- 1Hubei Key Laboratory of Plasma Chemistry and Advanced Materials, School of Materials Science and Engineering, Wuhan Institute of Technology, Wuhan, China
- 2Department of Food Science and Chemical Engineering, Hubei University of Arts and Science, Xiangyang, China
Developing efficient catalysts is vital for the application of electrochemical sensors. Metal–organic frameworks (MOFs), with high porosity, large specific surface area, good conductivity, and biocompatibility, have been widely used in catalysis, adsorption, separation, and energy storage applications. In this invited review, the recent advances of a novel MOF-based catalysts in electrochemical sensors are summarized. Based on the structure–activity–performance relationship of MOF-based catalysts, their mechanism as electrochemical sensor, including metal cations, synthetic ligands, and structure, are introduced. Then, the MOF-based composites are successively divided into metal-based, carbon-based, and other MOF-based composites. Furthermore, their application in environmental monitoring, food safety control, and clinical diagnosis is discussed. The perspective and challenges for advanced MOF-based composites are proposed at the end of this contribution.
Introduction
With the development of science and technology, the demand for substance detection is becoming more selective (Sharma and Mutharasan, 2013). Sensors can effectively respond to an electrical, optical, or other signal in the presence of the analyte, and then, it will convert the physical parameters to complete the detection of the substance (Justino et al., 2015). The sensor system includes an identification element, a sensor, and a detector, which can be divided into several sensors (Chiu et al., 2017; Gogotsi et al., 2017; Zhou et al., 2017; Li S. et al., 2018; Shankar et al., 2018; Liu et al., 2021; Lin et al., 2022; Lu et al., 2022; Zamzami et al., 2022). Among them, electrochemical sensors, with simplicity, strong selectivity, and high sensitivity, have attracted wide attention (Yang S et al., 2021). Recently, nanomaterials with the advantages of high specific surface area, excellent catalytic performance, conductivity, and biocompatibility, can help the electrochemical sensors amplify signals and improve the sensitivity of the sensors as well as reduce the detection range (Yang Q et al., 2017). An efficient electrochemical sensor requires two requirements: high specificity of the signal tag and an electrode with superior sensitivity and stability (Yang ZH et al., 2017; Yang et al., 2022).
Metal–organic frameworks (MOFs) are known as coordination polymer networks or porous coordination polymers (Rosi, 2003; Zhang and Lin, 2014). The structure of different target molecules can be designed by selecting metal coordination nodes and organic junctions. Importantly, a metal-center (e.g., electrical, catalytic, or magnetic), an organic ligand (e.g., luminescent, fluorescent, or chiral), or a combination of both may produce a universal framework function that exceeds the accessible porosity (Liu et al., 2018). However, the slow mass transfer, low conductivity, and instable structure of MOF as catalysts limit their practical application (Ma and Zhu, 2020). MOF-based composites present higher surface area and richer active sites and exhibit highly ordered pore-like arrangement, which can expose active sites to a greater extent and make them have higher catalytic activity (Qin et al., 2018; Ma et al., 2020a; Ma et al., 2020b).
Recently, different effective strategies have been proposed to modify MOFs for improved electrocatalytic behavior, mechanical properties, and stability. Generally, metal nanoparticles, with the advantages of superior conductivity and high surface area, can be used to increase the electron transfer rate (Samadi-Maybodi et al., 2015; Da Silva et al., 2016). Thus, MOF–metal nanocomposites present versatility, high stability, and dispersibility. As a result, MOF composite with metal particles is an effective route to design the superior electrochemical sensors (Meng et al., 2018). MOFs composited with noble metals, transition metals, and two different metal cations are regarded as effective strategies to prepare sensing materials with higher stability and catalytic efficiency (Nan et al., 2020; Yang et al., 2020). Furthermore, MOFs show low electronic conductivity, electrical reactivity, and stability in aqueous media, which limit their applications in electrochemical sensors. Assembling MOFs with conductive materials, such as graphene, carbon nanotubes, carbon blocks, and carbon nanofibers, is an effective strategy (Wang et al., 2017; Lai et al., 2019; Li L et al., 2019; Zhou Y. et al., 2020). In addition, doping graphene with heteroatom can further improve the catalytic activity of MOF-based carbon materials (Deng et al., 2020a). Similar to carbon nanomaterials, conductive polymers present excellent electrical conductivity, low cost, and ease of polymerization that are ideal materials to overcome poor electrical conductivity of MOFs (Liu et al., 2020). The introduced nonnatural polymers, such as new functional groups, can significantly improve the structure and properties of MOFs. Moreover, by integrating heme into MOFs, the dimerization and oxidative self-destruction of heme are improved, contributing to their optimum detection performance and stability (Zhang et al., 2015).
In this review, advances of MOF-based catalysts in electrochemical sensors are comprehensively summarized. Based on the structure–activity–performance relationship of MOF-based catalysts, we introduce the mechanism of MOF-based catalysts as electrochemical sensors, including metal cations, synthetic ligands, and structure. Then, the MOF-based composites are successively divided into metal-based, carbon-based, and other MOF-based composites. Furthermore, their application in environmental monitoring, food safety control, and clinical diagnosis is discussed. The perspective and challenges for advanced MOF-based composites are proposed at the end of this contribution.
Mechanism of Metal–Organic Frameworks-Based Catalysts in Electrochemical Sensor
MOFs, with high porosity, biocompatibility, and superior specific surface area, have been widely applied in catalysis, adsorption, separation, and energy storage (Jiawen et al., 2019). The mechanism of MOFs in electrochemical sensors is as follows: 1) Signal amplification: loading different functional materials and signal molecules on MOFs is beneficial to the electrochemical detection (Yi et al., 2016; Liu et al., 2020). 2) Catalysts and signal probes: MOFs present periodic porous structure by coordination of metal cations and organic ligands, which can induce rich catalytic activity and redox activity centers, attributing to superior electrochemical properties (Liu et al., 2018). 3) Size selection: the macroporous structure of MOFs is helpful to easily introduce the guest materials and perform the size selection of substance molecules (Zhu and Xu, 2014; Zhang et al., 2019). 4) MOFs can generate interaction forces with analytes (including Van der Waals force, covalent bond, and p–p interaction), resulting in the improved selectivity for electrochemical detection (Carrasco, 2018).
In this review, the mechanism of MOFs in catalysts and signal probes, including the effects of catalytic active centers and redox active centers on electrochemical sensors, is mainly discussed (Liu et al., 2018). MOF materials with metal cations and ligands can provide the desired active sites, which deserve high catalytic activity for various detection molecules. In the electrochemical sensors, metal ions can be used as charge carriers. The interaction between the sensing material and target analyte is beneficial to enhance its selectivity at room temperature (Li et al., 2021). Furthermore, the active metal ions in MOF-based nanomaterials can be used as the catalysts, which can improve the activity of the oxidation–reduction reaction, resulting in the amplified electrochemical signals and the improved sensitivity (Liu et al., 2018). Furthermore, the metal cations in MOFs also act as coordination centers to form an infinite crystal network. For example, common active metal nodes, such as Co, Cu, Zn, and Cr, and their redox activity can enhance the catalytic ability of MOFs (Liu et al., 2020). In addition, organic ligands with redox activity are also attributed to the catalytic active sites of MOFs (Yang et al., 2022). In general, the organic ligands for MOFs can be divided into chemical ligands and biological ligands (Smaldone et al., 2010). For instance, porphyrin, heme, and amino acids are common organic ligands, which present affinity to metal cations and combine well with them.
The superior structure of MOFs also plays an important effect on the boosted electrochemical activity. Their flexible and highly porous structure can be helpful to the easy diffusion of analyte molecules, facilitating the interaction between the host and analyte (Liao et al., 2018; Yang et al., 2022). Furthermore, MOFs, with a porous structure and superior specific surface area, act as a good carrier to form composite materials, resulting in the improved electrochemical activity (Kitagawa et al., 2004; Cui et al., 2013; Wang C et al., 2018; Li D et al., 2019; Wang Y et al., 2020). Therefore, regulating the structure of MOFs is an effective route to adjust its composition and structure, leading to larger surface area, higher porosity, and better electrochemical activity (He et al., 2021).
Metal–Organic Framework-Based Composites
MOFs, with the merits of diverse chemical combinations, rich metal active sites, and adjustable structure, have attracted wide attention (Li et al., 2020). However, the slow mass transfer, low conductivity, and instable structure of MOFs as catalysts limit their practical application (Ma and Zhu, 2020). Various strategies are adopted to improve the electrocatalytic behavior, mechanical properties, and stability of MOFs. Designing MOF–metal, MOF–carbon, and other MOF-based nanocomposites is an effective strategy to induce MOFs with high porosity and ordered crystal pores (Bradshaw et al., 2012; Moon et al., 2013; Qiang et al., 2013; Falcaro et al., 2014; Li et al., 2016).
Metal–Organic Framework–Metal Nanocomposites
Due to the limited pore size of MOFs, the size of synthesized particles will be confined to nanoscale. Metal nanoparticles, with the advantages of superior conductivity and high surface area, can be used to increase the electron transfer rate (Ghaffari et al., 2015; Da Silva et al., 2016). Generally, MOF–metal nanocomposites present versatility, high stability, and dispersibility. Therefore, MOF composite with metal particles is an effective route to design the superior electrochemical sensors (Meng et al., 2018).
The size and morphology of noble metal nanoparticles can reduce the overpotential of oxidation and reduction, which can effectively regulate the electrocatalytic properties (Azad and Ganesan, 2010; Gupta and Ganesan, 2015; Sonkar and Ganesan, 2015). Recently, grafting noble metal nanoparticles on MOFs is widely applied as new electrode materials for various electrochemical and biochemical sensors (Turner et al., 2008; Sabo et al., 2007; Zhu et al., 2017; Mosleh et al., 2017). For instance, a porous rhombic dodecahedron structure of Ag@zeolitic imidazolate framework-67 is synthesized, which presents a strong electrocatalytic activity and low detection limit toward H2O2 reduction (Dong et al., 2019). Interestingly, combined with photocatalytic technology and electrochemical sensing, a novel synthetic method of Ag/MIL-160 hybrid is developed to detect p-nitrophenol (Figure 1A) (Liu Q. et al., 2019). The generated charge carrier in the Ag/MIL-160 organic molecule initiates its photocatalytic functionality (Figure 1B), the high sensitivity for pollutant reduction is dictated by the photocatalytic activity of Ag nanoparticles, and selective electron migration on the electrode interface (Figure 1C). In another example, Ag/MIL-101 composite-modified GCE is reported to be useful for monitoring tryptophan (Peng et al., 2016). The existed p–p accumulation between the ligands of MOF and tryptophan increases the diffusion of analyte molecules. Furthermore, the electromagnetic field generated by the noble metal nanoparticles promotes the accumulation of tryptophan molecules on the surface of MIL-101 (Yang J et al., 2015).
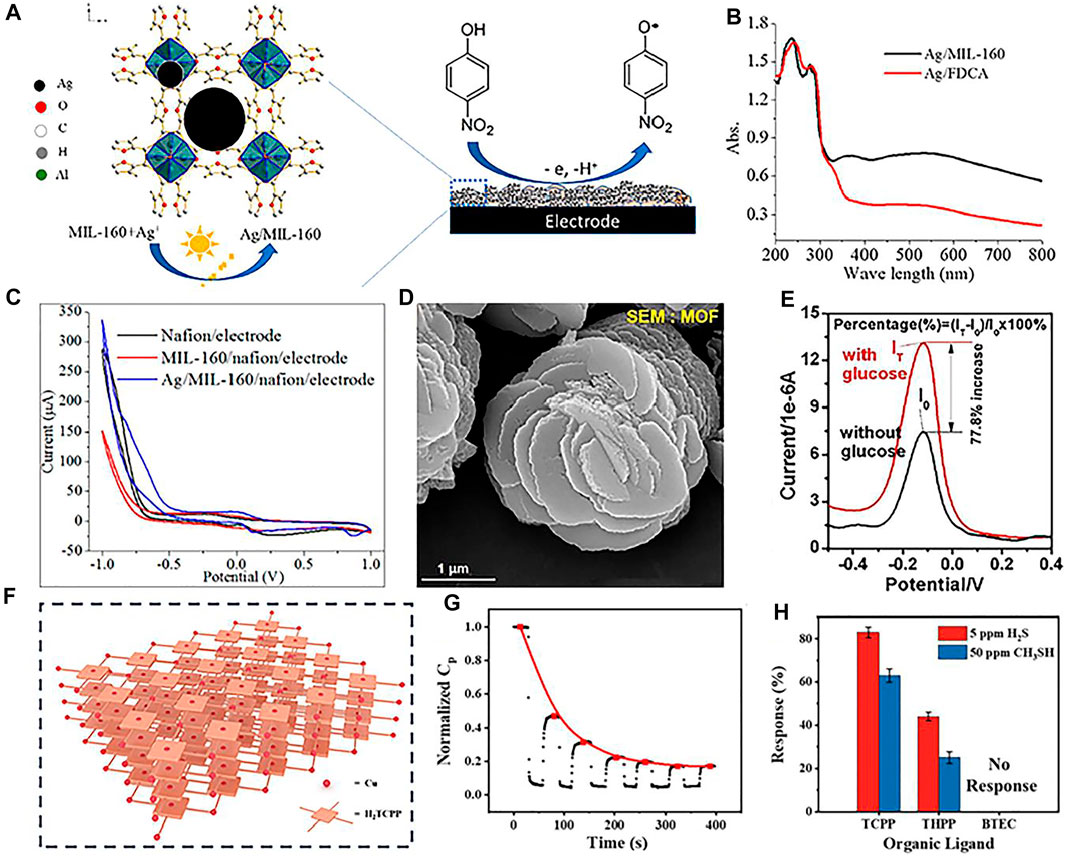
FIGURE 1. (A) Synthetic process of Ag/MIL-160; (B) UV–vis diffuse reflectance spectra of prepared composites; (C) CV curves of different electrodes at a scan rate of 50 mV s−1 in an electrolyte with p-NP. Reproduced with permission (Liu et al., 2019). Copyright 2019, ACS. Scanning electron microscope (SEM) image (D) and DPV responses without and with 4 mM glucose (E) of Cu(II)-anchored MOFs. Reproduced with permission (Zhang et al., 2020). Copyright 2020, Elsevier. Scheme (F), normalized capacitance response (G) of the Cu-TCPP IC-MOF sensor and (H) Cu-X IC-MOF sensors to 5 ppm H2S and 50 ppm CH3SH. Reproduced with permission (Li et al., 2021). Copyright 2020, Wiley.
Transition metals, such as Cu, Fe, Co, Ni, Zn, and Mg, present the merits of low price and high efficiency; they have been widely introduced to composites with MOF (Li et al., 2019; Hosseini et al., 2016). For instance, Cu(II)-anchored MOFs are constructed as signal probes (Figure 1D) (Zhang et al., 2020). This prepared composite presents an excellent glucose oxidation activity and amplified electrochemical signal, which can be ascribed to the oxidation of glucose by the generated Cu(III) from the oxidation of Cu(II) (Figure 1E). Furthermore, ionic conductive metal–organic framework sensor arrays act as charge carriers, which can directly and selectively interact with analytes (Lu et al., 2020). For example, a series of IC-MOF sensor arrays are constructed by modulating various metal nodes (Cu, Co, Ni, Zn, and Mg) and organic ligands (H2TCPP, H2THPP, and H4BTEC) (Figure 1F) (Li et al., 2021). Due to the strong interaction between H2S and Cu2+, the synthesized material can generate CuS during the detection process, resulting in an irreversible reaction, which can be adopted to detect volatile sulfide (Figures 1G,H).
The bonding between two different metal cations can increase electrical conductivity and improve the electrocatalytic efficiency, which is ascribed to different oxidation potentials and associated electron configurations (Yang et al., 2018). Therefore, a unique synergistic effect between two different metal elements is helpful in obtaining higher stability and efficiency (Wen et al., 2015; Jiang et al., 2018). Recently, bimetallic nanoparticles, including Fe, Co, Ni, or Cu, with a cooperative effect have been developed to optimize the performance of MOFs (Tang et al., 2016; Wang Z. et al., 2018). For instance, Au@Cu MOF nanomaterials with unique structures can effectively increase the number of binding sites on the polymer network, obtaining a more sensitive electrochemical sensor (Hatamluyi et al., 2020). The advanced core–shell heterostructure is introduced to fabricate composites by encapsulating metal oxides or metal nanoparticles as a core and MOFs as a shell (Paolo et al., 2016; Wengert et al., 2017; Yang Q. et al., 2017). The metal oxides or metal nanoparticle cores (e.g., magnetic, electrical, and catalytic properties, etc.) act as a catalyst, and MOFs shells (e.g., multiple coordination sites, ordered crystalline pores, structural adaptability advantages, and flexibility, etc.) act as a recognition agent for analog molecular sieves, may be combined. These advanced structures can greatly improve their anti-aggregation stability and avoid undesirable dissolution or corrosion in the photocatalytic process, resulting in boosted catalytic and adsorption properties (Liu and Tang, 2013; Kempahanumakkagari et al., 2018). For instance, Fe-MOF@Fe3O4@C core–shell nanostructured composite is composed of iron-based MOF and mesoporous Fe3O4@C (Zhang et al., 2017). Specific aptamer metal ions (e.g., Pb2+ and As3+) are attached to the constructed nanocomposites by supramolecular stacking and hydrogen bond interactions, exhibiting good anti-interference characteristics and detection of Pb2+ and As3+ ions in spiked river water.
Metal–Organic Framework–Carbon Nanomaterial Composites
The weak electronic conductivity, electrical reactivity, and low stability in aqueous media of MOFs limit their applications in electrochemical sensors. To overcome these technical shortcomings, they can be assembled with conductive materials, such as graphene, carbon nanotubes, carbon blocks, and carbon nanofibers, which are introduced to be assembled with MOFs (Wang et al., 2017; Lai et al., 2019; Li Y et al., 2019; Zhou et al., 2020). Among which, graphene oxide/reduced graphene oxides/carbon nanotubes as unique conductive additives can improve the electrical conductivity and mechanical strength of MOFs (Zhang et al., 2014; Liu et al., 2016).
The simplest way to improve the conductivity of MOF materials is to mix them with highly conductive carbon paste electrodes (Yang et al., 2014; Wang et al., 2013). The pore structure of carbon paste electrode-modified MOFs composite can allow the analyte to be pre-concentrated from the bulk solution onto the electrode surface, which helps to improve the selectivity of the analyte (Doménech et al., 2007). For instance, Co-based metal–organic coordination polymer-modified carbon paste electrodes are developed to analyze the electrocatalytic performance of redox glutathione (Figure 2A) (Yuan et al., 2014). This constructed composite exhibits excellent electrocatalytic oxidation–reduction and high selectivity of glutathione (Figures 2B,C). Furthermore, carbon paste electrodes modified with MOFs can be used for the electrocatalytic oxidation and detection of nitrite (Zhou et al., 2014). The modified material demonstrates improved sensitivity and selectivity. However, high background currents and continuous use of the electrode material will lack stability and reproducibility. Carbon nanotubes, with the advantages of the high aspect ratio, large specific surface area, and good mechanical properties and electrical properties, are widely used as electrode materials (Chen and Dai, 2013). Single-walled carbon nanotubes (SWCNTs) are covalently functionalized with benzoic acid and transition metal ions, which can form a 3D porous inorganic–organic hybrid framework, resulting in good electrochemical performance and reproducibility. Therefore, SWCNT–MOF composite is an effective electrochemical sensor material for organophosphorus pesticides.
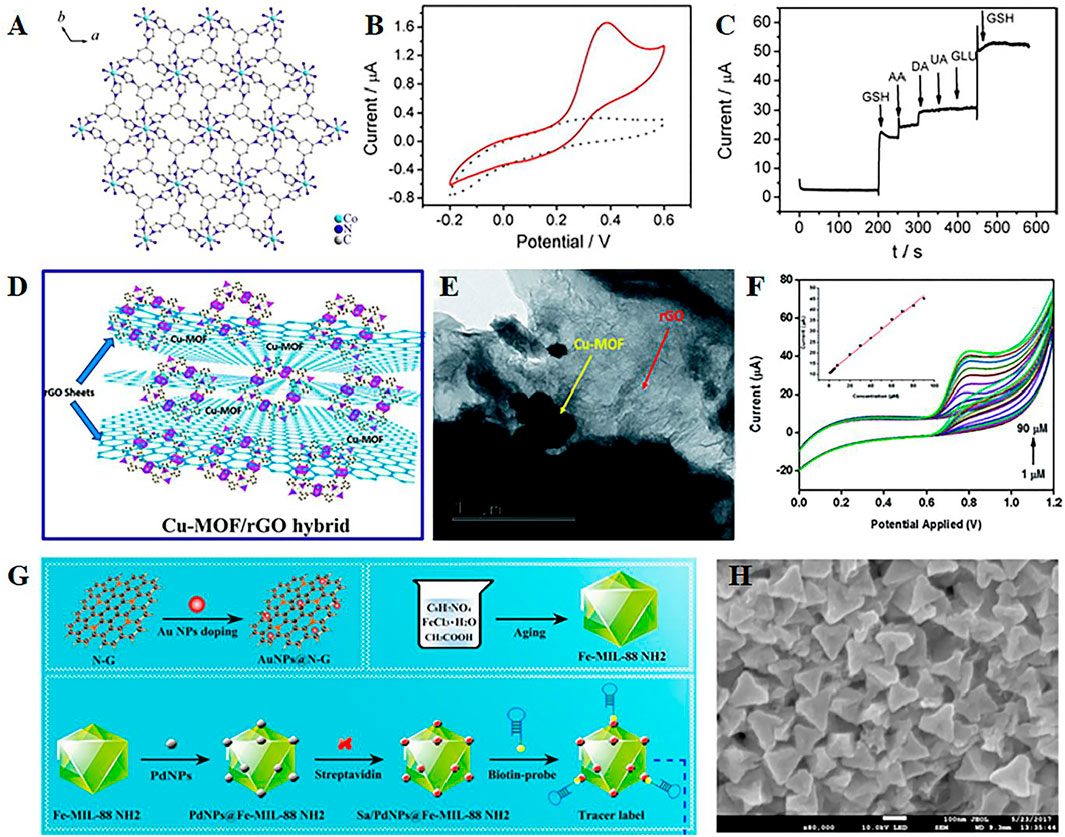
FIGURE 2. (A) Illustration of the structure of metal–organic coordination polymers with 1,3,5-tris (1-imidazolyl) benzene and transition element Co2+ (Co-MOCP), CVs (B), and interference test (C) of the Co-MOCP/carbon paste electrode in the different solution. Reproduced with permission (Yuan et al., 2014). Copyright 2014, Elsevier. Schematic structure (D), transmission electron microscope (TEM) images (E), and CV curves at different nitrite concentrations (F) of the Cu-MOF/rGO hybrid. Reproduced with permission (Saraf et al., 2016). Copyright 2016, RSC. (G) Synthetic route of different composites; (H) field emission scanning electron microscope image of PdNPs@Fe-MOFs. Reproduced with permission (Li et al., 2018). Copyright 2018, Elsevier.
Similarly, graphene is a well-known advanced two-dimensional nanomaterial with advantages of large specific surface area and ultrafast carrier mobility (Chen et al., 2013). Therefore, MOF/graphene (or graphene oxide) composites have been developed in various electrochemical sensing applications. A copper-based MOF is proposed by combining with graphene for electrochemical sensing of H2O2 and ascorbic acid (Yang T et al., 2015). Due to the hydrogen bond between Cu-MOF and graphene, p-p stacking, and Cu-O coordination, the synthesized nanocomposites exhibit high stability and good anti-interference properties detect H2O2 and ascorbic acid in various carbohydrates. Furthermore, rGO can be introduced to the composite with MOF for detection of nitrite 1 (Figure 2D) (Saraf et al., 2016). rGO can greatly improve the conductivity of MOF in the composite. The positive synergistic effects exist between Cu-MOF crystals and rGO nanosheets (Figure 2E), and these can be attributed to the improved electrocatalytic performance of the prepared electrochemical sensor electrode (Figure 2F).
In general, nitrogen-doped graphene can present more defect sites and lower aggregation of graphene sheets, which is beneficial to increase the biocompatibility of graphene sheets and functionalize easily with noble metal nanoparticles (Chen et al., 2016). A signal amplification strategy is developed to construct AuNPs-functionalized nitrogen-doped graphene as capture probes, and PdNPs@Fe-MOFs as nanocarriers (Figures 2G,H) (Li et al., 2018). This assembled structure can initiate the next reaction process, which induces numerous tracer indicators anchored onto the sensing interfaces, contributing to the superior specificity and recovery in spiked serum samples. In addition, black phosphorus can bind with antibodies and enhance electron transfer; thus, the black phosphorus electrochemical sensor based on magnetic covalent organic frameworks is developed to detect prostate-specific antigens, which can be widely applied in detecting biomarkers of cancer (Pandey et al., 2021).
Other Metal–Organic Framework-Based Composites
Similar to carbon nanomaterials, conductive polymers present excellent electrical conductivity, low cost, and ease of polymerization that are ideal materials to overcome poor electrical conductivity of MOFs (Liu et al., 2020; Deng et al., 2020b). For instance, MOF–polyaniline composite (UiO-66-NH2@PANI) was synthesized by polymerizing a conductive PANI in the presence of pre-synthesized UiO-66-NH2 (Wang et al., 2017). The composite exhibited excellent electrochemical redox performance of Cd2+ ions, which is related to the synergistic effect between UiO-66-NH2 and PANI (Figures 3A–C). Furthermore, the large surface area and the existing chelating groups in MOF increase the number of conduction paths and increase the electron transfer rate between the solution and the composite electrode surface. The introduced nonnatural polymers, such as new functional groups, can significantly improve the structure and properties of MOFs. MIL-53(Fe), a flexible material consisting of iron oxides and phthalate, is functionalized by polymethyl methacrylate and can be used to make electrochemical sensor materials for detecting melamine in milk samples (Yang Z et al., 2021). In addition, MOFs have the merits of abundant pores, large surface area, and good biocompatibility, which can effectively prevent the aggregation and leakage of enzymes and improve the biological activity and stability of enzymes (Liu et al., 2020). Therefore, MOFs are usually used to immobilize enzyme and other biomacromolecules.
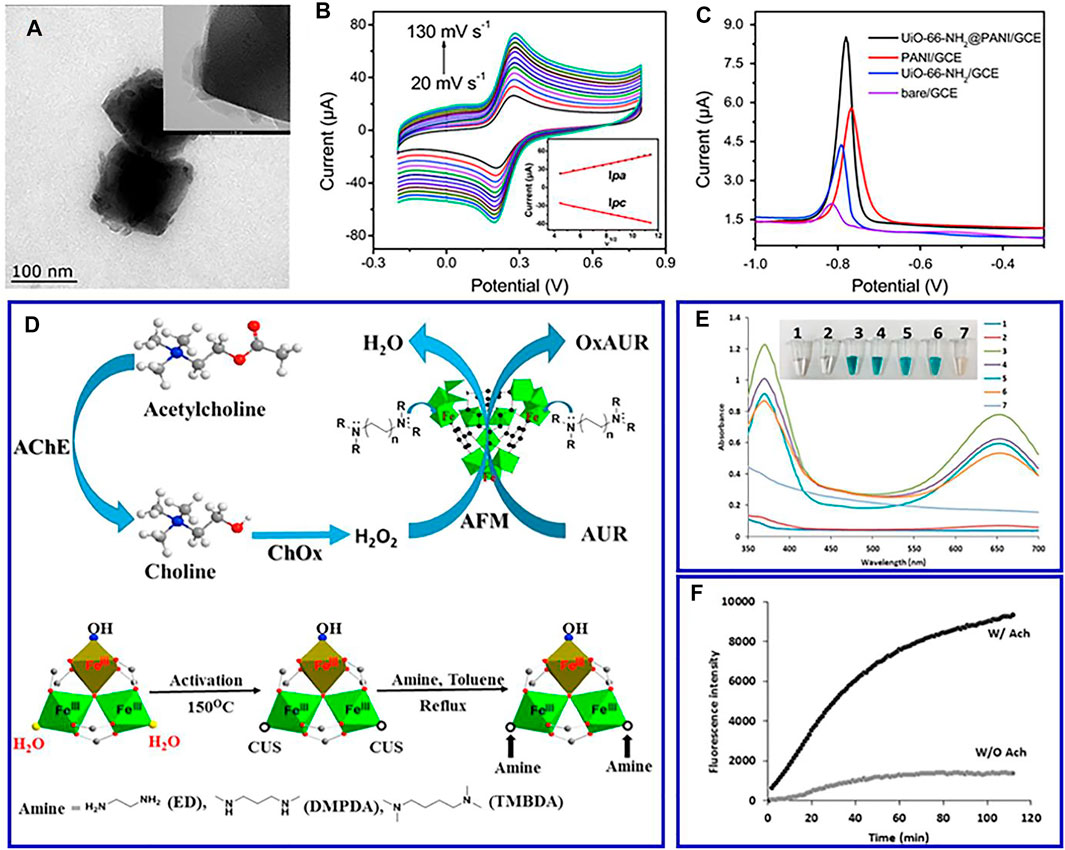
FIGURE 3. TEM images (A), CV in 0.1 mol L−1 KCl solution with 1 mmol L−1 Fe(CN)63−/4− at different scan rates (B), and differential pulse voltammograms of 100 μg L−1 Cd(ii) (C) of prepared UiO-66-NH2@PANI. Reproduced with permission (Wang et al., 2017). Copyright 2017, RSC. (D) Schematic representation of the route and coordinatively unsaturated grafted MIL-100(Fe); (E) comparison of the peroxidase-mimic activity of different samples; (F) time-dependent fluorescence intensities. Reproduced with permission (Valekar et al., 2018). Copyright 2018, Elsevier.
Heme is a famous natural metalloporphyrin, which acts as the active center of hemoglobin. Due to the reversible conversion of Fe(III)/Fe(II), heme presents significant peroxidase-like catalytic activity (Reuillard et al., 2017). However, its catalytic life is limited due to dimerization and oxidative self-destruction in the aqueous medium (Li et al., 2018). Anchoring hemin on a suitable carrier material is an effective strategy to remedy these shortcomings (Wang et al., 2016). MOFs with a regular porous structure are ideal candidates for hemin fixation (Zhang et al., 2015). By integrating heme into MOFs, the dimerization and oxidative self-destruction of heme are improved, contributing to the optimum catalytic performance and chemical stability of MOFs. For instance, a novel amine-grafted MOFs is designed as a promising alternative to peroxidase enzyme (Figure 3D) (Valekar et al., 2018). The synergetic effect of the enhanced negative potential and tuned molecular size of the grafted diamine are attributed to the improved fluorescent assay of choline and acetylcholine, which effectively detect choline and acetylcholine levels in real samples of milk and serum (Figures 3E,F).
Application of Metal–Organic Framework-Based Catalysts in Electrochemical Sensors
Environmental Monitoring
With rapid urbanization and industrialization, the ecological environment is suffering from serious damage (He et al., 2021). Developing satisfactory electrochemical sensors is an effective route to detect harmful chemicals, especially the detection of toxic gases with low concentrations and heavy metal ions in water (Dorda et al., 2003; Aslam et al., 2014; Zhang et al., 2017). MOF-based composites can be used to transform harmful chemicals in air and water into electrochemistry as fine-sensing materials. However, the formation of MOF inorganic clusters is highly dependent on the geometry, length, and connectivity of building organic linkers (Bai et al., 2016; Cho et al., 2019). Therefore, expanding the diversity and properties of MOFs is critical to their applications in environmental monitoring.
For instance, a layered porous Cu–benzene-1,3,5-tricarboxylic acid MOF is constructed for the glyphosate detection (Cao et al., 2019). The response current of the synthetic material is significantly increased, which can be ascribed to the strong affinity between chelate groups on the glyphosate with Cu2+. In another example, gold-modified MoS2/rGO and AuPd@Fe-MOFs are constructed as an electrochemical adapter sensor for detecting Pb2+ (Wang Z et al., 2020). The combination of catalytic chain and base complementary is contributed to the improved detection performance. Interestingly, the hinge-like organic ligand is obtained by the desymmetrization strategy (Feng et al., 2019). MOF materials are modified at the molecular level, which can realize the coexistence of acidic sites and alkaline sites in the material. This synthetic mesoporous composite with a functional structure is beneficial to the transformation of cascade catalytic, achieving high activity of a two-step efficient series catalytic reaction (Figure 4A).
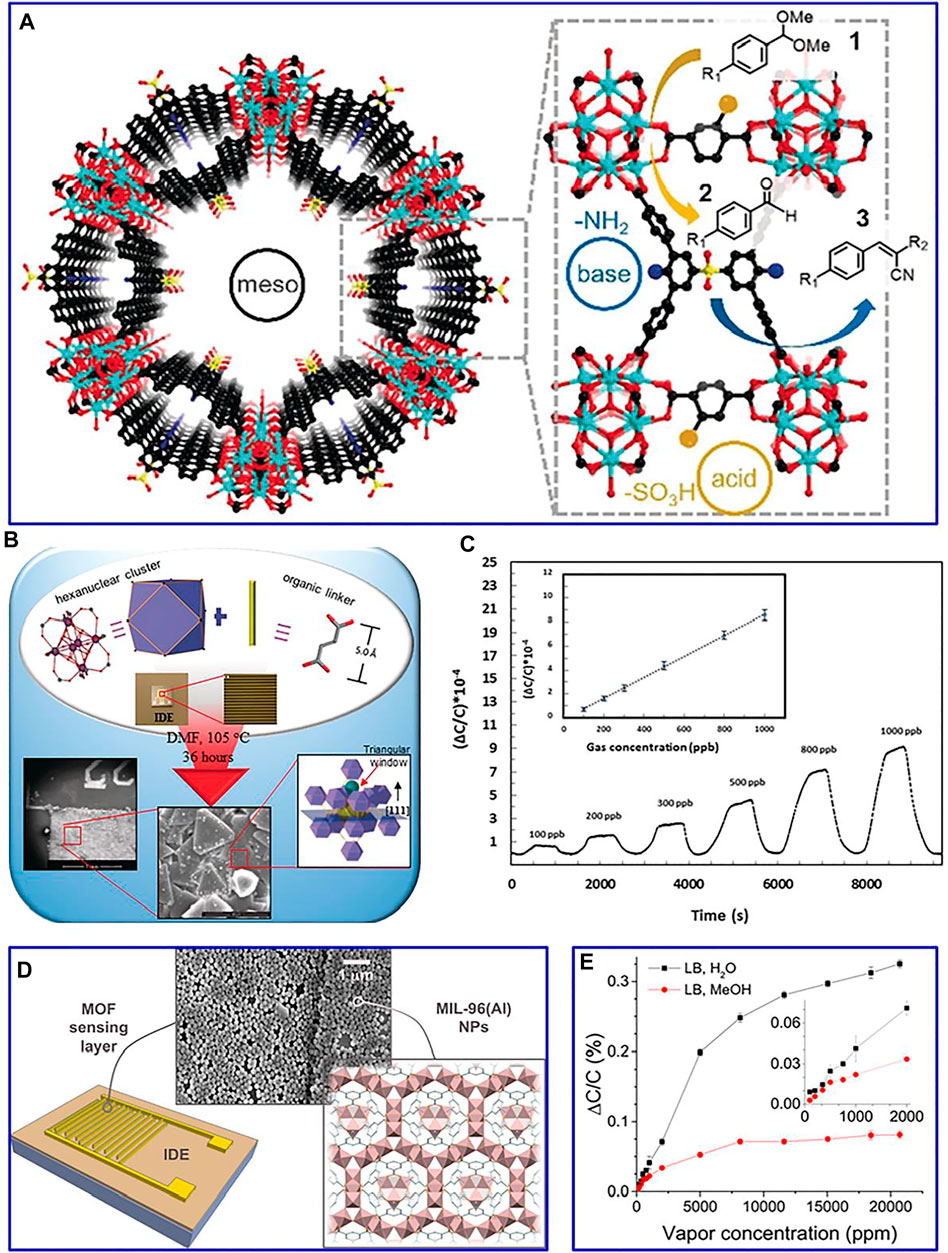
FIGURE 4. (A) One-pot tandem reaction of benzaldehyde dimethylacetal and malononitrile by bifunctional mesoporous catalyst. Reproduced with permission (Feng et al., 2019). Copyright 2019, Wiley. (B) Schematic of the prepared approach of the fumarate-based fcu-MOF; (C) detection of H2S with concentrations of 1–100 ppm. Reproduced with permission (Yassine et al., 2016). Copyright 2016, Wiley. (D) Illustration of the structure of the used IDEs showing the MOF LB film characterization by SEM; (E) normalized capacitive response of IDEs to water and methanol. Reproduced with permission (Andrés et al., 2020). Copyright 2020, ACS.
MOF-based composites with the merits of excellent gas adsorption/separation capability can be adopted as electrically transduced gas sensors (Yao et al., 2021). Integrating MOFs onto capacitive sensors based on interdigitated electrode chips is an effective route to improve their detection performance. For example, an in situ growth strategy is adopted to synthesize fumarate-based fcu-MOF thin film on an interdigitated electrode (Figure 4B). This constructed sensor presents a remarkable detection sensitivity (down to 100 ppb) and lower detection limit (around 5 ppb) for H2S (Figure 4C). Furthermore, the Langmuir–Blodgett method is used to deposit MIL-96(Al) MOF thin films on the interdigitated electrode chips (Figure 4D) (Andrés et al., 2020). These prepared films achieve superior selective and short response/recovery for water and methanol (Figure 4E), which can be also extended to the detection of methanol, toluene, and chloroform, etc.
Food Safety Control
Electrochemical (biological) sensors are one of the most sensitive, simple, and selective chemical sensors, which have been widely used in rapid and reliable food safety control (Otles and Yalcin, 2012). MOFs, with the merits of uniform structures, ultrahigh porosity, and tunable composition, act as the promising sensor for food safety control. Nevertheless, the reuse and long-term storage of electrochemical sensor materials fabricated from MOFs in complex sample matrices remain a challenge. Inexpensive microbial sensors are designed for single use to avoid degradation of biosensor elements in complex matrices (Semih Otles, 2012).
In a recent study, Pt nanoparticles were decorated on a glassy carbon electrodes-modified Fe-based MOFs, and this designed MOF-based composites acted as a sensitive label-free electrochemical aptasensor to detect aflatoxin M1 (Figure 5A) (Jahangiri-Dehaghani et al., 2020). The fabricated aptasensor was successfully applied to measure AFM1 concentration in powder and pasteurized milk samples. Furthermore, molecularly imprinted mMOFs was synthesized by layer–layer modification to detect turtotomycin (Li et al., 2021). The magnetic pole in molecularly imprinted mMOFs is beneficial to form an electrochemical sensing interface, and its imprinted cavity can serve as electronic channels for probes for label-free detection of over-the-counter drugs.
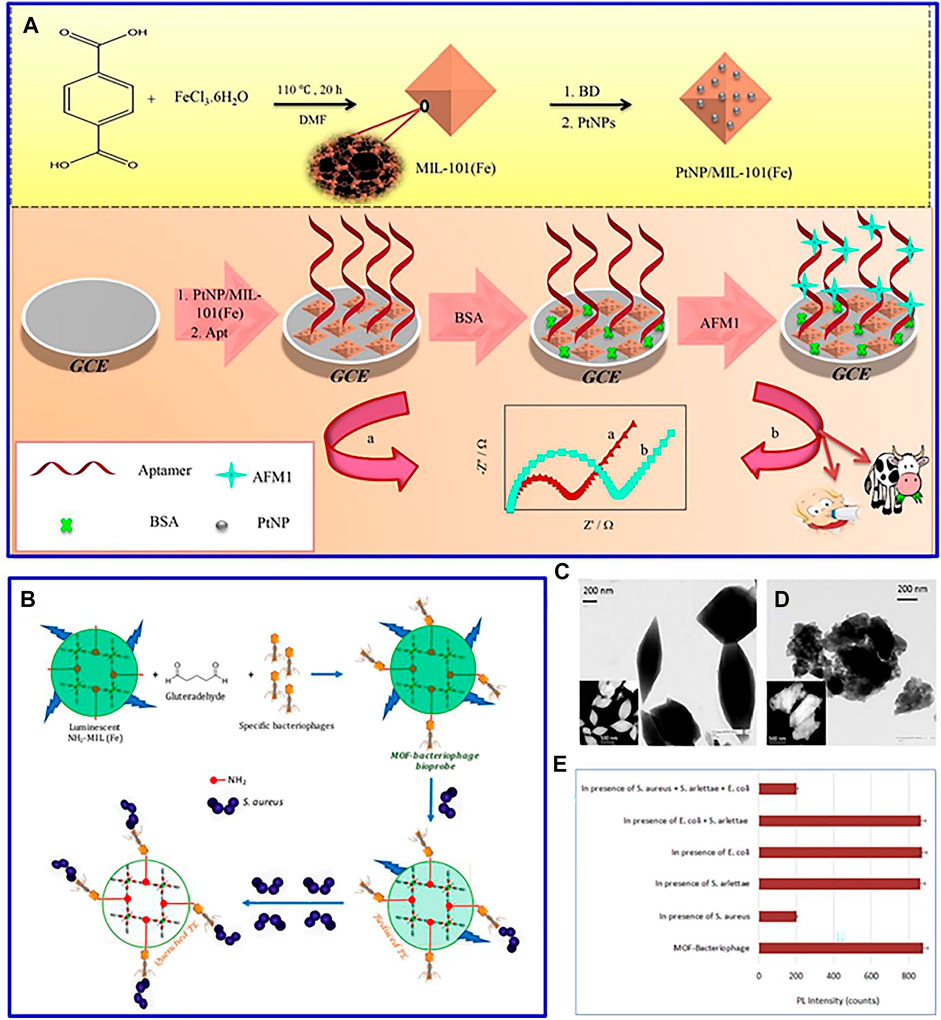
FIGURE 5. (A) Schematic diagram for the preparation schematic diagram and electrochemical test of PtNP/MIL-101(Fe). Reproduced with permission (Jahangiri-Dehaghani et al., 2020). Copyright 2020, Elsevier. (B) Schematic of NH2-MIL53(Fe)-bacteriophage biosensor; TEM image of (C) NH2-MIL-53 MOF and (D) bacteriophage-loaded NH2-MIL-53 MOF; (E) specificity of the proposed biosensor. Reproduced with permission (Bhardwaj et al., 2017). Copyright 2017, ACS.
In general, when food with packaging is stored for a long time, foodborne pathogen infection will occur, resulting in many health-related problems (Jahangiri-Dehaghani et al., 2020). This MOF-based luminescent sensor can be adopted to detect these foodborne pathogens. For instance, a fluorescent MOF [NH2-MIL-53(Fe)] with a target-specific bacteriophage was synthesized for the detection of Staphylococcus aureus (Figure 5B) (Bhardwaj et al., 2017). The advanced structure of MOF can offer a precise control on particle size distribution (Figures 5C,D), which is helpful to construct a better structural compatibility with bacteriophages, contributing to the high stability, specificity, reusability, and wider linear range of the bacteriophage sensor (Figure 5E). In addition, CeO2/CuOx@MC nanocomposite is introduced as a carrier to detect the microtobramycin in milk (Cheng et al., 2021). The combination of different materials presents a strong biological affinity for the adaptor chain, contributing to a wide linear range and low detection limit of tobramycin.
Clinical Diagnosis
MOF materials, with the advantages of selective composition, adjustable pore size, and large surface area are widely used as electrochemical sensor materials in biomedical fields, including cancer diagnosis (e.g., cancer markers, microRNA, and live cancer cells) and glucose detection (Chen et al., 2013; Qin et al., 2016; Xie et al., 2018; Petrosillo et al., 2020). Nevertheless, it is difficult to construct the nanoparticles and active biomolecules in the same MOF-based structure. Recently, many strategies, such as functional MOFs and combination with bionic enzyme, as well as utilization of biosensing and molecular recognition technology, have been developed to improve the photoelectric and catalytic properties of MOF materials (Liao et al., 2017; Shen et al., 2018).
For instance, a multifunctional homologous MOF hybrid material is designed with enhanced therapeutic effect on hypoxic tumor cells by the in situ growth method (Liu et al., 2019). Black phosphorus quantum dots and catalase were precisely assembled into the inner and outer layers of a layered MOF to form a multifunctional MOF heterostructure (Figure 6A). This advanced heterostructure converts excess H2O2 into O2 by catalase wrapped in its outer shell, improving the hypoxic microenvironment of tumor cells (Figure 6B). An innovative MOF-on-MOF method is adopted to construct Zn-MOF-on-Zr-MOF composite for detecting protein tyrosine kinase-7 (Nan et al., 2019). The synthetic Zn-MOF-on-Zr-MOF composite presents hierarchical cross leaves and multilayer nanosheet structures, which demonstrate excellent sensing capabilities for the detection of protein tyrosine kinase-7. This improved performance is mainly ascribed to the presence of Zr-MOF, which significantly facilitates aptamer fixation and stabilizes the formed G-tetrexes, providing a new avenue for the application of bimetallic MOFs in the early cancer diagnosis. Interestingly, multifunctional iron-based MOFs, with the advantages of superior peroxidase-like activity, are developed as a sandwich-type biosensor (Figure 6C) (Li et al., 2018). The biosensor demonstrates a low detection limit (0.003 fM) and wide detection range (0.01 fM to 10 p.m.) for detecting miR-122 in human serum (Figure 6D). This strategy can be adopted to detect drug-induced liver injury at an early stage.
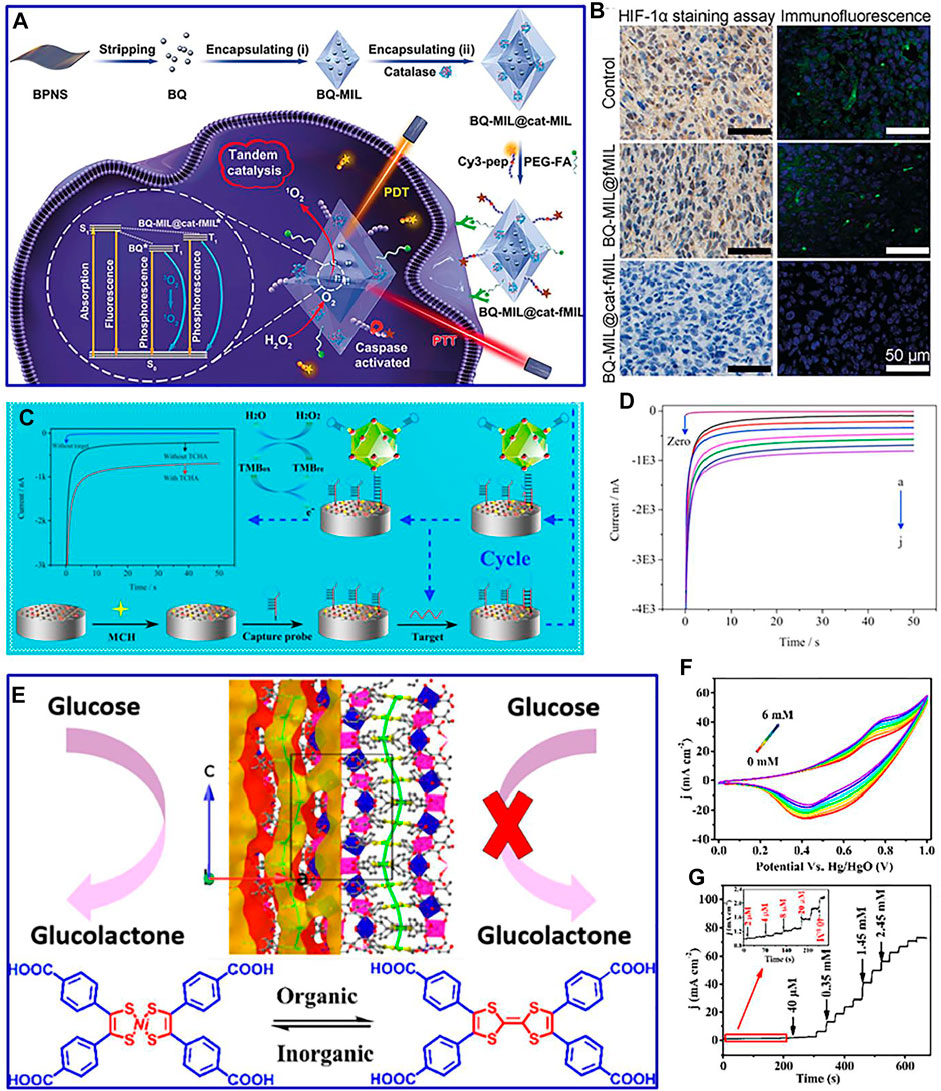
FIGURE 6. (A) Assemble process of synthetic material and its mechanism against hypoxic tumor cells; (B) immunohistochemistry and immunofluorescence staining of HIF-1α in tumor slices of different composites. Reproduced with permission (Liu et al., 2019). Copyright 2019, Wiley. (C) Fabrication process of the biosensor and the target-catalyzed hairpin assembly for target recycle; amperometric curves (D) of the proposed biosensor incubated with different concentrations of miR-122 containing 1 mM 3,3′,5,5′-tetramethylbenzidine and 20 μM H2O2. Reproduced with permission (Li et al., 2018). Copyright 2018, Elsevier. (E) Structures of the constructed material; CV curves (F) and amperometric response (G) of the constructed material with varied glucose concentrations. Reproduced with permission (Zhou et al., 2020). Copyright 2020, ACS.
Recently, commercial glucose sensors are mainly based on glucose oxidase-assisted electrooxidation. In order to avoid low reproducibility, complex immobilized enzyme process, and decreased enzyme activity of commercial glucose sensors, based on the direct electrocatalysis of electrode materials, the application of MOFs in nonenzymatic sensors has been designed and developed (Burke and Gorodetsky, 2012). Nevertheless, the existing small detection range, low sensitivity, and poor stability of MOFs in nonenzymatic glucose electrochemical sensors limit their commercial application (Al-Zoubi et al., 2020). Therefore, it is vital to develop MOF-based composites with good stability and high activity for glucose detection. For example, the Au/Cu MOFs coupled with a capture probe and a convertase form a bioconjugate (Liu et al., 2020). This modified electrode is incubated in sucrose solution, which can effectively detect glucose. Furthermore, coordinating metals with the functional tetrathiafulvalene core is an insightful route to modulate the catalytic performance of MOF-based composites. For instance, nickel bis(dithiolene-dibenzoic acid) as a redox-active linker is constructed for functional MOFs (Figure 6E) (Zhou et al., 2020). This synthetic composite presents high sensitivity, wide detection range, and low detection limit for the detection of glucose (Figures 6F,G), which is ascribed to the oxidation of glucolactone by several reversible and stable oxidation states of nickel bis(dithiolene) compounds.
Perspective and Prospect
Developing efficient catalysts is vital for the application of electrochemical sensors. MOFs, with high porosity, large specific surface area, good conductivity, and biocompatibility, have been widely used in catalysis, adsorption, separation, and energy storage applications. In this review, based on the structure–activity–performance relationship of MOF-based catalysts, the mechanism of MOF-based catalysts as electrochemical sensors, including metal cations, synthetic ligands, and structure, is introduced. Then, the MOF-based composites are successively divided into metal-based, carbon-based, and other MOF-based composites. Furthermore, their application in environmental monitoring, food safety control, and clinical diagnosis is discussed (Figure 7). Recently, many efforts have been devoted to constructing high efficiency MOF-based catalysts. However, there still exist some challenges to achieve superior MOF-based catalysts for electrochemical sensors.
First, MOF materials with an excellent redox and catalytic activity in electrochemical sensors are still an urgent need. Due to the large surface area of MOFs, the nonspecific adsorption of coexisting substances will occur in the complex matrix, which will negatively affect the sensing performance. The fine regulation of the pore structure of MOFs can provide precision for the selective adsorption of the target. Furthermore, most MOFs with weak mechanical properties are unstable in water. Surface modification can be adopted to hydrophobic materials or introduce surface functional groups, which is a good method to improve the stability of MOFs in water.
Second, MOF-based catalysts for peroxidase-like enzymes have much lower catalytic activity than natural enzymes. Construction of MOF-based catalysts with higher surface area and richer active sites can alleviate this problem. Furthermore, the catalytic activity of MOFs can also be improved by reasonable selection of multivalent ligands and metal nodes. The weak binding force between the target analyte and MOFs affects the detection sensitivity. Surface functional group modification is an effective strategy to provide stronger binding sites for the adsorption of the target analyte. In addition to carbonizing MOFs and introducing highly conductive species to the host MOFs, in situ or posttreatment of doped conductive impurities to MOFs is an alternative to improve their conductivity, resulting in the improved performance.
In addition, during the synthetic and detection process, developing advanced observation techniques will be helpful to understand the structure–activity–performance relationship of MOF-based catalysts for electrochemical sensors. Moreover, the electrochemical sensors based on MOF catalysts still have limitations under laboratory conditions. Further research on MOF-based catalysts is needed to improve their electrochemical properties and pave the way for further application. With the development of nanoscience and biotechnology, it is believed that MOF-based electrochemical sensors will bring a broader development prospect in environmental, food safety, and clinical aspects.
Author Contributions
HY conceived and guided the project. YC, ZY, HH, and XZ performed the manuscript parts. FY, CY, FL, PY, DW, and JY discussed the work and revised the manuscript. RH, XJ, and HY discussed, wrote, and revised the manuscript. All of the authors have commented on the manuscript.
Conflict of Interest
The authors declare that the research was conducted in the absence of any commercial or financial relationships that could be construed as a potential conflict of interest.
Publisher’s Note
All claims expressed in this article are solely those of the authors and do not necessarily represent those of their affiliated organizations, or those of the publisher, the editors, and the reviewers. Any product that may be evaluated in this article, or claim that may be made by its manufacturer, is not guaranteed or endorsed by the publisher.
Acknowledgments
We acknowledge the support of the National Natural Science Foundation of China (22102125), the Scientific Research Foundation of Wuhan Institute of Technology (K2021040), the Graduate Education Innovation Fund of Wuhan Institute of Technology (CX2021168), and the Innovation Foundation of Key Laboratory of Green Chemical Engineering Process of Ministry of Education (GCX202108).
References
Al-Zoubi, T., Zhou, Y., Yin, X., Janicek, B., Sun, C., Schulz, C. E., et al. (2020). Preparation of Nonprecious Metal Electrocatalysts for the Reduction of Oxygen Using a Low-Temperature Sacrificial Metal. J. Am. Chem. Soc. 142, 5477–5481. doi:10.1021/jacs.9b11061
Andrés, M. A., Vijjapu, M. T., Surya, S. G., Shekhah, O., Salama, K. N., Serre, C., et al. (2020). Methanol and Humidity Capacitive Sensors Based on Thin Films of MOF Nanoparticles. ACS Appl. Mater. Inter. 12, 4155–4162. doi:10.1021/acsami.9b20763
Aslam, M., Ismail, I. M. I., Almeelbi, T., Salah, N., Chandrasekaran, S., and Hameed, A. (2014). Enhanced Photocatalytic Activity of V2O5 -ZnO Composites for the Mineralization of Nitrophenols. Chemosphere 117, 115–123. doi:10.1016/j.chemosphere.2014.05.076
Azad, U. P., and Ganesan, V. (2010). Influence of Metal Nanoparticles on the Electrocatalytic Oxidation of Glucose by poly(NiIIteta) Modified Electrodes. Electroanalysis 22, 575–583. doi:10.1002/elan.200900435
Bai, Y., Dou, Y., Xie, L.-H., Rutledge, W., Li, J.-R., and Zhou, H.-C. (2016). Zr-based Metal-Organic Frameworks: Design, Synthesis, Structure, and Applications. Chem. Soc. Rev. 45, 2327–2367. doi:10.1039/C5CS00837A
Bhardwaj, N., Bhardwaj, S. K., Mehta, J., Kim, K.-H., and Deep, A. (2017). MOF-bacteriophage Biosensor for Highly Sensitive and Specific Detection of staphylococcus Aureus. ACS Appl. Mater. Inter. 9, 33589–33598. doi:10.1021/acsami.7b07818
Blöcher, C., Dorda, J., Mavrov, V., Chmiel, H., Lazaridis, N. K., and Matis, K. A. (2003). Hybrid Flotation-Mmembrane Filtration Process for the Removal of Heavy Metal Ions from Wastewater. Water Res. 37, 4018–4026. doi:10.1016/S0043-1354(03)00314-2
Bradshaw, D., Garai, A., and Huo, J. (2012). Metal-organic Framework Growth at Functional Interfaces: Thin Films and Composites for Diverse Applications. Chem. Soc. Rev. 41, 2344–2381. doi:10.1039/c1cs15276a
Burke, A. M., and Gorodetsky, A. A. (2012). Taking Charge of Detection. Nat. Chem 4, 595–597. doi:10.1038/nchem.1418
Cao, Y., Wang, L., Shen, C., Wang, C., Hu, X., and Wang, G. (2019). An Electrochemical Sensor on the Hierarchically Porous Cu-BTC MOF Platform for Glyphosate Determination. Sensors Actuators B: Chem. 283, 487–494. doi:10.1016/j.snb.2018.12.064
Carrasco, S. (2018). Metal-organic Frameworks for the Development of Biosensors: a Current Overview. Biosensors 8, 92. doi:10.3390/bios8040092
Chen, L., Zheng, H., Zhu, X., Lin, Z., Guo, L., Qiu, B., et al. (2013). Metal-organic Frameworks-Based Biosensor for Sequence-specific Recognition of Double-Stranded DNA. Analyst 138, 3490–3493. doi:10.1039/c3an00426k
Chen, M., Hou, C., Huo, D., Bao, J., Fa, H., and Shen, C. (2016). An Electrochemical DNA Biosensor Based on Nitrogen-Doped graphene/Au Nanoparticles for Human Multidrug Resistance Gene Detection. Biosens. Bioelectron. 85, 684–691. doi:10.1016/j.bios.2016.05.051
Chen, Q., SongChang, W.-C., Song, H., Song, H.-B., Hu, T.-L., and Bu, X.-H. (2013). A Controllable Gate Effect in Cobalt(II) Organic Frameworks by Reversible Structure Transformations. Angew. Chem. Int. Ed. 52, 11550–11553. doi:10.1002/anie.201306304
Chen, T., and Dai, L. (2013). Carbon Nanomaterials for High-Performance Supercapacitors. Mater. Today 16, 272–280. doi:10.1016/j.mattod.2013.07.002
Cheng, W., Tang, X., Zhang, Y., Wu, D., and Yang, W. (2021). Applications of Metal-Organic Framework (MOF)-based Sensors for Food Safety: Enhancing Mechanisms and Recent Advances. Trends Food Sci. Technol. 112, 268–282. doi:10.1016/j.tifs.2021.04.004
Chiu, N.-F., Fan, S.-Y., Yang, C.-D., and Huang, T.-Y. (2017). Carboxyl-functionalized Graphene Oxide Composites as SPR Biosensors with Enhanced Sensitivity for Immunoaffinity Detection. Biosens. Bioelectron. 89, 370–376. doi:10.1016/j.bios.2016.06.073
Cho, H. S., Yang, J., Gong, X., Zhang, Y.-B., Momma, K., Weckhuysen, B. M., et al. (2019). Isotherms of Individual Pores by Gas Adsorption Crystallography. Nat. Chem. 11, 562–570. doi:10.1038/s41557-019-0257-2
Cui, J., Li, Y., Guo, Z., and Zheng, H. (2013). A Porous Metal-Organic Framework Based on Zn6O2 clusters: Chemical Stability, Gas Adsorption Properties and Solvatochromic Behavior. Chem. Commun. 49, 555–557. doi:10.1039/c2cc36713k
Da Silva, C. T. P., Veregue, F. R., Aguiar, L. W., Meneguin, J. G., Moisés, M. P., Fávaro, S. L., et al. (2016). AuNp@MOF Composite as Electrochemical Material for Determination of Bisphenol A and its Oxidation Behavior Study. New J. Chem. 40, 8872–8877. doi:10.1039/C6NJ00936K
Deng, Y., Tian, X., Chi, B., Wang, Q., Ni, W., Gao, Y., et al. (2020a). Hierarchically Open-Porous Carbon Networks Enriched with Exclusive Fe-Nx Active Sites as Efficient Oxygen Reduction Catalysts towards Acidic H2-O2 PEM Fuel Cell and Alkaline Zn-Air Battery. Chem. Eng. J. 390, 124479. doi:10.1016/j.cej.2020.124479
Deng, Y., Tian, X., Shen, G., Gao, Y., Lin, C., Ling, L., et al. (2020b). Coupling Hollow Fe3O4 Nanoparticles with Oxygen Vacancy on Mesoporous Carbon as a High-Efficiency ORR Electrocatalyst for Zn-Air Battery. J. Colloid Interf. Sci. 567, 410–418. doi:10.1016/j.jcis.2020.02.013
Doménech, A., García, H., Doménech-Carbó, M. T., and Llabrés-i-Xamena, F. (2007). Electrochemistry of Metal-Organic Frameworks: A Description from the Voltammetry of Microparticles Approach. J. Phys. Chem. C 111, 13701–13711. doi:10.1021/jp073458x
Dong, Y., Duan, C., Sheng, Q., and Zheng, J. (2019). Preparation of Ag@zeolitic Imidazolate Framework-67 at Room Temperature for Electrochemical Sensing of Hydrogen Peroxide. Analyst 144, 521–529. doi:10.1039/C8AN01641K
Falcaro, P., Ricco, R., Doherty, C. M., Liang, K., Hill, A. J., and Styles, M. J. (2014). MOF Positioning Technology and Device Fabrication. Chem. Soc. Rev. 43, 5513–5560. doi:10.1039/C4CS00089G
Falcaro, P., Ricco, R., Yazdi, A., Imaz, I., Furukawa, S., Maspoch, D., et al. (2016). Application of Metal and Metal Oxide nanoparticles@MOFs. Coord. Chem. Rev. 307, 237–254. doi:10.1016/j.ccr.2015.08.002
Feng, L., Wang, Y., Zhang, K., Wang, K. Y., Fan, W., Wang, X., et al. (2019). Molecular Pivot‐Hinge Installation to Evolve Topology in Rare‐Earth Metal-Organic Frameworks. Angew. Chem. Int. Ed. 58, 16682–16690. doi:10.1002/anie.201910717
Gupta, R., and Ganesan, V. (2015). Gold Nanoparticles Impregnated Mesoporous Silica Spheres for Simultaneous and Selective Determination of Uric Acid and Ascorbic Acid. Sensors Actuators B: Chem. 219, 139–145. doi:10.1016/j.snb.2015.05.018
Hatamluyi, B., Rezayi, M., Beheshti, H. R., and Boroushaki, M. T. (2020). Ultra-sensitive Molecularly Imprinted Electrochemical Sensor for Patulin Detection Based on a Novel Assembling Strategy Using Au@Cu-Mof/n-GQDs. Sensors Actuators B: Chem. 318, 128219. doi:10.1016/j.snb.2020.128219
He, Y., Wang, Z., Wang, H., Wang, Z., Zeng, G., Xu, P., et al. (2021). Metal-organic Framework-Derived Nanomaterials in Environment Related fields: Fundamentals, Properties and Applications. Coord. Chem. Rev. 429, 213618. doi:10.1016/j.ccr.2020.213618
Hosseini, M. S., Zeinali, S., and Sheikhi, M. H. (2016). Fabrication of Capacitive Sensor Based on Cu-BTC (MOF-199) Nanoporous Film for Detection of Ethanol and Methanol Vapors. Sensors Actuators B: Chem. 230, 9–16. doi:10.1016/j.snb.2016.02.008
Jahangiri–Dehaghani, F., Zare, H. R., and Shekari, Z. (2020). Measurement of Aflatoxin M1 in Powder and Pasteurized Milk Samples by Using a Label-free Electrochemical Aptasensor Based on Platinum Nanoparticles Loaded on Fe-Based Metal-Organic Frameworks. Food Chem. 310, 125820. doi:10.1016/j.foodchem.2019.125820
Jiang, D., Xu, P., Wang, H., Zeng, G., Huang, D., Chen, M., et al. (2018). Strategies to Improve Metal Organic Frameworks Photocatalyst's Performance for Degradation of Organic Pollutants. Coord. Chem. Rev. 376, 449–466. doi:10.1016/j.ccr.2018.08.005
Justino, C. I. L., Freitas, A. C., Pereira, R., Duarte, A. C., and Rocha Santos, T. A. P. (2015). Recent Developments in Recognition Elements for Chemical Sensors and Biosensors. Trac Trends Anal. Chem. 68, 2–17. doi:10.1016/j.trac.2015.03.006
Kempahanumakkagari, S., Vellingiri, K., Deep, A., Kwon, E. E., Bolan, N., and Kim, K.-H. (2018). Metal-Organic Framework Composites as Electrocatalysts for Electrochemical Sensing Applications. Coord. Chem. Rev. 357, 105–129. doi:10.1016/j.ccr.2017.11.028
Kitagawa, S., Kitaura, R., and Noro, S.-i. (2004). Functional Porous Coordination Polymers. Angew. Chem. Int. Ed. 43, 2334–2375. doi:10.1002/anie.200300610
Lai, C., Huang, F., Zeng, G., Huang, D., Qin, L., Cheng, M., et al. (2019). Fabrication of Novel Magnetic MnFe2O4/bio-Char Composite and Heterogeneous Photo-Fenton Degradation of Tetracycline in Near Neutral pH. Chemosphere 224, 910–921. doi:10.1016/j.chemosphere.2019.02.193
Li, B., Wen, H.-M., Cui, Y., Zhou, W., Qian, G., and Chen, B. (2016). Emerging Multifunctional Metal-Organic Framework Materials. Adv. Mater. 28, 8819–8860. doi:10.1002/adma.201601133
Li, D., Xu, H.-Q., Jiao, L., and Jiang, H.-L. (2019). Metal-organic Frameworks for Catalysis: State of the Art, Challenges, and Opportunities. EnergyChem 1, 100005. doi:10.1016/j.enchem.2019.100005
Li, H.-Y., Zhao, S.-N., Zang, S.-Q., and Li, J. (2020). Functional Metal-Organic Frameworks as Effective Sensors of Gases and Volatile Compounds. Chem. Soc. Rev. 49, 6364–6401. doi:10.1039/C9CS00778D
Li, L., Lai, C., Huang, F., Cheng, M., Zeng, G., Huang, D., et al. (2019). Degradation of Naphthalene with Magnetic Bio-Char Activate Hydrogen Peroxide: Synergism of Bio-Char and Fe-Mn Binary Oxides. Water Res. 160, 238–248. doi:10.1016/j.watres.2019.05.081
Li, L., Zhang, S., Lu, Y., Zhang, J., Zhang, X., Wang, R., et al. (2021). Highly Selective and Sensitive Detection of Volatile Sulfur Compounds by Ionically Conductive Metal‐Organic Frameworks. Adv. Mater. 33, 2104120. doi:10.1002/adma.202104120
Li, P.-P., Liu, X.-P., Mao, C.-J., Jin, B.-K., and Zhu, J.-J. (2019). Photoelectrochemical DNA Biosensor Based on G-C3N4/MoS2 2D/2D Heterojunction Electrode Matrix and Co-sensitization Amplification with CdSe QDs for the Sensitive Detection of ssDNA. Analytica Chim. Acta 1048, 42–49. doi:10.1016/j.aca.2018.09.063
Li, S., Liu, X., Chai, H., and Huang, Y. (2018). Recent Advances in the Construction and Analytical Applications of Metal-Organic Frameworks-Based Nanozymes. Trac Trends Anal. Chem. 105, 391–403. doi:10.1016/j.trac.2018.06.001
Li, Y., Xie, M., Zhang, X., Liu, Q., Lin, D., Xu, C., et al. (2019). Co-MOF Nanosheet Array: A High-Performance Electrochemical Sensor for Non-enzymatic Glucose Detection. Sensors Actuators B: Chem. 278, 126–132. doi:10.1016/j.snb.2018.09.076
Li, Y., Yu, C., Yang, B., Liu, Z., Xia, P., and Wang, Q. (2018). Target-catalyzed Hairpin Assembly and Metal-Organic Frameworks Mediated Nonenzymatic Co-reaction for Multiple Signal Amplification Detection of miR-122 in Human Serum. Biosens. Bioelectron. 102, 307–315. doi:10.1016/j.bios.2017.11.047
Liao, F.-S., Lo, W.-S., Hsu, Y.-S., Wu, C.-C., Wang, S.-C., Shieh, F.-K., et al. (2017). Shielding against Unfolding by Embedding Enzymes in Metal-Organic Frameworks via a De Novo Approach. J. Am. Chem. Soc. 139, 6530–6533. doi:10.1021/jacs.7b01794
Liao, J., Jin, B., Zhao, Y., and Liang, Z. (2019). Highly Efficient and Durable Metal-Organic Framework Material Derived Ca-Based Solid Sorbents for CO2 Capture. Chem. Eng. J. 372, 1028–1037. doi:10.1016/j.cej.2019.04.212
Liao, P.-Q., Shen, J.-Q., and Zhang, J.-P. (2018). Metal-organic Frameworks for Electrocatalysis. Coord. Chem. Rev. 373, 22–48. doi:10.1016/j.ccr.2017.09.001
Lin, H., Wang, F., Duan, Y., Kang, W., Chen, Q., and Xue, Z. (2022). Early Detection of Wheat Aspergillus Infection Based on Nanocomposite Colorimetric Sensor and Multivariable Models. Sensors Actuators B: Chem. 351, 130910. doi:10.1016/j.snb.2021.130910
Liu, J., Liu, T., Du, P., Zhang, L., and Lei, J. (2019). Metal-organic Framework (MOF) Hybrid as a Tandem Catalyst for Enhanced Therapy against Hypoxic Tumor Cells. Angew. Chem. Int. Ed. 58, 7808–7812. doi:10.1002/anie.201903475
Liu, L., Zhou, Y., Liu, S., and Xu, M. (2018). The Applications of Metal-Organic Frameworks in Electrochemical Sensors. ChemElectroChem 5, 6–19. doi:10.1002/celc.201700931
Liu, Q., Dordick, J. S., and Dinu, C. Z. (2019). Metal-organic Framework-Based Composite for Photocatalytic Detection of Prevalent Pollutant. ACS Appl. Mater. Inter. 11, 31049–31059. doi:10.1021/acsami.9b10438
Liu, S., Lai, C., Liu, X., Li, B., Zhang, C., Qin, L., et al. (2020). Metal-organic Frameworks and Their Derivatives as Signal Amplification Elements for Electrochemical Sensing. Coord. Chem. Rev. 424, 213520. doi:10.1016/j.ccr.2020.213520
Liu, X.-W., Sun, T.-J., Hu, J.-L., and Wang, S.-D. (2016). Composites of Metal-Organic Frameworks and Carbon-Based Materials: Preparations, Functionalities and Applications. J. Mater. Chem. A. 4, 3584–3616. doi:10.1039/C5TA09924B
Liu, Y., and Tang, Z. (2013). Multifunctional Nanoparticle@MOF Core-Shell Nanostructures. Adv. Mater. 25, 5819–5825. doi:10.1002/adma.201302781
Liu, Y., Zhong, L., Zhang, S., Wang, J., and Liu, Z. (2022). An Ultrasensitive and Wearable Photoelectrochemical Sensor for Unbiased and Accurate Monitoring of Sweat Glucose. Sensors Actuators B: Chem. 354, 131204. doi:10.1016/j.snb.2021.131204
Lu, Y., Zhang, S., Dai, S., Liu, D., Wang, X., Tang, W., et al. (2020). Ultrasensitive Detection of Electrolyte Leakage from Lithium-Ion Batteries by Ionically Conductive Metal-Organic Frameworks. Matter 3, 904–919. doi:10.1016/j.matt.2020.05.021
Lu, Z., Zhong, Y., Li, G., and Hu, Y. (2022). Rapid Analysis of Quinoxalines in Feeds by a Fluorescence Sensor Based on Tetraphenylmethane Porous Organic Framework Membrane. Sensors Actuators B: Chem. 356, 131350. doi:10.1016/j.snb.2021.131350
Ma, D.-D., and Zhu, Q.-L. (2020). MOF-based Atomically Dispersed Metal Catalysts: Recent Progress towards Novel Atomic Configurations and Electrocatalytic Applications. Coord. Chem. Rev. 422, 213483. doi:10.1016/j.ccr.2020.213483
Ma, J., Chen, G., Bai, W., and Zheng, J. (2020a). Amplified Electrochemical Hydrogen Peroxide Sensing Based on Cu-Porphyrin Metal-Organic Framework Nanofilm and G-Quadruplex-Hemin DNAzyme. ACS Appl. Mater. Inter. 12, 58105–58112. doi:10.1021/acsami.0c09254
Ma, J., Zhang, X., Zhang, Y., Li, H., Wei, C., Yang, J., et al. (2020b). A New Watermelon Cultivar-'ShanNong No.4'. Acta Hortic. 58, 389–392. doi:10.17660/ActaHortic.2020.1282.58
Meng, W., Wen, Y., Dai, L., He, Z., and Wang, L. (2018). A Novel Electrochemical Sensor for Glucose Detection Based on Ag@ZIF-67 Nanocomposite. Sensors Actuators B: Chem. 260, 852–860. doi:10.1016/j.snb.2018.01.109
Moon, H. R., Lim, D.-W., and Suh, M. P. (2013). Fabrication of Metal Nanoparticles in Metal-Organic Frameworks. Chem. Soc. Rev. 42, 1807–1824. doi:10.1039/C2CS35320B
Mosleh, S., RahimiGhaedi, M. R., Ghaedi, M., Dashtian, K., Hajati, S., and Wang, S. (2017). Ag3PO4/AgBr/Ag-HKUST-1-MOF Composites as Novel Blue LED Light Active Photocatalyst for Enhanced Degradation of Ternary Mixture of Dyes in a Rotating Packed Bed Reactor. Chem. Eng. Process. Process Intensification 114, 24–38. doi:10.1016/j.cep.2017.01.009
Nan, H., Su, Y.-Q., Tang, C., Cao, R., Li, D., Yu, J., et al. (2020). Engineering the Electronic and Strained Interface for High Activity of PdMcore@Ptmonolayer Electrocatalysts for Oxygen Reduction Reaction. Sci. Bull. 65, 1396–1404. doi:10.1016/j.scib.2020.04.015
Nan, Z. A., Fs, B., Cg, B., Lh, B., Zj, A., Mw, B., et al. (2019). Two-dimensional Oriented Growth of Zn-MOF-On-Zr-MOF Architecture: A Highly Sensitive and Selective Platform for Detecting Cancer Markers-ScienceDirect. Biosens.Bioelectron 123, 51–58. doi:10.1016/j.bios.2018.09.079
Otles, S., and Yalcin, B. (2012). Review on the Application of Nanobiosensors in Food Analysis. Acta Sci. Pol. Technol. Aliment. 11, 7–18. doi:10.1007/s11783-011-0280-z
Otles, S., and Yalcin, B. (2012). Review on the Application of Nanobiosensors in Food Analysis. Acta Sci. Pol. Technol. Aliment. 11, 7–18. doi:10.1007/s11783-011-0280-z
Pandey, A., Nikam, A. N., Padya, B. S., Kulkarni, S., Fernandes, G., Shreya, A. B., et al. (2021). Surface Architectured Black Phosphorous Nanoconstructs Based Smart and Versatile Platform for Cancer Theranostics. Coord. Chem. Rev. 435, 213826. doi:10.1016/j.ccr.2021.2138210.1016/j.ccr.2021.213826
Peng, Z., Jiang, Z., and Li, Y. (2016). A Novel Electrochemical Sensor of Tryptophan Based on Silver Nanoparticles/metal-Organic Framework Composite Modified Glassy Carbon Electrode. RSC Adv. 6, 13742–13748. doi:10.1039/C5RA25251B
Petrosillo, N., Viceconte, G., Ergonul, O., Ippolito, G., and Petersen, E. (2020). COVID-19, SARS and MERS: Are They Closely Related? Clin. Microbiol. Infect. 26, 729–734. doi:10.1016/j.cmi.2020.03.026
Qin, L., Lin, L.-X., Fang, Z.-P., Yang, S.-P., Qiu, G.-H., Chen, J.-X., et al. (2016). A Water-Stable Metal-Organic Framework of a Zwitterionic Carboxylate with Dysprosium: a Sensing Platform for Ebolavirus RNA Sequences. Chem. Commun. 52, 132–135. doi:10.1039/C5CC06697B
Qin, L., Wang, X., Liu, Y., and Wei, H. (2018). 2D-metal-organic-framework-nanozyme Sensor Arrays for Probing Phosphates and Their Enzymatic Hydrolysis. Anal. Chem. 90, 9983–9989. doi:10.1021/acs.analchem.8b02428
Reuillard, B., Carrière, S., Le Goff, A., and Cosnier, S. (2017). Biomimetic versus Enzymatic High-Potential Electrocatalytic Reduction of Hydrogen Peroxide on a Functionalized Carbon Nanotube Electrode. Chem. Sci. 6, 5139–5143. doi:10.1039/C5SC01473E
Rosi, N. L., Eckert, J., Eddaoudi, M., Vodak, D. T., Kim, J., O'Keeffe, M., et al. (2003). Hydrogen Storage in Microporous Metal-Organic Frameworks. Science 300, 1127–1129. doi:10.1126/science.1083440
Sabo, M., Henschel, A., Fröde, H., Klemm, E., and Kaskel, S. (2007). Solution Infiltration of Palladium into MOF-5: Synthesis, Physisorption and Catalytic Properties. J. Mater. Chem. 17, 3827–3832. doi:10.1039/B706432B
Samadi-Maybodi, A., Ghasemi, S., and Ghaffari-Rad, H. (2015). A Novel Sensor Based on Ag-Loaded Zeolitic Imidazolate Framework-8 Nanocrystals for Efficient Electrocatalytic Oxidation and Trace Level Detection of Hydrazine. Sensors Actuators B: Chem. 220, 627–633. doi:10.1016/j.snb.2015.05.127
Saraf, M., Rajak, R., and Mobin, S. M. (2016). A Fascinating Multitasking Cu-MOF/rGO Hybrid for High Performance Supercapacitors and Highly Sensitive and Selective Electrochemical Nitrite Sensors. J. Mater. Chem. A. 4, 16432–16445. doi:10.1039/C6TA06470A
Sarycheva, A., Makaryan, T., Maleski, K., Satheeshkumar, E., Melikyan, A., Minassian, H., et al. (2017). Two-dimensional Titanium Carbide (MXene) as Surface-Enhanced Raman Scattering Substrate. J. Phys. Chem. C 121, 19983–19988. doi:10.1021/acs.jpcc.7b08180
Sharma, H., and Mutharasan, R. (2013). Review of Biosensors for Foodborne Pathogens and Toxins. Sensors Actuators B: Chem. 183, 535–549. doi:10.1016/j.snb.2013.03.137
Shen, H., Liu, J., Lei, J., and Ju, H. (2018). A Core-Shell Nanoparticle-Peptide@metal-Organic Framework as pH and Enzyme Dual-Recognition Switch for Stepwise-Responsive Imaging in Living Cells. Chem. Commun. 54, 9155–9158. doi:10.1039/C8CC04621B
Singha, S. S., Mondal, S., BhattacharyaShankar, T. S., Das, L., Sen, K., Satpati, B., et al. (2018). Au Nanoparticles Functionalized 3D-MoS2 Nanoflower: An Efficient SERS Matrix for Biomolecule Sensing. Biosens. Bioelectron. 119, 10–17. doi:10.1016/j.bios.2018.07.061
Smaldone, R. A., Forgan, R. S., Furukawa, H., Gassensmith, J. J., Slawin, M. Z., Yaghi, O. M., et al. (2010). Metal-organic Frameworks from Edible Natural Products. Angew. Chem. 122, 8812–8816. doi:10.1002/anie.20100234310.1002/ange.201002343
Sonkar, P. K., and Ganesan, V. (2015). Synthesis and Characterization of Silver Nanoparticle-Anchored Amine-Functionalized Mesoporous Silica for Electrocatalytic Determination of Nitrite. J. Solid State. Electrochem. 19, 2107–2115. doi:10.1007/s10008-014-2725-3
Tang, H., Cai, S., Xie, S., Wang, Z., Tong, Y., Pan, M., et al. (2016). Metal-Organic-Framework-Derived Dual Metal- and Nitrogen-Doped Carbon as Efficient and Robust Oxygen Reduction Reaction Catalysts for Microbial Fuel Cells. Adv. Sci. 3, 1500265. doi:10.1002/advs.201500265
Turner, S., Lebedev, O. I., Schröder, F., Esken, D., Fischer, R. A., and Tendeloo, G. V. (2008). Direct Imaging of Loaded Metal-Organic Framework Materials (Metal@MOF-5). Chem. Mater. 20, 5622–5627. doi:10.1021/cm801165s
Valekar, A. H., Batule, B. S., Kim, M. I., Cho, K.-H., Hong, D.-Y., Lee, U.-H., et al. (2018). Novel Amine-Functionalized Iron Trimesates with Enhanced Peroxidase-like Activity and Their Applications for the Fluorescent Assay of Choline and Acetylcholine. Biosens. Bioelectron. 100, 161–168. doi:10.1016/j.bios.2017.08.056
Wang, C., Langrock, C., Marandi, A., Jankowski, M., Zhang, M., Desiatov, B., et al. (2018). Ultrahigh-efficiency Wavelength Conversion in Nanophotonic Periodically Poled Lithium Niobate Waveguides. Optica 5, 1438–1441. doi:10.1364/OPTICA.5.001438
Wang, L., Teng, Q., Sun, X., Chen, Y., Wang, Y., Wang, H., et al. (2018). Facile Synthesis of Metal-Organic Frameworks/ordered Mesoporous Carbon Composites with Enhanced Electrocatalytic Ability for Hydrazine. J. Colloid Interf. Sci. 512, 127–133. doi:10.1016/j.jcis.2017.10.050
Wang, L., Yang, H., He, J., Zhang, Y., Yu, J., and Song, Y. (2016). Cu-hemin Metal-Organic-Frameworks/chitosan-Reduced Graphene Oxide Nanocomposites with Peroxidase-like Bioactivity for Electrochemical Sensing. Electrochimica Acta 213, 691–697. doi:10.1016/j.electacta.2016.07.162
Wang, Y., Wang, L., Huang, W., Zhang, T., Hu, X., Perman, J. A., et al. (2017). A Metal-Organic Framework and Conducting Polymer Based Electrochemical Sensor for High Performance Cadmium Ion Detection. J. Mater. Chem. A. 5, 8385–8393. doi:10.1039/C7TA01066D
Wang, Y., Wu, Y., Xie, J., and Hu, X. (2013). Metal-organic Framework Modified Carbon Paste Electrode for lead Sensor. Sensors Actuators B: Chem. 177, 1161–1166. doi:10.1016/j.snb.2012.12.048
Wang, Y., Zhao, G., Zhang, G., Zhang, Y., Wang, H., Cao, W., et al. (2020). An Electrochemical Aptasensor Based on Gold-Modified MoS2/rGO Nanocomposite and Gold-Palladium-Modified Fe-MOFs for Sensitive Detection of lead Ions. Sensors Actuators B: Chem. 319, 128313. doi:10.1016/j.snb.2020.128313
Wang, Z., Jin, H., Meng, T., Liao, K., Meng, W., Yang, J., et al. (2018). Fe, Cu-Coordinated ZIF-Derived Carbon Framework for Efficient Oxygen Reduction Reaction and Zinc-Air Batteries. Adv. Funct. Mater. 28, 1802596. doi:10.1002/adfm.201802596
Wang, Z., Wang, H., Zeng, Z., Zeng, G., Xu, P., Xiao, R., et al. (2020). Metal-organic Frameworks Derived Bi2O2CO3/porous Carbon Nitride: A Nanosized Z-Scheme Systems with Enhanced Photocatalytic Activity. Appl. Catal. B: Environ. 267, 118700. doi:10.1016/j.apcatb.2020.118700
Wen, J., Li, X., Liu, W., Fang, Y., Xie, J., and Xu, Y. (2015). Photocatalysis Fundamentals and Surface Modification of TiO2 Nanomaterials. Chin. J. Catal. 36, 2049–2070. doi:10.1016/S1872-2067(15)60999-8
Wengert, S., Albrecht, J., Ruoss, S., Stahl, C., Schütz, G., and Schäfer, R. (2017). Smooth and Rapid Microwave Synthesis of MIL-53(Fe) Including Superparamagnetic γ-Fe2O3 Nanoparticles. J. Magnetism Magn. Mater. 444, 168–172. doi:10.1016/j.jmmm.2017.08.013
Xie, B.-P., Qiu, G.-H., Hu, P.-P., Liang, Z., Liang, Y.-M., Sun, B., et al. (2018). Simultaneous Detection of Dengue and Zika Virus RNA Sequences with a Three-Dimensional Cu-Based Zwitterionic Metal-Organic Framework, Comparison of Single and Synchronous Fluorescence Analysis. Sensors Actuators B: Chem. 254, 1133–1140. doi:10.1016/j.snb.2017.06.085
Yang, H., Han, X., Douka, A. I., Huang, L., Gong, L., Xia, C., et al. (2020). Advanced Oxygen Electrocatalysis in Energy Conversion and Storage. Adv. Funct. Mater. 31, 2007602. doi:10.1002/adfm.202007602
Yang, H. M., Song, X. L., Yang, T. L., Liang, Z. H., Fan, C. M., and Hao, X. G. (2014). Electrochemical Synthesis of Flower Shaped Morphology MOFs in an Ionic Liquid System and Their Electrocatalytic Application to the Hydrogen Evolution Reaction. RSC Adv. 4, 15720–15726. doi:10.1039/C3RA47744D
Yang, J., Zhao, F., and Zeng, B. (2015). One-step Synthesis of a Copper-Based Metal-Organic Framework-Graphene Nanocomposite with Enhanced Electrocatalytic Activity. RSC Adv. 5, 22060–22065. doi:10.1039/c4ra16950f
Yang, Q., Xu, Q., and Jiang, H.-L. (2017). Metal-organic Frameworks Meet Metal Nanoparticles: Synergistic Effect for Enhanced Catalysis. Chem. Soc. Rev. 46, 4774–4808. doi:10.1039/C6CS00724D
Yang, S., Karve, V. V., Justin, A., Kochetygov, I., Espín, J., Asgari, M., et al. (2021). Enhancing MOF Performance through the Introduction of Polymer Guests. Coord. Chem. Rev. 427, 213525. doi:10.1016/j.ccr.2020.213525
Yang, T., Yang, H., Zhen, S. J., and Huang, C. Z. (2015). Hydrogen-bond-mediated In Situ Fabrication of AgNPs/Agar/PAN Electrospun Nanofibers as Reproducible SERS Substrates. ACS Appl. Mater. Inter. 7, 1586–1594. doi:10.1126/science.108344010.1021/am507010q
Yang, Y., Zeng, Z., Zhang, C., Huang, D., Zeng, G., Xiao, R., et al. (2018). Construction of Iodine Vacancy-Rich BiOI/Ag@AgI Z-Scheme Heterojunction Photocatalysts for Visible-Light-Driven Tetracycline Degradation: Transformation Pathways and Mechanism Insight. Chem. Eng. J. 349, 808–821. doi:10.1016/j.cej.2018.05.093
Yang, Z.-H., Ren, S., Zhuo, Y., Yuan, R., and Chai, Y.-Q. (2017). Cu/Mn Double-Doped CeO2 Nanocomposites as Signal Tags and Signal Amplifiers for Sensitive Electrochemical Detection of Procalcitonin. Anal. Chem. 89, 13349–13356. doi:10.1021/acs.analchem.7b03502
Yang, Z., Zhang, W., Yin, Y., Fang, W., and Xue, H. (2022). Metal-organic Framework-Based Sensors for the Detection of Toxins and Foodborne Pathogens. Food Control 133, 108684. doi:10.1016/j.foodcont.2021.108684
Yang, Z., Zhou, X., Yin, Y., and Fang, W. (2021). Determination of Nitrite by noble Metal Nanomaterial-Based Electrochemical Sensors: A Minireview. Anal. Lett. 54, 2826–2850. doi:10.1080/00032719.2021.1897134
Yao, M.-S., Li, W.-H., and Xu, G. (2021). Metal-organic Frameworks and Their Derivatives for Electrically-Transduced Gas Sensors. Coord. Chem. Rev. 426, 213479. doi:10.1016/j.ccr.2020.213479
Yassine, O., Shekhah, O., Assen, A. H., Belmabkhout, Y., Salama, K. N., and Eddaoudi, M. (2016). H2S Sensors: Fumarate-Based Fcu-MOF Thin Film Grown on a Capacitive Interdigitated Electrode. Angew. Chem. Int. Ed. 55, 15879–15883. doi:10.1002/anie.201608780
Yi, F.-Y., Chen, D., Wu, M.-K., Han, L., and Jiang, H.-L. (2016). Chemical Sensors Based on Metal-Organic Frameworks. ChemPlusChem 81, 675–690. doi:10.1002/cplu.201600137
Yuan, B., Zhang, R., Jiao, X., Li, J., Shi, H., and Zhang, D. (2014). Amperometric Determination of Reduced Glutathione with a New Co-based Metal-Organic Coordination Polymer Modified Electrode. Electrochemistry Commun. 40, 92–95. doi:10.1016/j.elecom.2014.01.006
Zamzami, M. A., Rabbani, G., Ahmad, A., Basalah, A. A., Al-Sabban, W. H., Nate Ahn, S., et al. (2022). Carbon Nanotube Field-Effect Transistor (CNT-FET)-based Biosensor for Rapid Detection of SARS-CoV-2 (COVID-19) Surface Spike Protein S1. Bioelectrochemistry 143, 107982. doi:10.1016/j.bioelechem.2021.107982
Zhang, M., Gu, Z.-Y., Bosch, M., Perry, Z., and Zhou, H.-C. (2015). Biomimicry in Metal-Organic Materials. Coord. Chem. Rev. 293-294, 327–356. doi:10.1016/j.ccr.2014.05.031
Zhang, T., and Lin, W. (2014). Metal-organic Frameworks for Artificial Photosynthesis and Photocatalysis. Chem. Soc. Rev. 43, 5982–5993. doi:10.1039/c4cs00103f
Zhang, X., Jiang, Y., Zhu, M., Xu, Y., Guo, Z., Shi, J., et al. (2020). Electrochemical DNA Sensor for Inorganic Mercury(II) Ion at Attomolar Level in Dairy Product Using Cu(II)-anchored Metal-Organic Framework as Mimetic Catalyst. Chem. Eng. J. 383, 123182. doi:10.1016/j.cej.2019.123182
Zhang, X., Li, G., Wu, D., Li, X., Hu, N., Chen, J., et al. (2019). Recent Progress in the Design Fabrication of Metal-Organic Frameworks-Based Nanozymes and Their Applications to Sensing and Cancer Therapy. Biosens. Bioelectron. 137, 178–198. doi:10.1016/j.bios.2019.04.061
Zhang, Y., Brooks, W. A., Goswami, D., Rahman, M., Luby, S. P., and Erdman, D. D. (2014). A Duplex Recombinant Viral Nucleoprotein Microbead Immunoassay for Simultaneous Detection of Seroresponses to Human Respiratory Syncytial Virus and Metapneumovirus Infections. J. Virol. Methods 206, 55–62. doi:10.1016/j.jviromet.2014.05.008
Zhang, Z., Ji, H., Song, Y., Zhang, S., Wang, M., Jia, C., et al. (2017). Fe(III)-based Metal-Organic Framework-Derived Core-Shell Nanostructure: Sensitive Electrochemical Platform for High Trace Determination of Heavy Metal Ions. Biosens. Bioelectron. 94, 358–364. doi:10.1016/j.bios.2017.03.014
Zhou, B., Liang, L.-M., and Yao, J. (2014). Effects of Isomorphous Substitution of a Coordination Polymer on the Properties and its Application in Electrochemical Sensing. J. Solid State. Chem. 215, 109–113. doi:10.1016/j.jssc.2014.03.028
Zhou, Q., Lin, Y., Zhang, K., Li, M., and Tang, D. (2018). Reduced Graphene oxide/BiFeO3 Nanohybrids-Based Signal-On Photoelectrochemical Sensing System for Prostate-specific Antigen Detection Coupling with Magnetic Microfluidic Device. Biosens. Bioelectron. 101, 146–152. doi:10.1016/j.bios.2017.10.027
Zhou, X., Zeng, Z., Zeng, G., Lai, C., Xiao, R., Liu, S., et al. (2020). Persulfate Activation by Swine Bone Char-Derived Hierarchical Porous Carbon: Multiple Mechanism System for Organic Pollutant Degradation in Aqueous media. Chem. Eng. J. 383, 123091. doi:10.1016/j.cej.2019.123091
Zhou, Y., Hu, Q., Yu, F., Ran, G.-Y., Wang, H.-Y., Shepherd, N. D., et al. (2020). A Metal-Organic Framework Based on a Nickel Bis(dithiolene) Connector: Synthesis, crystal Structure, and Application as an Electrochemical Glucose Sensor. J. Am. Chem. Soc. 142, 20313–20317. doi:10.1021/jacs.0c09009
Zhu, N.-N., Liu, X.-H., Li, T., Ma, J.-G., Cheng, P., and Yang, G.-M. (2017). Composite System of Ag Nanoparticles and Metal-Organic Frameworks for the Capture and Conversion of Carbon Dioxide under Mild Conditions. Inorg. Chem. 56, 3414–3420. doi:10.1021/acs.inorgchem.6b02855
Keywords: metal–organic frameworks (MOFs), electrochemical sensors, composites, sensitivity, stability
Citation: Chen Y, Yang Z, Hu H, Zhou X, You F, Yao C, Liu FJ, Yu P, Wu D, Yao J, Hu R, Jiang X and Yang H (2022) Advanced Metal–Organic Frameworks-Based Catalysts in Electrochemical Sensors. Front. Chem. 10:881172. doi: 10.3389/fchem.2022.881172
Received: 22 February 2022; Accepted: 08 March 2022;
Published: 31 March 2022.
Edited by:
Fei Xiao, Huazhong University of Science and Technology, ChinaCopyright © 2022 Chen, Yang, Hu, Zhou, You, Yao, Liu, Yu, Wu, Yao, Hu, Jiang and Yang. This is an open-access article distributed under the terms of the Creative Commons Attribution License (CC BY). The use, distribution or reproduction in other forums is permitted, provided the original author(s) and the copyright owner(s) are credited and that the original publication in this journal is cited, in accordance with accepted academic practice. No use, distribution or reproduction is permitted which does not comply with these terms.
*Correspondence: Ruofei Hu, rhuvip@hbuas.edu.cn; Xueliang Jiang, jiangxl@wit.edu.cn; Huan Yang, yangh@wit.edu.cn