- 1Department of Chemistry, BITS-Pilani K. K. Birla Goa Campus, Zuarinagar, India
- 2Department of Chemistry, Dnyanprassarak Mandal’s College and Research Centre, Assagao-Bardez, India
In the present investigation, an attempt has been made to evaluate internal pressure
Introduction
Volumetric, acoustic, and thermophysical properties of nonaqueous binary mixtures provide valuable information about molecular interactions in systems resulting from solute–solute, solvent–solvent, solute–solvent interactions, structural effects, molecular orientation, energy changes, and free volume (Hemmat et al., 2017; Shakila et al., 2020; Jóźwiak et al., 2021). Knowledge of these properties has considerable significance in theoretical and applied areas of research (Rehman et al., 2020; Ezazi et al., 2021; Li et al., 2021; Nain, 2021; Sharma et al., 2021; Wan et al., 2021). Over the past several decades, internal pressure has played a key role in the study of the thermodynamics of liquid mixtures as it provides insights into the internal structure, clustering, structure making, and breaking along with various intermolecular interactions, namely, ionic, dipole–dipole interaction, and dipole-induced dipole attraction. (Marcus, 2013).
Two more significant thermophysical parameters, namely, the energy of vaporization
Alkanone and amine mixtures help us to gain insights into interactions in the amide solution due to the presence of carbonyl and amine groups in the component molecules. It is a well-known fact that proteins and amino acids are linked to each other by peptide bonds. For a better knowledge of biologically complex molecules, the first step involves understanding the intermolecular interactions in the liquid mixtures involving the amide functional group (Alonso et al., 2010a). Aniline is used in the manufacture of polyurethanes and in the pharmaceutical industry to produce drugs such as paracetamol. Aniline is also used in the synthesis of dyes, rubber, etc. N-Methylaniline (NMA) is used as an intermediate for dyes, agrochemicals, and other organic products. It is also used as a coupling solvent. Pyridine, an important raw material of chemical industries, is used as a precursor for the synthesis of various organic products in pharmaceutical and agrochemical industries (Alonso et al., 2011a; Kijevčanin et al., 2013). The increase in atmospheric CO2 due to the increase in fossil fuel combustion is one of the factors affecting global climate change. Newly developed modes of CO2 capture can help reduce atmospheric CO2 concentration significantly over traditional modes. Solvents and solid sorbents such as supported amine, ammonium material, and metal–organic frameworks (MOFs) are widely used for the process (Sanz -Pérez et al., 2016). The combination of aqueous alkanolamine solution with ionic liquid has reported anticorrosion protection property and acts as a better CO2 capture solvent (Bernard et al., 2016; Varghese and Karanikolos, 2020). Also, alkanolamine and room temperature ionic liquid emulsions are practicable to capture CO2 through crystallization of CO2-captured products (Hasib-ur-Rahman et al., 2012). The carbonated aqueous mixtures of alkanolamines and ionic liquids are also studied to understand the carbon steel corrosion behavior (Ali et al., 2012). A comprehensive review of the literature reveals that there is scarcity, pertaining to the thermophysical properties of these industrially significant binary mixtures, which has prompted the present work.
In the present investigation, internal pressure
The investigated systems comprise binary mixtures of the following:
1. 2-Propanone + (aniline/N-methylaniline/pyridine).
2. 2-Butanone + (aniline/N-methylaniline/pyridine).
3. 2-Heptanone + (aniline/N-methylaniline/pyridine).
Theory
The change in intermolecular interactions upon mixing of the liquids is directly affected by the change in the volume and internal energy of the liquid mixture. Internal pressure can be interpreted as the volume derivative of internal energy
Internal pressure
where
The internal pressure consists of attractive and repulsive forces between molecules. It is observed that when internal pressure is plotted against volume for a typical liquid at high volume (low P, high T), the attractive forces dominate
The energy of vaporization
where
Enthalpy of vaporization
where all the symbols have their usual meaning.
Excess entropy is calculated from free volume
where
Since P is very small as compared to Pi, it is neglected in Eq 5.
Free volume
The cohesive energy density (CED) represents the total cohesion per volume of the liquid, and it occurs due to the intermolecular forces present within the liquid (Dack, 1975). The cohesive energy density (CED) (Dack, 1975; Almasi, 2020a; Marcus, 2020) has been evaluated using the energy of vaporization
The solubility parameter (δ) represents the strength of intermolecular interactions between solvent molecules (Weerachanchai et al., 2012; Marcus, 2020). It is given by Pandey et al. (2020):
The coefficient of thermal expansion
where
Results and Discussion
The binary systems comprising alkanones and aromatic amines have been studied at three different temperatures (293.15, 298.15, and 303.15 K). Internal pressure, energy, and enthalpy of vaporization together with excess entropy have been evaluated employing thermodynamic properties to understand the intermolecular interactions present in the systems under investigation. All the requisite thermophysical properties of the pure components have been taken from the literature (Alonso et al., 2010a; Alonso et al., 2010b; Alonso et al., 2011a).
The coefficient of thermal expansion
The
Isothermal compressibility
In the present investigation, isothermal compressibility
Excess isothermal compressibility has been evaluated using the following expression (Alonso et al., 2010a):
where
The required experimental data of isentropic compressibility
The internal pressure
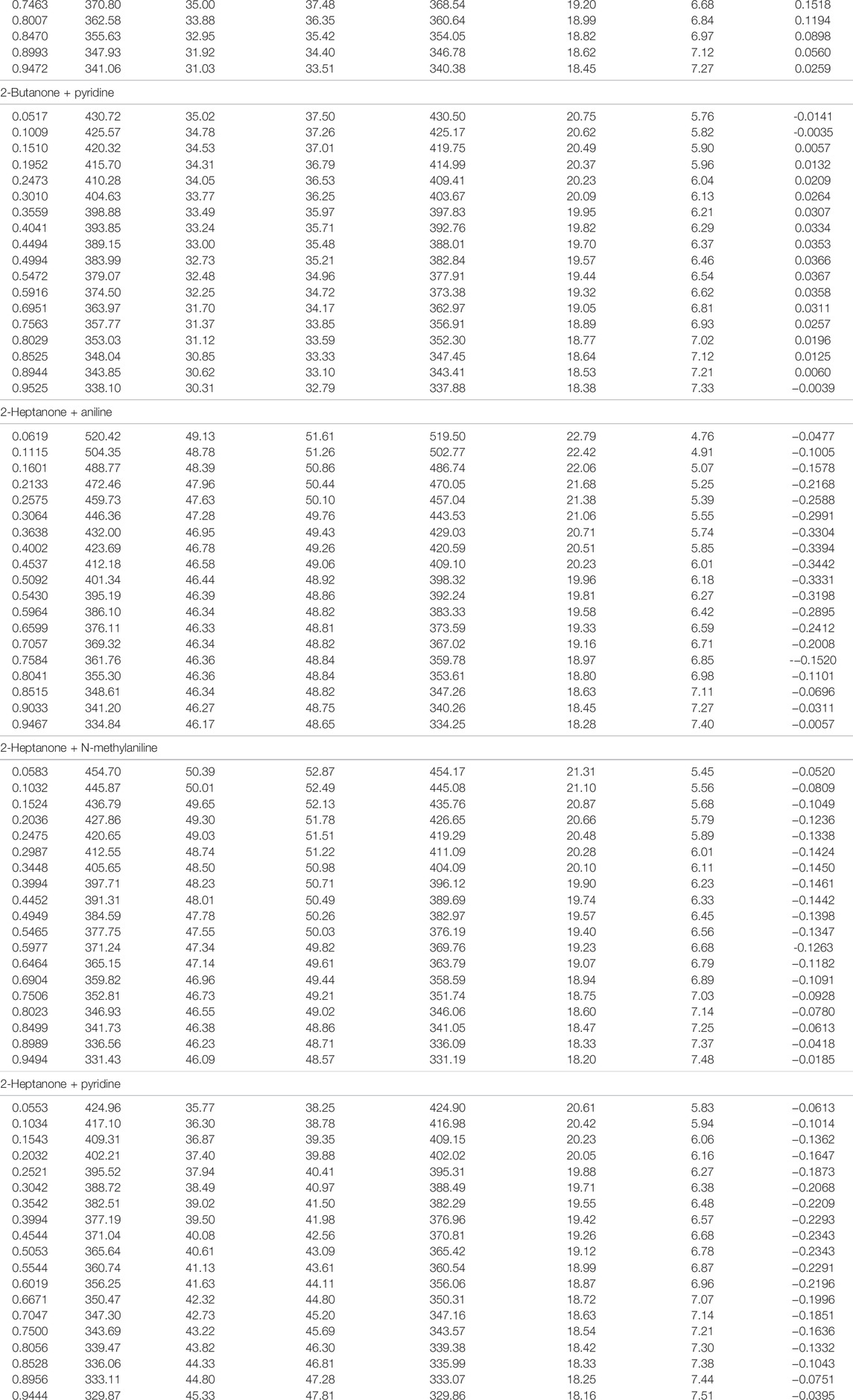
TABLE 1. Thermodynamic properties of alkanones + aromatic amines at 298.15 K (Alonso et al., 2010a; Alonso et al., 2010b; Alonso et al., 2011a).
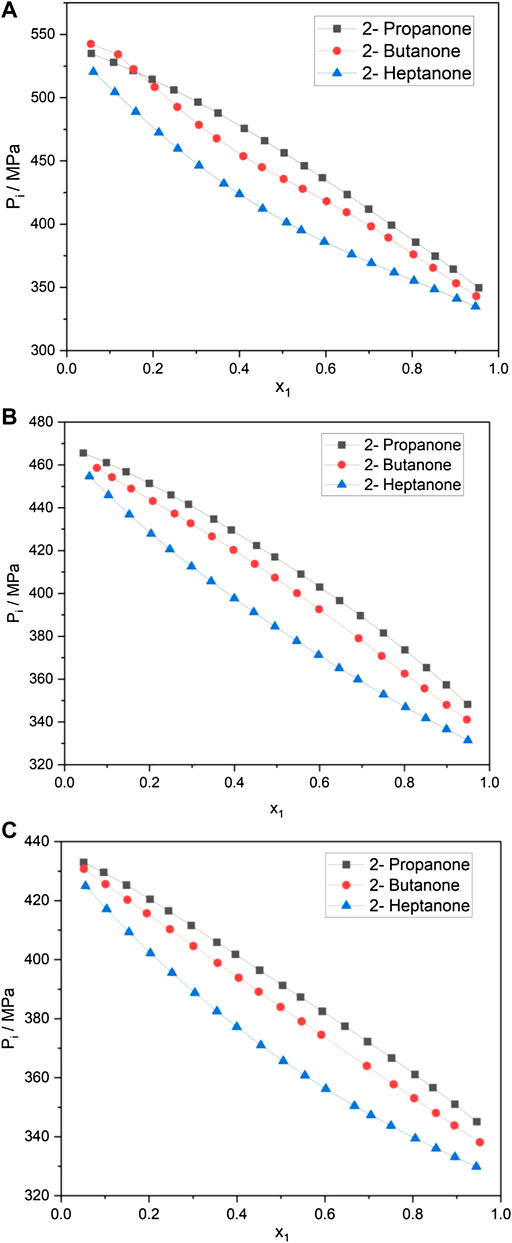
FIGURE 1. (A) Internal pressure of alkanone + aniline at 298.15 K. (B) Internal pressure of alkanone + N-methylaninline at 298.15 K. (C) Internal pressure of alkanone + pyridine at 298.15.
Figures 1B, C show a similar trend in the
The internal pressure of a liquid accounts for the change in internal energy with volume under isothermal conditions and arises due to the presence of various forces such as repulsion, dispersion, and ionic and dipole interaction, which contribute to the overall cohesion in the liquid system. The decrease in
A plot of excess isothermal compressibility
The values of energy of vaporization
The cohesive energy density (CED) and solubility parameter (δ) are evaluated using Eqs. 8, 9 (Almasi, 2020a; Almasi, 2020b) at 298.15 K and are recorded in Table 1. The cohesive energy density represents the total cohesion per volume of the liquid. Cohesion in a liquid is a resultant of the intermolecular forces, especially attractive forces evolving from hydrogen bonding and dipole–dipole and dispersion interactions. The cohesion creates around 1,000–10,000 atm pressure within the liquid. A solute molecule experiences this pressure when dissolved in the solvent, which increases as the interaction between solute–solvent molecules increases. This implies that the solution exists under higher internal pressure than the pure solvent. Even though
Free volume
The thermal expansivity
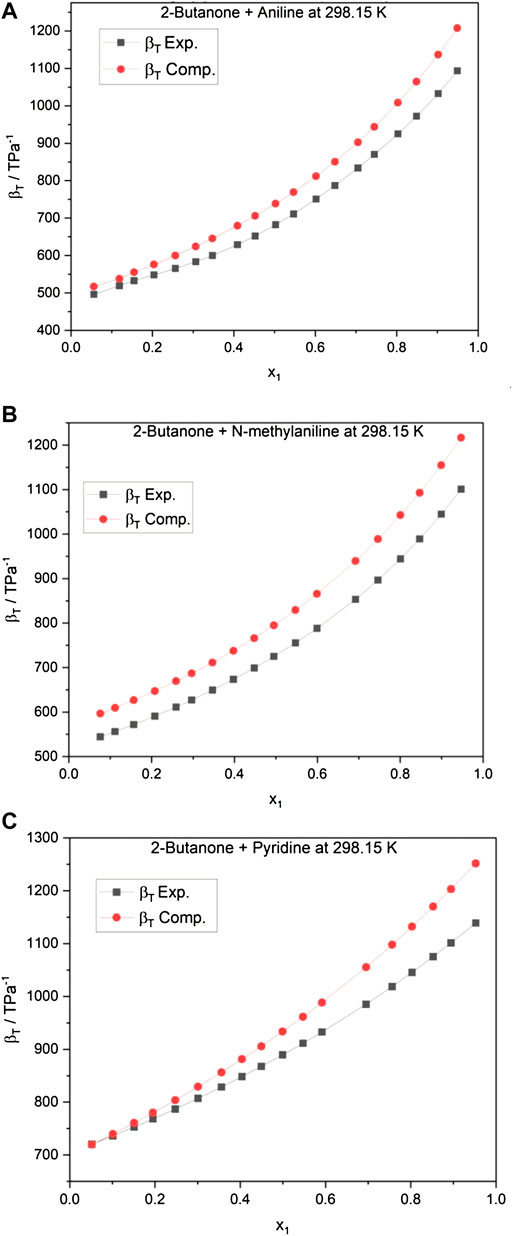
FIGURE 3. (A) Isothermal compressibility of 2-butanone + aniline at 298.15 K. (B) Isothermal compressibility of 2-butanone + N-methylaniline. (C) Isothermal compressibility of 2-butanone + pyridine at 298.15 K.
Excess entropy
The excess entropy
The evaluated values of
An overview of the plots in Figures 1A–C; Table 1; and Supplementary Tables S2, S3 reveals that while keeping alkanone constant, the extent of interactions follows the order: aniline > N-methylaniline > pyridine. These results are in excellent agreement with the
The strong ability of aniline to form a hydrogen bond arises due to the presence of strong dipolar interactions among the aniline molecules (González et al., 2005) The presence of strong interactions between polar molecules is also observed by miscibility gaps between the upper critical solution temperatures (UCSTs) in liquid–liquid equilibria curves (Alonso et al., 2010b). For systems such as aniline + hexane and aniline + heptane, UCSTs are 342.7 and 343.11 K, respectively, while for pyridine systems, UCSTs with hexane and heptane are 252.1 and 255.2 K, respectively, which indicates the ability of aniline to have strong interactions with corresponding molecules (Alonso et al., 2010b). Aniline and NMA are self-associated liquids via hydrogen bonds in the pure state, and this self-association decreases when it is mixed with other alkanones (Alonso et al., 2010b). The structural effects are understood by the contribution to HE for a system that includes positive values due to the breaking of propanone–propanone and aniline–aniline interactions while negative values are due to propanone–aniline interactions (Alonso et al., 2010b). The higher negative
The variation in molecular interactions when alkanone is varied and aromatic amines being kept constant follows the order: 2-propanone > 2-butanone > 2-heptanone. This may be attributed to the fact that as the chain length increases, the ability of amines to form a hydrogen bond with the oxygen atom of alkanone decreases. The C=O group in 2-propanone is more polar than that in 2-butanone or 2-heptanone, which makes it easier for 2-propanone to form a hydrogen bond with aniline. This fact is also supported by the dipole moment of the alkanones wherein 2-propanone has the highest dipole moment (2.88D) and 2-heptanone has the least (2.59 D) (Haynes, 2013). It is pertinent to point out that the steric restriction to the approaching alkanone molecule would be very high when the H atoms of the amino group in aniline are substituted by the methyl group (Nakanishi and Touhara, 1986).
It is evidenced from Figures 1A–C that the variation in the internal pressure values for an amine with the common alkanone exhibits a decreasing trend with the increase in temperature. The energy and enthalpy of vaporization values listed in Table 1 and Supplementary Tables S3, S4 show an increase with the change in temperature for an amine with a set of alkanones. It is observed that CED and solubility parameter values tend to decrease with an increase in temperature. Figure 4 and Supplementary Figures S1, S2 show that excess entropy values are increasing with the rise in temperature, indicating an increase in a disorder with the temperature in the liquid system.
Conclusion
In the present investigation, internal pressure
Data Availability Statement
The original contributions presented in the study are included in the article/Supplementary Material, further inquiries can be directed to the corresponding author.
Author Contributions
The work was conceptualized under the supervision of RD, Associate Professor, Department of Chemistry, BITS Pilani K. K. Birla Goa Campus. AP and AN are research scholars working under the supervision of RD and have carried out the evaluation work. Both have contributed equally to the work. All the results were validated by the corresponding author before submission. Editing of the manuscript was carried out by all the authors.
Conflict of Interest
The authors declare that the research was conducted in the absence of any commercial or financial relationships that could be construed as a potential conflict of interest.
Publisher’s Note
All claims expressed in this article are solely those of the authors and do not necessarily represent those of their affiliated organizations, or those of the publisher, the editors, and the reviewers. Any product that may be evaluated in this article, or claim that may be made by its manufacturer, is not guaranteed or endorsed by the publisher.
Acknowledgments
The corresponding author, RD, acknowledges SRCD, BITS-Pilani K. K. Birla Goa Campus, for the Interim Relief Grant.
Supplementary Material
The Supplementary Material for this article can be found online at: https://www.frontiersin.org/articles/10.3389/fchem.2022.868836/full#supplementary-material
References
Ali, B. S., Ali, B. H., Yusoff, R., and Aroua, M. K. (2012). Carbon Steel Corrosion Behaviors in Carbonated Aqueous Mixtures of Monoethanolamine and 1-N-Butyl-3-Methylimidazolium Tetrafluoroborate. Int. J. Electrochem. Sci. 7, 3835–3853. Available at: www.electrochemsci.org.
Almasi, M. (2020). Cohesive Energy Density and Internal Pressure of Benzene and 1-alkanol Binary Mixtures. J. Mol. Liquids 313, 113459. doi:10.1016/j.molliq.2020.113459
Almasi, M. (2020). Studies on the Structure of [Bmim][NO3] and 1-alkanol: Cohesive Energy Density and Internal Pressure. J. Mol. Struct. 1219, 128576. doi:10.1016/j.molstruc.2020.128576
Alonso, I., Alonso, V., Mozo, I., de La Fuente, I. G., González, J. A., and Cobos, J. C. (2010). Thermodynamics of Ketone + Amine Mixtures. I. Volumetric and Speed of Sound Data at (293.15, 298.15, and 303.15) K for 2-Propanone + Aniline, + N-Methylaniline, or + Pyridine Systems. J. Chem. Eng. Data 55, 2505–2511. doi:10.1021/je900874z
Alonso, I., Mozo, I., de La Fuente, I. G., González, J. A., and Cobos, J. C. (2010). Thermodynamics of Ketone + Amine Mixtures. Part III. Volumetric and Speed of Sound Data at (293.15, 298.15, and 303.15) K for 2-Butanone + Aniline, + N-Methylaniline, or + Pyridine Systems. J. Chem. Eng. Data 55, 5400–5405. doi:10.1021/je100472t
Alonso, I., Mozo, I., de La Fuente, I. G., González, J. A., and Cobos, J. C. (2011). Thermodynamics of Ketone+amine Mixtures 7. Volumetric and Speed of Sound Data at (293.15, 298.15 and 303.15) K for 2-pentanone+aniline, +N-Methylaniline, or +pyridine Systems. J. Mol. Liquids 160, 180–186. doi:10.1016/J.MOLLIQ.2011.03.015
Alonso, I., Mozo, I., De La Fuente, I. G., González, J. A., and Cobos, J. C. (2011). Thermodynamics of Ketone + Amine Mixtures Part V. Volumetric and Speed of Sound Data at (293.15, 298.15 and 303.15) K for Mixtures of 2-heptanone with Aniline, N-Methylaniline or Pyridine. J. Solution Chem. 40, 2057–2071. doi:10.1007/s10953-011-9774-3
Alonso, I., Mozo, I., García de la Fuente, I., González, J. A., and Cobos, J. C. (2011). Thermodynamics of Ketone + Amine Mixtures. Part VIII. Molar Excess Enthalpies at 298.15 K for N-Alkanone + Aniline or + N-Methylaniline Systems. J. Chem. Eng. Data 56, 3236–3241. doi:10.1021/je200333p
Baluja, S., Solanki, A., and Kachhadia, N. (2010). Studies on Thermodynamic Properties of Some Imidazolinone Derivatives in DMF at 308.15 K. Chin. J. Chem. Eng. 18, 306–311. doi:10.1016/S1004-9541(08)60357-2
Bernard, F. L., Dalla Vecchia, F., Rojas, M. F., Ligabue, R., Vieira, M. O., Costa, E. M., et al. (2016). Anticorrosion Protection by Amine-Ionic Liquid Mixtures: Experiments and Simulations. J. Chem. Eng. Data, 61, 1803–1810. doi:10.1021/ACS.JCED.5B00996/SUPPL_FILE/JE5B00996_SI_001
Dack, M. R. J. (1975). The Importance of Solvent Internal Pressure and Cohesion to Solution Phenomena. Chem. Soc. Rev. 4, 211–229. doi:10.1039/CS9750400211
Ezazi, M., Ghaffari, F., Karimi, S., and Shekaari, H. (2021). Thermophysical and Taste Behavior of Sucrose in Aqueous Solution of Some Deep Eutectic Solvents at T= (288.15 to 318.15) K. J. Mol. Liquids 338, 116599. doi:10.1016/J.MOLLIQ.2021.116599
González, J. A., Mozo, I., García de la Fuente, I., and Cobos, J. C. (2005). Thermodynamics of Organic Mixtures Containing Amines. IV. Systems with Aniline. Can. J. Chem. 83, 1812–1825. doi:10.1139/v05-190
Hasib-ur-Rahman, M., Siaj, M., and Larachi, F. (2012). CO2 Capture in Alkanolamine/room-Temperature Ionic Liquid Emulsions: A Viable Approach with Carbamate Crystallization and Curbed Corrosion Behavior. Int. J. Greenhouse Gas Control. 6, 246–252. doi:10.1016/J.IJGGC.2011.10.014
Hemmat, M., Moosavi, M., and Rostami, A. A. (2017). Study on Volumetric and Viscometric Properties of 1,4-dioxane and 1,2-Ethanediol/1,3-Propanediol Binary Liquid Mixtures, Measurement and Prediction. J. Mol. Liquids 225, 107–117. doi:10.1016/J.MOLLIQ.2016.11.052
Hildebrand, J. H., and Scott, R. L. (1950). Solubility of Non-electrolytes. 3rd ed. New York: Reinhold.
Hildebrand, J. H. (1947). The Entropy of Solution of Molecules of Different Size. J. Chem. Phys. 15, 225–228. doi:10.1063/1.1746484
Jóźwiak, M., Urban, A., and Tyczyńska, M. (2021). Effect of Properties of N,N-dimethylformamide + Propan-1-Ol Mixtures on the Solution Enthalpy of Selected Cyclic Ethers in These Mixtures at 298.15 K. The Contribution of Solvent to the Enthalpy of Solvation of Cyclic Ethers. J. Mol. Liquids 321, 114754. doi:10.1016/J.MOLLIQ.2020.114754
Kijevčanin, M. L., Živković, E. M., Djordjević, B. D., Radović, I. R., Jovanović, J., and Šerbanović, S. P. (2013). Experimental Determination and Modeling of Excess Molar Volumes, Viscosities and Refractive Indices of the Binary Systems (Pyridine+1-propanol, +1,2-propanediol, +1,3-propanediol, and +glycerol). New UNIFAC-VISCO Parameters Determination. The J. Chem. Thermodynamics 56, 49–56. doi:10.1016/J.JCT.2012.06.031
Kim, S., Thiessen, P. A., Bolton, E. E., Chen, J., Fu, G., Gindulyte, A., et al. (2016). PubChem Substance and Compound Databases. Nucleic Acids Res. 44, D1202–D1213. doi:10.1093/NAR/GKV951
Levine, I. N. (2011). “Physical Chemistry,” in Physical Chemistry. Sixth Edition (New York: McGraw-Hill).
Li, W., Yuan, J., Wang, X., Shi, W., Zhao, H., Xing, R., et al. (2021). Solubility and Thermodynamic Aspects of Etonogestrel in Several Aqueous Co-solvent Solutions. J. Mol. Liquids 338, 116624. doi:10.1016/J.MOLLIQ.2021.116624
Marcus, Y. (2013). Internal Pressure of Liquids and Solutions. Chem. Rev. 113, 6536–6551. doi:10.1021/cr3004423
Marcus, Y. (2020). The Structure of Mixtures of Water and Acetone Derived from Their Cohesive Energy Densities and Internal Pressures. J. Mol. Liquids 320, 112801. doi:10.1016/j.molliq.2020.112801
Nain, A. K. (2013). Densities, Ultrasonic Speeds, Viscosities and Excess Properties of Binary Mixtures of Methyl Methacrylate with N,N-dimethylformamide and N,N-dimethylacetamide at Different Temperatures. J. Chem. Thermodynamics 60, 105–116. doi:10.1016/j.jct.2013.01.013
Nain, A. K. (2021). Interactions of Some α-amino Acids with Antibacterial Drug Gentamicin Sulphate in Aqueous Medium Probed by Using Physicochemical Approaches. J. Mol. Liquids 321, 114757. doi:10.1016/J.MOLLIQ.2020.114757
Nain, A. K. (2008). Inversion of the Kirkwood-Buff Theory of Solutions: Application to Tetrahydrofuran + Aromatic Hydrocarbon Binary Liquid Mixtures. J. Solution Chem. 37, 1541–1559. doi:10.1007/s10953-008-9326-7
Nakanishi, K., and Touhara, H. (1986). Excess Molar Enthalpies of (Methanol + Aniline), (Methanol + N-Methylaniline), and (Methanol + N,N-dimethylaniline). J. Chem. Thermodynamics 18, 657–660. doi:10.1016/0021-9614(86)90067-4
Nanda, B. B., Nanda, B., and Mohanty, P. C. (2012). Effect of Concentration on Thermo Acoustic and Nonlinearity Parameters (B/A) of Barium Chloride Solutions in Glycol-Water Mixtures at 303.15K. J. Mol. Liquids 171, 50–53. doi:10.1016/j.molliq.2012.03.011
Pandey, J. D., Shukla, A. K., Singh, N., and Sanguri, V. (2020). Estimation of Thermodynamic Properties of Ionic Liquids. J. Mol. Liquids 315, 113585. doi:10.1016/j.molliq.2020.113585
Rama Rao, P. V. S. S., Krishna, T. S., Bharath, P., Dey, R., and Ramachandran, D. (2021). Understanding of Molecular Interactions between Ethyl Acetate and 1-Butyl-3-Methyl-Imidazolium Bis(trifluoromethylsulfonyl)imide: A Thermophysical Study. J. Chem. Thermodynamics 156, 106383. doi:10.1016/j.jct.2020.106383
Rastogi, R. P., and Misra, R. R. (1995). An Introduction to Chemical Thermodynamics. Sixth Revised. New Delhi, India: Vikas Publishing House PVT LTD.
Rehman, L. M., Dey, R., Lai, Z., Ghosh, A. K., and Roy, A. (2020). Reliable and Novel Approach Based on Thermodynamic Property Estimation of Low to High Salinity Aqueous Sodium Chloride Solutions for Water-Energy Nexus Applications. Ind. Eng. Chem. Res. 59, 16029–16042. doi:10.1021/acs.iecr.0c02575
Saini, A., Prabhune, A., Mishra, A. P., and Dey, R. (2021). Density, Ultrasonic Velocity, Viscosity, Refractive index and Surface Tension of Aqueous Choline Chloride with Electrolyte Solutions. J. Mol. Liquids 323, 114593. doi:10.1016/j.molliq.2020.114593
Sanz-Pérez, E. S., Murdock, C. R., Didas, S. A., and Jones, C. W. (2016). Direct Capture of CO2 from Ambient Air. Chem. Rev. 116, 11840–11876. doi:10.1021/ACS.CHEMREV.6B00173
Shakila, A., Raju, R., Srinivasa Krishna, T., Dey, R., and Pandiyan, V. (2020). Molecular Interaction Studies in Binary Mixtures of Tetrahydrofuran with Arene-Substituted Alcohols: Acoustic and Volumetric Study. Phys. Chem. Liquids 58, 263–279. doi:10.1080/00319104.2018.1564752
Sharma, A., Rani, M., and Maken, S. (2021). Thermodynamics of Haloarenes with N-Hexane at 298.15-318.15 K: Density, Ultrasonic Speed and Viscosity. J. Mol. Liquids 321, 114366. doi:10.1016/J.MOLLIQ.2020.114366
Shukla, R. K., Awasthi, N., Kumar, A., Shukla, A., and Pandey, V. K. (2011). Prediction of Associational Behaviour of Binary Liquid Mixtures from Viscosity Data at 298.15, 303.15, 308.15 and 313.15K. J. Mol. Liquids 158, 131–138. doi:10.1016/j.molliq.2010.11.006
Suryanarayana, C. V. (1986). Measurement of Internal Pressure in Liquid Systems. Indian J. Chem. 25, 538–540.
Varghese, A. M., and Karanikolos, G. N. (2020). CO2 Capture Adsorbents Functionalized by Amine - Bearing Polymers: A Review. Int. J. Greenhouse Gas Control. 96, 103005. doi:10.1016/J.IJGGC.2020.103005
Wan, Y., He, H., Sha, J., Sun, R., Li, L., Jiang, G., et al. (2021). Determination and Modelling of Density, Viscosity and Solubility of (R)-(-)-phenylephrine Hydrochloride in Methanol + Ethyl Acetate at (278.15-323.15) K and 0.1 MPa. J. Mol. Liquids 321, 114311. doi:10.1016/J.MOLLIQ.2020.114311
Weerachanchai, P., Chen, Z., Leong, S. S. J., Chang, M. W., and Lee, J.-M. (2012). Hildebrand Solubility Parameters of Ionic Liquids: Effects of Ionic Liquid Type, Temperature and DMA Fraction in Ionic Liquid. Chem. Eng. J. 213, 356–362. doi:10.1016/J.CEJ.2012.10.012
Keywords: internal pressure, cohesive energy density (CED), excess entropy, isothermal compressibility, binary
Citation: Prabhune A, Natekar A and Dey R (2022) Thermophysical Properties of Alkanone + Aromatic Amine Mixtures at Varying Temperatures. Front. Chem. 10:868836. doi: 10.3389/fchem.2022.868836
Received: 03 February 2022; Accepted: 11 April 2022;
Published: 26 May 2022.
Edited by:
Debabrata Seth, Indian Institute of Technology Patna, IndiaReviewed by:
Kasibhatta Siva Kumar, Sri Venkateswara University, IndiaVitaly V. Chaban, P. E. S., Russia
Copyright © 2022 Prabhune, Natekar and Dey. This is an open-access article distributed under the terms of the Creative Commons Attribution License (CC BY). The use, distribution or reproduction in other forums is permitted, provided the original author(s) and the copyright owner(s) are credited and that the original publication in this journal is cited, in accordance with accepted academic practice. No use, distribution or reproduction is permitted which does not comply with these terms.
*Correspondence: Ranjan Dey, cmFuamFuZGV5QGdvYS5iaXRzLXBpbGFuaS5hYy5pbg==