- Research and Development Centre, Dubai Electricity and Water Authority (DEWA), Dubai, United Arab Emirates
The electrochemical stability of 22 commercially available hydrophobic ionic liquids was measured at different temperatures (288.15, 298.15, 313.15, 333.15 and 358.15 K), to systematically investigate ionic liquids towards electrolytes for supercapacitors in harsh weather conditions. Bis(trifluoromethanesulfonyl)imide and bis(fluorosulfonyl)imide anions in combination with 1-Butyl-1-methylpyrrolidinium, 1-Ethyl-3-methylimidazolium, N-Ethyl-N, N-dimethyl-N(2methoxyethyl)ammonium, 1-Methyl-1-(2-methoxyethyl)pyrrolidinium, N-Pentyl-N-methylpyrrolidinium, N, N-Diethyl-N-methyl-N-propylammonium, N, N-Dimethyl-N-ethyl-N-benzyl ammonium, N, N-Dimethyl-N-Ethyl-N-phenylethylammonium, N-Butyl-N-methylpiperidinium, 1-Methyl-1-propylpiperidinium, N-Tributyl-N-methylammonium, N-Trimethyl-N-butylammonium, N-Trimethyl-N-butylammonium, N-Trimethyl-N-propylammonium, N-Propyl-N-methylpyrrolidinium cations were selected for the study. Linear regression with a numerical model was used in combination with voltammetry experiments to deduce the temperature sensitivity of both anodic and cathodic potential limits (defining the electrochemical stability window), in addition to extrapolating results to 283.15 and 363.15 K. We evaluated the influence of the cations, anions, and the presence of functional groups on the observed electrochemical stability window which ranged from 4.1 to 6.1 V.
1 Introduction
Ionic liquids (ILs) are being used to replace conventional organic solvents in various applications because of their unique features such as inherent ionic conductivity, high thermal stability, wide liquid state temperature range, and high electrochemical stability (Wasserscheid and Welton, 2008) (Paul et al., 2020) (Lethesh et al., 2021) (Kermanioryani et al., 2016) (Grøssereid et al., 2019). Recently, the application of ionic liquid (IL) based electrolytes in energy storage devices has been an active area of research (Chellappan et al., 2020) (Bahadori et al., 2020) (Doherty, 2018) (Ma et al., 2018). The systematic measurement and analysis of IL electrochemical stability window (
As observed experimentally, recorded faradaic currents describing
The presence of water also reduces the electrochemical stability of ILs due to hydrogen and oxygen evolution reactions from the electrolysis of water (O’Mahony et al., 2008). In addition, the combination of I− and water can form electroactive species, while HF can also be formed by the reaction of [PF6]−/[BF4]− anions with water (Huddleston et al., 2001). The purification of ILs is energy extensive and can be quite complex, making it impractical for large-scale applications. Therefore, it is crucial to evaluate the electrochemical properties of commercial ILs to be used in large scale electrochemical applications like supercapacitor development (Lethesh et al., 2021).
This work focuses on
2 Materials and Methods
2.1 Chemicals
The ILs used in this study are; [Pyr 1,4][FSI], [Pyr 1,4][TFSI], [Pyr 1,3][FSI], [Pyr 1,3][TFSI], [Pyr 1,102][FSI], [Pyr 1,102][TFSI], [Pyr 1,5][TFSI], [Pyr 1,103][TFSI], [Pip 1,3][TFSI], [Pip 1,3][FSI], [Pip 1,4][TFSI], [EMIm][FSI], [EMIm][TFSI], [N 1,1, 2, 102][FSI], [N 1,1, 2, 102][TFSI], [N 1,1,2,Benz][TFSI], [N 1,1,2, PhenylEth][TFSI], [N 2,2,1, 102][FSI], [N 4,4,4,1][TFSI], [N 1,1,1,4][TFSI], [N 1,1,1,6][TFSI], [N 1,1,1,3][FSI]. All ILs were purchased from Solvionic and used without further purification. The full names of the ILs, purity and further details are shown in Supplementary Table S1 of supporting information (SI). As per the material data sheet, the water content in all the ILs used were less than 20 ppm. Ferrocene (99%) was purchased from Alfa Aesar. All chemicals were handled in an Argon-filled glovebox (Mbraun), while the closed, completely secluded, air/water-free cell assemblies were transferred outside the glovebox for electrochemical testing.
2.2 Electrochemical Characterisation
Cyclic voltammetry (CV) was performed using a microcell setup (TSC 70 closed-cell, rhd instruments) with a potentiostat (AutoLab PGSTAT302N, Metrohm) and workstation. The microcell’s airtight compartment is insulated from the atmosphere and utilised in normal room conditions. The microcell setup establishes; a Pt crucible as a counter electrode and four separately connectable Pt wire ends as working electrodes. The exposed working electrode diameter to the IL electrolytes is 0.25 mm. An AgCl coated Ag wire in direct contact with the ILs being measured was used as a quasi-reference for all measurements. For each test, ca. 100 µL of IL was used in the microcell. The microcell setup can be seen in Supplementary Figure S1 of the supporting information (SI).
CV scans were done at a scan rate of 50 mV s⁻1 for all experiments within high enough vertex potentials to accommodate significant anodic and cathodic degradation currents. The microcell setup uses a Peltier element in the cell stand for active heating/cooling of the compartment for accurate temperature control from the potentiostat software. We further verified the temperatures on the cell stand to be consistent with set points with a thermal camera. The CV scans were performed at 288.15, 298.15, 313.15, 333.15 and 358.15 K in this study at standard pressure. The experiments were done twice for each IL-temperature point combination, while the last of 4 CV cycles for each test was selected for analyses–allowing the recording of steady-state voltametric responses.
To determine the values of the electrochemical stability limits
Calibration of the quasi-reference was done to determine its formal potential and temperature coefficient vs. a known ferrocene/ferrocenium (Fc/Fc+) reference, as described by Bard and Faulkner (de Rooij, 2003). In summary, the calibration was done by determining the equilibrium potential of Fc/Fc+ redox couple in one of the ILs used ([EMim][TFSI]) at the tested temperatures (288.15, 298.15, 313.15, 333.15 and 358.15 K), using a small amount of ferrocene (0.1 mM) dissolved in the IL. The CV measurements for the calibration exercise (see Supplementary Figure S3 of the SI) shows the Ag/Ag+ quasi-reference is -0.355 V vs. Fc/Fc+ at standard temperature (298.15 K), with a temperature coefficient of about
2.3 Linear Regression
Given that the CVs were performed at 288.15, 298.15, 313.15, 333.15 and 358.15 K. A linear regression model was used to fit the experimental
Obtained values of
3 Results and Discussion
Before presenting experimental results from the measurements, the structures of the ions making up the ILs used in this study are shown in Figure 1. In the figure, the chemical structure of the several cations paired with either bis(trifluoromethanesulfonyl)imide (TFSI) or bis(fluorosulfonyl)imide (FSI) anions are shown. The different Imidazolium, pyrrolidinium and piperidinium cations in combination with bis(trifluoromethanesulfonyl)imide ([TFSI]-) and bis(fluorosulfonyl)imide ([FSI]-) anions were selected because of their hydrophobicity.
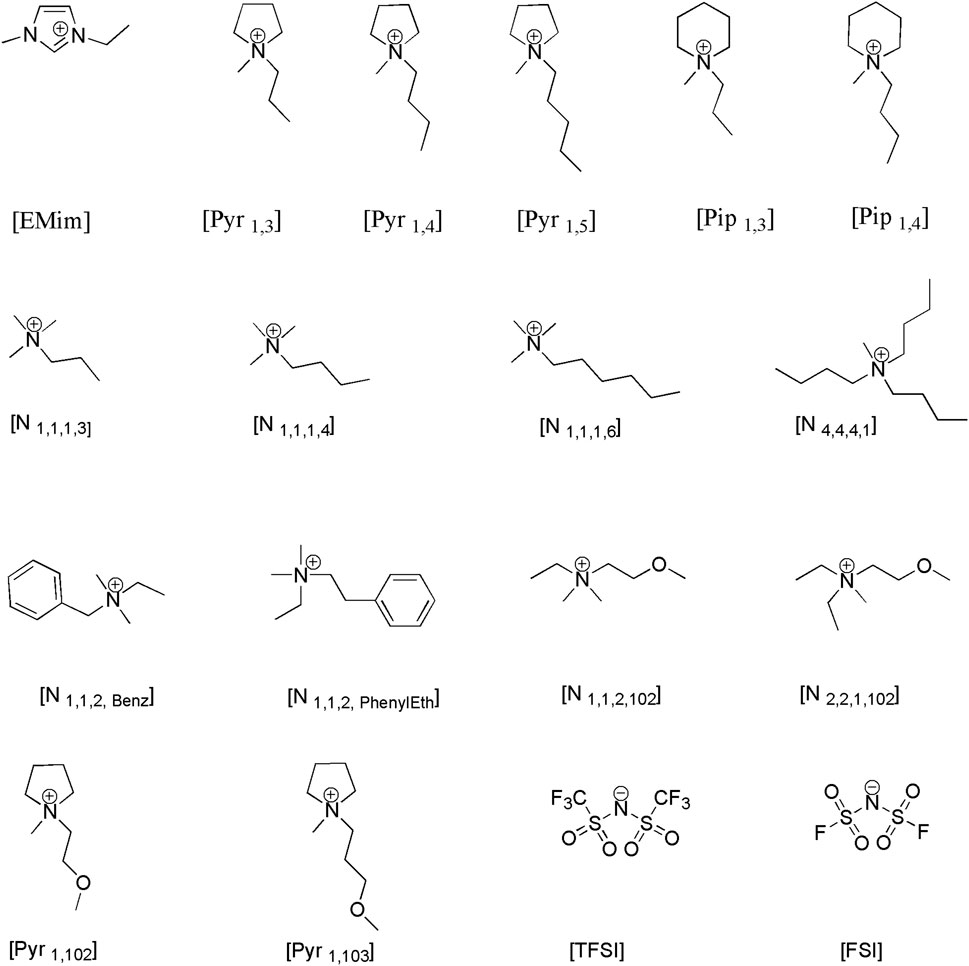
FIGURE 1. Structure of cations: 1-Ethyl-3-methylimidazolium, 1-Propyl-1-methylpyrrolidinium, 1-Butyl-1-methylpyrrolidinium, 1-Pentyl-1-methylpyrrolidinium, 1-Methyl-1-propylpiperidinium, N-Trimethyl-N-propylammonium, N-Trimethyl-N-butylammonium, N-Trimethyl-N-hexylammonium, N-Tributyl-N-methylammonium, N,N-Dimethyl-N-ethyl-N-benzylAmmonium, N,N-Dimethyl-N-Ethyl-N-Phenyl ethylammonium, N-ethyl-N,N-dimethyl-N-(2methoxyethyl)ammonium, N-N-Diethyl-N-methyl-N-(2methoxyethyl)ammonium,1-Methyl-1-(2-methoxyethyl)pyrrolidinium, 1-Methyl-1-(2-methoxypropyl)pyrrolidinium, and anions: bis(trifluoromethanesulfonyl)imide, bis(fluorosulfonyl)imide, under study.
3.1 Temperature-Dependent Electrochemical Stability Window
Table 1 presents the anodic and cathodic potential limits (
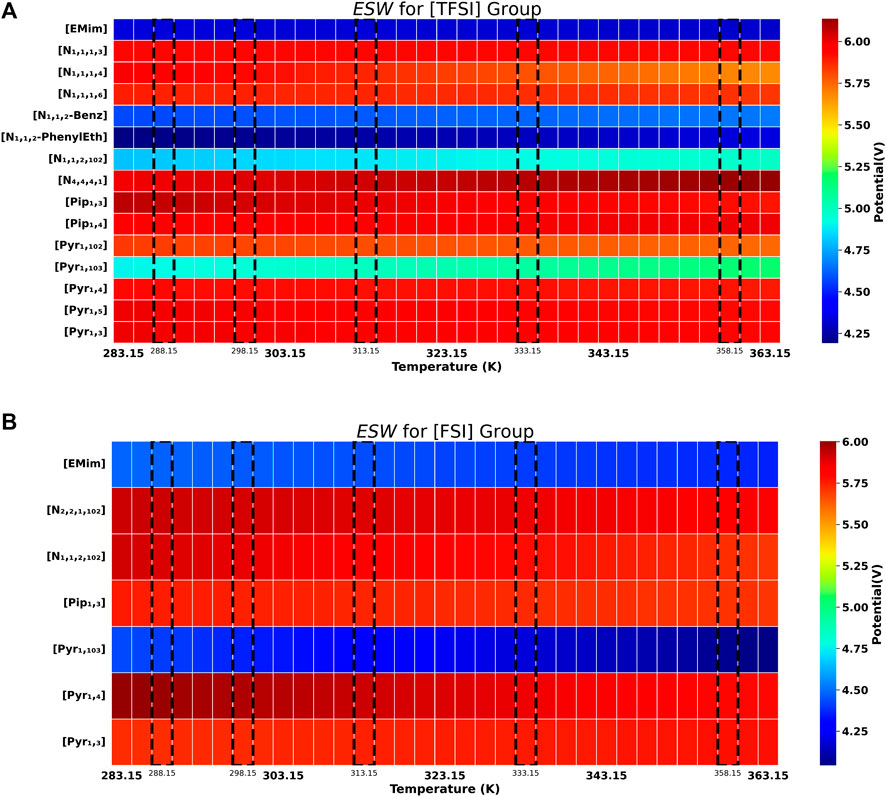
FIGURE 2. Electrochemical stability (
Given the absence of data showing
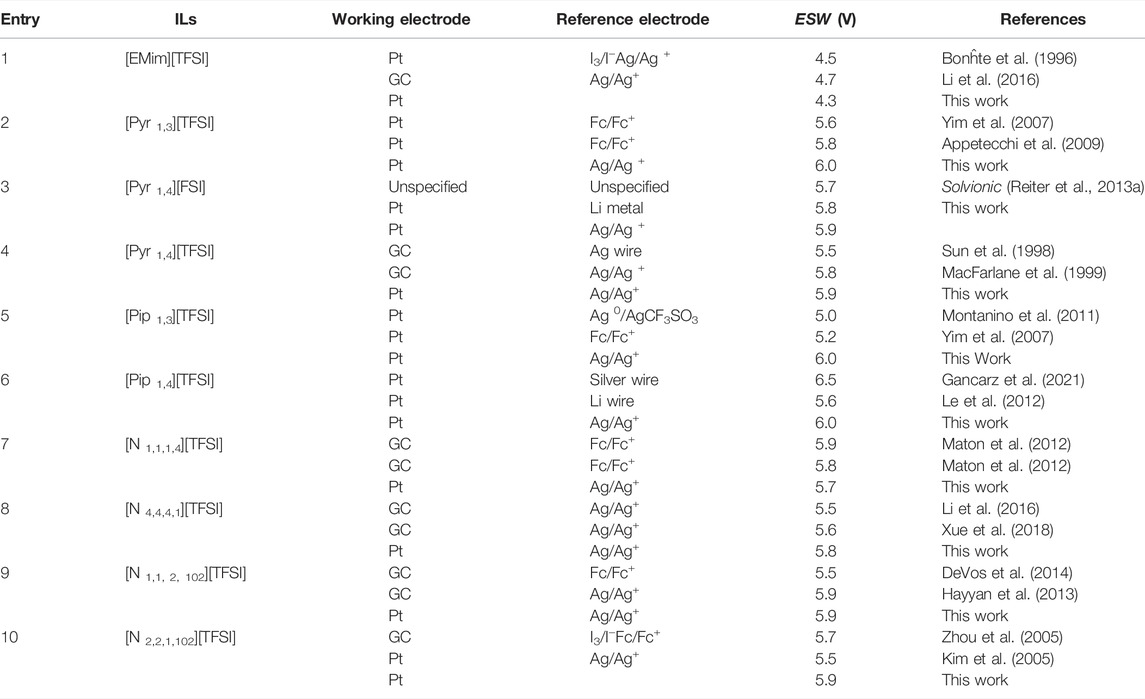
TABLE 2. Comparison of some measured electrochemical stability window (
It can be seen in the Table that the
Interestingly, only one ILs, [Pip 1,3][TFSI] showed
Theoretically, the voltametric response recorded with the CVs at different temperatures is governed by the interplay of thermodynamic, reaction kinetics and mass transport influences. Temperature influence on the thermodynamic equilibrium potential of reactions at both anodic and cathodic limits is well described with the Nernst equation. Increasing temperature is generally expected to favour reaction kinetics, as seen in Butler-Volmer-type kinetic relations (de Rooij, 2003). Furthermore, the sensitivity of IL viscosities (and their ionic conductivities/mobilities) to temperature varies significantly among the different IL groups, thereby affecting ionic transport to and from the electrode surface. All else being equal—if the ionic mobility/diffusivity for some ILs does not significantly increase within the reported temperature range, the resulting change in
As explained previously in Section 2.3, the slope and constant (
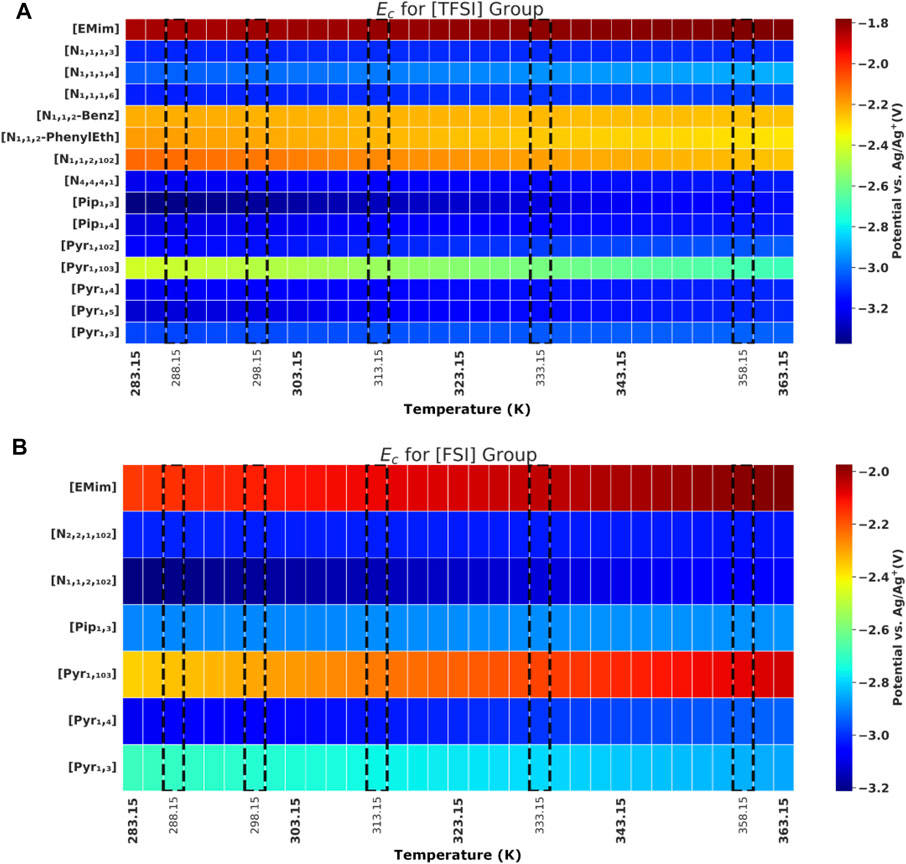
FIGURE 3. Cathodic potential (
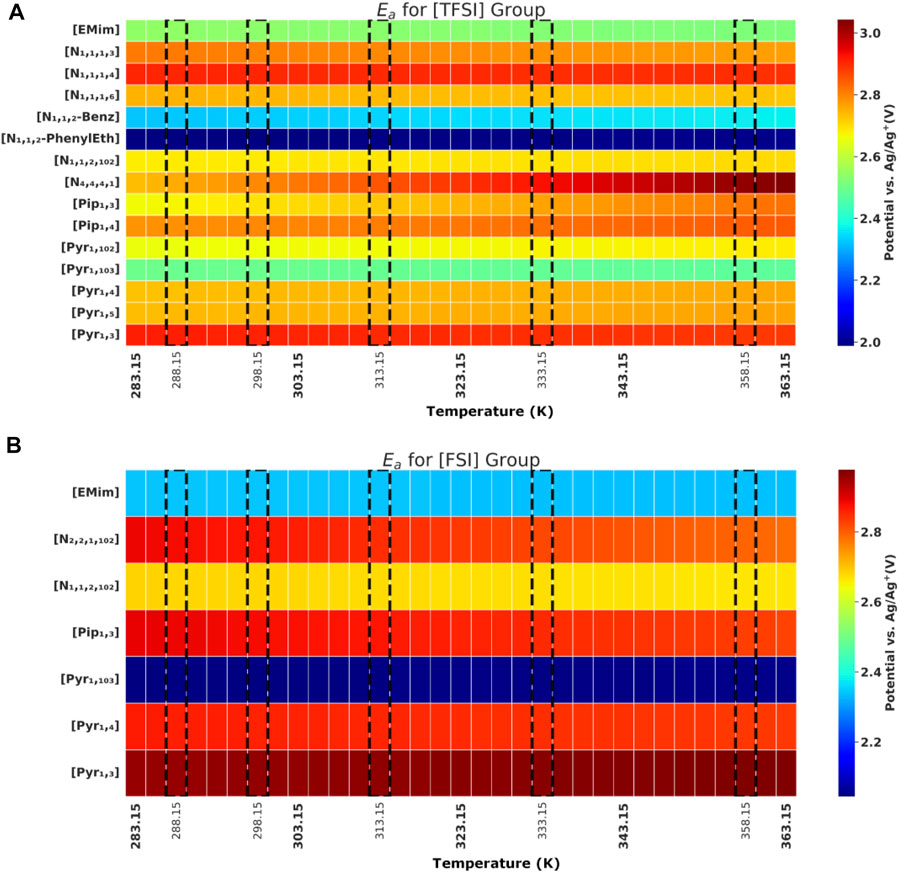
FIGURE 4. Anodic potential (
3.2 Effect of Cation
The structure of the ILs (cations and anions) greatly influences their electrochemical stability. Among different cations studied, piperidinium and pyrrolidinium cations show the highest
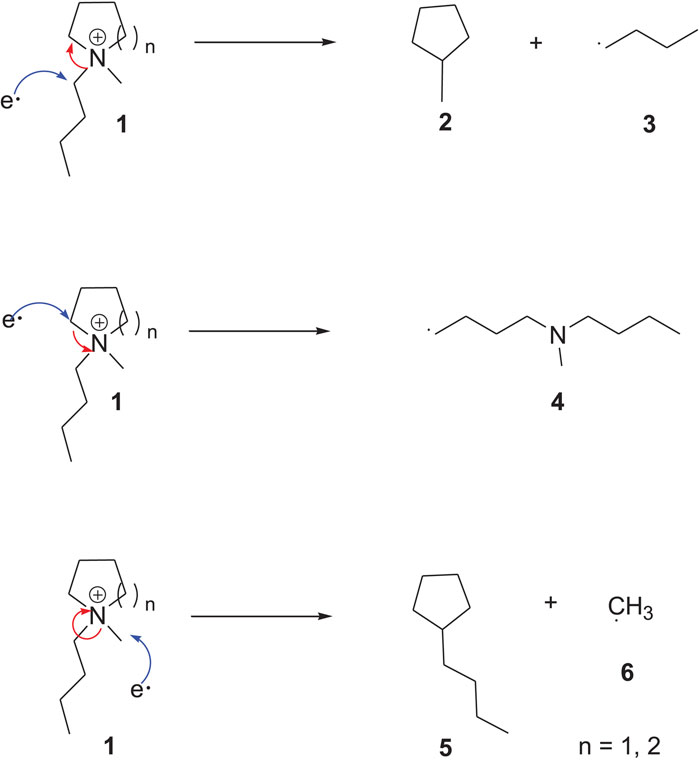
SCHEME 1. Electrochemical decomposition of pyrrolidinium/piperidinium cation (Kroon et al., 2006).
It has been reported that
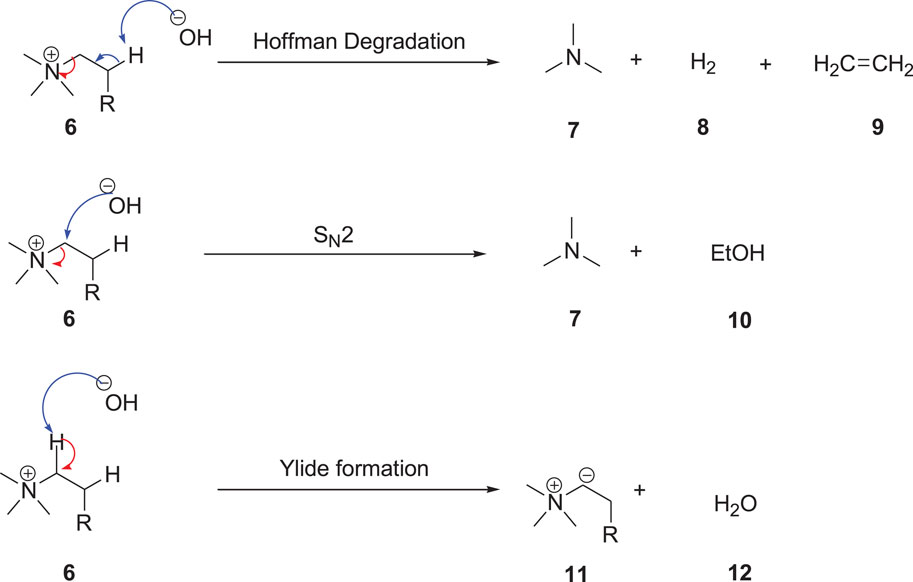
SCHEME 2. Degradation pathways of ammonium ILs (Ramnial et al., 2008).
Table 3 also shows that the magnitude of
The expected, resulting increase in
As expected, both Imidazolium ILs (with [EMim]+ cation) showed the lowest

SCHEME 3. Imidazolium cation reduction by carbene and radical formation(Xiao, 2002) (Noack et al., 2010).
Nuclear magnetic resonance (NMR) spectroscopy analysis of the imidazolium ILs after continuous electrolysis showed that the C2 position of the imidazolium ring was altered and resulted in the loss of aromaticity of the imidazolium cation and the formation of a neutral radical 14. The radical can subsequently convert to carbene 15 with the evolution of H2 gas (Noack et al., 2010). This carbene can exist in the ILs phase if the substituents on the imidazolium nitrogen atoms are suitable. Otherwise, it can react further and result in the formation of dimer 17, or it can react with other imidazolium species and form a saturated C2 carbon atom containing cage-like molecule 21, as shown in Scheme 4 (Xiao, 2002).
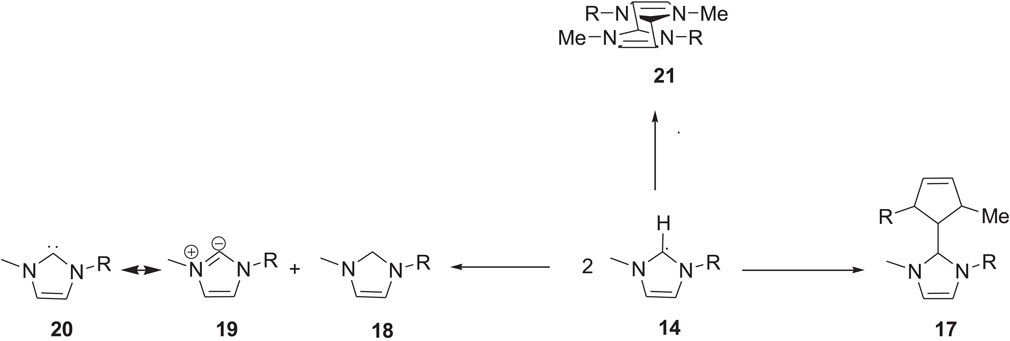
SCHEME 4. Dimer and cage-like structure formation of the imidazolium (Xiao, 2002).
The C2 hydrogen is also primarily responsible for the thermal degradation of imidazolium ILs. The C2 hydrogen is acidic and can undergo deprotonation even in neutral media if the anion is slightly basic (Handy and Okello, 2005). In the presence of strongly basic anions such as hydroxide (Noack et al., 2010), the deprotonation occurs faster. It will result in the formation of N-heterocyclic carbenes (Díez-González et al., 2009), which eventually opens the imidazolium ring by the nucleophilic addition of the hydroxide ion (Chetsanga and Makaroff, 1982).
The
3.3 Effect of Anions
It has been reported (Matsumoto et al., 2005) that [TFSI]- anion based ILs have wider
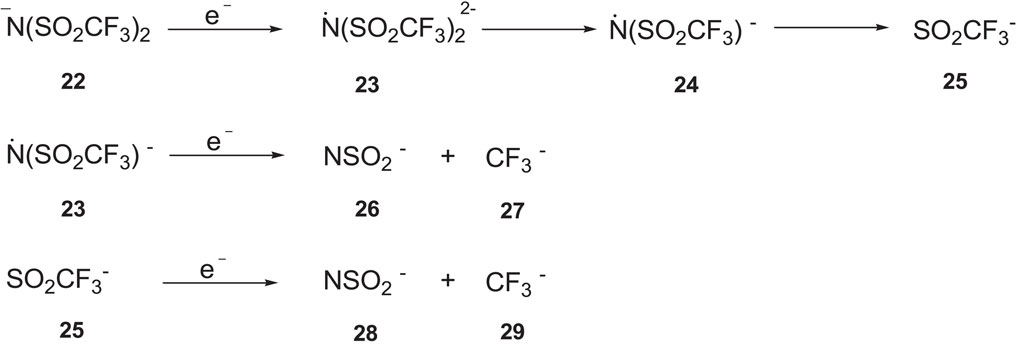
SCHEME 5. Electrochemical degradation of [TFSI] anion (Howlett et al., 2006).
Comparing ILs herein with both [TFSI]- and [FSI]- anions, we observed a mixed trend in electrochemical stability (Table 3 and Figure 4), which is largely due to the similarity in both anion structures. In the case of Imidazolium ILs, the [TFSI]- anions have a noticeable higher
In general, ILs with [FSI] anion showed higher Ec in comparison to its [TFSI] anion counterpart. Although the cathodic limit is largely attributed to the reduction potential of the constituent cation (earlier hinted in introduction), earlier discussions (DeVos et al., 2014) also indicate that for some ion pairs, further reduction of the anion could be preferential at the cathode–just as further oxidation of the cation could also be preferential on the anode. This could explain out observations why; out of 6 cations combined with both [TFSI] and [FSI] in our study, three of them showed this trend (Ec higher with FSI than TFSI), two showed the opposite, while only one reported same value for Ec (when [TFSI] and [FSI] are in the presence of [Pyr 1,4] cation). The actual Ec values tagged when [FSI] and [TFSI] are paired with the same cation could also differ given ion mobility variation with different bond strength with specific cation, among other reasons.
For ether functionalized ILs (like [N 1,1,2,102][TFSI] and [N 1,1,2,102][FSI]),
Although [TFSI]- anion paired with pyrrolidinium ILs showed lower
The
For
3.4 Effect of Functional Groups
In general, the inclusion of functional groups can affect the properties of ILs. For example, the introduction of ether groups on IL cations can reduce their viscosity (Raj et al., 2019). It was suspected that the ether functionality could increase the
The introduction of the aromatic groups on ammonium cations has a similar effect as the ether functionality. The presence of the aromatic groups reduced the electrochemical window considerably. The standard
4 Conclusion
Twenty two commercially available ionic liquids with bis(trifluoromethanesulfonyl)imide and bis(fluorosulfonyl)imide anions were used to investigate temperature effect on electrochemical stability window on ionic liquids. Within the temperature range investigated (283.15–363.15 K), the increasing temperature had mixed results on the electrochemical stability of ionic liquids with different cation types investigated. Although both anions used in the study have a similar structure, bis(trifluoromethanesulfonyl) imide anions showed slightly higher electrochemical stability overall than bis(fluorosulfonyl)imide anions.
We confirm that increase in the alkyl spacer length on the cation increased ionic liquids’ electrochemical stability compared to their short alkyl chain counterpart. In contrast, the aromatic functional groups on the cation significantly reduced their electrochemical stability window, as seen in the case of N, N-Dimethyl-N-ethyl-N-benzylammonium bis(trifluoromethanesulfonyl)imide. The presence of ether functionality on the cationic core also reduces the electrochemical stability window, which is evident in the case of N-Ethyl-N, N-dimethyl-N-(2-methoxyethyl) ammonium bis(trifluoromethanesulfonyl)imide. Among the different ionic liquids studied, Imidazolium ionic liquids (1-Ethyl-3-methylimidazolium bis(fluorosulfonyl)imide) showed the lowest electrochemical window and pyrrolidinium (N-Pentyl-N-methylpyrrolidinium bis(trifluoromethanesulfonyl)imide) and tetraalkylammonium ionic liquids (N-Tributyl-N-methylammonium bis(trifluoromethanesulfonyl)imide) with more extended alkyl groups showed the largest electrochemical stability window.
The limited variation of ionic mobilities/diffusivities within the temperature range investigated is a significant factor behind generally observed changes for anodic, cathodic, and subsequently electrochemical stability of the ILs. Given the different cation types were paired with two anion types, recorded cathodic potential limits were more sensitive to increasing temperature than the anodic potential limits.
Data Availability Statement
The raw data supporting the conclusion of this article will be made available by the authors, following institutional clearance.
Author Contributions
KL: Conceptualization, Verification, Writing an original draft. AB: Methodology, Verification, Analysis. MA: Software. MB: Software, Validation, Methodology, Reviewing and editing. RS: Conceptualization, Methodology, Supervision, Reviewing and editing.
Funding
The authors acknowledge the financial support from the R&D centre, Dubai Electricity and Water Authority (DEWA), for the project, high-voltage supercapacitor cell for grid applications.
Conflict of Interest
The authors declare that the research was conducted in the absence of any commercial or financial relationships that could be construed as a potential conflict of interest.
Publisher’s Note
All claims expressed in this article are solely those of the authors and do not necessarily represent those of their affiliated organizations, or those of the publisher, the editors and the reviewers. Any product that may be evaluated in this article, or claim that may be made by its manufacturer, is not guaranteed or endorsed by the publisher.
Supplementary Material
The Supplementary Material for this article can be found online at: https://www.frontiersin.org/articles/10.3389/fchem.2022.859304/full#supplementary-material
References
Appetecchi, G. B., Montanino, M., Zane, D., Carewska, M., Alessandrini, F., and Passerini, S. (2009). Effect of the Alkyl Group on the Synthesis and the Electrochemical Properties of N-Alkyl-N-Methyl-Pyrrolidinium Bis(trifluoromethanesulfonyl)imide Ionic Liquids. Electrochimica Acta 54, 1325–1332. doi:10.1016/j.electacta.2008.09.011
Bahadori, L., Boyd, R., Warrington, A., Shafeeyan, M. S., and Nockemann, P. (2020). Evaluation of Ionic Liquids as Electrolytes for Vanadium Redox Flow Batteries. J. Mol. Liq. 317, 114017. doi:10.1016/j.molliq.2020.114017
Bonhôte, P., Dias, A.-P., Papageorgiou, N., Kalyanasundaram, K., and Grätzel, M. (1996). Hydrophobic, Highly Conductive Ambient-Temperature Molten Salts. Inorg. Chem. 35, 1168–1178. doi:10.1021/ic951325x
Buzzeo, M. C., Evans, R. G., and Compton, R. G. (2004). Non-Haloaluminate Room-Temperature Ionic Liquids in Electrochemistry-A Review. ChemPhysChem 5, 1106–1120. doi:10.1002/cphc.200301017
Cao, Y., Chen, Y., Sun, X., Zhang, Z., and Mu, T. (2012). Water Sorption in Ionic Liquids: Kinetics, Mechanisms and Hydrophilicity. Phys. Chem. Chem. Phys. 14, 12252–12262. doi:10.1039/C2CP41798G
Cao, Y., and Mu, T. (2014). Comprehensive Investigation on the Thermal Stability of 66 Ionic Liquids by Thermogravimetric Analysis. Ind. Eng. Chem. Res. 53, 8651–8664. doi:10.1021/ie5009597
Chellappan, L. K., Kvello, J., Tolchard, J. R., Dahl, P. I., Hanetho, S. M., Berthelot, R., et al. (2020). Non-nucleophilic Electrolyte Based on Ionic Liquid and Magnesium Bis(diisopropyl)amide for Rechargeable Magnesium-Ion Batteries. ACS Appl. Energy Mat. 3, 9585–9593. doi:10.1021/acsaem.0c01026
Chetsanga, C. J., and Makaroff, C. (1982). Alkaline Opening of Imidazole Ring of 7-methylguanosine. 2. Further Studies on Reaction Mechanisms and Products. Chemico-Biological Interact. 41, 235–249. doi:10.1016/0009-2797(82)90092-8
de Rooij, D. M. R. (2003). Electrochemical Methods: Fundamentals and Applications. Anti-Corrosion Methods Mater 50. doi:10.1108/acmm.2003.12850eae.001
De Vos, N., Maton, C., and Stevens, C. V. (2014). Electrochemical Stability of Ionic Liquids: General Influences and Degradation Mechanisms. ChemElectroChem 1, 1258–1270. doi:10.1002/celc.201402086
Díez-González, S., Marion, N., and Nolan, S. P. (2009). N-heterocyclic Carbenes in Late Transition Metal Catalysis. Chem. Rev. 109, 3612–3676. doi:10.1021/cr900074m
Doherty, A. P. (2018). Redox-active Ionic Liquids for Energy Harvesting and Storage Applications. Curr. Opin. Electrochem. 7, 61–65. doi:10.1016/j.coelec.2017.10.009
Freire, M. G., Neves, C. M. S. S., Carvalho, P. J., Gardas, R. L., Fernandes, A. M., Marrucho, I. M., et al. (2007). Mutual Solubilities of Water and Hydrophobic Ionic Liquids. J. Phys. Chem. B 111, 13082–13089. doi:10.1021/jp076271e
Gancarz, P., Zorębski, E., and Dzida, M. (2021). Influence of Experimental Conditions on the Electrochemical Window. Case Study on Bis(trifluoromethylsulfonyl)imide-Based Ionic Liquids. Electrochem. Commun. 130, 107107. doi:10.1016/j.elecom.2021.107107
Grøssereid, I., Lethesh, K. C., Venkatraman, V., and Fiksdahl, A. (2019). New Dual Functionalized Zwitterions and Ionic Liquids; Synthesis and Cellulose Dissolution Studies. J. Mol. Liq. 292, 111353. doi:10.1016/j.molliq.2019.111353
Handy, S. T., and Okello, M. (2005). The 2-position of Imidazolium Ionic Liquids: Substitution and Exchange. J. Org. Chem. 70, 1915–1918. doi:10.1021/jo0480850
Hayyan, M., Mjalli, F. S., Hashim, M. A., AlNashef, I. M., and Mei, T. X. (2013). Investigating the Electrochemical Windows of Ionic Liquids. J. Industrial Eng. Chem. 19, 106–112. doi:10.1016/j.jiec.2012.07.011
Howlett, P. C., Izgorodina, E. I., Forsyth, M., and MacFarlane, D. R. (2006). Electrochemistry at Negative Potentials in Bis(trifluoromethanesulfonyl)amide Ionic Liquids. Z. fur Phys. Chem. 220, 1483–1498. doi:10.1524/zpch.2006.220.10.1483
Huddleston, J. G., Visser, A. E., Reichert, W. M., Willauer, H. D., Broker, G. A., and Rogers, R. D. (2001). Characterization and Comparison of Hydrophilic and Hydrophobic Room Temperature Ionic Liquids Incorporating the Imidazolium Cation. Green Chem. 3, 156–164. doi:10.1039/b103275p
Kermanioryani, M., Abdul Mutalib, M. I., Gonfa, G., El-Harbawi, M., Mazlan, F. A., Lethesh, K. C., et al. (2016). Using Tunability of Ionic Liquids to Remove Methylene Blue from Aqueous Solution. J. Environ. Chem. Eng. 4, 2327–2332. doi:10.1016/j.jece.2016.04.008
Kim, Y.-J., Matsuzawa, Y., Ozaki, S., Park, K. C., Kim, C., Endo, M., et al. (2005). High Energy-Density Capacitor Based on Ammonium Salt Type Ionic Liquids and Their Mixing Effect by Propylene Carbonate. J. Electrochem. Soc. 152, A710. doi:10.1149/1.1869232
Koch, V. R., Dominey, L. A., Nanjundiah, C., and Ondrechen, M. J. (1996). The Intrinsic Anodic Stability of Several Anions Comprising Solvent‐Free Ionic Liquids. J. Electrochem. Soc. 143, 798–803. doi:10.1149/1.1836540
Kroon, M. C., Buijs, W., Peters, C. J., and Witkamp, G.-J. (2006). Decomposition of Ionic Liquids in Electrochemical Processing. Green Chem. 8, 241–245. doi:10.1039/b512724f
Le, M.-L. -P., Alloin, F., Strobel, P., Leprêtre, J.-C., Cointeaux, L., and del Valle, C. P. (2012). Electrolyte Based on Fluorinated Cyclic Quaternary Ammonium Ionic Liquids. Ionics 18, 817–827. doi:10.1007/s11581-012-0688-x
Lethesh, K. C., Bamgbopa, M. O., and Susantyoko, R. A. (2021). Prospects and Design Insights of Neat Ionic Liquids as Supercapacitor Electrolytes. Front. Energy Res. 9. doi:10.3389/fenrg.2021.741772
Lethesh, K. C., Dehaen, W., and Binnemans, K. (2014). Base Stable Quaternary Ammonium Ionic Liquids. RSC Adv. 4, 4472–4477. doi:10.1039/c3ra45126g
Lethesh, K. C., Evjen, S., Raj, J. J., Roux, D. C. D., Venkatraman, V., Jayasayee, K., et al. (2019). Hydroxyl Functionalized Pyridinium Ionic Liquids: Experimental and Theoretical Study on Physicochemical and Electrochemical Properties. Front. Chem. 7, 625. doi:10.3389/fchem.2019.00625
Li, Q., Jiang, J., Li, G., Zhao, W., Zhao, X., and Mu, T. (2016). The Electrochemical Stability of Ionic Liquids and Deep Eutectic Solvents. Sci. China Chem. 59, 571–577. doi:10.1007/s11426-016-5566-3
Lian, C., Liu, H., Li, C., and Wu, J. (2019). Hunting Ionic Liquids with Large Electrochemical Potential Windows. AIChE J. 65, 804–810. doi:10.1002/aic.16467
Lin, X., Salari, M., Arava, L. M. R., Ajayan, P. M., and Grinstaff, M. W. (2016). High Temperature Electrical Energy Storage: Advances, Challenges, and Frontiers. Chem. Soc. Rev. 45, 5848–5887. doi:10.1039/c6cs00012f
Liu, K., Zhou, Y.-X., Han, H.-B., Zhou, S.-S., Feng, W.-F., Nie, J., et al. (2010). Ionic Liquids Based on (Fluorosulfonyl)(pentafluoroethanesulfonyl)imide with Various Oniums. Electrochimica Acta 55, 7145–7151. doi:10.1016/j.electacta.2010.06.085
Long, H., Kim, K., and Pivovar, B. S. (2012). Hydroxide Degradation Pathways for Substituted Trimethylammonium Cations: A DFT Study. J. Phys. Chem. C 116, 9419–9426. doi:10.1021/jp3014964
Ma, K., Zhang, C., Woodward, C. E., and Wang, X. (2018). Bridging the Gap between Macroscopic Electrochemical Measurements and Microscopic Molecular Dynamic Simulations: Porous Carbon Supercapacitor with Ionic Liquids. Electrochimica Acta 289, 29–38. doi:10.1016/j.electacta.2018.09.016
MacFarlane, D. R., Meakin, P., Sun, J., Amini, N., and Forsyth, M. (1999). Pyrrolidinium Imides: A New Family of Molten Salts and Conductive Plastic Crystal Phases. J. Phys. Chem. B 103, 4164–4170. doi:10.1021/jp984145s
Maton, C., De Vos, N., Roman, B. I., Vanecht, E., Brooks, N. R., Binnemans, K., et al. (2012). Continuous Synthesis of Peralkylated Imidazoles and Their Transformation into Ionic Liquids with Improved (Electro)chemical Stabilities. ChemPhysChem 13, 3146–3157. doi:10.1002/cphc.201200343
Maton, C., De Vos, N., and Stevens, C. V. (2013). Ionic Liquid Thermal Stabilities: Decomposition Mechanisms and Analysis Tools. Chem. Soc. Rev. 42, 5963–5977. doi:10.1039/c3cs60071h
Matsumoto, H., Sakaebe, H., and Tatsumi, K. (2005). Preparation of Room Temperature Ionic Liquids Based on Aliphatic Onium Cations and Asymmetric Amide Anions and Their Electrochemical Properties as a Lithium Battery Electrolyte. J. Power Sources 146, 45–50. doi:10.1016/j.jpowsour.2005.03.103
Montanino, M., Carewska, M., Alessandrini, F., Passerini, S., and Appetecchi, G. B. (2011). The Role of the Cation Aliphatic Side Chain Length in Piperidinium Bis(trifluoromethansulfonyl)imide Ionic Liquids. Electrochimica Acta 57, 153–159. Elsevier Ltd. doi:10.1016/j.electacta.2011.03.089
Mousavi, M. P. S., Dittmer, A. J., Wilson, B. E., Hu, J., Stein, A., and Bühlmann, P. (2015). Unbiased Quantification of the Electrochemical Stability Limits of Electrolytes and Ionic Liquids. J. Electrochem. Soc. 162, A2250–A2258. doi:10.1149/2.0271512jes
Neale, A. R., Murphy, S., Goodrich, P., Hardacre, C., and Jacquemin, J. (2017). Thermophysical and Electrochemical Properties of Ethereal Functionalised Cyclic Alkylammonium-Based Ionic Liquids as Potential Electrolytes for Electrochemical Applications. ChemPhysChem 18, 2040–2057. doi:10.1002/cphc.201700246
Neale, A. R., Murphy, S., Goodrich, P., Schütter, C., Hardacre, C., Passerini, S., et al. (2016). An Ether-Functionalised Cyclic Sulfonium Based Ionic Liquid as an Electrolyte for Electrochemical Double Layer Capacitors. J. Power Sources 326, 549–559. doi:10.1016/j.jpowsour.2016.06.085
Noack, K., Schulz, P. S., Paape, N., Kiefer, J., Wasserscheid, P., and Leipertz, A. (2010). The Role of the C2 Position in Interionic Interactions of Imidazolium Based Ionic Liquids: A Vibrational and NMR Spectroscopic Study. Phys. Chem. Chem. Phys. 12, 14153–14161. doi:10.1039/c0cp00486c
Olson, E. J., and Bühlmann, P. (2013). Unbiased Assessment of Electrochemical Windows: Minimizing Mass Transfer Effects on the Evaluation of Anodic and Cathodic Limits. J. Electrochem. Soc. 160, A320–A323. doi:10.1149/2.068302jes
O’Mahony, A. M., Silvester, D. S., Aldous, L., Hardacre, C., and Compton, R. G. (2008). Effect of Water on the Electrochemical Window and Potential Limits of Room-Temperature Ionic Liquids. J. Chem. Eng. Data 53, 2884–2891. doi:10.1021/je800678e
Pandian, S., Hariharan, K. S., Adiga, S. P., and Kolake, S. M. (2020). Evaluation of Electrochemical Stability and Li-Ion Interactions in Ether Functionalized Pyrrolidinium and Phospholanium Ionic Liquids. J. Electrochem. Soc. 167, 070550. doi:10.1149/1945-7111/ab8061
Paul, A., Muthukumar, S., and Prasad, S. (2020). Review-Room-Temperature Ionic Liquids for Electrochemical Application with Special Focus on Gas Sensors. J. Electrochem. Soc. 167, 037511. doi:10.1149/2.0112003jes
Raj, J. J., Magaret, S., Pranesh, M., Lethesh, K. C., Devi, W. C., and Mutalib, M. I. A. (2019). Dual Functionalized Imidazolium Ionic Liquids as a Green Solvent for Extractive Desulfurization of Fuel Oil: Toxicology and Mechanistic Studies. J. Clean. Prod. 213, 989–998. doi:10.1016/j.jclepro.2018.12.207
Ramnial, T., Taylor, S. A., Bender, M. L., Gorodetsky, B., Lee, P. T. K., Dickie, D. A., et al. (2008). Carbon-centered Strong Bases in Phosphonium Ionic Liquids. J. Org. Chem. 73, 801–812. doi:10.1021/jo701289d
Randström, S., Appetecchi, G. B., Lagergren, C., Moreno, A., and Passerini, S. (2007). The Influence of Air and its Components on the Cathodic Stability of N-Butyl-N-Methylpyrrolidinium Bis(trifluoromethanesulfonyl)imide. Electrochimica Acta 53, 1837–1842. doi:10.1016/j.electacta.2007.08.029
Randström, S., Montanino, M., Appetecchi, G. B., Lagergren, C., Moreno, A., and Passerini, S. (2008). Effect of Water and Oxygen Traces on the Cathodic Stability of N-Alkyl-N-Methylpyrrolidinium Bis(trifluoromethanesulfonyl)imide. Electrochimica Acta 53, 6397–6401. doi:10.1016/j.electacta.2008.04.058
Reiter, J., Jeremias, S., Paillard, E., Winter, M., and Passerini, S. (2013a). Fluorosulfonyl-(trifluoromethanesulfonyl)imide Ionic Liquids with Enhanced Asymmetry. Phys. Chem. Chem. Phys. 15, 2565–2571. doi:10.1039/c2cp43066e
Reiter, J., Paillard, E., Grande, L., Winter, M., and Passerini, S. (2013b). Physicochemical Properties of N-Methoxyethyl-N-Methylpyrrolidinum Ionic Liquids with Perfluorinated Anions. Electrochimica Acta 91, 101–107. doi:10.1016/j.electacta.2012.12.086
Shen, F., Wang, S., and Gao, Y. (2021). Making SOCl2 Rechargeable. Joule 5, 2766–2767. doi:10.1016/j.joule.2021.09.013
Sun, J., Forsyth, M., and MacFarlane, D. R. (1998). Room-temperature Molten Salts Based on the Quaternary Ammonium Ion. J. Phys. Chem. B 102, 8858–8864. doi:10.1021/JP981159P
Ue, M., Ida, K., and Mori, S. (1994). Electrochemical Properties of Organic Liquid Electrolytes Based on Quaternary Onium Salts for Electrical Double‐Layer Capacitors. J. Electrochem. Soc. 141, 2989–2996. doi:10.1149/1.2059270
Wasserscheid, P., and Welton, T. (2008). Ionic Liquids in Synthesis. Second Edition. doi:10.1002/9783527621194
Xiao, L. (2002). Electrochemistry of 1-Butyl-3-Methyl-1h-Imidazolium Tetrafluoroborate Ionic Liquid. Proc. Vol. 2002-19, 910–922. doi:10.1149/200219.0910pv
Xu, K., Ding, M. S., and Jow, T. R. (2001). Quaternary Onium Salts as Nonaqueous Electrolytes for Electrochemical Capacitors. J. Electrochem. Soc. 148, A267. doi:10.1149/1.1350665
Xue, Z., Qin, L., Jiang, J., Mu, T., and Gao, G. (2018). Thermal, Electrochemical and Radiolytic Stabilities of Ionic Liquids. Phys. Chem. Chem. Phys. 20, 8382–8402. doi:10.1039/c7cp07483b
Yim, T., Choi, C. Y., Mun, J., Oh, S., and Kim, Y. G. (2009). Synthesis and Properties of Acyclic Ammonium-Based Ionic Liquids with Allyl Substituents as Electrolytes. Molecules 14, 1840–1851. doi:10.3390/molecules14051840
Yim, T., Hyun, Y. L., Kim, H. J., Mun, J., Kim, S., Oh, S. M., et al. (2007). Synthesis and Properties of Pyrrolidinium and Piperidinium Bis(trifluoromethanesulfonyl)imide Ionic Liquids with Allyl Substituents. Bull. Korean Chem. Soc. 28, 1567–1572. doi:10.5012/bkcs.2007.28.9.1567
Zhang, J., and Bond, A. M. (2005). Practical Considerations Associated with Voltammetric Studies in Room Temperature Ionic Liquids. Analyst 130, 1132–1147. doi:10.1039/b504721h
Zhang, L., Tsay, K., Bock, C., and Zhang, J. (2016). Ionic Liquids as Electrolytes for Non-aqueous Solutions Electrochemical Supercapacitors in a Temperature Range of 20 °C-80 °C. J. Power Sources 324, 615–624. doi:10.1016/j.jpowsour.2016.05.008
Zhang, S., Sun, N., He, X., Lu, X., and Zhang, X. (2006). Physical Properties of Ionic Liquids: Database and Evaluation. J. Phys. Chem. Reference Data 35, 1475–1517. doi:10.1063/1.2204959
Keywords: electrochemical stability, temperature, linear regression, TFSI, FSI, bis(trifluoromethanesulfonyl)imide, bis(fluorosulfonyl)imide, ionic liquids
Citation: Lethesh KC, Bahaa A, Abdullah M, Bamgbopa MO and Susantyoko RA (2022) Temperature-Dependent Electrochemical Stability Window of Bis(trifluoromethanesulfonyl)imide and Bis(fluorosulfonyl)imide Anion Based Ionic Liquids. Front. Chem. 10:859304. doi: 10.3389/fchem.2022.859304
Received: 21 January 2022; Accepted: 26 April 2022;
Published: 17 June 2022.
Edited by:
Vito Di Noto, University of Padua, ItalyReviewed by:
Kazuhide Ueno, Yokohama National University, JapanGioele Pagot, University of Padua, Italy
Copyright © 2022 Lethesh, Bahaa, Abdullah, Bamgbopa and Susantyoko. This is an open-access article distributed under the terms of the Creative Commons Attribution License (CC BY). The use, distribution or reproduction in other forums is permitted, provided the original author(s) and the copyright owner(s) are credited and that the original publication in this journal is cited, in accordance with accepted academic practice. No use, distribution or reproduction is permitted which does not comply with these terms.
*Correspondence: Rahmat Agung Susantyoko, cmFobWF0LnN1c2FudHlva29AZGV3YS5nb3YuYWU=, cmFobWF0LmEuc3VzYW50eW9rb0BhbHVtLm1pdC5lZHU=
†These authors have contributed equally to this work