- 1Department of Chemistry and Biochemistry, Utah State University, Logan, UT, United States
- 2Laboratoire de Chimie Supramoléculaire, Institut de Science et d’Ingénierie, Supramoléculaires Université de Strasbourg, Strasbourg, France
A series of complexes of Na, K, NH4, and H3O with [bpy.bpy.bpy]cryptand, [2.2.2]cryptand, and spherical cryptand were investigated via DFT and ab initio methods. We found that by coating Rydberg molecules with the “organic skin” one could further decrease their ionization potential energy, reaching the values of ∼1.5 eV and a new low record of 1.3 eV. The neutral cryptand complexes in this sense possess a weakly bounded electron and may be considered as very strong reducing agents. Moreover, the presence of an organic cage increases the thermodynamic stability of Rydberg molecules making them stable toward the proton detachment.
Introduction
The [2.2.2]cryptand and spherical cryptand (Scheme 1A, B) invented by Lehn (Lehn, 1977), have been a subject of both theoretical (Elroby et al., 2006; Elroby, 2009; Puchta et al., 2019; Isaeva et al., 2021; Ćoćić et al., 2021; Ariyarathna, 2022) and experimental (Lehn, 1977; Lehn, 1978; Lehn, 1979; Lehn, 1980; Echegoyen et al., 1991; Arnaud-Neu et al., 2002; Cram et al., 2002; Izatt et al., 2002; Miyamoto et al., 2002; Badjić et al., 2011; Chung et al., 2020) studies for decades. The discovery of those fascinating compounds opened a huge field of supramolecular chemistry. Their unique guest particle selectivity and extremely low ionization potentials of neutral alkali-metal complexes (Cram and Lein, 1985; Huang et al., 1988; Kim et al., 1999) found an application in synthetic organic and inorganic chemistry. In particular, a huge number of multiply-charged Zintl anions with unusual structures have been synthesized using the popular [K⊂[2.2.2]cryptand] complex (Sun et al., 2018; Tkachenko et al., 2020; Wang et al., 2020).
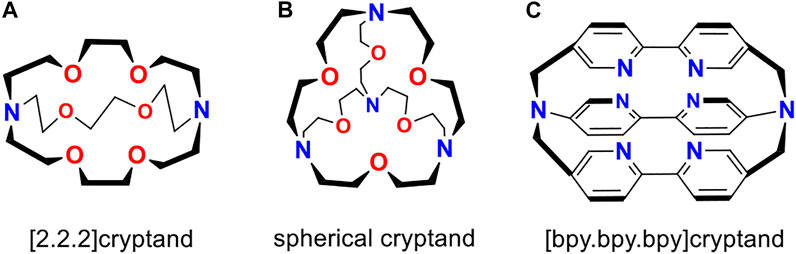
SCHEME 1. The structures of organic cages considered in this work. [2.2.2]cryptand (A), spherical cryptand (B), and [bpy.bpy.bpy]cryptand (C) are shown.
Firstly, introduced by Gutsev and Boldyrev (Gutsev and Boldyrev, 1982), the family of superalkalis has been growing significantly. Despite the initially proposed Mk+1L family, where M is an alkali atom and L is an electronegative atom of valence k, other superalkalis have been proposed and synthesized. Along with other inorganic binuclear superalkali (Tong et al., 2009), the definition of superalkalis was extended to polynuclear species such as polynuclear aromatic superalkalis (Sun et al., 2013; Parida et al., 2018), superalkali cations (Tong et al., 2011; Tong et al., 2012a; Tong et al., 2012b; Hou et al., 2013), organo-Zintl clusters (Giri et al., 2016; Reddy and Giri, 2016). Another family of compounds with low ionization potential is Rydberg molecules. Vivid examples of Rydberg molecules are NH4 and H3O neutral species, whose unpa`ired electron occupies a diffuse orbital around the molecule. It has been shown that such Rydberg molecules are not long-living particles. Although the Td structure of NH4 radical is a local minimum, it is only a metastable molecule and undergoes a dissociation into NH3 and H* radical species (Herzberg, 1981; Signorell et al., 1997). Similar behavior is found for H3O neutral species (Luo and Jungen, 1999; Melin et al., 2005). It has been shown before that cryptand compounds can bind both NH4+ and H3O+ cations with a great selectivity (Cram et al., 1985; Behr et al., 2002; Junk, 2008). Thus, it will be interesting to investigate the electronic properties of neutral [R⊂cryptand] (R = NH4, H3O) complexes, since the organic coating could stabilize the Rydberg molecules and decrease their ionization potential as it was observed for alkali metal complexes (Cram and Lein, 1985; Huang et al., 1988; Kim et al., 1999). In this work, we investigate the electronic properties of coated Rydberg molecules via DFT and ab initio methods and compare their properties with alkali-metal cryptand complexes.
Computational Methods
All structures were optimized using Perdew–Burke-Ernzerhof (PBE0) (Perdew et al., 1996) and Tao-Perdew-Staroverov-Scuseria (TPSSh) (Staroverov et al., 2003) hybrid functionals using def2-SVP basis set (Weigend and Ahlrichs, 2005). The frequency calculations were performed at the same level of theory. No imaginary frequencies were present, showing that the optimized structures are at local minima on the given PES. Ionization potentials were calculated at three different levels of theory. In particular, the single-point calculations at optimized geometry using DFT functionals (PBE0 and TPSSh) and a moderately large basis set def2-TZVPPD (Weigend and Ahlrichs, 2005) were carried out. In addition, single-point calculations using MP2 level of theory with cc-pvdz (C, N, O atoms) and aug-cc-pvdz (H, K, Na atoms) basis sets (Dunning, 1989; Kendall et al., 1992; Hill and Peterson, 2017) were performed. For convenience, we will denote this combination of basis functions as Basis-1. Due to the large values of spin contamination, the [bpy.bpy.bpy]cryptand complexes were calculated using ROHF-MBPT2 formalism (Lauderdale et al., 1991; Lauderdale et al., 1992). The vertical ionization potential (VIP) was calculated as the energy difference between the optimized neutral complex and the cation in the geometry of the neutral complex. The adiabatic ionization potential (AIP) was calculated as the energy differences between an optimized neutral cluster and an optimized cation. The natural charge distribution was calculated via NBO method as implemented in NBO7 software (Glendening et al., 2019). The topology analysis of electron localization function (ELF) (Silvi and Savin, 1994) was performed with the Multiwfn program (Lu and Chen, 2012). All calculations were performed with Gaussian 16 program (Frisch et al., 2016). The visualization of SOMO orbitals and geometries of the investigated species were performed using IboView software (Knizia, 2013; Knizia and Klein, 2015).
Results and Discussion
The neutral [Na⊂[bpy.bpy.bpy]cryptand] was firstly synthesized in 1991 by Lehn and coworkers (Echegoyen et al., 1991) through the electrochemical reduction of [Na+⊂[bpy.bpy.bpy]cryptand] cation. This approach potentially can be used for the synthesis of superalkali cryptand complexes with Rydberg molecules. To investigate the electronic properties of such species, we chose three different organic cages ([2.2.2]cryptand, [bpy.bpy.bpy]cryptand, and spherical cryptand) that are very promising candidates for the capturing of NH4 and H3O species. The structures of those cages are given in Scheme 1. For the comparison of ionization potentials, two alkali metal complexes were also considered. In particular [Na⊂[bpy.bpy.bpy]cryptand] was chosen as the first synthesized cryptand-superalkali species, and [K⊂[2.2.2]cryptand] was chosen as one of the most popular examples of alkali metal macrocyclic complex.
The geometries of neutral and cationic complexes were optimized with two different DFT hybrid functionals. It was shown before that PBE0 and TPSSh functionals can provide accurate geometries for macrocyclic and cryptand complexes (Tkachenko et al., 2019). The optimized geometries are consistent within two methods, showing the functional independence of the results. The geometries of neutral species are only slightly distorted from the geometries of cationic species, showing that the additional electron of neutral complexes does not participate in a significant bonding formation process. The optimized structures of selected neutral species are given in Figure 1. Cartesian coordinates of all optimized structures are provided in the Supporting Information file (Supplementary Table S1). The natural charge distribution of neutral species showed that the negative charge is mainly distributed over the oxygen and nitrogen atoms of the organic ligand, while the central unit (either H3O or NH4 species) formally possesses a +1 positive charge. In particular 0.757–0.806 and 0.794–0.876 positive natural charges on H3O and NH4 molecules, respectively, were found in investigated complexes. This might be one of the key reasons for the stabilization of those Rydberg molecules, which are thermodynamically unstable toward dissociation of a proton in their naked form.
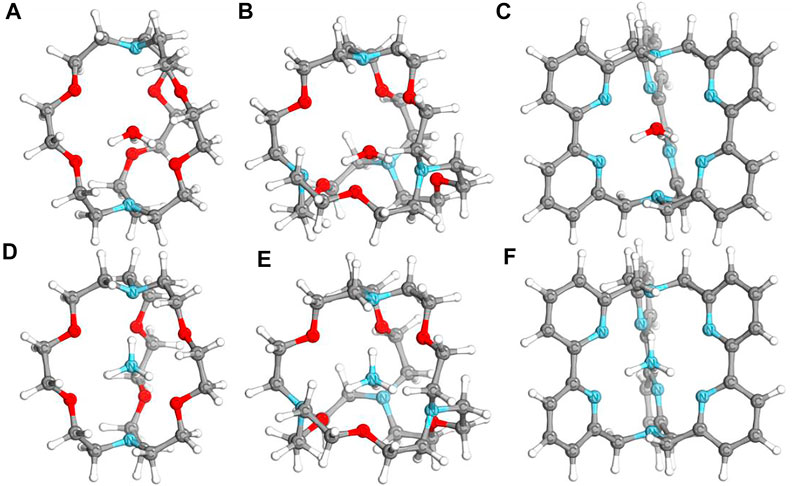
FIGURE 1. Structures of optimized neutral complexes of NH4 and H3O. (A) [H3O⊂[2.2.2]cryptand]; (B) [H3O⊂spherical cryptand]; (C) [H3O⊂[bpy.bpy.bpy]cryptand]; (D) [NH4⊂[2.2.2]cryptand]; (E) [NH4⊂spherical cryptand]; (F) [NH4⊂[bpy.bpy.bpy]cryptand].
To illustrate the enhanced stability of encapsulated neutral molecules we performed calculations of dissociation energies for both naked and coated species. The reaction that was considered is a dissociation of a proton from the central unit with a formal reaction:

TABLE 1. Free energies [kcal/mol] for the dissociation reaction of hydrogen radical from the central unit of investigated species calculated at TPSSh/def2-TZVPPD//TPSSh/def2-SVP level.
Interestingly, for both NH4 and H3O, a significant decrease in ionization potentials was found after encapsulating the corresponding Rydberg molecules into organic cages. Particularly, the naked NH4 and H3O molecules possess 4.57 and 5.55 eV VIP, respectively. Whereas the NH4 and H3O encapsulated in [2.2.2]cryptand and spherical cryptand possess ionization potentials about 3–4 eV lower than the naked species (Table 2). Interestingly [bpy.bpy.bpy]cryptand systems show larger IPs by ∼1.1 eV. A similar but not so pronounced pattern was found for alkali metals encapsulated in the [bpy.bpy.bpy]cryptand. The nature of such an increase in IPs is discussed below and related to the presence of a diffuse SOMO orbital in the system. We note, that the obtained IPs for NH4 and H3O species are even lower than IPs of [K⊂[2.2.2]cryptand] which was shown before to be a superalkali with record low ionization potential (Tkachenko et al., 2019).
Similar results were obtained using PBE0 and TPSSh functionals with def2-TZVPPD basis set. Although the values of IPs are slightly higher than it was obtained for the MP2 method, the main trends preserve the same (Table 3).
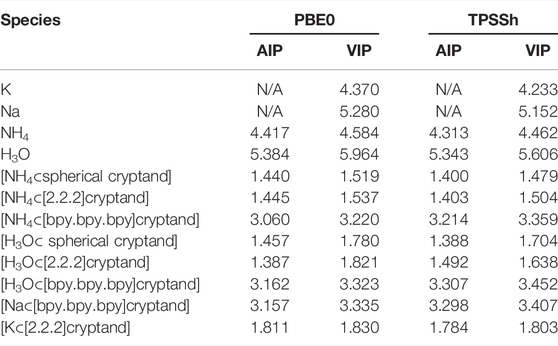
TABLE 3. Values of VIP and AIP [eV] obtained using PBE0 and TPSSh functionals with def2-TZVPPD basis set.
To illustrate the diffuse nature of SOMO of investigated species, we plotted the isosurface graphs of corresponding orbitals shown in Figure 2 (the orbitals were obtained from quasi-restricted orbitals formalism). We can see that for [2.2.2]cryptand and spherical cryptand complexes (Figures 2A,B,D,E), SOMO orbitals have a diffuse nature and surround the whole molecule entirely. In contrast, the unpaired electron of [bpy.bpy.bpy]cryptand complexes sit on the antibonding orbital of a π-conjugated system (Figures 2C,F). Isosurface plots of SOMO visualized with a different contour value can be found in the supporting information file (Supplementary Figure S1). Such an interesting difference in SOMO can be explained by the fact that different organic cages form different types of complexes with Rydberg molecules. Thus [2.2.2]cryptand and spherical cryptand complexes behave as electrides, possessing an electron density outside of the molecule, whereas [bpy.bpy.bpy]cryptand complexes form an ionic molecular compound bearing a negative charge entirely on the organic ligand. Such behavior can also be explained by the possibility of bipyridine molecules to form stable anionic species, that were experimentally isolated before (Bock et al., 1999; Gore-Randall et al., 2009). To further show the differences between the two types of complexes we performed an ELF basins analysis. The basins laying outside of the molecule were found for [2.2.2]cryptand and spherical cryptand complexes (Supplementary Figure S2). The integration of the electron density within the volume of the found ELF basins resulted in 0.3–0.6 |e| basins occupancy. In turn, no outside lying ELF basins were found for [bpy.bpy.bpy]cryptand complexes. Thus [2.2.2]cryptand and spherical cryptand complexes demonstrate an electride nature which is the reason for their lower IP values in comparison to [bpy.bpy.bpy]cryptand complexes.
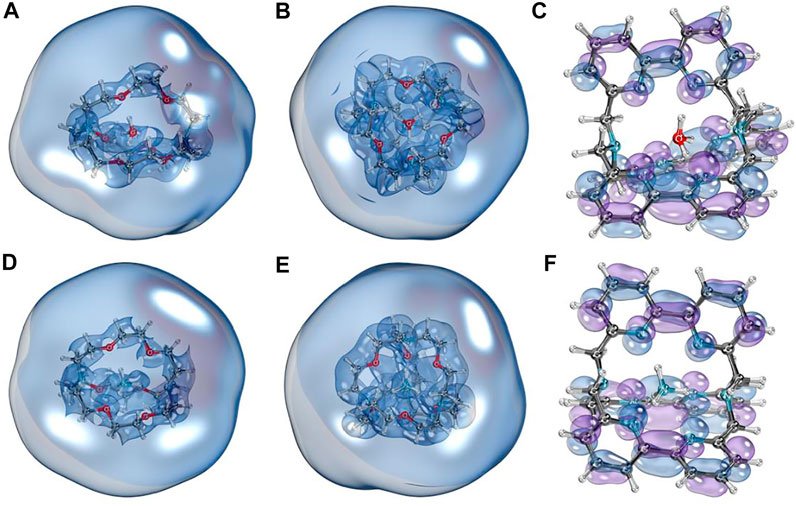
FIGURE 2. Isosurface plots of singly occupied molecular orbitals of NH4 and H3O complexes. (A) [H3O⊂[2.2.2]cryptand]; (B) [H3O⊂spherical cryptand]; (C) [H3O⊂[bpy.bpy.bpy]cryptand]; (D) [NH4⊂[2.2.2]cryptand]; (E) [NH4⊂spherical cryptand]; (F) [NH4⊂[bpy.bpy.bpy]cryptand]. A threshold of 100% was used for A, B, D, E to illustrate the diffuse nature of those orbitals. For C and F a lower 80% threshold was used for visualization.
Conclusion
In this work we investigated the electronic properties of Rydberg molecules coated with cryptand organic cages. We showed that it is possible to significantly decrease the values of the ionization potentials by covering Rydberg molecules with an “organic skin.” In particular, we found that the IP could be decreased, reaching the values of ∼1.5 eV and a new low record of 1.3 eV (at MP2/Basis-1 level). In addition, the coating ligand can increase the thermodynamic stability of a Rydberg molecule, opening an opportunity to obtain such strong reducing agents in the experiment.
Data Availability Statement
The additional data that support the findings of this study are available from the corresponding author on a reasonable request.
Author Contributions
All authors listed have made a substantial, direct, and intellectual contribution to the work and approved it for publication. NVT and PR performed the quantum chemical calculations and analyzed the data. AIB and JML conceived and designed the project.
Funding
AIB acknowledges financial support from the R. Gaurth Hansen Professorship fund.
Conflict of Interest
The authors declare that the research was conducted in the absence of any commercial or financial relationships that could be construed as a potential conflict of interest.
Publisher’s Note
All claims expressed in this article are solely those of the authors and do not necessarily represent those of their affiliated organizations or those of the publisher, the editors, and the reviewers. Any product that may be evaluated in this article, or claim that may be made by its manufacturer, is not guaranteed or endorsed by the publisher.
Acknowledgments
The support and resources from the Centre for High Performance Computing at the University of Utah are gratefully acknowledged. The authors thank the reviewer 1 for proposing a discussion on electride nature of [2.2.2]cryptand and spherical cryptand complexes.
Supplementary Material
The Supplementary Material for this article can be found online at: https://www.frontiersin.org/articles/10.3389/fchem.2022.880804/full#supplementary-material
References
Ariyarathna, I. R. (2022). Superatomic Chelates: The Cases of Metal Aza-Crown Ethers and Cryptands. Inorg. Chem. 61 (1), 579–585. doi:10.1021/ACS.INORGCHEM.1C03261
Arnaud-Neu, F., Spiess, B., and Schwing-Weill, M. J. (2002). Solvent Effects in the Complexation of [2]-Cryptands and Related Monocycles with Transition- and Heavy-Metal Cations. J. Am. Chem. Soc. 104 (21), 5641–5645. doi:10.1021/JA00385A014
Badjić, J. D., Stojanović, S., and Ruan, Y. (2011). Kinetically and Thermodynamically Controlled Syntheses of Covalent Molecular Capsules. Adv. Phys. Org. Chem. 45, 1–37. doi:10.1016/B978-0-12-386047-7.00001-1
Behr, J. P., Dumas, P., and Moras, D. (2002). The Oxonium (H3O+) Cation: Molecular Structure of an Oxonium-Macrocyclic Polyether Complex. J. Am. Chem. Soc. 104 (17), 4540–4543. doi:10.1021/JA00381A007
Bock, H., Lehn, J. M., Pauls, J., Holl, S., Krenzel, V., Rousset, C. J., et al. (1999). Sodium Salts of the Bipyridine Dianion: Polymer [(bpy)2−{Na+(dme)}2]∞, Cluster [(Na8O)6+ Na+6(bpy)2−6 (Tmeda)6, and Monomer [(bpy)2−{Na+(Pmdta)}2]. Angew. Chem. Intl Edit 38 (7), 952–955. doi:10.1002/(sici)1521-3773(19990401)38:7<952:aid-anie952>3.0.co;2-#
Chung, A. B., Huh, D. N., Ziller, J. W., and Evans, W. J. (2020). 2.2.2-Cryptand as a Bidentate Ligand in Rare-Earth Metal Chemistry. Inorg. Chem. Front. 7 (22), 4445–4451. doi:10.1039/D0QI00746C
Ćoćić, D., Manaa, A., Siegl, S., Puchta, R., and Eldik, R. (2021). [3.2.1] and [4.1.1] Isomers of Lehn's [2.2.2] Cryptand: Prediction of Ion Selectivity by Quantum Chemical Calculations XV**. Z. Anorg. Allg. Chem. 647 (8), 915–921. doi:10.1002/ZAAC.202000452
Cram, D. J., Kaneda, T., Helgeson, R. C., Brown, S. B., Knobler, C. B., Maverick, E., et al. (1985). Host-guest Complexation. 35. Spherands, the First Completely Preorganized Ligand Systems. J. Am. Chem. Soc. 107 (12), 3645–3657. doi:10.1021/JA00298A040
Cram, D. J., Kaneda, T., Helgeson, R. C., and Lein, G. M. (2002). Spherands - Ligands Whose Binding of Cations Relieves Enforced Electron-Electron Repulsions. J. Am. Chem. Soc. 101 (22), 6752–6754. doi:10.1021/JA00516A048
Cram, D. J., and Lein, G. M. (1985). Host-guest Complexation. 36. Spherand and Lithium and Sodium Ion Complexation Rates and Equilibria. J. Am. Chem. Soc. 107 (12), 3657–3668. doi:10.1021/JA00298A041
Dunning, T. H. (1989). Gaussian Basis Sets for Use in Correlated Molecular Calculations. I. The Atoms boron through Neon and Hydrogen. J. Chem. Phys. 90 (2), 1007–1023. doi:10.1063/1.456153
Echegoyen, L., DeCian, A., Fischer, J., and Lehn, J.-M. (1991). Cryptatium: A Species of Expanded Atom/Radical Ion Pair Type from Electroreductive Crystallization of the Macrobicyclic Sodium Tris(Bipyridine) Cryptate. Angew. Chem. Int. Ed. Engl. 30 (7), 838–840. doi:10.1002/ANIE.199108381
Elroby, S. A. K. (2009). The Effect of Donor Atoms on the Complexation of Alkali Cations with Spherands: A Density Functional Investigation. Int. J. Quan. Chem. 109 (7), 1515–1521. doi:10.1002/QUA.21970
Elroby, S. A., Lee, K. H., Cho, S. J., and Hinchliffe, A. (2006). A DFT Study of Spherands Containing Five Anisyl Groups - Highly Preorganized to Bind the Alkali Metal. Can. J. Chem. 84 (8), 1045–1049. doi:10.1139/V06-130
Frisch, M. J., Trucks, G. W., Schlegel, H. B., Scuseria, G. E., Robb, M. A., Cheeseman, J. R., et al. (2016). Gaussian 16, Revision B.01. Wallingford, CT: Gaussian, Inc.
Giri, S., Reddy, G. N., and Jena, P. (2016). Organo-Zintl Clusters [P7R4]: A New Class of Superalkalis. J. Phys. Chem. Lett. 7 (5), 800–805. doi:10.1021/ACS.JPCLETT.5B02892/SUPPL_FILE/JZ5B02892_LIVESLIDES.MP4
Glendening, E. D., Landis, C. R., and Weinhold, F. (2019). NBO 7.0 : New Vistas in Localized and Delocalized Chemical Bonding Theory. J. Comput. Chem. 40 (25), 2234–2241. doi:10.1002/JCC.25873
Gore-Randall, E., Irwin, M., Denning, M. S., and Goicoechea, J. M. (2009). Synthesis and Characterization of Alkali-Metal Salts of 2,2′- and 2,4′-Bipyridyl Radicals and Dianions. Inorg. Chem. 48 (17), 8304–8316. doi:10.1021/IC9009459
Gutsev, G. L., and Boldyrev, A. I. (1982). DVM Xα Calculations on the Electronic Structure of “superalkali” Cations. Chem. Phys. Lett. 92 (3), 262–266. doi:10.1016/0009-2614(82)80272-8
Herzberg, G. (1981). Rydberg Spectra of Triatomic Hydrogen and of the Ammonium Radical. Faraday Discuss. Chem. Soc. 71 (0), 165–173. doi:10.1039/DC9817100165
Hill, J. G., and Peterson, K. A. (2017). Gaussian Basis Sets for Use in Correlated Molecular Calculations. XI. Pseudopotential-Based and All-Electron Relativistic Basis Sets for Alkali Metal (K-Fr) and Alkaline Earth (Ca-Ra) Elements. J. Chem. Phys. 147 (24), 244106. doi:10.1063/1.5010587
Hou, N., Li, Y., Wu, D., and Li, Z.-R. (2013). Do Nonmetallic Superalkali Cations Exist? Chem. Phys. Lett. 575, 32–35. doi:10.1016/J.CPLETT.2013.05.014
Huang, R. H., Faber, M. K., Moeggenborg, K. J., Ward, D. L., and Dye, J. L. (1988). Structure of K+(cryptand[2.2.2]) Electride and Evidence for Trapped Electron Pairs. Nature 331 (6157), 599–601. doi:10.1038/331599a0
Isaeva, V. A., Gamov, G. A., and Sharnin, V. A. (2021). Quantum-Chemical Calculations and Stability Analysis of Copper(II) Complexes with Cryptand[2.2.2]. Russ. J. Inorg. Chem. 66 (11), 1696–1702. doi:10.1134/S0036023621110097
Izatt, R. M., Bradshaw, J. S., Nielsen, S. A., Lamb, J. D., Christensen, J. J., and Sen, D. (2002). Thermodynamic and Kinetic Data for Cation-Macrocycle Interaction. Chem. Rev. 85 (4), 271–339. doi:10.1021/CR00068A003
Junk, P. C. (2008). Crown Ethers as Stabilising Ligands for Oxonium Ions. New J. Chem. 32 (5), 762–773. doi:10.1039/B800122G
Kendall, R. A., Dunning, T. H., and Harrison, R. J. (1992). Electron Affinities of the First‐row Atoms Revisited. Systematic Basis Sets and Wave Functions. J. Chem. Phys. 96 (9), 6796–6806. doi:10.1063/1.462569
Kim, J., Ichimura, A. S., Huang, R. H., Redko, M., Phillips, R. C., Jackson, J. E., et al. (1999). Crystalline Salts of Na- and K- (Alkalides) that Are Stable at Room Temperature. J. Am. Chem. Soc. 121 (45), 10666–10667. doi:10.1021/JA992667V
Knizia, G. (2013). Intrinsic Atomic Orbitals: An Unbiased Bridge between Quantum Theory and Chemical Concepts. J. Chem. Theor. Comput. 9 (11), 4834–4843. doi:10.1021/CT400687B
Knizia, G., and Klein, J. E. M. N. (2015). Electron Flow in Reaction Mechanisms-Revealed from First Principles. Angew. Chem. Int. Ed. 54 (18), 5518–5522. doi:10.1002/ANIE.201410637
Lauderdale, W. J., Stanton, J. F., Gauss, J., Watts, J. D., and Bartlett, R. J. (1991). Many-body Perturbation Theory with a Restricted Open-Shell Hartree—Fock Reference. Chem. Phys. Lett. 187 (1–2), 21–28. doi:10.1016/0009-2614(91)90478-R
Lauderdale, W. J., Stanton, J. F., Gauss, J., Watts, J. D., and Bartlett, R. J. (1992). Restricted Open‐shell Hartree-Fock‐based Many‐body Perturbation Theory: Theory and Application of Energy and Gradient Calculations. J. Chem. Phys. 97 (9), 6606–6620. doi:10.1063/1.463664
Lehn, J.-M. (1979). Macrocyclic Receptor Molecules: Aspects of Chemical Reactivity. Investigations into Molecular Catalysis and Transport Processes. Pure Appl. Chem. 51 (5), 979–997. doi:10.1351/PAC197951050979
Lehn, J. M. (1980). Cryptate Inclusion Complexes, Effects on Solute-Solute and Solute-Solvent Interactions and on Ionic Reactivity. Pure Appl. Chem. 52 (10), 2303–2319. doi:10.1351/PAC198052102303
Lehn, J. M. (1978). Cryptates: Inclusion Complexes of Macropolycyclic Receptor Molecules. Pure Appl. Chem. 50 (9–10), 871–892. doi:10.1351/PAC197850090871
Lehn, J. M. (1977). Cryptates: Macropolycyclic Inclusion Complexes. Pure Appl. Chem. 49 (6), 857–870. doi:10.1351/PAC197749060857
Lu, T., and Chen, F. (2012). Multiwfn: A Multifunctional Wavefunction Analyzer. J. Comput. Chem. 33 (5), 580–592. doi:10.1002/JCC.22885
Luo, M., and Jungen, M. (1999). The H3O Rydberg Radical. Chem. Phys. 241 (3), 297–303. doi:10.1016/S0301-0104(98)00426-1
Melin, J., Ortiz, J. V., Martín, I., Velasco, A. M., and Lavín, C. (2005). Ground and Excited States of the Rydberg Radical H3O: Electron Propagator and Quantum Defect Analysis. J. Chem. Phys. 122 (23), 234317. doi:10.1063/1.1926286
Miyamoto, R., Sato, H., and Sudoh, S. (2002). EPR Spectral Study of Gadolinium(III) Cryptate. EPR in the 21st Century 2002, 316–321. doi:10.1016/B978-044450973-4/50058-5
Parida, R., Reddy, G. N., Ganguly, A., Roymahapatra, G., Chakraborty, A., and Giri, S. (2018). On the Making of Aromatic Organometallic Superalkali Complexes. Chem. Commun. 54 (31), 3903–3906. doi:10.1039/C8CC01170B
Perdew, J. P., Burke, K., and Ernzerhof, M. (1996). Generalized Gradient Approximation Made Simple. Phys. Rev. Lett. 77 (18), 3865–3868. doi:10.1103/PHYSREVLETT.77.3865
Puchta, R., Ćoćić, D., Michel, M., and van Eldik, R. (2019). Host-guest Complexes of the Beer-Can-Cryptand: Prediction of Ion Selectivity by Quantum Chemical Calculations XI. J. Coord. Chem. 72 (12), 2106–2114. doi:10.1080/00958972.2019.1636975
Reddy, G. N., and Giri, S. (2016). Organic Heterocyclic Molecules Become Superalkalis. Phys. Chem. Chem. Phys. 18 (35), 24356–24360. doi:10.1039/C6CP04430A
Signorell, R., Palm, H., and Merkt, F. (1997). Structure of the Ammonium Radical from a Rotationally Resolved Photoelectron Spectrum. J. Chem. Phys. 106 (16), 6523–6533. doi:10.1063/1.473653
Silvi, B., and Savin, A. (1994). Classification of Chemical Bonds Based on Topological Analysis of Electron Localization Functions. Nature 371 (6499), 683–686. doi:10.1038/371683a0
Staroverov, V. N., Scuseria, G. E., Tao, J., and Perdew, J. P. (2003). Comparative Assessment of a New Nonempirical Density Functional: Molecules and Hydrogen-Bonded Complexes. J. Chem. Phys. 119 (23), 12129–12137. doi:10.1063/1.1626543
Sun, W.-M., Li, Y., Wu, D., and Li, Z.-R. (2013). Designing Aromatic Superatoms. J. Phys. Chem. C 117 (46), 24618–24624. doi:10.1021/JP408810E
Sun, W.-M., Wu, D., Kang, J., Li, C.-Y., Chen, J.-H., Li, Y., et al. (2018). Decorating Zintl Polyanions with Alkali Metal Cations: A Novel Strategy to Design Superatom Cations with Low Electron Affinity. J. Alloys Compd. 740, 400–405. doi:10.1016/J.JALLCOM.2017.12.075
Tkachenko, N. V., Sun, Z. M., and Boldyrev, A. I. (2019). Record Low Ionization Potentials of Alkali Metal Complexes with Crown Ethers and Cryptands. ChemPhysChem 20 (16), 2060–2062. doi:10.1002/CPHC.201900422
Tkachenko, N. V., Zhang, X. W., Qiao, L., Shu, C. C., Steglenko, D., Muñoz‐Castro, A., et al. (2020). Spherical Aromaticity of All‐Metal [Bi@In8Bi12]3−/5− Clusters. Chem. Eur. J. 26 (9), 2073–2079. doi:10.1002/CHEM.201905264
Tong, J., Li, Y., Wu, D., Li, Z.-R., and Huang, X.-R. (2011). Ab Initio Investigation on a New Class of Binuclear Superalkali Cations M2Li2k+1+ (F2Li3+, O2Li5+, N2Li7+, and C2Li9+). J. Phys. Chem. A. 115 (10), 2041–2046. doi:10.1021/JP110417Z
Tong, J., Li, Y., Wu, D., Li, Z.-R., and Huang, X.-R. (2009). Low Ionization Potentials of Binuclear Superalkali B2Li11. J. Chem. Phys. 131 (16), 164307. doi:10.1063/1.3254835
Tong, J., Li, Y., Wu, D., and Wu, Z.-J. (2012). Theoretical Study on Polynuclear Superalkali Cations with Various Functional Groups as the Central Core. Inorg. Chem. 51 (11), 6081–6088. doi:10.1021/IC202675J
Tong, J., Wu, Z., Li, Y., and Wu, D. (2012). Prediction and Characterization of Novel Polynuclear Superalkali Cations. Dalton Trans. 42 (2), 577–584. doi:10.1039/C2DT31429K
Wang, Z.-C., Tkachenko, N. v., Qiao, L., Matito, E., Muñoz-Castro, A., Boldyrev, A. I., et al. (2020). All-metal σ-antiaromaticity in Dimeric Cluster Anion {[CuGe9Mes]2}4−. Chem. Commun. 56 (48), 6583–6586. doi:10.1039/D0CC02525A
Keywords: cryptands, cryptatium, superalkalis, Rydberg molecules, ionization potential (IP)
Citation: Tkachenko NV, Rublev P, Boldyrev AI and Lehn J-M (2022) Superalkali Coated Rydberg Molecules. Front. Chem. 10:880804. doi: 10.3389/fchem.2022.880804
Received: 21 February 2022; Accepted: 14 March 2022;
Published: 13 April 2022.
Edited by:
Suzana Velickovic, University of Belgrade, SerbiaReviewed by:
Cina Foroutan-Nejad, Institute of Organic Chemistry (PAN), PolandJose Luis Cabellos, Polytechnic University of Tapachula, Mexico
Copyright © 2022 Tkachenko, Rublev, Boldyrev and Lehn. This is an open-access article distributed under the terms of the Creative Commons Attribution License (CC BY). The use, distribution or reproduction in other forums is permitted, provided the original author(s) and the copyright owner(s) are credited and that the original publication in this journal is cited, in accordance with accepted academic practice. No use, distribution or reproduction is permitted which does not comply with these terms.
*Correspondence: Alexander I. Boldyrev, YS5pLmJvbGR5cmV2QHVzdS5lZHU=