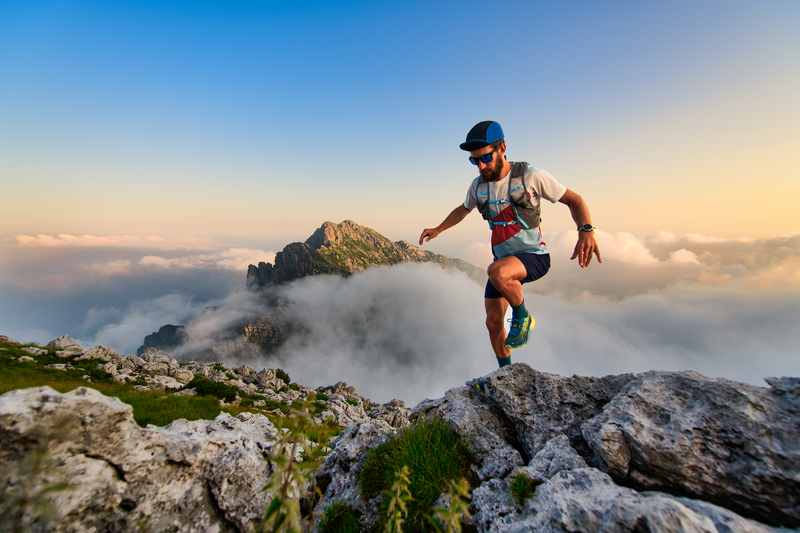
95% of researchers rate our articles as excellent or good
Learn more about the work of our research integrity team to safeguard the quality of each article we publish.
Find out more
MINI REVIEW article
Front. Chem. , 08 April 2022
Sec. Inorganic Chemistry
Volume 10 - 2022 | https://doi.org/10.3389/fchem.2022.866959
This article is part of the Research Topic 2021 Highlights from Marie Skłodowska-Curie Actions Fellows View all 11 articles
Solid-state electrolytes are necessary for high-density and safe lithium-ion batteries. Lithium borohydride (LiBH4) is one of the hydride compounds that shows promising candidates for solid-state electrolytes and enables all-solid-state batteries. LiBH4 has good wetting properties and preferable mechanical properties when used in battery cells. The Li-ion conduction in LiBH4 can be modified with nanoconfinement as a result of distinct properties on the interfaces. The ion conductivities can be modified further by choosing property support materials, i.e., composition, textural properties, and surface chemistry. The present work briefly reviews the Li-ion conduction in nanoconfined LiBH4. A future perspective on the development of LiBH4 as a solid-state electrolyte is further elaborated in the last section.
The availability and widespread use of clean and sustainable energy are a global challenge in the present century. Clean energy can be obtained from converting renewable energy sources such as solar, waves, and wind. However, most renewable energy sources are intermittent, and thus, storage technology is required to maximize widespread usage. Energy storage uses various technologies from mechanical, chemical, and electrochemical storage. Batteries and fuel cells are the two electrochemical storage technologies currently employed for mobile and stationary energy storage and have shown the potential to be developed further. For batteries, specific energy density, in terms of both weight and volume, is an essential parameter besides the costs and abundance of the element. Currently, the battery used in electric cars uses lithium-ion technology, which has a capacity ranging from 100 to 300 Wh/kg based on the intercalation electrode concepts (Schmuch et al., 2018). Increasing the energy density up to 10 times the current technology is expected based on the initial investigation and theoretical calculation using Li as an anode instead of carbon or silicon materials (Adelhelm et al., 2015). The advanced lithium cell battery has an energy density of around 260 Wh/kg using state-of-the-art intercalation electrodes, for example, in the NCA/Si–C cell (Schmuch et al., 2018). Nevertheless, there is enormous room for improvement in increasing the energy density, such as using the lithium anode and sulfur or lithium–air chemistry (Bruce et al., 2012).
Advanced development is enabled by a solid-state electrolyte that has the potential to overcome the well-known battery challenge, i.e., the formation of lithium dendrite. In addition to this safety issue, a high-energy density battery can be realized. Enabling a high-density battery and stable cell operation requires high ionic conductivity, a large potential window, and excellent wetting properties to achieve intimate contact with the electrode. To date, several classes of inorganic materials can be used for ionic conducting materials, such as oxide base LISICON-like, NASICON-like, perovskite, and hydride compound materials, with their advantages and disadvantages when implemented in the battery system.
A considerable effort to increase ionic conduction has been explored; this includes designing an alloying element that provides a tunable lattice volume, energy landscape, and phonon vibration (Krauskopf et al., 2018; Muy et al., 2018). Another approach is materials confinement to benefit from the support materials’ interfacial properties. The journey for finding a new class of electrolytes is ongoing; recently, a boron hydride class has been found to have high ionic conductivity and stability to be used in the Li–S system (Kim et al., 2019). In addition to the properties within the crystal, the interface properties such as grain boundaries play a role in the bulk ionic mobility due to space charge developing at the interface (Yu and Siegel, 2017).
LiBH4 is thermodynamically stable with an enthalpy of formation of 78 kJ/mol H2. The compound desorbs up to 18.5 wt% H and thus is well known as a promising material for hydrogen storage (Schlapbach and Züttel, 2001). However, hydrogen can only be released at above 673 K via various desorption steps hindering further practical application. LiBH4 experiences polymorph transformation from the orthorhombic phase into the hexagonal type structure. At room temperature, the crystalline phase LiBH4 has an orthorhombic structure. It transforms to the hexagonal phase at 383 K. Interestingly, for the hexagonal phase, Li+ in LiBH4 is highly mobile, resulting in high ionic conductivity, up to 10−3 S/cm, which is several orders of magnitude compared to that of the low-temperature phase (Matsuo et al., 2007) and has approximately 6 V stability. Further study using temperature- and frequency-dependent nuclear magnetic resonance (NMR) spectroscopy showed low dimensionality of Li+ in LiBH4 (Epp and Wilkening, 2010). Therefore, this material is promising for the solid-state ionic conductor in Li-ion batteries. These lead to further research on the application of LiBH4 as superionic conductors. Several light hydride materials based on boron have ionic conductivity (Callini et al., 2016; Mohtadi and Orimo, 2017; Guzik et al., 2019; Mohtadi, 2020). Lithium borohydride (LiBH4) consists of Li+ cation and [BH4]- anion complexes.
Various routes/methods have been explored in search of improvement of the properties of LiBH4, and several research groups have been reviewed on this topic (Ley et al., 2014; Bannenberg et al., 2020). Since it has low surface tension, LiBH4 infiltrates porous support such as SBA 15, in which the key was adding hydrogen pressure during the process (Ngene et al., 2010). The wetting properties lead to an excellent capillary infiltration on any porous material such as carbon materials and porous oxides (de Jongh and Eggenhuisen, 2013). This led to nanoconfined materials for hydrogen storage with enhanced properties (de Jongh and Adelhelm, 2010). Another strategy is to confine the metal hydrides in porous matrices, which can be achieved via melt infiltration (Ngene et al., 2010; de Jongh and Eggenhuisen, 2013).
Recently, fast ionic conductivity in silica-confined LiBH4 has been identified (Blanchard et al., 2014). The reason for that is an increased mobility of the [BH4]- units and possibly Li+ in the confined phase (Verkuijlen et al., 2012). Earlier neutron scattering experiments and NMR suggested that the confined materials comprise a highly mobile phase, which was argued to be a consequence of the confinement’s stress. It has also been recommended that the confined LiBH4 is composed of two distinct fractions of LiBH4: fast mobility in the interface and slow mobility at the core (Liu et al., 2013; Verdal et al., 2013; Breuer et al., 2018).
The Li+ conductivity is structurally dependent and reaches up to 1 × 10−3 S/cm, at high temperature and when LiBH4 is in its hexagonal phase. This value is close to that of liquid electrolytes primarily used in Li-ion batteries (Blanchard et al., 2015). The high conductivity has been explained because of the formation of highly mobile liquid-like LiBH4 close to the surface of the pores (Suwarno et al., 2017). Figures 1A,B show the pore size dependence of the transition temperature of the confined LiBH4. The interfacial thickness layer has been estimated to be 1.94 and 1.41 nm for silicon and carbon support materials (Figure 1C). Later studies using NMR confirmed the existence of two phases for the high mobility of lithium, as shown in Figure 1D (Lambregts et al., 2019).
FIGURE 1. Effect of nanoconfinement on LiBH4: (A) differential scanning calorimetry profile showing the other bottom of the profile that originated from confined LiBH4 at a lower temperature than that of the bulk; (B) transformation temperature depression (ΔT) as a function of the pore size for two different support materials; (C) ratio of enthalpy from confined and bulk LiBH4 in the composite for fitting the layer thickness of the mobile phase; (D) schematic of mobile phase dynamics from analyzing the NMR data (Suwarno et al., 2017; Lambregts et al., 2019).
The beneficial effect of LiBH4 as a potential solid-state electrolyte that can be realized is the low melting of LiBH4 and low surface tension in a liquid phase. Lower melting temperature and surface tension can be easier for fabrication and integration in ASSL batteries (Xiao et al., 2021). In addition, the softness increases the interfacial properties of the battery cell compared to the ceramic-based electrolyte. Another essential feature of the solid-state electrolyte used in the cell is the thermodynamic stability. A preliminary experiment showed that the voltage window was at 5–6 V, even though a recent investigation revealed that LiBH4 works well at 2–3 V in the Li–S system. The most important requirement of solid electrolytes is fast ionic conduction at room temperature (10−3 S/cm) to compete with the current liquid electrolyte.
The Li+ mobility in LiBH4 can be modified by using various methods. Figure 2 (left side) summarizes the different methods to increase the Li conductivity, and the initial attempt was by using anion doping. This can be achieved, for example, by doping with LiCl. The most successful was the addition of LiI (Maekawa et al., 2013). This earlier experiment showed that doping the anion dynamics enhanced the Li-ion conduction (Maekawa et al., 2013; Sveinbjörnsson et al., 2013). The second approach is nanoconfinement using support materials such as nanoporous silica or alumina. Since the hexagonal high-temperature phase LiBH4 has high conductivity, it is rational to stabilize the high-temperature phase by confinement. This comes from the Gibbs–Thomson relation of the transformation temperature dependent on the particle size. The third approach is a composite of two Li-based hydrides such as LiNH2.
FIGURE 2. Summary of various strategies for designing enhanced Li+ conductivity in LiBH4 and the Li+ conductivity plot from the previously reported study. The conductivity plot is taken from the study of Zettl et al. (2020).
The initial experiment on the nanoconfined LiBH4 mobility used NMR, which showed that the highly mobile phase is suggested from within a thin layer of high-temperature phase that is stable because of the stress by the confinement in SiO2 porous materials (Verkuijlen et al., 2010; Blanchard et al., 2015). The role of an interface has also been shown in the LiBH4/Al2O composite (Epp and Wilkening, 2013). Thus, the thickness of the interfacial layer could play a significant role in the conductivity. The possibility to tune a well-defined geometry and pore radius of ordered porous silica allowed further research to access the thickness interfacial layer. Furthermore, the surface effect is indicated by comparing silica and carbon to elucidate the interface effect. This was an initial attempt to study the surface effect in addition to the pore size dependency on controlling properties of LiBH4. A later study showed a significant impact on the surface of silica, in which the silanol group played/had a prominent role in Li+ conduction (Ngene et al., 2019). Experiments using various oxides to study the effects of support on the Li-ion conduction show that MgO has a better role in increasing the Li+ conductivity (Gulino et al., 2020, 2021). Figure 2 shows several results from an approach or combination of the Li+ conductivities to highlight a summary of results. As shown in Figure 2 (right), combining two approaches has also been tried, which showed a promising result. Experiments on the nanoconfined LiBH4–LiI in SiO2 support further improvement of ionic conductivity and decreased activation energy for Li+ diffusion (Zettl et al., 2020). The Li+ conductivity enhancement was also observed in LiBH4–LiNH2 confined in mesoporous silica (MCM-41). Surprisingly, in the confined LiBH4–LiNH2 composites, the surface effect is less dominant than that in LiBH4 alone (Kort et al., 2020). The recent study of the composites containing LiBH4–LiI/Al2O3 and LiBH4/Al2O3 highlighted the crucial effect of the insulator–conductor interface in creating the path for fast ion conduction (Zettl et al., 2021). Thus, further exploration of combining various approaches seems promising to enhance the Li+ conductivity in LiBH4.
Lithium borohydride has been widely studied in the last decade and is a promising candidate for solid-state electrolytes used in all-solid-state batteries. The hexagonal phase can be stabilized by nanoconfinement, and the interfacial properties between LiBH4 and the support materials determine the Li+ conductivity. Various approaches combining nanoconfinement with other approaches such as anion substitutions and surface modification yield an increase in ionic conductivities. However, the effect seems different between single-phase LiBH4 and when it is mixed with LiNH2. The effect of the pore volume is dominant rather than the surface effects in the confined LiBH4+LiNH2. Further studies on the impact of LiBH4 doping and textural properties of the support materials on the Li+ conductivity can be the direction of future explorations.
SS and AN conceptualized the research idea. SS acquired the funds. SS, IW, and PS wrote the original draft. SS, AN, IW, and PS reviewed the paper. All authors contributed to the article and approved the submitted version.
This work received support from the EU Horizon 2020 program by the European Commission in the H2020-MSCARISE-2017 action, under the HYDRIDE4MOBILITY project, Grant Agreement No. 778307. This work was partially funded by the Indonesian Ministry of Research and Technology/National Agency for Research and Innovation and the Indonesian Ministry of Education and Culture under the World Class University Program managed by Institut Teknologi Bandung.
The authors declare that the research was conducted in the absence of any commercial or financial relationships that could be construed as a potential conflict of interest.
All claims expressed in this article are solely those of the authors and do not necessarily represent those of their affiliated organizations, or those of the publisher, the editors, and the reviewers. Any product that may be evaluated in this article, or claim that may be made by its manufacturer, is not guaranteed or endorsed by the publisher.
SS acknowledges Petra de Jongh and Peter Ngene at Utrecht University, The Netherlands.
Adelhelm, P., Hartmann, P., Bender, C. L., Busche, M., Eufinger, C., and Janek, J. (2015). From Lithium to Sodium: Cell Chemistry of Room Temperature Sodium-Air and Sodium-Sulfur Batteries. Beilstein J. Nanotechnol. 6, 1016–1055. doi:10.3762/bjnano.6.105
Bannenberg, L. J., Heere, M., Benzidi, H., Montero, J., Dematteis, E. M., Suwarno, S., et al. (2020). Metal (Boro-) Hydrides for High Energy Density Storage and Relevant Emerging Technologies. Int. J. Hydrogen Energ. 45, 33687–33730. doi:10.1016/j.ijhydene.2020.08.119
Blanchard, D., Nale, A., Sveinbjörnsson, D., Eggenhuisen, T. M., Verkuijlen, M. H. W., Suwarno, , et al. (2014). Nanoconfined LiBH4 as a Fast Lithium Ion Conductor. Wiley Online Library. submitted.
Blanchard, D., Nale, A., Sveinbjörnsson, D., Eggenhuisen, T. M., Verkuijlen, M. H. W., Suwarno, T., et al. (2015). Nanoconfined LiBH4as a Fast Lithium Ion Conductor. Adv. Funct. Mater. 25, 184–192. doi:10.1002/adfm.201402538
Breuer, S., Uitz, M., and Wilkening, H. M. R. (2018). Rapid Li Ion Dynamics in the Interfacial Regions of Nanocrystalline Solids. J. Phys. Chem. Lett. 9, 2093–2097. doi:10.1021/acs.jpclett.8b00418
Bruce, P. G., Freunberger, S. A., Hardwick, L. J., and Tarascon, J.-M. (2012). Li-O2 and Li-S Batteries with High Energy Storage. Nat. Mater 11, 19–29. doi:10.1038/nmat3191
Callini, E., Atakli, Z. Ö. K., Hauback, B. C., Orimo, S.-i., Jensen, C., Dornheim, M., et al. (2016). Complex and Liquid Hydrides for Energy Storage. Appl. Phys. A. 122, 353. doi:10.1007/s00339-016-9881-5
de Jongh, P. E., and Adelhelm, P. (2010). Nanosizing and Nanoconfinement: New Strategies Towards Meeting Hydrogen Storage Goals. ChemSusChem 3, 1332–1348.
de jongh, P. E., and Eggenhuisen, T. M. (2013). Melt Infiltration: An Emerging Technique for the Preparation of Novel Functional Nanostructured Materials. Adv. Mater. 25, 6672–6690. doi:10.1007/s00339-016-9881-5
de Kort, L. M., Harmel, J., de Jongh, P. E., and Ngene, P. (2020). The Effect of Nanoscaffold Porosity and Surface Chemistry on the Li-Ion Conductivity of LiBH4-LiNH2/metal Oxide Nanocomposites. J. Mater. Chem. A. 8, 20687–20697. doi:10.1039/D0TA07600G
Epp, V., and Wilkening, M. (2010). Fast Li Diffusion in crystallineLiBH4due to Reduced Dimensionality: Frequency-dependent NMR Spectroscopy. Phys. Rev. B 82, 020301. doi:10.1103/PhysRevB.82.020301
Epp, V., and Wilkening, M. (2013). Motion of Li+in Nanoengineered LiBH4and LiBH4:Al2O3Comparison with the Microcrystalline Form. ChemPhysChem 14, 3706–3713. doi:10.1002/cphc.201300743
Gulino, V., Barberis, L., Ngene, P., Baricco, M., and de Jongh, P. E. (2020). Enhancing Li-Ion Conductivity in LiBH4-Based Solid Electrolytes by Adding Various Nanosized Oxides. ACS Appl. Energ. Mater. 3, 4941–4948. doi:10.1021/acsaem.9b02268
Gulino, V., Brighi, M., Murgia, F., Ngene, P., de Jongh, P., Černý, R., et al. (2021). Room-Temperature Solid-State Lithium-Ion Battery Using a LiBH4-MgO Composite Electrolyte. ACS Appl. Energ. Mater. 4, 1228–1236. doi:10.1021/acsaem.0c02525
Guzik, M. N., Mohtadi, R., and Sartori, S. (2019). Lightweight Complex Metal Hydrides for Li-, Na-, and Mg-Based Batteries. J. Mater. Res. 34, 877–904. doi:10.1557/jmr.2019.82
Kim, S., Oguchi, H., Toyama, N., Sato, T., Takagi, S., Otomo, T., et al. (2019). A Complex Hydride Lithium Superionic Conductor for High-Energy-Density All-Solid-State Lithium Metal Batteries. Nat. Commun. 10, 1081. doi:10.1038/s41467-019-09061-9
Krauskopf, T., Muy, S., Culver, S. P., Ohno, S., Delaire, O., Shao-Horn, Y., et al. (2018). Comparing the Descriptors for Investigating the Influence of Lattice Dynamics on Ionic Transport Using the Superionic Conductor Na3PS4-xSex. J. Am. Chem. Soc. 140, 14464–14473. doi:10.1021/jacs.8b09340
Lambregts, S. F. H., van Eck, E. R. H., SuwarnoNgene, P., Ngene, P., de Jongh, P. E., and Kentgens, A. P. M. (2019). Phase Behavior and Ion Dynamics of Nanoconfined LiBH4 in Silica. J. Phys. Chem. C 123, 25559–25569. doi:10.1021/acs.jpcc.9b06477
Ley, M. B., Jepsen, L. H., Lee, Y.-S., Cho, Y. W., Bellosta von Colbe, J. M., Dornheim, M., et al. (2014). Complex Hydrides for Hydrogen Storage - New Perspectives. Mater. Today 17, 122–128. doi:10.1016/j.mattod.2014.02.013
Liu, X., Majzoub, E. H., Stavila, V., Bhakta, R. K., Allendorf, M. D., Shane, D. T., et al. (2013). Probing the Unusual Anion Mobility of LiBH4 Confined in Highly Ordered Nanoporous Carbon Frameworks via Solid State NMR and Quasielastic Neutron Scattering. J. Mater. Chem. A. 1, 9935–9941. doi:10.1039/c3ta12051a
Maekawa, H., Matsuo, M., Takamura, H., Ando, M., Noda, Y., Karahashi, T., et al. (2009). Halide-Stabilized LiBH4, a Room-Temperature Lithium Fast-Ion Conductor. J. Am. Chem. Soc. 131, 894–895. doi:10.1021/ja807392k
Matsuo, M., Nakamori, Y., Orimo, S.-i., Maekawa, H., and Takamura, H. (2007). Lithium Superionic Conduction in Lithium Borohydride Accompanied by Structural Transition. Appl. Phys. Lett. 91, 224103. doi:10.1063/1.2817934
Mohtadi, R. (2020). Beyond Typical Electrolytes for Energy Dense Batteries. Molecules 25, 1791. doi:10.3390/molecules25081791
Mohtadi, R., and Orimo, S.-i. (2017). The Renaissance of Hydrides as Energy Materials. Nat. Rev. Mater. 2, 16091. doi:10.1038/natrevmats.2016.91
Muy, S., Bachman, J. C., Chang, H.-H., Abernathy, D. L., Bansal, D., Delaire, O., et al. (2018). Tuning Mobility and Stability of Lithium Ion Conductors Based on Lattice Dynamics. Energy Environ. Sci. 11, 850–859. doi:10.1039/C7EE03364H
Ngene, P., Adelhelm, P., Beale, A. M., de Jong, K. P., and de Jongh, P. E. (2010). LiBH4/SBA-15 Nanocomposites Prepared by Melt Infiltration Under Hydrogen Pressure: Synthesis and Hydrogen Sorption Properties. J. Phys. Chem. C 114, 6163–6168. doi:10.1021/jp9065949
Ngene, P., Lambregts, S. F. H., Vegge, T., Sharma, M., Hagemann, H., de Jongh, P. E., et al. (2019). The Influence of Silica Surface Groups on the Li-Ion Conductivity of LiBH4/SiO2 Nanocomposites. Phys. Chem. Chem. Phys. 21, 22456–22466. doi:10.1039/C9CP04235K
Schlapbach, L., and Züttel, A. (2001). Hydrogen-storage Materials for mobile Applications. Nature 414, 353–358. doi:10.1038/35104634
Schmuch, R., Wagner, R., Hörpel, G., Placke, T., and Winter, M. (2018). Performance and Cost of Materials for Lithium-Based Rechargeable Automotive Batteries. Nat. Energ. 3, 267–278. doi:10.1038/s41560-018-0107-2
Suwarno, S., Ngene, P., Nale, A., Eggenhuisen, T. M., Oschatz, M., Embs, J. P., et al. (2017). Confinement Effects for Lithium Borohydride: Comparing Silica and Carbon Scaffolds. J. Phys. Chem. C 121, 4197–4205. doi:10.1021/acs.jpcc.6b13094
Sveinbjörnsson, D., Myrdal, J. S. G., Blanchard, D., Bentzen, J. J., Hirata, T., Mogensen, M. B., et al. (2013). Effect of Heat Treatment on the Lithium Ion Conduction of the LiBH4-LiI Solid Solution. J. Phys. Chem. C 117, 3249–3257. doi:10.1021/jp310050g
Verdal, N., Udovic, T. J., Rush, J. J., Liu, X., Majzoub, E. H., Vajo, J. J., et al. (2013). Dynamical Perturbations of Tetrahydroborate Anions in LiBH4 Due to Nanoconfinement in Controlled-Pore Carbon Scaffolds. J. Phys. Chem. C 117, 17983–17995. doi:10.1021/jp4063737
Verkuijlen, M. H. W., Gao, J., Adelhelm, P., van Bentum, P. J. M., de Jongh, P. E., and Kentgens, A. P. M. (2010). Solid-State NMR Studies of the Local Structure of NaAlH4/C Nanocomposites at Different Stages of Hydrogen Desorption and Rehydrogenation. J. Phys. Chem. C 114, 4683–4692. doi:10.1021/jp911228x
Verkuijlen, M. H. W., Ngene, P., de Kort, D. W., Barré, C., Nale, A., van Eck, E. R. H., et al. (2012). Nanoconfined LiBH4 and Enhanced Mobility of Li+ and BH4- Studied by Solid-State NMR. J. Phys. Chem. C 116, 22169–22178. doi:10.1021/jp306175b
Xiao, Y., Turcheniuk, K., Narla, A., Song, A.-Y., Ren, X., Magasinski, A., et al. (2021). Electrolyte Melt Infiltration for Scalable Manufacturing of Inorganic All-Solid-State Lithium-Ion Batteries. Nat. Mater. 20, 984–990. doi:10.1038/s41563-021-00943-2
Yu, S., and Siegel, D. J. (2017). Grain Boundary Contributions to Li-Ion Transport in the Solid Electrolyte Li7La3Zr2O12 (LLZO). Chem. Mater. 29, 9639–9647. doi:10.1021/acs.chemmater.7b02805
Zettl, R., de Kort, L., Gombotz, M., Wilkening, H. M. R., de Jongh, P. E., and Ngene, P. (2020). Combined Effects of Anion Substitution and Nanoconfinement on the Ionic Conductivity of Li-Based Complex Hydrides. J. Phys. Chem. C 124, 2806–2816. doi:10.1021/acs.jpcc.9b10607
Keywords: hydrides, lithium borohydrides, battery, electrolyte, solid-state, batteries and energy storage
Citation: Suwarno S, Nale A, Suwarta P, Wijayanti ID and Ismail M (2022) Designing Nanoconfined LiBH4 for Solid-State Electrolytes. Front. Chem. 10:866959. doi: 10.3389/fchem.2022.866959
Received: 31 January 2022; Accepted: 07 March 2022;
Published: 08 April 2022.
Edited by:
Hai-Wen Li, Hefei General Machinery Research Institute, ChinaReviewed by:
Martin Wilkening, Graz University of Technology, AustriaCopyright © 2022 Suwarno, Nale, Suwarta, Wijayanti and Ismail. This is an open-access article distributed under the terms of the Creative Commons Attribution License (CC BY). The use, distribution or reproduction in other forums is permitted, provided the original author(s) and the copyright owner(s) are credited and that the original publication in this journal is cited, in accordance with accepted academic practice. No use, distribution or reproduction is permitted which does not comply with these terms.
*Correspondence: Suwarno Suwarno, d2Fybm9AbWUuaXRzLmFjLmlk
Disclaimer: All claims expressed in this article are solely those of the authors and do not necessarily represent those of their affiliated organizations, or those of the publisher, the editors and the reviewers. Any product that may be evaluated in this article or claim that may be made by its manufacturer is not guaranteed or endorsed by the publisher.
Research integrity at Frontiers
Learn more about the work of our research integrity team to safeguard the quality of each article we publish.