- 1Orthopedic Surgery Department, Institute of Arthritis Research in Integrative Medicine, Shanghai Academy of Traditional Chinese Medicine, Guanghua Hospital Affiliated to Shanghai University of Traditional Chinese Medicine, Shanghai, China
- 2Cancer Center, Department of Ultrasound Medicine, Zhejiang Provincial People’s Hospital, Affiliated People’s Hospital, Hangzhou Medical College, Hangzhou, China
- 3Central Laboratory, Shanghai Tenth People’s Hospital, Tongji University School of Medicine, Shanghai, China
Cancer is a serious health problem which increasingly causes morbidity and mortality worldwide. It causes abnormal and uncontrolled cell division. Traditional cancer treatments include surgery, chemotherapy, radiotherapy and so on. These traditional therapies suffer from high toxicity and arouse safety concern in normal area and have difficulty in accurately targeting tumour. Recently, a variety of nanomaterials could be used for cancer diagnosis and therapy. Nanomaterials have several advantages, e.g., high concentration in tumour via targeting design, reduced toxicity in normal area and controlled drug release after various rational designs. They can combine with many types of biomaterials in order to improve biocompatibility. In this review, we outlined the latest research on the use of bioresponsive nanomaterials for various cancer imaging modalities (magnetic resonance imaging, positron emission tomography and phototacoustic imaging) and imaging-guided therapy means (chemotherapy, radiotherapy, photothermal therapy and photodynamic therapy), followed by discussing the challenges and future perspectives of this bioresponsive nanomaterials in biomedicine.
Introduction
Cancer is one of malignant diseases that can happen in different parts of the body (Barreto et al.,2011). It causes abnormal cells division and various malignant behaviors such as cancer cells uncontrolled growth, invasion, metastasis and immortality (Barreto et al.,2011). Gene mutations that control cell cycles are mostly associated with cancer progression (Husband & Reznek, 2000). There are a variety of factors can trigger gene mutations such as exposure to ultraviolet light, infectious pathogens and so on (Rai et al.,2021). Cancer is an extremely complex disease and its treatment is still unspecific. Chemotherapy, radiotherapy, immunotherapy and surgery are main treatment methods for cancer (Mahasneh et al.,2017). Drug resistance is a major obstacle in cancer treatment due to epigenetic changes, upregulated drug efflux and many cellular mechanisms (Holohan et al.,2013; Turner & Reis-Filho, 2012). Both chemotherapy and radiotherapy have side effects on healthy tissues and poor specificities for cancer tissues. For instance, the common chemotherapy agents such as doxorubicin and paclitaxel can exhibit anti-cancer effects and kill normal cells as well (Yoo et al.,2000)I, which is inevitable to cause many side effects due to its non-selective cytotoxic effects. Every year, cancer-arised deaths account for about 13% of all deaths, cancer-related mortality is expected to rise to about 13.1 million until 2030 (Tan et al.,2020). Therefore, cancer has become a huge health burden wordwide (Vogelstein et al.,2013).
Nanomaterials (NMs)-based cancer diagnostics is a relatively-new field, which can be used to diagnose cancer rapidly, regenerate drug delivery tissue, develop new medical products and enhance the efficiency of cancer treatment (Chen et al.,2016; Shapira et al.,2011). Currently, nanomaterials can be divided into many types according to different internal or external stimuli such as mechanical, magnetic, electrical, optical and biological ones (Wei et al.,2019; Zhang et al.,2019). Nanomaterials have many advantages such as nanoscale size, high surface-to-volume ratio, promising and controlled drug release profiles, and the robust ability to differentiate and eradicate malignant cells selectively (Tran et al.,2017; Wang et al.,2019). Because of these advantages, nanotechnology has attracted interests in cancer therapeutics. For instance, many nanovehicle platforms (10–200 nm) are favorable for loading drugs, specifically targeting tumor tissues and entering tumor cells (Davis et al.,2008; Duncan, 2003). This review aims to discuss different types of nanomaterials used in different imaging modalities with an highlight on the applications of bioresponsive nanomaterials in cancer imaging-guided therapy.
Brief Overview of Different Kinds of Nanomaterials
Nanomaterials are chemical substances including Nanoparticles, nanosphere, nanostars, nanorods and nanoshells (Siddique & Chow, 2020). Nanomaterials are used in many fields, e.g., drug carriers, chemotherapy agents, photoacoustic agents, molecular manufacturing, photothermal agents, radiation dose enhancers, materials reactivity, biomarker discovery substances, molecular target therapy, biomedical imaging and so on (Hull et al.,2014; Mehta et al.,2019). Various types of nanomaterials can be used in cancer treatment such as quantum dots, graphene, gold nanoparticles, polymeric micelles, liposomes, silica nanoparticles, magnetic nanoparticles, carbon nanotubes, polymer-drug conjugates, and polymeric nanoparticles (Rai et al.,2021) (Figure 1).
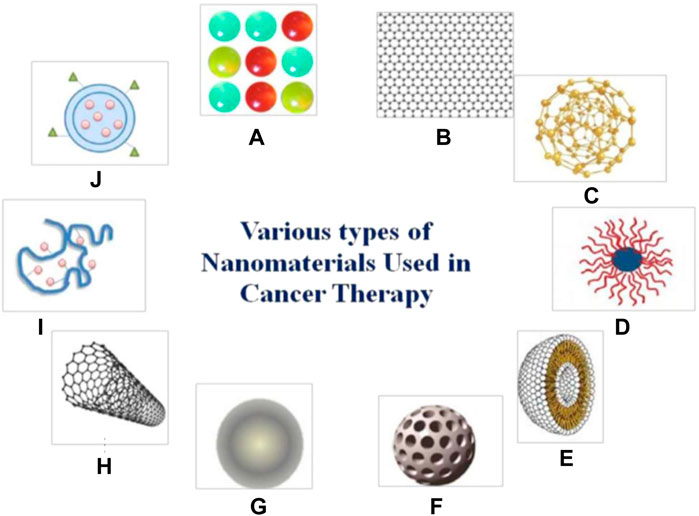
FIGURE 1. Different types of nanomaterials which are used in cancer treatment (A) quantum dots. (B) grapheme. (C) gold nanoparticles. (D) polymeric micelles. (E) liposomes. (F) silica nanoparticles. (G) magnetic nanoparticles. (H) carbon nanotubes. (I) polymer-drug conjugates. (J) polymeric nanoparticles. Reprinted (adapted) with permission from Rai et al. (2021). Medicina (Kaunas). 2021,57 (2),91. Copyright 2021 by the authors.
In recent years, nanotechnology made tremendous progress in developing many nanostructured materials for diagnosis and treatment of cancer (Couvreur & Vauthier, 2006). Nanomaterials have a variety of unique advantages compared with other conventional anticancer agents. First, nanomaterials feature small size, approximately 100–1,000 times smaller than the size of a cancer cell. As a result, nanomaterials exhibit higher intracellular uptake and favorable targeting drug delivery (Goldberg et al.,2007). Second, nanomaterials hold great potential to overcome many limitations of the conventional chemotherapeutic agents. As a paradigm, paclitaxel is one of chemotherapeutic agents, which can treat breast, ovarian and other cancers (Lee et al.,2009). However, it has poor solubility and cannot be dissolved in aqueous solution readily (Bae et al.,2007). Nanovehicles such as liposomes and polymeric micelles impart them with an ability to enhance water solubility of theses hydrophobic drugs by incorporating them in the hydrophobic microenvironments (Hubbell, 2003). Third, owing to the enormous surface area, nanomaterials can carry many imaging and therapeutic agents. It is reported that a polymeric nanoparticle with an average diameter of 70 nm has the ability to accommodate almost 2000 drug molecules (Bartlett & Davis, 2007). Such a high drug loading capacity significantly improves the therapeutic efficacy of chemotherapeutic agents against cancer.
Additionally, nanomaterials paly a vital role in medical imaging such as magnetic resonance imaging (MRI), positron emission tomography (PET) and phototacoustic imaging. It is of great significance using iron oxide nanoparticles in T1-weighted and/or T2-weighted MRI and radioisotope chelator-free particles in PET (Rosado-de-Castro et al.,2018). Over the past few years, although nanomaterials have made some success in imaging and treatment of cancer to some extent, there are still many obstacles in its clinical application. For example, MRI has a high-resolution but its sensitivity is low; radioisotope imaging has high sensitivity but a relatively poor resolution is inevitable. Therefore, single imaging modality cannot offer comprehensive data, multiple imaging combining is expected to enhance cancer imaging systems (Burke et al.,2017).
Nanomaterials offer lots of opportunities to many cancer therapies such as chemotherapy, radiotherapy, photothermal therapy (PTT), photodynamic therapy (PDT), chemodynamic therapy and starving therapy due to their high permeability and retention. Because of the high surface area, nanomaterials can load or co-load a large number of different drugs. Thus nanomaterials are helpful as therapeutic carriers for realizing synergistic therapy such as PTT, PDT. Compared to monotherapy, nanomaterials avoid drug resistance effectively. Scientists made great efforts to combine cancer diagnosis with treatment using nanomaterials (Her et al.,2017).
Applications of Bioresponsive Nanomaterials in Multimodal Imaging Diagnosis
Magnetic Resonance Imaging
MRI is used as a non-invasive imaging method that is especially appropriate for soft tissue based on the property of nuclear magnetic resonance (Harisinghani et al.,2003). After excitation by a selective radio-frequency pulse, the active nuclei will be relaxed rapidly and then return to their initial state in MRI procedure. This relaxation procedure is divided into two parts, i.e., longitudinal relaxation (T1) and transverse relaxation (T2). Both can generate magnetic resonance images for discriminating different tissues. Although MRI is a relatively expensive and time-consuming tool, it has enormous advantages such as high spatial resolution in three-dimensions, high contrast of soft tissues, facile extractions of physiological, anatomical and molecular information (Veiseh et al.,2010). Gadolinium (Gd3+) chelates that behave as paramagnetic complexes are usually used in MRI contrast agents, e.g., Gd-DTPA is widely used in recent years (Tamada et al.,2009). However, repeated injections are needed with high chelates dosage to elongate blood circulation time, which, nevertheless, inevitably brought about some inaccuracies due to the false positive contrast enhancement (Huang et al.,2012).
MRI contrast agents that refer to nanomaterials with uniform size, enhanced relaxation properties and biocompatibility attract scientists’ eyes (Jun et al., 2005; Park et al.,2004). Food and Drug Administration (FDA) approved super paramagnetic iron oxide (SPIO) as a nanoparticle contrast agent in clinical trials (Thorek et al.,2006). SPIO is now widely used in T2 contrast agents. Many nanoparticles such as NiFe2O4, MnFeO4 and CoFe2O4 have also been proved for T2 contrast agents (Khemtong et al.,2011). Nanoparticle Gd-based contrast agents such as Gd2O3, GdPO4 and GdF3 can enhance the signal of T1-weighted MRI (Figure 2) (Jun et al., 2005; Jung et al., 2009; Park et al., 2004; Yin et al., 2012; Zhang et al., 2011). Intriguingly, ultrasmall-sized iron oxide nanoparticles (ESIONs) with sizes less than 4 nm have been proved as good candidates for T1-weighted imaging (Kim et al.,2011). However, these novel nanoparticles are still in the stage of preliminary animal studies in vivo for MRI. There is a long way to before entering human before addressing their biocompatibility and pharmacokinetics concerns (Huang et al.,2012).
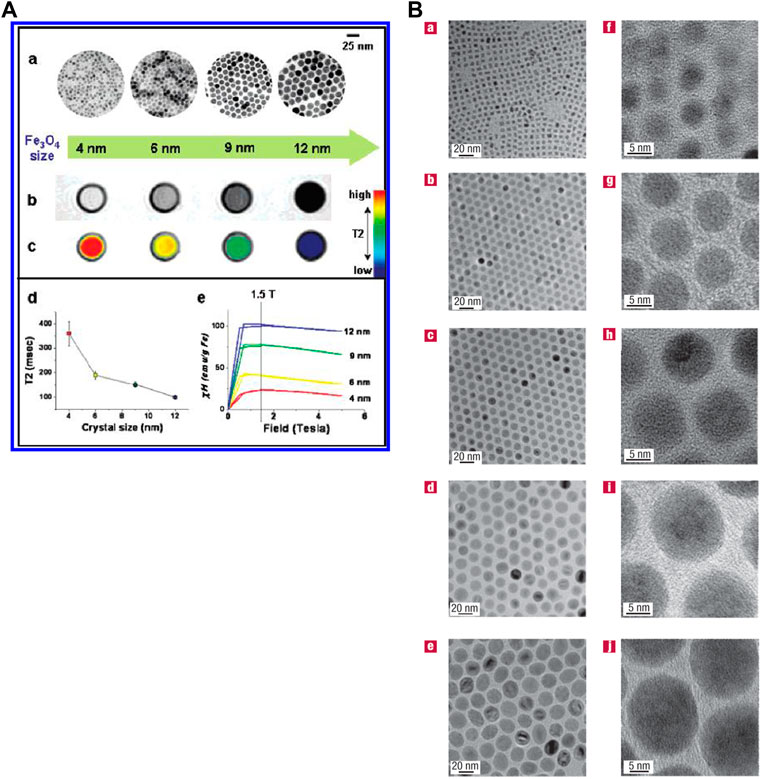
FIGURE 2. (A) Water-soluble Fe3O4 nanocrystals’ size effects on magnetism and MR signals. (B) Transmission electron microscope images of different oleic-Fe3O4 nanoparticles. Reprinted (adapted) with permission from Park et al. (2004). Nat Mater. 2004,3 (12),891-5. Copyright 2004 Nature Publishing Group. Reprinted (adapted) with permission from Jun et al. (2005). J Am Chem Soc. 2005,27,127 (16),5732-3. Copyright 2005 American Chemical Society.
Positron Emission Tomography
PET is a nuclear medicine imaging method, and it can provide quantitative and sensitive readout of an administered radiotracer in order to evaluate the targeting pharmacokinetics and efficiency objective to tissues or organs (Ametamey et al.,2008; Shokeen & Anderson, 2009). Gold nanoparticles are mostly used nanomaterials in PET. Radioiodine-124-labelled tannic acid gold core–shell nanoparticles are used in PET for dendritic cell labelling and tracking, because it shares high radiosensitivity, desirable labelling efficiency and excellent chemical stability. The novel nanoparticles are also good at monitoring cell biological functions such as proliferation and phenotype marker expression (Lee et al.,2017). Results showed that 124I-labelled gold nanostar probes could be used for brain tumors in PET. 124I-labelled gold nanostar probes can reach sub-mm intracranial for brain tumour detection (Liu et al.,2019). Radioactive [64Cu]CuS nanoparticles is also used for PET. Especially when they were conjugated to RGDfK peptides by PEG linkers, they are equipped with a robust ability to target tumor and allowed to be uptaken by tumors. Thus, they can be used to enhance PET for realizing theranostic application (Cui et al.,2018). Very recently, pharmacokinetically-optimized 64Cu-labelled polyglucose nanoparticles (Macrin) were developed for quantitative PET imaging of macrophages (Kim et al.,2018).
Besides above gold and copper sulfide nanoparticles, there are many other nanoparticles that can be used in PET, e.g., 11C, 13N, 15O and 18F are also radioactive contrast agents for PET. Typically, 18F-Macroflor modified polyglucose nanoparticles have a relatively high avidity for macrophages. They are small and excreted renally, thus increasing the PET signal (Keliher et al.,2017).
Photoacoustic Imaging
Based on photoacoustic effect, photoacoustic imaging is a promising branch of ultrasound or optical modalities. Photoacoustic effect is a part of non-ionizing laser pulse which is absorbed by tissues and then converted into heat. Due to transient thermoelastic expansion, there are wideband ultrasonic waves. So photoacoustic imaging is a hybrid biomedical imaging modality. Ultrasonic images can be made by the generated ultrasonic waves in photoacousic imaging (Zhang et al.,2006). It is an excellent method and outperforms optical imaging in visualizing both biological structures and functions due to its penetration depth, prominent contrast and spatial resolution (Figure 3) (Wang et al.,2003; Yao & Wang, 2000).
Some exogenous agents can improve the photoacoustic imaging. Generally, the most important abilities of PAI contrast agents is to convert the light into heat and produce ultrasound waves. Almost all photothermal materials such as carbon, silver, gold, etc., have this ability. After reconstructing, these materials can distinguish cancer tissues from healthy tissues. Gold-based nanomaterials are promising PAI contrast agents because of their localized surface plasmon resonance effect and can convert light into heat efficiently. The surface of gold is inert, determining the biocompatibility in vivo research. Li et al. constructed PEG-HAuNS with both ultrasound and optical properties as contrast agents. This nanomaterial has no acute toxicity in many organs. Its properties in spatial resolution are admirable and good for photoacoustic imaging (Lu et al.,2010). The silver is also known as high surface plasmon resonance. Due to stronger light absorption, silver is theoretically preferable than gold in constructing photoacoustic contrast agent. However, they are more cytotoxic in vivo. So, silver-based nanomaterials demand more studies to address their biocompatibility. Carbon-based materials have high resolution and allow imaging of deep areas, holding high potential in clinical translation. Gambhir et al. constructed single-walled carbon nanotubes with a cyclic Arg-Gly-Asp (RGD) peptide as agents in photoacoustic imaging (De la Zerda et al., 2008).
Cancer Therapy Based on Nanomaterials
Chemotherapy
Chemotherapy is always used in treating metastatic types of cancers (Wang et al.,2017). Doxorubicin (DOX) and paclitaxel are popular chemotherapeutic drugs which can be used in breast cancer. Chemotherapy regimens such as tamoxifen, trastuzumab, docetaxel and cisplatin are also used in chemotherapy (Siddique & Chow, 2020). Nanoparticle-based carriers conjugating with these chemotherapeutic drugs are often used in targeted delivery. For example, nanoparticles based on polymer, metal, mesoporous silica, protein and carbon, are used in chemotherapy (Liyanage et al.,2019). Different kinds of proteins and peptides are combined with nanoparticles to help improve selectivity of chemotherapeutice drugs.
Gold nanoparticles have distinctive characteristics such as multifunctionalities, high stability, high surface plasmon resonance and large surface area-to-volume ratio. Importantly, Au nanoparticles exhibit additional benefits such as nonimmunogenic nature, nontoxic, high permeability and retention effect. Thus Au nanoparticles carrier enabled penetration and accumulation of chemotherapeutic drugs at tumor areas. Typically, EpCAM-RPAnN and DOX-BLM-PEG-Au NPs are Au nanoparticle-based drug delivery systems that can be applied into chemotherapy (Singh et al.,2018). As well, organic nanoparticles are also good choice for drug delivery in chemotherapy since they can increase tumor accumulation of drugs and prolong their circulation half-time. Briefly, nanoparticles can behave as theranostic platform and deliver many drugs to targeted area (Meng et al.,2017).
Radiotherapy
Radiotherapy is also primarily used for various nonmetastatic cancers (Wang et al.,2017). Nanovectorized radiotherapy based on nanoparticles for radionuclides delivery can serve as a reservoir to supply radionuclides to cancer cells specifically. This novel method stimulates immune response and cell killing by using peculiar biomaterials (Vanpouille-Box et al.,2011). Many studies have found that nanomaterials can significantly increase the efficiency of radiotherapy.
Nanomaterials have many advantages, such as high toleration, low toxicity and long circulation time. Some of nanomaterials can even act as radioprotectors when exposed to radioactive substances (Nambiar & Yeow, 2012). Colon et al. indicated that cerium oxide (CeO2) nanoparticles protected the cells from radiation-induced damages both in vivo and in vitro (Colon et al.,2009; Heckert et al.,2008). Schweitzer et al. synthesized melanin-covered nanoparticles and found that they could provide a new way to protect bone marrow from ionizing radiation (Schweitzer et al.,2010). Brown et al. found that fullerene compound DF-1 could serve as a radiation protector. As a radiation protector, DF-1 has modest activity in vivo and could reduce DNA double strand (Brown et al.,2010).
Photothermal Therapy
Photothermal therapy (PTT) is a cancer treatment method based on hyperthermia. It can destroy tumor cells and simultaneously avoid to heat normal areas. Nanomaterials such as nanocages, nanoshells, nanostars and nanocages are mostly used as photothermal transducers. Au nanoparticles and near-infrared (NIR) can be used to selectively and precisely heat tumors (Hirschberg & Madsen, 2017). With rich amine and thiol groups, gold nanoparticles can be used as drug products to realize targeted delivery when combining with antibodies. Colloidal gold always localizes plasmon surface resonance, thus absorbing light with specific wavelengths. In this regard, it is helpful in hyperthermic cancer treatment. As the shape or size of particles change, the localized plasmon surface resonance of gold nanoparticles can also be tuned. Accordingly, the properties of photothermal and photoacoustic can be altered (Vines et al.,2019).
PTT suffers from one major problem that is the heterogeneous heat distribution in tumors, which thus leaves some tumor area untreated and causes incomplete ablation. Silica-coated gold nanoshells are proposed to deliver fractionated PTT (Simon et al.,2019). PTT’s main mediators are gold nanoshells due to their high photothermal conversion efficient, excellent biocompatibility, facile gold thiol bioconjugation chemistry and high tumor penetration. The temperature at the tumor is raised aboveb42°C in order to destroy cancer cells. Therefore, a light-absorbing material should improve the energy selectivity for heat transduction. Although gold is mostly used in PTT, magnetic nanoparticles are good alternatives (Siddique & Chow, 2020). Magnetic nanoparticles can combine with other materials or serve as photothermal agents themselves. They can penetrate into tumor area magnetically and their molar absorption coefficient in NIR is low when used alone. Magnetic nanoparticles can also be used in drug delivery, which enables the marriage of PTT and chemotherapy (Estelrich & Busquets, 2018).
Photodynamic Therapy
Photodynamic therapy (PDT) is a combination of light and photosensitizers. When exposed to light, photosensitizer is able to convert light into reactive oxygen species (ROS) and kill tumor cells through necrosis or apoptosis (Celli et al.,2010). PDT can exert the potent effects to destroy cells and vasculature of the tumor. Because of the light-absorbing photosensitizer’s special and designated localization, the subsequent biological responses can happen in those designated areas (Brown et al.,2004). PDT has potential in clinical cancer treatment with reduced side effects.
Imaging is added as a direct guidance for localizing photosensitizers. Rai et al. integrated PDT with imaging using the NIR-light absorbed photosensitive molecules (Figure 4)(Rai et al.,2021). So far, a variety of nanomaterials have been developed to behave as carriers to enhance PDT such as semiconductor quantum dots, silica nanoparticles, nanocapsules, gold-based nanomaterials and some metal-based nanomaterials (Gary-Bobo et al.,2011; Son et al.,2011). Gold-based nanomaterials have excellent characterisitics for both PDT and optical imaging. Choi et al. proposed a nanomedicine platform consisting of photosensitizer AlPcS4 and gold nanorods (Jang et al.,2011). Based on this nanoplatform, tumor areas can be discerned by NIR fluorescence imaging, and then dual PDT and PTT was carried out effectively ablate them (Figure 5) (Jang et al.,2011). Wang et al. used photosensitizer Chlorine6 (Ce6) to conjugate with NIR light-excited upconversion nanoparticles (UCNPs) for tumor therapy (Wang et al.,2011).
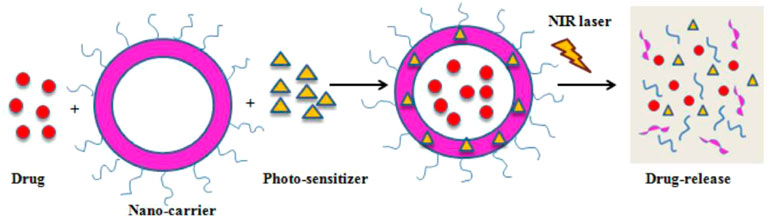
FIGURE 4. A schematic illustration of PDT-mediated drug release. Reprinted (adapted) with permission from Rai et al. (2021). Medicina (Kaunas). 2021,57 (2),91. Copyright 2021 by the authors.
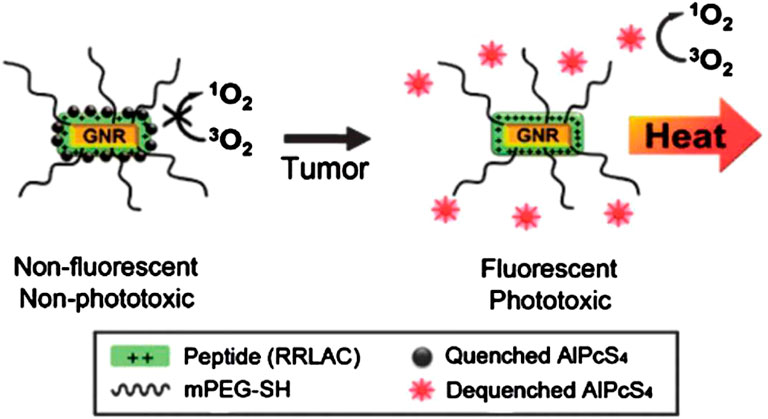
FIGURE 5. A schematic diagram of the gold nanorods which conjugated with AlPcS4 for simultaneous NIR optical imaging and phototherapy. Reprinted (adapted) with permission from Jang et al. (2011). ACS Nano. 2011,22,5 (2),1,086-94. Copyright 2011 American Chemical Society.
Nanomaterials’ Theranostics Application in Multimodality Imaging, Image-Guided Therapy and Combination Therapy
Multimodal imaging that uses nanomaterials can combine their personal advantages and also overcome limitations of each single imaging modality (Rosenkrans et al.,2021). PET-MRI combination perfectly integrates high sensitivity of PET with the excellent spatial resolution and contrast of soft tissue in MRI. SPIONs, Feraheme (FH) and [89Zr]Zr were used for PET-MRI as an integral nanoplatform (Gholami et al.,2020), wherein FH shorten T2 transverse relaxation time for negative contrast enhancement. In addition, 68Ga-AGulX@NODAGA showed high potential in PET/MRI-guided radiotherapy (Thomas et al.,2017). They not only behave as a dual-modal imaging agent, but also serve as an interstatial radiotherapy agent which can accumulate in the targeted diseased area (Thomas et al.,2017).
Imaging-guided radiotherapy is effective in treating cancer (Chow and Albayedh, 2018; Martelli & Chow, 2020). Combination of gadolinium and bismuth as a theranostic platform can be used for onsite radiosensitization and contrast enhancement. As well, there are other candidates for combination therapy, e.g., mesoporous silica nanoparticles can serve as CT and optical imaging agents. The high density of platinum nanoparticles could significantly enhance CT contrast, and nontoxic Co2+ ions were also used as radioactive tracers (Pham et al.,2017).
Combination therapy can cause synergistic actions and work against drug resistance. Several nanoparticles can be used in combined therapy. Liposomes that are established as drug delivery vehicles have various clinical products. Polymeric nanoparticles are also used in combination therapy, and they can be used to deliver site-specific anticancers to tumors. Other nanoparticles such as dendrimers, carbon nanoparticles, metallic nanoparticles and nanodiamonds can be used in combination therapy (Gurunathan et al.,2018).
Chemotherapy is a common cancer treatment method. However, it shows off-target effect and cause toxicity in normal issues. Almost 90% of cancer treatment failures is attributed to the chemoresistance. Some tumor cells such as cancer stem cells or progenitor cells have potent radioresistance against many chemotherapeutic agents. Inspiringly, PTT combination with chemotherapy arouses an effect of triggering powerful antitumor immunity. Especially, PTT combination with immunotherapy can further minimize the risk of metastasis or recurrence. As the paradigms, polydopamine-coated spiky Au nanoparticles have high photothermal stability and can be used in PTT-chemotherapy; and polydopamine-coated AI2O3 nanoparticles can be used for PTT-immunotherapy (Chen et al., 2018). Fluorescence imaging has high resolution and sensitivity. It is real-time imaging capture and is suitable for diagnosing tumors (Zhong, 2009). Chemodynamic therapy is a specific tumor treatment and can make tumor cells sensitive to chemotherapy drugs thus improving the chemo therapy effect (Wang et al.,2018). Recent study indicated that a novel theranostics nanozymes can be used for both fluorescence imaging and enhancing chemo-chemodynamic therapy (Figure 6) (Zhao et al.,2021).
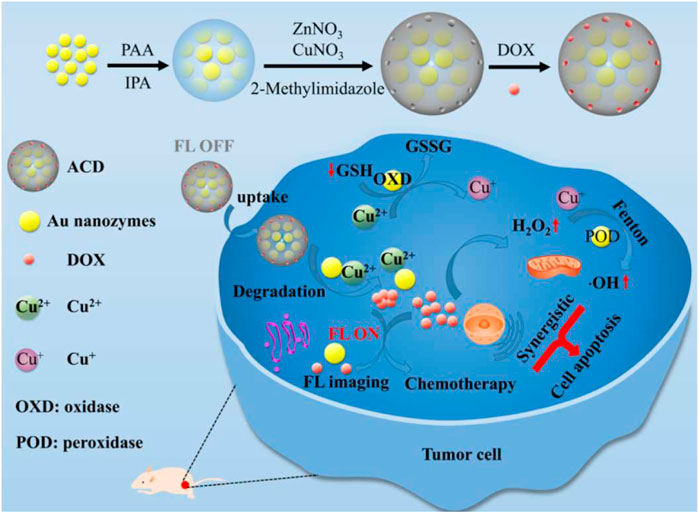
FIGURE 6. A schematic diagram of fabrication procedure of ACD (ACD, A: Aunanoclusters, C: copper ions, D: DOX) and its use for both fluorescence imaging and enhancing chemo-chemodynamic therapy. Reprinted (adapted) with permission from Zhao et al. (2021). ACS Appl Mater Interfaces. 2021,1,13 (47):55,780-55,789. Copyright 2021 American Chemical Society.
Conclusion and Perspectives
Nanomaterials are useful tool for cancer diagnosis and therapy. Due to their excellent cellular uptake, targeted drug delivery, high drug loading, prolonged circulation time, high surface to volume ratio, good permeability and retention effect, nanomaterials can enhance the therapeutic effect and reduce the side effects at the same time. In this review, we overviewed different kinds of nanomaterials and explained the latest research on the use of bioresponsive nanomaterials for cancer imaging modalities (magnetic resonance imaging, positron emission tomography and photoacoustic imaging) and imaging-guided therapy (chemotherapy, radiotherapy, photothermal therapy and photodynamic therapy). Nanomaterials are also promising labeling agents for biosensing. Fluorescence-based detection is always used in biosensing due to its diversity, simplicity and sensitivity. Nanomaterials can offer superior optical and acoustic properties, e.g., bright fluorescence and robust acoustic scattering, which can be engineered to realize multiple treatments such as sonodynamic therapy (SDT) (Zhang et al., 2021).
In last few decades, nanomaterials were conjugated with different membranes and biomaterials in order to improve biocompatibility and overcome drug resistance which were observed in cancer treatment commonly. Despite all advantages, it is still a great challenge to use nanomaterials in clinical trials because of their unknown toxicity, cytotoxicity, biocompatibility, in vivo targeting efficiency, physicochemical properties, production costs and so on. Novel nanoenzyme is a catalytic nanomaterial with enzymatic properties. It can be used for fluorescence imaging and enhanced chemo-chemodynamic therapy of tumors (Lee et al., 2022). It is still expected that new developments of nanotechnology can happen in nanomaterials in clinics in the near future.
Author Contributions
ZZ and HG contributed equally to this work, and then co-wrote this manuscript. CC sorted the references, and LX and KZ revised manuscript. All authors commented this manuscript.
Funding
We acknowledge the support from National Natural Science Foundation of China (Grant No. 82022033, 81771836), the Fundamental Research Funds for the Central Universities (22120210561), Shanghai Rising-Star Program (Grant No. 19QA1406800) and Shanghai Talent Development Fund (Grant No. 2019040) and Shanghai Young Top-Notch Talent.
Conflict of Interest
The authors declare that the research was conducted in the absence of any commercial or financial relationships that could be construed as a potential conflict of interest.
Publisher’s Note
All claims expressed in this article are solely those of the authors and do not necessarily represent those of their affiliated organizations, or those of the publisher, the editors, and the reviewers. Any product that may be evaluated in this article, or claim that may be made by its manufacturer, is not guaranteed or endorsed by the publisher.
References
Ametamey, S. M., Honer, M., and Schubiger, P. A. (2008). Molecular Imaging with PET. Chem. Rev. 108, 1501–1516. doi:10.1021/cr0782426
Bae, K. H., Lee, Y., and Park, T. G. (2007). Oil-Encapsulating PEO−PPO−PEO/PEG Shell Cross-Linked Nanocapsules for Target-specific Delivery of Paclitaxel. Biomacromolecules 8, 650–656. doi:10.1021/bm0608939
Barreto, J. A., O’Malley, W., Kubeil, M., Graham, B., Stephan, H., and Spiccia, L. (2011). Nanomaterials: Applications in Cancer Imaging and Therapy. Adv. Mater. 23, H18–H40. doi:10.1002/adma.201100140
Bartlett, D. W., and Davis, M. E. (2007). Physicochemical and Biological Characterization of Targeted, Nucleic Acid-Containing Nanoparticles. Bioconjug. Chem. 18, 456–468. doi:10.1021/bc0603539
Brown, A. P., Chung, E. J., Urick, M. E., Shield, W. P., Sowers, A. L., Thetford, A., et al. (2010). Evaluation of the Fullerene Compound DF-1 as a Radiation Protector. Radiat. Oncol. 5, 34. doi:10.1186/1748-717X-5-34
Brown, S. B., Brown, E. A., and Walker, I. (2004). The Present and Future Role of Photodynamic Therapy in Cancer Treatment. Lancet Oncol. 5, 497–508. doi:10.1016/S1470-2045(04)01529-3
Burke, B. P., Cawthorne, C., and Archibald, S. J. (2017). Multimodal Nanoparticle Imaging Agents: Design and Applications. Phil. Trans. R. Soc. A. 375, 20170261. doi:10.1098/rsta.2017.0261
Celli, J. P., Spring, B. Q., Rizvi, I., Evans, C. L., Samkoe, K. S., Verma, S., et al. (2010). Imaging and Photodynamic Therapy: Mechanisms, Monitoring, and Optimization. Chem. Rev. 110, 2795–2838. doi:10.1021/cr900300p
Chen, C., Wang, S., Li, L., Wang, P., Chen, C., Sun, Z., et al. (2016). Bacterial Magnetic Nanoparticles for Photothermal Therapy of Cancer under the Guidance of MRI. Biomaterials 104, 352–360. doi:10.1016/j.biomaterials.2016.07.030
Chen, W., Qin, M., Chen, X., Wang, Q., Zhang, Z., and Sun, X. (2018). Combining Photothermal Therapy and Immunotherapy against Melanoma by Polydopamine-Coated Al2O3 Nanoparticles. Theranostics 8, 2229–2241. doi:10.7150/thno.24073
Chow, J. L., and Albayedh, F. (2018). Monte Carlo Simulation on the Imaging Contrast Enhancement in Nanoparticle-Enhanced Radiotherapy. J. Med. Phys. 43, 195–199. doi:10.4103/jmp.JMP_141_17
Colon, J., Herrera, L., Smith, J., Patil, S., Komanski, C., Kupelian, P., et al. (2009). Protection from Radiation-Induced Pneumonitis Using Cerium Oxide Nanoparticles. Nanomedicine: Nanotechnology, Biol. Med. 5, 225–231. doi:10.1016/j.nano.2008.10.003
Couvreur, P., and Vauthier, C. (2006). Nanotechnology: Intelligent Design to Treat Complex Disease. Pharm. Res. 23, 1417–1450. doi:10.1007/s11095-006-0284-8
Cui, L., Xiong, C., Zhou, M., Shi, S., Chow, D. S.-L., and Li, C. (2018). Integrin αvβ3-Targeted [64Cu]CuS Nanoparticles for PET/CT Imaging and Photothermal Ablation Therapy. Bioconjug. Chem. 29, 4062–4071. doi:10.1021/acs.bioconjchem.8b00690
Davis, M. E., Chen, Z., and Shin, D. M. (2008). Nanoparticle Therapeutics: an Emerging Treatment Modality for Cancer. Nat. Rev. Drug Discov. 7, 771–782. doi:10.1038/nrd2614
De la Zerda, A., Zavaleta, C., Keren, S., Vaithilingam, S., Bodapati, S., Liu, Z., et al. (2008). Carbon Nanotubes as Photoacoustic Molecular Imaging Agents in Living Mice. Nat. Nanotech 3, 557–562. doi:10.1038/nnano.2008.231
Duncan, R. (2003). The Dawning Era of Polymer Therapeutics. Nat. Rev. Drug Discov. 2, 347–360. doi:10.1038/nrd1088
Estelrich, J., and Busquets, M. (2018). Iron Oxide Nanoparticles in Photothermal Therapy. Molecules 23, 1567. doi:10.3390/molecules23071567
Gary-Bobo, M., Mir, Y., Rouxel, C., Brevet, D., Basile, I., Maynadier, M., et al. (2011). Mannose-functionalized Mesoporous Silica Nanoparticles for Efficient Two-Photon Photodynamic Therapy of Solid Tumors. Angew. Chem. Int. Ed. 50, 11425–11429. doi:10.1002/anie.201104765
Gholami, Y. H., Yuan, H., Wilks, M. Q., Maschmeyer, R., Normandin, M. D., Josephson, L., et al. (2020). A Radio-Nano-Platform for T1/T2 Dual-Mode PET-MR Imaging. Int. J. Nanomedicine 15, 1253–1266. doi:10.2147/IJN.S241971
Goldberg, M., Langer, R., and Jia, X. (2007). Nanostructured Materials for Applications in Drug Delivery and Tissue Engineering. J. Biomater. Sci. Polym. Edition 18, 241–268. doi:10.1163/156856207779996931
Gurunathan, S., Kang, M.-H., Qasim, M., and Kim, J.-H. (2018). Nanoparticle-Mediated Combination Therapy: Two-In-One Approach for Cancer. Int. J. Mol. Sci. 19, 3264. doi:10.3390/ijms19103264
Harisinghani, M. G., Barentsz, J., Hahn, P. F., Deserno, W. M., Tabatabaei, S., van de Kaa, C. H., et al. (2003). Noninvasive Detection of Clinically Occult Lymph-Node Metastases in Prostate Cancer. N. Engl. J. Med. 348, 2491–2499. doi:10.1056/NEJMoa022749
Heckert, E. G., Karakoti, A. S., Seal, S., and Self, W. T. (2008). The Role of Cerium Redox State in the SOD Mimetic Activity of Nanoceria. Biomaterials 29, 2705–2709. doi:10.1016/j.biomaterials.2008.03.014
Her, S., Jaffray, D. A., and Allen, C. (2017). Gold Nanoparticles for Applications in Cancer Radiotherapy: Mechanisms and Recent Advancements. Adv. Drug Deliv. Rev. 109, 84–101. doi:10.1016/j.addr.2015.12.012
Hirschberg, H., and Madsen, S. J. (2017). Cell Mediated Photothermal Therapy of Brain Tumors. J. Neuroimmune Pharmacol. 12, 99–106. doi:10.1007/s11481-016-9690-9
Holohan, C., Van Schaeybroeck, S., Longley, D. B., and Johnston, P. G. (2013). Cancer Drug Resistance: an Evolving Paradigm. Nat. Rev. Cancer 13, 714–726. doi:10.1038/nrc3599
Huang, Y., He, S., Cao, W., Cai, K., and Liang, X.-J. (2012). Biomedical Nanomaterials for Imaging-Guided Cancer Therapy. Nanoscale 4, 6135–6149. doi:10.1039/c2nr31715j
Hull, L. C., Farrell, D., and Grodzinski, P. (2014). Highlights of Recent Developments and Trends in Cancer Nanotechnology Research-View from NCI Alliance for Nanotechnology in Cancer. Biotechnol. Adv. 32, 666–678. doi:10.1016/j.biotechadv.2013.08.003
Husband, J. E., and Reznek, R. H. (2000). Cancer Imaging 2000: Principles, Strategies, Challenges. Cancer Imaging 1, 1–4. doi:10.1102/1470-7330/00/010001+04
Jang, B., Park, J.-Y., Tung, C.-H., Kim, I.-H., and Choi, Y. (2011). Gold Nanorod−Photosensitizer Complex for Near-Infrared Fluorescence Imaging and Photodynamic/Photothermal Therapy In Vivo. ACS Nano 5, 1086–1094. doi:10.1021/nn102722z
Jun, Y.-w., Huh, Y.-M., Choi, J.-s., Lee, J.-H., Song, H.-T., Kim, S., et al. (2005). Nanoscale Size Effect of Magnetic Nanocrystals and Their Utilization for Cancer Diagnosis via Magnetic Resonance Imaging. J. Am. Chem. Soc. 127, 5732–5733. doi:10.1021/ja0422155
Jung, J. Y., Kwon, J. H., Kim, J. H., Song, H. H., Kim, I., Lee, K. S., et al. (2009). Phase II Study of the Paclitaxel, Cisplatin, 5-fluorouracil and Leucovorin (TPFL) Regimen in the Treatment of Advanced or Metastatic Gastric Cancer. Oncol. Rep. 21, 523–529.
Keliher, E. J., Ye, Y.-X., Wojtkiewicz, G. R., Aguirre, A. D., Tricot, B., Senders, M. L., et al. (2017). Polyglucose Nanoparticles with Renal Elimination and Macrophage Avidity Facilitate PET Imaging in Ischaemic Heart Disease. Nat. Commun. 8, 14064. doi:10.1038/ncomms14064
Khemtong, C., Togao, O., Ren, J., Kessinger, C. W., Takahashi, M., Sherry, A. D., et al. (2011). Off-resonance Saturation MRI of Superparamagnetic Nanoprobes: Theoretical Models and Experimental Validations. J. Magn. Reson. 209, 53–60. doi:10.1016/j.jmr.2010.12.013
Kim, B. H., Lee, N., Kim, H., An, K., Park, Y. I., Choi, Y., et al. (2011). Large-scale Synthesis of Uniform and Extremely Small-Sized Iron Oxide Nanoparticles for High-Resolution T1 Magnetic Resonance Imaging Contrast Agents. J. Am. Chem. Soc. 133, 12624–12631. doi:10.1021/ja203340u
Kim, H.-Y., Li, R., Ng, T. S. C., Courties, G., Rodell, C. B., Prytyskach, M., et al. (2018). Quantitative Imaging of Tumor-Associated Macrophages and Their Response to Therapy Using 64Cu-Labeled Macrin. ACS Nano 12, 12015–12029. doi:10.1021/acsnano.8b04338
Lee, A. L. Z., Wang, Y., Cheng, H. Y., Pervaiz, S., and Yang, Y. Y. (2009). The Co-delivery of Paclitaxel and Herceptin Using Cationic Micellar Nanoparticles. Biomaterials 30, 919–927. doi:10.1016/j.biomaterials.2008.10.062
Lee, J., Liao, H., Wang, Q., Han, J., Han, J. H., ShinGe, H. E. M., et al. (2022). Exploration of Nanozymes in Viral Diagnosis and Therapy. Exploration 2, 20210086. doi:10.1002/EXP.20210086
Lee, S. B., Lee, S.-W., Jeong, S. Y., Yoon, G., Cho, S. J., Kim, S. K., et al. (2017). Engineering of Radioiodine-Labeled Gold Core-Shell Nanoparticles as Efficient Nuclear Medicine Imaging Agents for Trafficking of Dendritic Cells. ACS Appl. Mater. Inter. 9, 8480–8489. doi:10.1021/acsami.6b14800
Liu, Y., Carpenter, A. B., Pirozzi, C. J., Yuan, H., Waitkus, M. S., Zhou, Z., et al. (2019). Non-invasive Sensitive Brain Tumor Detection Using Dual-Modality Bioimaging Nanoprobe. Nanotechnology 30, 275101. doi:10.1088/1361-6528/ab0e9c
Liyanage, P. Y., Hettiarachchi, S. D., Zhou, Y., Ouhtit, A., Seven, E. S., Oztan, C. Y., et al. (2019). Nanoparticle-mediated Targeted Drug Delivery for Breast Cancer Treatment. Biochim. Biophys. Acta (Bba) - Rev. Cancer 1871, 419–433. doi:10.1016/j.bbcan.2019.04.006
Lu, W., Huang, Q., Ku, G., Wen, X., Zhou, M., Guzatov, D., et al. (2010). Photoacoustic Imaging of Living Mouse Brain Vasculature Using Hollow Gold Nanospheres. Biomaterials 31, 2617–2626. doi:10.1016/j.biomaterials.2009.12.007
Mahasneh, A., Al-Shaheri, F., and Jamal, E. (2017). Molecular Biomarkers for an Early Diagnosis, Effective Treatment and Prognosis of Colorectal Cancer: Current Updates. Exp. Mol. Pathol. 102, 475–483. doi:10.1016/j.yexmp.2017.05.005
Martelli, S., and Chow, J. C. L. (2020). Dose Enhancement for the Flattening-filter-free and Flattening-Filter Photon Beams in Nanoparticle-Enhanced Radiotherapy: A Monte Carlo Phantom Study. Nanomaterials 10, 637. doi:10.3390/nano10040637
Mehta, M., Deeksha,, , Sharma, N., Vyas, M., Khurana, N., Maurya, P. K., et al. (2019). Interactions with the Macrophages: An Emerging Targeted Approach Using Novel Drug Delivery Systems in Respiratory Diseases. Chemico-Biological Interactions 304, 10–19. doi:10.1016/j.cbi.2019.02.021
Meng, F., Han, N., and Yeo, Y. (2017). Organic Nanoparticle Systems for Spatiotemporal Control of Multimodal Chemotherapy. Expert Opin. Drug Deliv. 14, 427–446. doi:10.1080/17425247.2016.1218464
Nambiar, S., and Yeow, J. T. W. (2012). Polymer-composite Materials for Radiation protection. ACS Appl. Mater. Inter. 4, 5717–5726. doi:10.1021/am300783d
Park, J., An, K., Hwang, Y., Park, J.-G., Noh, H.-J., Kim, J.-Y., et al. (2004). Ultra-large-scale Syntheses of Monodisperse Nanocrystals. Nat. Mater 3, 891–895. doi:10.1038/nmat1251
Pham, T., Lengkeek, N., Greguric, I., Kim, B., Pellegrini, P., Bickley, S., et al. (2017). Tunable and Noncytotoxic PET/SPECT-MRI Multimodality Imaging Probes Using Colloidally Stable Ligand-free Superparamagnetic Iron Oxide Nanoparticles. Int. J. Nanomedicine 12, 899–909. doi:10.2147/IJN.S127171
Rai, A., Noor, S., Ahmad, S. I., Alajmi, M. F., Hussain, A., Abbas, H., et al. (2021). Recent Advances and Implication of Bioengineered Nanomaterials in Cancer Theranostics. Medicina 57, 91. doi:10.3390/medicina57020091
Rosado-de-Castro, P. H., Morales, M. d. P., Pimentel-Coelho, P. M., Mendez-Otero, R., and Herranz, F. (2018). Development and Application of Nanoparticles in Biomedical Imaging. Contrast Media Mol. Imaging 2018, 1–2. doi:10.1155/2018/1403826
Rosenkrans, Z. T., Ferreira, C. A., Ni, D., and Cai, W. (2021). Internally Responsive Nanomaterials for Activatable Multimodal Imaging of Cancer. Adv. Healthc. Mater. 10, 2000690. doi:10.1002/adhm.202000690
Schweitzer, A. D., Revskaya, E., Chu, P., Pazo, V., Friedman, M., Nosanchuk, J. D., et al. (2010). Melanin-covered Nanoparticles for protection of Bone Marrow during Radiation Therapy of Cancer. Int. J. Radiat. Oncology*Biology*Physics 78, 1494–1502. doi:10.1016/j.ijrobp.2010.02.020
Shapira, A., Livney, Y. D., Broxterman, H. J., and Assaraf, Y. G. (2011). Nanomedicine for Targeted Cancer Therapy: towards the Overcoming of Drug Resistance. Drug Resist. Updates 14, 150–163. doi:10.1016/j.drup.2011.01.003
Shokeen, M., and Anderson, C. J. (2009). Molecular Imaging of Cancer with Copper-64 Radiopharmaceuticals and Positron Emission Tomography (PET). Acc. Chem. Res. 42, 832–841. doi:10.1021/ar800255q
Siddique, S., and Chow, J. C. L. (2020). Application of Nanomaterials in Biomedical Imaging and Cancer Therapy. Nanomaterials 10, 1700. doi:10.3390/nano10091700
Simón, M., Nørregaard, K., Jørgensen, J. T., Oddershede, L. B., and Kjaer, A. (2019). Fractionated Photothermal Therapy in a Murine Tumor Model: Comparison with Single Dose. Int. J. Nanomedicine 14, 5369–5379. doi:10.2147/IJN.S205409
Singh, P., Pandit, S., Mokkapati, V. R. S. S., Garg, A., Ravikumar, V., and Mijakovic, I. (2018). Gold Nanoparticles in Diagnostics and Therapeutics for Human Cancer. Int. J. Mol. Sci. 19, 1979. doi:10.3390/ijms19071979
Son, K. J., Yoon, H.-J., Kim, J.-H., Jang, W.-D., Lee, Y., and Koh, W.-G. (2011). Photosensitizing Hollow Nanocapsules for Combination Cancer Therapy. Angew. Chem. Int. Ed. 50, 11968–11971. doi:10.1002/anie.201102658
Tamada, T., Ito, K., Sone, T., Yamamoto, A., Yoshida, K., Kakuba, K., et al. (2009). Dynamic Contrast-Enhanced Magnetic Resonance Imaging of Abdominal Solid Organ and Major Vessel: Comparison of Enhancement Effect between Gd-EOB-DTPA and Gd-DTPA. J. Magn. Reson. Imaging 29, 636–640. doi:10.1002/jmri.21689
Tan, Y. Y., Yap, P. K., Xin Lim, G. L., Mehta, M., Chan, Y., Ng, S. W., et al. (2020). Perspectives and Advancements in the Design of Nanomaterials for Targeted Cancer Theranostics. Chemico-Biological Interactions 329, 109221. doi:10.1016/j.cbi.2020.109221
Thomas, E., Colombeau, L., Gries, M., Peterlini, T., Mathieu, C., Thomas, N., et al. (2017). Ultrasmall AGuIX Theranostic Nanoparticles for Vascular-Targeted Interstitial Photodynamic Therapy of Glioblastoma. Int. J. Nanomedicine 12, 7075–7088. doi:10.2147/IJN.S141559
Thorek, D. L. J., Chen, A. K., Czupryna, J., and Tsourkas, A. (2006). Superparamagnetic Iron Oxide Nanoparticle Probes for Molecular Imaging. Ann. Biomed. Eng. 34, 23–38. doi:10.1007/s10439-005-9002-7
Tran, S., DeGiovanni, P. J., Piel, B., and Rai, P. (2017). Cancer Nanomedicine: a Review of Recent success in Drug Delivery. Clin. Translational Med. 6, 44. doi:10.1186/s40169-017-0175-0
Turner, N. C., and Reis-Filho, J. S. (2012). Genetic Heterogeneity and Cancer Drug Resistance. Lancet Oncol. 13, e178–e185. doi:10.1016/S1470-2045(11)70335-7
Vanpouille-Box, C., Lacoeuille, F., Roux, J., Aubé, C., Garcion, E., Lepareur, N., et al. (2011). Lipid Nanocapsules Loaded with Rhenium-188 Reduce Tumor Progression in a Rat Hepatocellular Carcinoma Model. PLoS One 6, e16926. doi:10.1371/journal.pone.0016926
Veiseh, O., Gunn, J. W., and Zhang, M. (2010). Design and Fabrication of Magnetic Nanoparticles for Targeted Drug Delivery and Imaging. Adv. Drug Deliv. Rev. 62, 284–304. doi:10.1016/j.addr.2009.11.002
Vines, J. B., Yoon, J.-H., Ryu, N.-E., Lim, D.-J., and Park, H. (2019). Gold Nanoparticles for Photothermal Cancer Therapy. Front. Chem. 7, 167. doi:10.3389/fchem.2019.00167
Vogelstein, B., Papadopoulos, N., Velculescu, V. E., Zhou, S., Diaz, L. A., and Kinzler, K. W. (2013). Cancer Genome Landscapes. Science 339, 1546–1558. doi:10.1126/science.1235122
Wang, C., Tao, H., Cheng, L., and Liu, Z. (2011). Near-infrared Light Induced In Vivo Photodynamic Therapy of Cancer Based on Upconversion Nanoparticles. Biomaterials 32, 6145–6154. doi:10.1016/j.biomaterials.2011.05.007
Wang, H., Gao, Z., Liu, X., Agarwal, P., Zhao, S., Conroy, D. W., et al. (2018). Targeted Production of Reactive Oxygen Species in Mitochondria to Overcome Cancer Drug Resistance. Nat. Commun. 9, 562. doi:10.1038/s41467-018-02915-8
Wang, J., Li, Y., Nie, G., and Zhao, Y. (2019). Precise Design of Nanomedicines: Perspectives for Cancer Treatment. Natl. Sci. Rev. 6, 1107–1110. doi:10.1093/nsr/nwz012
Wang, X., Pang, Y., Ku, G., Xie, X., Stoica, G., and Wang, L. V. (2003). Noninvasive Laser-Induced Photoacoustic Tomography for Structural and Functional In Vivo Imaging of the Brain. Nat. Biotechnol. 21, 803–806. doi:10.1038/nbt839
Wang, Y., Li, P., Chen, F., Jia, L., Xu, Q., Gai, X., et al. (2017). A Novel pH-Sensitive Carrier for the Delivery of Antitumor Drugs: Histidine-Modified Auricularia Auricular Polysaccharide Nano-Micelles. Sci. Rep. 7, 4751. doi:10.1038/s41598-017-04428-8
Wei, W., Rosenkrans, Z. T., Luo, Q.-Y., Lan, X., and Cai, W. (2019). Exploiting Nanomaterial-Mediated Autophagy for Cancer Therapy. Small Methods 3, 1800365. doi:10.1002/smtd.201800365
Yao, G., and Wang, L. V. (2000). Theoretical and Experimental Studies of Ultrasound-Modulated Optical Tomography in Biological Tissue. Appl. Opt. 39, 659–664. doi:10.1364/ao.39.000659
Yin, W., Zhao, L., Zhou, L., Gu, Z., Liu, X., Tian, G., et al. (2012). Enhanced Red Emission from GdF3:Yb3+,Er3+ Upconversion Nanocrystals by Li+ Doping and Their Application for Bioimaging. Chem. Eur. J. 18, 9239–9245. doi:10.1002/chem.201201053
Yoo, H. S., Lee, K. H., Oh, J. E., and Park, T. G. (2000). In Vitro and In Vivo Anti-tumor Activities of Nanoparticles Based on Doxorubicin-PLGA Conjugates. J. Controlled Release 68, 419–431. doi:10.1016/s0168-3659(00)00280-7
Zhang, H. F., Maslov, K., Stoica, G., and Wang, L. V. (2006). Imaging Acute thermal burns by Photoacoustic Microscopy. J. Biomed. Opt. 11, 054033. doi:10.1117/1.2355667
Zhang, H., Pan, X., Wu, Q., Guo, J., Wang, C., and Liu, H. (2021). Manganese Carbonate Nanoparticles‐mediated Mitochondrial Dysfunction for Enhanced Sonodynamic Therapy. Exploration 1, 20210010. doi:10.1002/EXP.20210010
Zhang, L., Yin, M., You, H., Yang, M., Song, Y., and Huang, Y. (2011). Mutifuntional GdPO4:Eu3+ Hollow Spheres: Synthesis and Magnetic and Luminescent Properties. Inorg. Chem. 50, 10608–10613. doi:10.1021/ic200867a
Zhang, Y., Zhang, L., Gao, J., and Wen, L. (2019). Pro-Death or Pro-survival: Contrasting Paradigms on Nanomaterial-Induced Autophagy and Exploitations for Cancer Therapy. Acc. Chem. Res. 52, 3164–3176. doi:10.1021/acs.accounts.9b00397
Zhao, D.-H., Li, C.-Q., Hou, X.-L., Xie, X.-T., Zhang, B., Wu, G.-Y., et al. (2021). Tumor Microenvironment-Activated Theranostics Nanozymes for Fluorescence Imaging and Enhanced Chemo-Chemodynamic Therapy of Tumors. ACS Appl. Mater. Inter. 13, 55780–55789. doi:10.1021/acsami.1c12611
Keywords: nanomaterials, cancer, imaging, photothemal, photodynamic therapy
Citation: Zeng Z, Gao H, Chen C, Xiao L and Zhang K (2022) Bioresponsive Nanomaterials: Recent Advances in Cancer Multimodal Imaging and Imaging-Guided Therapy. Front. Chem. 10:881812. doi: 10.3389/fchem.2022.881812
Received: 23 February 2022; Accepted: 04 March 2022;
Published: 18 March 2022.
Edited by:
Dalong Ni, Shanghai Jiao Tong University, ChinaReviewed by:
Chengcheng Niu, Central South University, ChinaYang Sun, Second Affiliated Hospital of Chongqing Medical University, China
Copyright © 2022 Zeng, Gao, Chen, Xiao and Zhang. This is an open-access article distributed under the terms of the Creative Commons Attribution License (CC BY). The use, distribution or reproduction in other forums is permitted, provided the original author(s) and the copyright owner(s) are credited and that the original publication in this journal is cited, in accordance with accepted academic practice. No use, distribution or reproduction is permitted which does not comply with these terms.
*Correspondence: Lianbo Xiao, MTM3MDE4ODgxNzhAMTYzLmNvbQ==; Kun Zhang, emhhbmcxOTg2a3VuQDEyNi5jb20=
†These authors have contributed equally to this work