important role in the production of fuel ethanol by the enzymatic hydrolysis of lignocellulose, and the immobilization of cellulase on the nanocarriers is an effective way to improve hydrolysis efficiency. However, the nanocarrier structure characteristics, solid-solid contact obstacles, external diffusion resistance, limited recycling frequency of nanocarriers, and nonproductive combination of enzyme active centers restricted the further improvement of hydrolysis efficiency in the complex multiphase system. Surfactants can promote the enzymatic hydrolysis of lignocellulose and play an important role in the preparation of nanocarriers. The special SRM system caused by the amphiphilicity in the oil-water reaction system can provide effective protection to obtain the immobilization of single-layer cellulase, which can effectively prevent the immobilization of cellulase and increase the effective attachment of immobilized cellulase and solid substrates.
Author Contributions
ZW: conceptualization, investigation, methodology, and writing (original draft preparation). CF: methodology and article revision. XZ: data collection and writing (review and editing). ZJ: methodology and investigation. KB: formal analysis and investigation. MZ: data collection, article revision and funding acquisition. HK: methodology, writing (review and editing) and supervision.
Funding
This work was supported by the major projects of study on National Social Science Foundation of China and the spirit explanation of the Fifth Plenary Session of the 19th CPC Central Committee (21ZDA028)“Construction of ecological friendly water environment management system in new rural areas of socialist modernization pilot area”. And this work was also supported by the Major Project of Science and Technology in Zhejiang Province (No. 2020C02010-002).
Conflicts of Interest
The authors declare that the research was conducted in the absence of any commercial or financial relationships that could be construed as a potential conflict of interest.
Publisher’s Note
All claims expressed in this article are solely those of the authors and do not necessarily represent those of their affiliated organizations, or those of the publisher, the editors and the reviewers. Any product that may be evaluated in this article, or claim that may be made by its manufacturer, is not guaranteed or endorsed by the publisher.
References
Al-Shemmari, F. H. J., Al-Mulla, E. A. J., and Rabah, A. A. (2014). A Comparative Study of Different Surfactants for Natural Rubber clay Nanocomposite Preparation. Rend. Fis. Acc. Lincei 25 (3), 409–413. doi:10.1007/s12210-014-0307-z
Alexander, K., Gajghate, S., Katarkar, A. S., Majumder, A., and Bhaumik, S. (2020). Role of Nanomaterials and Surfactants for the Preparation of Graphene Nanofluid: A Review. Mater. Today 44, 1136–1143. doi:10.1016/j.matpr.2020.11.231
Alftrén, J., Hobley, T. J., Prins, W., and Overend, R. (2014). Immobilization of Cellulase Mixtures on Magnetic Particles for Hydrolysis of Lignocellulose and Ease of Recycling. Biomass Bioenerg. 65 (3), 72–78. doi:10.1016/j.biombioe.2014.03.009
Alonso-Gómez, L. A., Heredia-Olea, E., Serna-Saldivar, S. O., and Bello-Pérez, L. A. (2019). Whole Unripe Plantain (Musa Paradisiaca L.) as Raw Material for Bioethanol Production. J. Sci. Food Agric. 99 (13), 5784–5791. doi:10.1002/jsfa.9847
Anjum, S., Shaheen, S., Awan, M. S., and Zia, R. (2019). Effect of Various Surfactants on Optical and Electrical Properties of Cu+2-Doped ZnS Semiconductor Nanoparticles. Appl. Phys. A. 125 (4), 273. doi:10.1007/s00339-019-2558-0
Arslan, B., Colpan, M., Ju, X., Zhang, X., Kostyukova, A., and Abu-Lail, N. I. (2016). The Effects of Noncellulosic Compounds on the Nanoscale Interaction Forces Measured between Carbohydrate-Binding Module and Lignocellulosic Biomass. Biomacromolecules 17 (5), 1705–1715. doi:10.1021/acs.biomac.6b00129
Asghar, K., Qasim, M., Nelabhotla, D. M., and Das, D. (2016). Effect of Surfactant and Electrolyte on Surface Modification of C-Plane GaN Substrate Using Chemical Mechanical Planarization (CMP) Process. Colloids Surf. A: Physicochemical Eng. Aspects 497, 133–145. doi:10.1016/j.colsurfa.2016.02.035
Bao, Y., Guo, J., Ma, J., Liu, P., Kang, Q., and Zhang, J. (2017). Cationic Silicon-Based Gemini Surfactants: Effect of Hydrophobic Chains on Surface Activity, Physic-Chemical Properties and Aggregation Behaviors. J. Ind. Eng. Chem. 53 (25), 51–61. doi:10.1016/j.jiec.2017.03.045
Bao, Y., Liu, P., and Guo, J. (2019). Research Progress on the Preparation of Nanomaterials and Mesoporous Materials Using Gemini Surfactants. Mater. Rep. 33 (21), 3678–3685. doi:10.11896/cldb.18050316
Barbosa, O., Ortiz, C., Berenguer-Murcia, Á., Torres, R., Rodrigues, R. C., and Fernandez-Lafuente, R. (2015). Strategies for the One-step Immobilization-Purification of Enzymes as Industrial Biocatalysts. Biotechnol. Adv. 33 (5), 435–456. doi:10.1016/j.biotechadv.2015.03.006
Begum, G., Oschatz, C., Oschatz, M., Kaskel, S., Brunner, E., and Kröger, N. (2019). Influence of Silica Architecture on the Catalytic Activity of Immobilized Glucose Oxidase. Bioinspired, Biomimetic and Nanobiomaterials 8 (1), 72–80. doi:10.1680/jbibn.18.00002
Berlin, Z., Jun, R., Li, X., and Lingyun, J. (2016). Direct Site-specific Immobilization of Protein A via Aldehyde-Hydrazide Conjugation. J. Chromatogr. B. 1008, 132–138. doi:10.1016/j.jchromb.2015.11.019
Bhawna, S., Christian, L., and Claude-Gilles, D. (2020). Comprehensive Assessment of 2G Bioethanol Production. Bioresour. Technol. 313, 123630. doi:10.1016/j.biortech.2020.123630
Bindhu, L. V., and Abraham, E. T. (2010). Immobilization of Horseradish Peroxidase on Chitosan for Use in Nonaqueous media. J. Appl. Polym. Sci. 88 (6), 1456–1464. doi:10.1002/app.11815
Brady, D., and Jordaan, J. (2009). Advances in Enzyme Immobilisation. Biotechnol. Lett. 31 (11), 1639–1650. doi:10.1007/s10529-009-0076-4
Cao, S., Xu, P., Ma, Y., Yao, X., Yao, Y., Zong, M., et al. (2016). Recent Advances in Immobilized Enzymes on Nanocarriers. Chin. J. Catal. 37 (11), 1814–1823. doi:10.1016/s1872-2067(16)62528-7
Carlsson, N., Gustafsson, H., Thörn, C., Olsson, L., Holmberg, K., and Åkerman, B. (2014). Enzymes Immobilized in Mesoporous Silica: A Physical-Chemical Perspective. Adv. Colloid Interf. Sci. 205, 339–360. doi:10.1016/j.cis.2013.08.010
Carrillo, A. I., Linares, N., Serrano, E., and García-Martínez, J. (2011). Well-ordered Mesoporous Interconnected Silica Spheres Prepared Using Extremely Low Surfactant Concentrations. Mater. Chem. Phys. 129 (1), 261–269. doi:10.1016/j.matchemphys.2011.04.015
C. Carter, K., and Puig-Sellart, M. (2016). Nanocarriers Made from Non-ionic Surfactants or Natural Polymers for Pulmonary Drug Delivery. Cpd 22 (22), 3324–3331. doi:10.2174/1381612822666160418121700
Chang, R. H.-Y., Jang, J., and Wu, K. C.-W. (2011). Cellulase Immobilized Mesoporous Silica Nanocatalysts for Efficient Cellulose-To-Glucose Conversion. Green. Chem. 13 (10), 2844–2850. doi:10.1039/c1gc15563f
Chaudhary, S., Rohilla, D., and Mehta, S. K. (2014). Surfactant Adsorption and Aggregate Structure of Silica Nanoparticles: a Versatile Stratagem for the Regulation of Particle Size and Surface Modification. Mater. Res. Express 1 (1), 015011. doi:10.1088/2053-1591/1/1/015011
Chen, L. F., Gong, C. S., and Tsao, G. T. (2010). Immobilized Glucose Isomerase on DEAE Cellulose Beads. Starch - Stärke 33 (2), 58–63. doi:10.1002/star.19810330207
Cherian, E., Dharmendirakumar, M., and Baskar, G. (2015). Immobilization of Cellulase onto MnO2 Nanoparticles for Bioethanol Production by Enhanced Hydrolysis of Agricultural Waste. Chin. J. Catal. 36 (8), 1223–1229. doi:10.1016/s1872-2067(15)60906-8
Chi, X., Peters, G. M., Brockman, C., Lynch, V. M., and Sessler, J. L. (2018). Controlling Structure beyond the Initial Coordination Sphere: Complexation-Induced Reversed Micelle Formation in Calix[4]pyrrole-Containing Diblock Copolymers. J. Am. Chem. Soc. 140 (41), 13219–13222. doi:10.1021/jacs.8b09620
Chu, Q., Tong, W., Wu, S., Jin, Y., Hu, J., and Song, K. (2021). Modification of Lignin by Various Additives to Mitigate Lignin Inhibition for Improved Enzymatic Digestibility of Dilute Acid Pretreated Hardwood. Renew. Energ. 177, 992–1000. doi:10.1016/j.renene.2021.06.048
Cipolatti, E. P., Silva, M. J. A., Klein, M., Feddern, V., Feltes, M. M. C., Oliveira, J. V., et al. (2014). Current Status and Trends in Enzymatic Nanoimmobilization. J. Mol. Catal. B: Enzymatic 99 (1), 56–67. doi:10.1016/j.molcatb.2013.10.019
Cristina, G-G., Ángel, B-M., Roberto, F-L., and Rodrigues, R. C. (2011). Potential of Different Enzyme Immobilization Strategies to Improve Enzyme Performance. Adv. Synth. Catal. 353, 2885–2904. doi:10.1002/adsc.201100534
Cui, X., Wang, J., Zhang, X., Wang, Q., Song, M., and Chai, J. (2019). Preparation of Nano-TiO2 by a Surfactant-free Microemulsion-Hydrothermal Method and its Photocatalytic Activity. Langmuir 35 (28), 9255–9263. doi:10.1021/acs.langmuir.9b01392
DiCosimo, R., McAuliffe, J., Poulose, A. J., and Bohlmann, G. (2013). Industrial Use of Immobilized Enzymes. Chem. Soc. Rev. 42 (15), 6437–6475. doi:10.1039/c3cs35506c
Djajadi, D. T., Pihlajaniemi, V., Rahikainen, J., Kruus, K., and Meyer, A. S. (2018). Cellulases Adsorb Reversibly on Biomass Lignin. Biotechnol. Bioeng. 115 (12), 2869–2880. doi:10.1002/bit.26820
Dong, X. Y., Feng, X. D., and Sun, Y. (2010). His-tagged Protein Purification by Metal-Chelate Affinity Extraction with Nickel-Chelate Reverse Micelles. Biotechnol. Prog. 26 (4), 1088–1094. doi:10.1002/btpr.428
Du, J., Cao, Y., Liu, G., Zhao, J., Li, X., and Qu, Y. (2017). Identifying and Overcoming the Effect of Mass Transfer Limitation on Decreased Yield in Enzymatic Hydrolysis of Lignocellulose at High Solid Concentrations. Bioresour. Tech. 229, 88–95. doi:10.1016/j.biortech.2017.01.011
Eckard, A. D., Muthukumarappan, K., and Gibbons, W. (2012). Pretreatment of Extruded Corn stover with Polyethylene Glycol to Enhance Enzymatic Hydrolysis: Optimization, Kinetics, and Mechanism of Action. Bioenerg. Res. 5 (2), 424–438. doi:10.1007/s12155-011-9162-2
Fan, Y., Wu, G., Su, F., Li, K., Xu, L., Han, X., et al. (2016). Lipase Oriented-Immobilized on Dendrimer-Coated Magnetic Multi-Walled Carbon Nanotubes toward Catalyzing Biodiesel Production from Waste Vegetable Oil. Fuel 178, 172–178. doi:10.1016/j.fuel.2016.03.071
Fiorati, A., Florit, F., Mazzei, A., Buzzaccaro, S., Rossi, B., Piazza, R., et al. (2021). Dispersions of Zirconia Nanoparticles Close to the Phase Boundary of Surfactant-free Ternary Mixtures. Langmuir 37 (14), 4072–4081. doi:10.1021/acs.langmuir.0c03401
Frančič, N., Bellino, M. G., Solerillia, G. J., and Lobnik, A. (2016). Mesoporous Titania Thin Films as Efficient Enzyme Carriers for Paraoxon Determination/detoxification: Effects of Enzyme Binding and Pore Hierarchy on the Biocatalyst Activity and Reusability. Analyst 141 (13), 4235. doi:10.1039/c4an00152d
Fujita, K., and Ohno, H. (2010). Enzymatic Activity and thermal Stability of Metallo Proteins in Hydrated Ionic Liquids. Biopolymers 93 (12), 1093–1099. doi:10.1002/bip.21526
Gao, S., Wang, Y., Wang, T., Luo, G., and Dai, Y. (2009). Immobilization of Lipase on Methyl-Modified Silica Aerogels by Physical Adsorption. Bioresour. Tech. 100 (2), 996–999. doi:10.1016/j.biortech.2008.06.060
geor malar, C., Seenuvasan, M., Kumar, K. S., Kumar, A., and Parthiban, R. (2020). Review on Surface Modification of Nanocarriers to Overcome Diffusion Limitations: An Enzyme Immobilization Aspect. Biochem. Eng. J. 158, 107574. doi:10.1016/j.bej.2020.107574
Ghasemi, S., Yousefi, M., Nikseresht, A., and Omidi, H. (2021). Covalent Binding and In-Situ Immobilization of Lipases on a Flexible Nanoporous Material. Process Biochem. 102, 92–101. doi:10.1016/j.procbio.2020.12.013
Gokhale, A. A., Lu, J., and Lee, I. (2013). Immobilization of Cellulase on Magnetoresponsive Graphene Nano-Supports. J. Mol. Catal. B: Enzymatic 90 (3), 76–86. doi:10.1016/j.molcatb.2013.01.025
Gonçalves Lopes, R. C. F., Silvestre, O. F., Faria, A. R., C do Vale, M. L., Marques, E. F., and Nieder, J. B. (2019). Surface Charge Tunable Catanionic Vesicles Based on Serine-Derived Surfactants as Efficient Nanocarriers for the Delivery of the Anticancer Drug Doxorubicin. Nanoscale 11 (25), 5932–5941. doi:10.1039/c8nr06346j
Gutierrez, J. A., Cruz, J., Rondón, P., Jones, N., and Ortiz, C. (2016). Small Gold Nanocomposites Obtained in Reverse Micelles as Nanoreactors. Effect of Surfactant, Optical Properties and Activity against Pseudomonas aeruginosa. New J. Chem. 40 (12), 10432–10439. doi:10.1039/c6nj02259f
Hassanzadeh-Tabrizi, S. A., Bigham, A., and Rafienia, M. (2016). Surfactant-assisted Sol-Gel Synthesis of Forsterite Nanoparticles as a Novel Drug Delivery System. Mater. Sci. Eng. C 58 (Jan.), 737–741. doi:10.1016/j.msec.2015.09.020
Hernandez, K., and Fernandez-Lafuente, R. (2011). Control of Protein Immobilization: Coupling Immobilization and Site-Directed Mutagenesis to Improve Biocatalyst or Biosensor Performance. Enzyme Microb. Tech. 48 (2), 107–122. doi:10.1016/j.enzmictec.2010.10.003
Hosseini, S. H., Hosseini, S. A., Zohreh, N., Yaghoubi, M., and Pourjavadi, A. (2018). Covalent Immobilization of Cellulase Using Magnetic Poly(ionic Liquid) Support: Improvement of the Enzyme Activity and Stability. J. Agric. Food Chem. 66 (4), 789–798. doi:10.1021/acs.jafc.7b03922
Hui, J., Yingwu, W., Yan, B., Rong, L., and Renjun, G. (2016). Site-specific, Covalent Immobilization of Dehalogenase ST2570 Catalyzed by Formylglycine-Generating Enzymes and its Application in Batch and Semi-continuous Flow Reactors. Molecules 21 (7), 895. doi:10.3390/molecules21070895
Itabaiana, I., Gonçalves, K. M., Zoumpanioti, M., Leal, I. C. R., Miranda, L. S. M. e., Xenakis, A., et al. (2014). Microemulsion-based Organogels as an Efficient Support for Lipase-Catalyzed Reactions under Continuous-Flow Conditions. Org. Process. Res. Dev. 18 (11), 1372–1376. doi:10.1021/op500136c
Jia, W., Collins, S. R. A., Adam, E., Nikolaus, W., Jo, D., Roberts, I. N., et al. (2018). Release of Cell wall Phenolic Esters during Hydrothermal Pretreatment of rice Husk and rice Straw. Biotechnol. Biofuels 11 (1), 162. doi:10.1186/s13068-018-1157-1
Jiang, F., Qian, C., Esker, A. R., and Roman, M. (2017). Effect of Nonionic Surfactants on Dispersion and Polar Interactions in the Adsorption of Cellulases onto Lignin. J. Phys. Chem. B 121 (41), 9607–9620. doi:10.1021/acs.jpcb.7b07716
Jiang, J., Carrillo-Enríquez, N. C., Oguzlu, H., Han, X., Bi, R., Song, M., et al. (2020). High Production Yield and More Thermally Stable Lignin-Containing Cellulose Nanocrystals Isolated Using a Ternary Acidic Deep Eutectic Solvent. ACS Sust. Chem. Eng. 8, 7182–7191. doi:10.1021/acssuschemeng.0c01724
Jordan, J., Kumar, C. S. S. R., and Theegala, C. (2011). Preparation and Characterization of Cellulase-Bound Magnetite Nanoparticles. J. Mol. Catal. B: Enzymatic 68 (2), 139–146. doi:10.1016/j.molcatb.2010.09.010
Kalantari, M., Kazemeini, M., and Arpanaei, A. (2013). Evaluation of Biodiesel Production Using Lipase Immobilized on Magnetic Silica Nanocomposite Particles of Various Structures. Biochem. Eng. J. 79, 267–273. doi:10.1016/j.bej.2013.09.001
Karimi, S., Karri, R. R., Yaraki, M. T., and Koduru, J. R. (2021). Processes and Separation Technologies for the Production of Fuel-Grade Bioethanol: a Review. Environ. Chem. Lett. 19 (4), 1–18. doi:10.1007/s10311-021-01208-9
Kawasaki, H. (2013). Surfactant-free Solution-Based Synthesis of Metallic Nanoparticles toward Efficient Use of the Nanoparticles' Surfaces and Their Application in Catalysis and Chemo-/biosensing. Nanotechnol. Rev. 2 (1), 5–25. doi:10.1515/ntrev-2012-0079
Kayhomayun, Z., Ghani, K., and Zargoosh, K. (2020). T Template-Directed Synthesis of Sm2Ti2O7 Nanoparticles: a Novel FRET-Based Fluorescent Chemosensor for the Fast and Selective Determination of Picric Acid. New J. Chem. 44 (38), 1–12. doi:10.1039/d0nj04219f
Kellock, M., Rahikainen, J., Marjamaa, K., and Kruus, K. (2017). Lignin-derived Inhibition of Monocomponent Cellulases and a Xylanase in the Hydrolysis of Lignocellulosics. Bioresour. Tech. 232, 183–191. doi:10.1016/j.biortech.2017.01.072
Kovalchuk, N. M. (2015). Spontaneous Non-linear Oscillations of Interfacial Tension at Oil/water Interface. Open Chem. 13 (1), 1–16. doi:10.1515/chem-2015-0009
Kumar, R., and Wyman, C. E. (2010). Effect of Additives on the Digestibility of Corn stover Solids Following Pretreatment by Leading Technologies. Biotechnol. Bioeng. 102 (6), 1544–1557. doi:10.1002/bit.22203
Lai, N., Nie, X., Zheng, X., Zhao, W., Zhao, X., and Wang, Y. (2020). Experimental Investigation on a Novel Polyelectrolyte Molecular Deposition Film for Improved Injectivity in Low-Permeability Reservoirs. ACS Omega 5 (45), 29300–29311. doi:10.1021/acsomega.0c04084
Leonidas, M., Vijayendran, R., Olga, Y., Gustav, P., Eva, O., Ulrika, R., et al. (2019). Lignin-first Biomass Fractionation Using a Hybrid Organosolv-Steam Explosion Pretreatment Technology Improves the Saccharification and Fermentability of spruce Biomass. Bioresour. Technol. 273, 521–528. doi:10.1016/j.biortech.2018.11.055
Li, F. H., Tang, N., Wang, Y. Q., Zhang, L., Du, W., Xiang, J., et al. (2018). Synthesis and Characterization of Magnetic Carriers Based on Immobilized Enzyme. IOP Conf. Ser. Mater. Sci. Eng. 359, 012044. doi:10.1088/1757-899x/359/1/012044
Li, J., Li, S., Fan, C., and Yan, Z. (2012). The Mechanism of Poly(ethylene Glycol) 4000 Effect on Enzymatic Hydrolysis of Lignocellulose. Colloids Surf. B: Biointerfaces 89 (1), 203–210. doi:10.1016/j.colsurfb.2011.09.019
Li, M., Yue, Y., Zhang, Z.-J., Wang, Z.-Y., Tan, T.-W., and Fan, L.-H. (2016). Site-specific and High-Loading Immobilization of Proteins by Using Cohesin-Dockerin and CBM-Cellulose Interactions. Bioconjug. Chem. 27 (7), 1579–1583. doi:10.1021/acs.bioconjchem.6b00282
Li, Y., Guan, X., Chaffey, P. K., Ruan, Y., Ma, B., Shang, S., et al. (2020). Carbohydrate-binding Module O-Mannosylation Alters Binding Selectivity to Cellulose and Lignin. Chem. Sci. 11, 9262–9271. doi:10.1039/d0sc01812k
Liu, J., Shi, J., Li, J., and Yuan, X. (2011). Characterization of the Interaction between Surfactants and Enzymes by Fluorescence Probe. Enzyme Microb. Tech. 49 (4), 360–365. doi:10.1016/j.enzmictec.2011.06.014
Liu, Y., and Yu, J. (2016). Oriented Immobilization of Proteins on Solid Supports for Use in Biosensors and Biochips: a Review. Microchim. Acta 183, 1–19. doi:10.1007/s00604-015-1623-4
Lok-Kumar, S., Rekha, G. S., Neus, V., Carlos, R. A., and Katsuhiko, A. (2014). In-situ Formation of Silver Nanoparticles Using Nonionic Surfactant Reverse Micelles as Nanoreactors. J. Nanosci. Nanotechnol. 14 (3), 2238–2244. doi:10.1166/jnn.2014.9229
Lou, H., Zeng, M., Hu, Q., Cai, C., Lin, X., Qiu, X., et al. (2017). Nonionic Surfactants Enhanced Enzymatic Hydrolysis of Cellulose by Reducing Cellulase Deactivation Caused by Shear Force and Air-Liquid Interface. Bioresour. Technol. 249, 1–8. doi:10.1016/j.biortech.2017.07.066
Lou, H., Zhou, H., Li, X., Wang, M., Zhu, J. Y., and Qiu, X. (2014). Understanding the Effects of Lignosulfonate on Enzymatic Saccharification of Pure Cellulose. Cellulose 21 (3), 1351–1359. doi:10.1007/s10570-014-0237-z
Luan, Y., and Ramos, L. (2010). Role of the Preparation Procedure in the Formation of Spherical and Monodisperse Surfactant/polyelectrolyte Complexes. Chemistry 13 (21), 6108–6114. doi:10.1002/chem.200601422
Maia, J. L. d., Cardoso, J. S., Mastrantonio, D. J. d. S., Bierhals, C. K., Moreira, J. B., Costa, J. A. V., et al. (2020). Microalgae Starch: A Promising Raw Material for the Bioethanol Production. Int. J. Biol. Macromolecules 165, 2739–2749. doi:10.1016/j.ijbiomac.2020.10.159
Malar, C. G., Seenuvasan, M., and Kumar, K. S. (2018). Prominent Study on Surface Properties and Diffusion Coefficient of Urease-Conjugated Magnetite Nanoparticles. Appl. Biochem. Biotechnol. 186, 174–185. doi:10.1007/s12010-018-2719-1
Marhuendaegea, F. C., Pieravelázquez, S., Cadenas, C., and Cadenas, E. (2015). Reverse Micelles in Organic Solvents: a Medium for the Biotechnological Use of Extreme Halophilic Enzymes at Low Salt Concentration. Archaea 1 (2), 105.
Mehta, J., Bhardwaj, N., Bhardwaj, S. K., Kim, K.-H., and Deep, A. (2016). Recent Advances in Enzyme Immobilization Techniques: Metal-Organic Frameworks as Novel Substrates. Coord. Chem. Rev. 322, 30–40. doi:10.1016/j.ccr.2016.05.007
Mita, D. G., and Eldin, M. S. M. (2014). Immobilized Enzymes: Strategies for Overcoming the Substrate Diffusion- Limitation Problem. Curr. Biotechnol. 3 (3), 207–217. doi:10.2174/221155010303140918114737
Mnich, E., Bjarnholt, N., Eudes, A., Harholt, J., Holland, C., Jørgensen, B., et al. (2020). Phenolic Cross-Links: Building and De-constructing the Plant Cell wall. Nat. Prod. Rep. 37 (4), 919–961. doi:10.1039/c9np00028c
Mo, H., Qiu, J., Yang, C., Zang, L., Sakai, E., and Chen, J. (2020). Porous Biochar/chitosan Composites for High Performance Cellulase Immobilization by Glutaraldehyde. Enzyme Microb. Tech. 138, 109561. doi:10.1016/j.enzmictec.2020.109561
Moniruzzaman, M., Kamiya, N., and Goto, M. (2010). Activation and Stabilization of Enzymes in Ionic Liquids. Org. Biomol. Chem. 8 (13), 2887–2899. doi:10.1039/b926130c
Mroczkiewicz, M., Bronowska, A., Pietrzak, M., and Malinowska, E. (2012). Different Methods of Acid Phosphatase Immobilization for its Application in FIA Systems with Potentiometric Detection. Proced. Eng. 47 (5), 265–268. doi:10.1016/j.proeng.2012.09.134
Muginova, S. V., Galimova, A. Z., Polyakov, A. E., and Shekhovtsova, T. N. (2010). Ionic Liquids in Enzymatic Catalysis and Biochemical Methods of Analysis: Capabilities and Prospects. J. Anal. Chem. 65 (4), 331–351. doi:10.1134/s1061934810040027
Nakayama, R.-i., Imai, M., and Suzuki, I. (2009). Enzymatic Cellulose Degradation Using Suitable Combination of Cellulase and Enhancement of Reaction Rate with the Aid of Ultrasonic Pretreatment. J. Biosci. Bioeng. 108 (Suppl. S1), S89. doi:10.1016/j.jbiosc.2009.08.261
Neira, H. D., and Herr, A. E. (2017). Kinetic Analysis of Enzymes Immobilized in Porous Film Arrays. Anal. Chem. 89 (19), 10311–10320. doi:10.1021/acs.analchem.7b02075
Nicoletti, G., Cipolatti, E. P., Valério, A., Carbonera, N. G., Soares, N. S., Theilacker, E., et al. (2015). Evaluation of Different Methods for Immobilization of Candida antarctica Lipase B (CalB Lipase) in Polyurethane Foam and its Application in the Production of Geranyl Propionate. Bioproc. Biosyst Eng 38 (9), 1739–1748. doi:10.1007/s00449-015-1415-6
Orellana, J. L., Wichhart, D., and Kitchens, C. L. (20182018). Mechanical and Optical Properties of Polylactic Acid Films Containing Surfactant-Modified Cellulose Nanocrystals. J. Nanomater. 2018, 1–12. doi:10.1155/2018/7124260
Ortiz-Martínez, V., Gómez-Coma, L., Ortiz, A., and Ortiz, I. (2019). Overview on the Use of Surfactants for the Preparation of Porous Carbon Materials by the Sol-Gel Method: Applications in Energy Systems. Rev. Chem. Eng. 36 (7), 1–17. doi:10.1515/revce-2018-0056
Ouyang, J., Pu, S., Wang, J., Deng, Y., Yang, C., Naseer, S., et al. (2020). Enzymatic Hydrolysate of Geniposide Directly Acts as Cross-Linking Agent for Enzyme Immobilization. Process Biochem. 99, 187–195. doi:10.1016/j.procbio.2020.09.006
Pavlidis, I. V., Tzafestas, K., and Stamatis, H. (2010). Water-in-ionic Liquid Microemulsion-Based Organogels as Novel Matrices for Enzyme Immobilization. Biotechnol. J. 5 (8), 805–812. doi:10.1002/biot.201000052
Predvoditelev, D. A., Suvorkin, S. V., and Vasyanina, E. (2010). 4-Chloromethyl-1,3,2-dioxaphospholanes in the Synthesis of New Types of Diglycerophospholipids. Cheminform 28, 208. doi:10.1002/chin.200128218
Priydarshani, S., Mustafa, M., Yuan, G., Robinson, A. J., and Ilias, K. (2018). Immobilization and Stabilization of Alcohol Dehydrogenase on Polyvinyl Alcohol Fibre. Biotechnol. Rep. 19, e00260. doi:10.1016/j.btre.2018.e00260
Qing, Q., Yang, B., and Wyman, C. E. (2010). Impact of Surfactants on Pretreatment of Corn stover. Bioresour. Tech. 101 (15), 5941–5951. doi:10.1016/j.biortech.2010.03.003
Qiu, X., Wang, S., Miao, S., Suo, H., Xu, H., and Hu, Y. (2021). Co-immobilization of Laccase and ABTS onto Amino-Functionalized Ionic Liquid-Modified Magnetic Chitosan Nanoparticles for Pollutants Removal. J. Hazard. Mater. 401, 123353. doi:10.1016/j.jhazmat.2020.123353
Rahikainen, J., Mikander, S., Marjamaa, K., Tamminen, T., Lappas, A., Viikari, L., et al. (2011). Inhibition of Enzymatic Hydrolysis by Residual Lignins from Softwood-Study of Enzyme Binding and Inactivation on Lignin-Rich Surface. Biotechnol. Bioeng. 108 (12), 2823–2834. doi:10.1002/bit.23242
Rajnish, K. N., Samuel, M. S., John J, A., Datta, S., Chandrasekar, N., Balaji, R., et al. (2021). Immobilization of Cellulase Enzymes on Nano and Micro-materials for Breakdown of Cellulose for Biofuel Production-A Narrative Review. Int. J. Biol. Macromolecules 182 (6), 1793–1802. doi:10.1016/j.ijbiomac.2021.05.176
Riedel, M., Sabir, N., Scheller, F. W., Parak, W. J., and Lisdat, F. (2017). Connecting Quantum Dots with Enzymes: Mediator-Based Approaches for the Light-Directed Read-Out of Glucose and Fructose Oxidation. Nanoscale 9 (8), 2814–2823. doi:10.1039/c7nr00091j
Rocha-Martín, J., Martínez-Bernal, C., Zamorano, L. S., Reyes-Sosa, F. M., and Díez García, B. (2018). Inhibition of Enzymatic Hydrolysis of Pretreated Corn stover and Sugar Cane Straw by Laccases. Process Biochem. 67 (APR), 88–91. doi:10.1016/j.procbio.2018.01.021
Roth, H.-C., Schwaminger, S. P., Peng, F., and Berensmeier, S. (2016). Immobilization of Cellulase on Magnetic Nanocarriers. Chemistryopen 5 (3), 183–187. doi:10.1002/open.201600028
Saha, K., Verma, P., Sikder, J., Chakraborty, S., and Curcio, S. (2019). Synthesis of Chitosan-Cellulase Nanohybrid and Immobilization on Alginate Beads for Hydrolysis of Ionic Liquid Pretreated Sugarcane Bagasse. Renew. Energ. 133, 66–76. doi:10.1016/j.renene.2018.10.014
Santos, J. C. S. d., Barbosa, O., Ortiz, C., Berenguer-Murcia, A., Rodrigues, R. C., and Fernandez-Lafuente, R. (2015). Importance of the Support Properties for Immobilization or Purification of Enzymes. Chemcatchem 7 (16), 2413–2432. doi:10.1002/cctc.201500310
Savic, S., Petrovic, S., Savic, S., and Petronijevic, Z. (2021). Immobilization of Horseradish Peroxidase on Modified Cellulose Carriers via Hydrophobic Interactions: Catalytic Properties and Stability. Iran J. Sci. Technol. Trans. Sci. 45 (1), 55–63. doi:10.1007/s40995-020-01027-7
Seo, D.-J., Fujita, H., and Sakoda, A. (2011a). Effects of a Non-ionic Surfactant, Tween 20, on Adsorption/desorption of Saccharification Enzymes Onto/from Lignocelluloses and Saccharification Rate. Adsorption 17 (5), 813–822. doi:10.1007/s10450-011-9340-8
Seo, D.-J., Fujita, H., and Sakoda, A. (2011b). Structural Changes of Lignocelluloses by a Nonionic Surfactant, Tween 20, and Their Effects on Cellulase Adsorption and Saccharification. Bioresour. Tech. 102 (20), 9605–9612. doi:10.1016/j.biortech.2011.07.034
Shil, A., Hussain, S. A., and Bhattacharjee, D. (2017). Surfactant Concentration Dependent Metachromasy of an Anionic Cyanine Dye in Adsorbed and Deposited Langmuir Films. Chem. Phys. Lett. 676, 99–107. doi:10.1016/j.cplett.2017.03.052
Singh, T. A., Jajoo, A., and Bhasin, S. (2020). Optimization of Various Encapsulation Systems for Efficient Immobilization of Actinobacterial Glucose Isomerase. Biocatal. Agric. Biotechnol. 29, 101766. doi:10.1016/j.bcab.2020.101766
Sipos, B., Dienes, D., Schleicher, Á., Perazzini, R., Crestini, C., Siika-aho, M., et al. (2010). Hydrolysis Efficiency and Enzyme Adsorption on Steam-Pretreated spruce in the Presence of Poly(ethylene Glycol). Enzyme Microb. Tech. 47 (3), 84–90. doi:10.1016/j.enzmictec.2010.05.010
Song, Y., Chandra, R. P., Zhang, X., and Saddler, J. N. (2020). Non-productive Celluase Binding onto Deep Eutectic Solvent (DES) Extracted Lignin from Willow and Corn stover with Inhibitory Effects on Enzymatic Hydrolysis of Cellulose. Carbohydr. Polym. 250, 116956. doi:10.1016/j.carbpol.2020.116956
Steen Redeker, E., Ta, D. T., Cortens, D., Billen, B., Guedens, W., and Adriaensens, P. (2013). Protein Engineering for Directed Immobilization. Bioconjug. Chem. 24 (11), 1761–1777. doi:10.1021/bc4002823
Suárez, S., Guerrero, C., Vera, C., and Illanes, A. (2018). Effect of Particle Size and Enzyme Load on the Simultaneous Reactions of Lactose Hydrolysis and Transgalactosylation with Glyoxyl-Agarose Immobilized β-galactosidase from Aspergillus oryzae. Process. Biochem. 73 (OCT), 56–64. doi:10.1016/j.procbio.2018.08.016
Suhartini, S., Rohma, N. A., Mardawati, E., KasbawatiHidayat, N., and Melville, L. (2022). Biorefining of Oil palm Empty Fruit Bunches for Bioethanol and Xylitol Production in Indonesia: A Review. Renew. Sust. Energ. Rev. 154, 11187. doi:10.1016/j.rser.2021.111817
Sui, C., Cheng, W., Dong, S., Han, L., and Pharmacy, D. O. (2015). Immobilization of Glucoamylase onto the Electrospinning PEI/PVA Composite Nanofiber Membrane via Ionic Adsorption. Biotechnol. Bull. 2, 167–172. doi:10.13560/j.cnki.biotech.bull.1985.2015.02.025
Takagi, S., Arakawa, K., Shimada, T., and Inoue, H. (2019). Reversed Micelles Formed by Polyfluorinated Surfactant Ii; the Properties of Core Water Phase in Reversed Micelle. Bcsj 92 (7), 1200–1204. doi:10.1246/bcsj.20190086
Tan, J., Xiong, X., He, Z., Cao, F., and Sun, D. (2019). Aggregation Behavior of Polyether Based Siloxane Surfactants in Aqueous Solutions: Effect of Alkyl Groups and Steric Hindrance. J. Phys. Chem. B 123 (6), 1390–1399. doi:10.1021/acs.jpcb.8b10727
Tao, L., Zhao, Y., Wang, X., Xiang, L., and Yan, Y. (2013). A Novel Oriented Immobilized Lipase on Magnetic Nanoparticles in Reverse Micelles System and its Application in the Enrichment of Polyunsaturated Fatty Acids. Bioresour. Technol. 132 (7), 99–102. doi:10.1016/j.biortech.2012.12.191
Teresa, V. S., Rebolledo, A. F., Sevilla, M., Valle-Vigon, P., and Tartaj, P. (2009). Preparation, Characterization, and Enzyme Immobilization Capacities of Superparamagnetic Silica/iron Oxide Nanocomposites with Mesostructured Porosity. Chem. Mater. 21 (9), 1806–1814. doi:10.1021/cm8005937
Tomme, P., Creagh, A. L., Kilburn, D. G., and Haynes, C. A. (2015). Interaction of Polysaccharides with the N-Terminal Cellulose-Binding Domain of Cellulomonas Fimi CenC. 1. Binding Specificity and Calorimetric Analysis. Biochemistry 35 (44), 13885–13894. doi:10.1021/bi961185i
Urrutia, P., Bernal, C., Wilson, L., and Illanes, A. (2018). Use of Chitosan Heterofunctionality for Enzyme Immobilization: β-galactosidase Immobilization for Galacto-Oligosaccharide Synthesis. Int. J. Biol. Macromolecules 116, 182–193. doi:10.1016/j.ijbiomac.2018.04.112
Valencia, P., Wilson, L., Aguirre, C., and Illanes, A. (2010). Evaluation of the Incidence of Diffusional Restrictions on the Enzymatic Reactions of Hydrolysis of Penicillin G and Synthesis of Cephalexin. Enzyme Microb. Tech. 47 (6), 268–276. doi:10.1016/j.enzmictec.2010.07.010
Wang, M., Wang, Y., Yu, D., Han, Y., and Wang, Y. (2013a). Salt Effects on the Aggregation Behavior of Tripolar Zwitterionic Surfactants with Different Inter-charge Spacers in Aqueous Solution. Colloid Polym. Sci. 291 (7), 1613–1621. doi:10.1007/s00396-013-2895-z
Wang, Z., Lan, T., and Zhu, J. (2013b). Lignosulfonate and Elevated pH Can Enhance Enzymatic Saccharification of Lignocelluloses. Biotechnol. Biofuels 6 (1), 9. doi:10.1186/1754-6834-6-9
Watanabe, T., Mori, T., Tosa, T., and Chibata, I. (2010). Immobilization of Aminoacylase by Adsorption to Tannin Immobilized on Aminohexyl Cellulose. Biotechnol. Bioeng. 21 (3), 477–486. doi:10.1002/bit.260210309
Webster, I. A., Schwier, C. E., and Bates, F. S. (2015). Using the Rotational Masking Concept to Enhance Substrate Inhibited Reaction Rates: Controlled Pore Supports for Enzyme Immobilization. Enzyme Microb. Technol. 7 (6), 266–274. doi:10.1016/0141-0229(85)90084-5
Wei, J., Wen, X., and Zhu, F. (2018). Influence of Surfactant on the Morphology and Photocatalytic Activity of Anatase TiO2 by Solvothermal Synthesis. J. Nanomater. 2018, 1–7. doi:10.1155/2018/3086269
Winarni, I., Bardant, T., and Hendra, D. (2020). Enhancement the Added Value of Sengon wood Waste Pulp as Bioenergy Raw Material for Bioethanol Production. IOP Conf. Ser. Earth Environ. Sci. 415, 012012. doi:10.1088/1755-1315/415/1/012012
Xing, Z., Su, Y., Zhang, Q., Ruan, X., Lin, Y., Wang, X., et al. (2015). Research Progress on Cellulase Immobilized by Magnetic Nanoparticles as Carriers. Biotechnol. Bull. 8, 59–65. doi:10.13560/j.cnki.biotech.bull.1985.2015.08.009
Xu, G., Jiang, Y., Tao, R., Wang, S., Zeng, H., and Yang, S. (2016). A Recyclable Biotransformation System for L-2-Aminobutyric Acid Production Based on Immobilized Enzyme Technology. Biotechnol. Lett. 38 (1), 123–129. doi:10.1007/s10529-015-1957-3
Xu, M., Ji, D., Deng, Y., and Agyei, D. (2020). Preparation and Assessment of Cross-Linked Enzyme Aggregates (CLEAs) of β-galactosidase from Lactobacillus Leichmannii 313. Food Bioproducts Process. 124, 82–96. doi:10.1016/j.fbp.2020.08.004
Xu, S., Sun, X., Ye, H., You, T., Song, X., and Sun, S. (2010). Selective Synthesis of Copper Nanoplates and Nanowires via a Surfactant-Assisted Hydrothermal Process. Mater. Chem. Phys. 120 (1), 1–5. doi:10.1016/j.matchemphys.2009.10.049
Yang, Q., Wang, B., Zhang, Z., Lou, D., Tan, J., and Zhu, L. (2017). The Effects of Macromolecular Crowding and Surface Charge on the Properties of an Immobilized Enzyme: Activity, thermal Stability, Catalytic Efficiency and Reusability. RSC Adv. 7 (60), 38028–38036. doi:10.1039/c7ra06544b
Yiamsawas, D., Beckers, S., Lu, H., Landfester, K., and Wurm, F. R. (2017). Morphology-controlled Synthesis of Lignin Nanocarriers for Drug Delivery and Carbon Materials. ACS Biomater. Sci. Eng. 3 (10), 1–26. doi:10.1021/acsbiomaterials.7b00278
You, J., Choi, H. H., Kim, T. A., Park, M., Ha, J. S., Lee, S. S., et al. (2019). High-performance Polyketone Nanocomposites Achieved via Plasma-Assisted Mechanochemistry. Compos. Sci. Technol. 183 (Oct.20), 107801–107808. doi:10.1016/j.compscitech.2019.107800
Yu, C.-C., Kuo, Y.-Y., Liang, C.-F., Chien, W.-T., Wu, H.-T., Chang, T.-C., et al. (2012). Site-specific Immobilization of Enzymes on Magnetic Nanoparticles and Their Use in Organic Synthesis. Bioconjug. Chem. 23 (4), 714–724. doi:10.1021/bc200396r
Yu, Y., and Zhang, J. (2016). Interaction of Cellulase with Surfactants and Their Application in Detergent. CIESC J. 67 (7), 3023–3031.
Zang, L., Qiu, J., Wu, X., Zhang, W., Sakai, E., and Wei, Y. (2014). Preparation of Magnetic Chitosan Nanoparticles as Support for Cellulase Immobilization. Ind. Eng. Chem. Res. 53 (9), 3448–3454. doi:10.1021/ie404072s
Zeng, Q., Chang, S., Beyhaqi, A., Wang, M., and Hu, C. (2019). Efficient Electricity Production Coupled with Water Treatment via a Highly Adaptable, Successive Water-Energy Synergistic System. Nano Energy 67, 104237.
Zeng, Q., Chang, S., Wang, M., Li, M., Deng, Q., Xiong, Z., et al. (2021). Highly-active, Metal-free, Carbon-Based Orr Cathode for Efficient Organics Removal and Electricity Generation in a Pfc System. Chin. Chem. Lett. 32 (7), 2212–2216. doi:10.1016/j.cclet.2020.12.062
Zhang, B., Li, P., Zhang, H., Wang, H., Li, X., Tian, L., et al. (2016). Preparation of lipase/Zn3(PO4)2 Hybrid Nanoflower and its Catalytic Performance as an Immobilized Enzyme. Chem. Eng. J. 291, 287–297. doi:10.1016/j.cej.2016.01.104
Zhang, H., and Hay, A. G. (2019). Magnetic Biochar Derived from Biosolids via Hydrothermal Carbonization: Enzyme Immobilization, Immobilized-Enzyme Kinetics, Environmental Toxicity. J. Hazard. Mater. 384, 121272. doi:10.1016/j.jhazmat.2019.121272
Zhang, W.-W., Wang, N., Zhou, Y.-J., He, T., and Yu, X.-Q. (2012). Enhancement of Activity and Stability of Lipase by Microemulsion-Based Organogels (MBGs) Immobilization and Application for Synthesis of Arylethyl Acetate. J. Mol. Catal. B: Enzymatic 78, 65–71. doi:10.1016/j.molcatb.2012.02.005
Zhang, X., Wang, S., Wu, X., Liu, S., Li, D., Xu, H., et al. (2015). Subsite-specific Contributions of Different Aromatic Residues in the Active Site Architecture of Glycoside Hydrolase Family 12. Sci. Rep. 5, 18357. doi:10.1038/srep18357
Zheng, Y., Yu, Y., Lin, W., Jin, Y., Yong, Q., and Huang, C. (2021). Enhancing the Enzymatic Digestibility of Bamboo Residues by Biphasic Phenoxyethanol-Acid Pretreatment. Bioresour. Tech. 325 (3), 124691. doi:10.1016/j.biortech.2021.124691
Zhou, W., Rao, Y., Zhuang, W., Ge, L., Lin, R., Tang, T., et al. (2021). Improved Enzymatic Activity by Oriented Immobilization on Graphene Oxide with Tunable Surface Heterogeneity. Composites B: Eng. 216, 108788. doi:10.1016/j.compositesb.2021.108788
Zhou, Y., Chen, H., Qi, F., Zhao, X., and Liu, D. (2015). Non-ionic Surfactants Do Not Consistently Improve the Enzymatic Hydrolysis of Pure Cellulose. Bioresour. Tech. 182, 136–143. doi:10.1016/j.biortech.2015.01.137
Zhou, Y., Zhang, L., and Tao, S. (2018). Porous TiO2with Large Surface Area Is an Efficient Catalyst Carrier for the Recovery of Wastewater Containing an Ultrahigh Concentration of Dye. RSC Adv. 8 (7), 3433–3442. doi:10.1039/c7ra11985b
Ziaei-Rad, Z., Fooladi, J., Pazouki, M., and Gummadi, S. N. (2021). Lignocellulosic Biomass Pre-treatment Using Low-Cost Ionic Liquid for Bioethanol Production: An Economically Viable Method for Wheat Straw Fractionation. Biomass and Bioenergy 151, 106140. doi:10.1016/j.biombioe.2021.106140
Keywords: cellulase, surfactants, nanocarriers, reversed micelle system, oriented immobilization
Citation: Wang Z, Fan C, Zheng X, Jin Z, Bei K, Zhao M and Kong H (2022) Roles of Surfactants in Oriented Immobilization of Cellulase on Nanocarriers and Multiphase Hydrolysis System. Front. Chem. 10:884398. doi: 10.3389/fchem.2022.884398
Received: 26 February 2022; Accepted: 09 March 2022;
Published: 23 March 2022.
Edited by:
Qingyi Zeng, University of South China, ChinaCopyright © 2022 Wang, Fan, Zheng, Jin, Bei, Zhao and Kong. This is an open-access article distributed under the terms of the Creative Commons Attribution License (CC BY). The use, distribution or reproduction in other forums is permitted, provided the original author(s) and the copyright owner(s) are credited and that the original publication in this journal is cited, in accordance with accepted academic practice. No use, distribution or reproduction is permitted which does not comply with these terms.
*Correspondence: Xiangyong Zheng, eC56aGVuZ0B3enUuZWR1LmNu