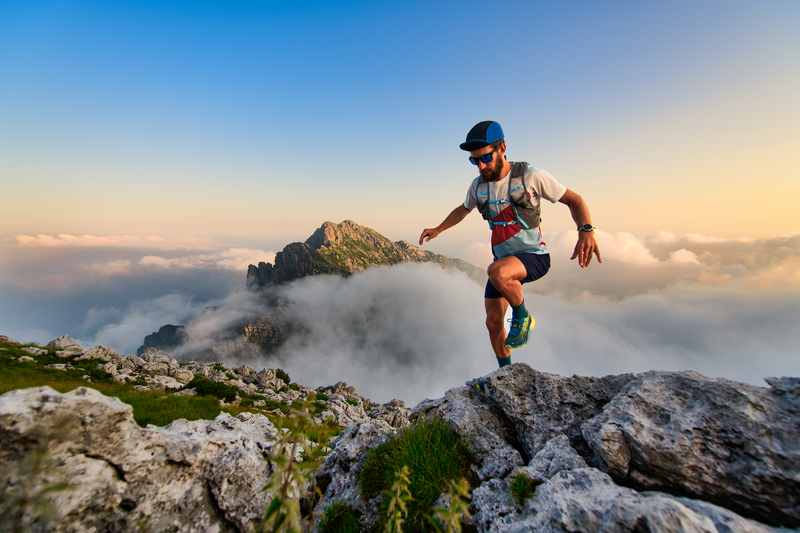
95% of researchers rate our articles as excellent or good
Learn more about the work of our research integrity team to safeguard the quality of each article we publish.
Find out more
ORIGINAL RESEARCH article
Front. Chem. , 11 March 2022
Sec. Analytical Chemistry
Volume 10 - 2022 | https://doi.org/10.3389/fchem.2022.856003
This article is part of the Research Topic Optical and Electrochemical Biosensing View all 11 articles
Hydrogen peroxide (H2O2) is the most significant reactive oxygen species in biological systems. Here, we reported an electrochemical sensor for the detection of H2O2 on the basis of bimetallic gold-platinum nanoparticles (Au3Pt7 NPs) supported by Co-based metal organic frameworks (Co-MOFs). First, Au3Pt7 NPs, with optimal electrocatalytic activity and accessible active surface, can be deposited on the surface of the Co-MOF–modified glassy carbon electrodes (Au3Pt7/Co-MOFs/GCE) by one-step electrodeposition method. Then, the electrochemical results demonstrated that the two-dimensional (2D) Co-MOF nanosheets as the supporting material displayed better electrocatalytic properties than the 3D Co-MOF crystals for reduction of H2O2. The fabricated Au3Pt7/2D Co-MOF exhibited high electrocatalytic activity, and the catalytic current was linear with H2O2 concentration from 0.1 μM to 5 mM, and 5–60 mM with a low detection limit of 0.02 μM (S/N = 3). The remarkable electroanalytical performance of Au3Pt7/2D Co-MOF can be attributed to the synergistic effect of the high dispersion of the Au3Pt7 NPs with the marvelous electrochemical properties and the 2D Co-MOF with high-specific surface areas. Furthermore, this sensor has been utilized to detect H2O2 concentrations released from the human Hela cells. This work provides a new method for improving the performance of electrochemical sensors by choosing the proper support materials from diverse crystal morphology materials.
Hydrogen peroxide (H2O2), as one of the most significant reactive oxygen species (ROS), commonly exists in biological processes as a ubiquitous intracellular messenger or receptor signaling in various cells (Ushio-Fukai et al., 1999). On the one hand, H2O2 was considered as a toxic by-product during the process of aerobic metabolism (Rhee et al., 2005). On the other hand, some serious human diseases will be triggered by an abnormal concentration level of H2O2 (Trachootham et al., 2009; Yang et al., 2011), including myocardial infarct (Griendling, 2004), Alzheimer’s disease (Markesbery, 1997), aging (Giorgio et al., 2007; Hayyan et al., 2016), and cancers (Jorgenson et al., 2013). Therefore, developing an accurate and sensitive detection method to monitor the H2O2 concentration at the cellular level is vital and urgent for future clinic diagnosis and cancer treatment (Dong et al., 2019a). Until now, various analytical technologies have been utilized to detect H2O2, such as fluorescence (Ren et al., 2016; Ma et al., 2017), chemiluminescence (Yuan and Shiller, 1999; Xie and Huang, 2011), spectrophotometry (Yu et al., 2017), chromatography (Gimeno et al., 2015), and electrochemistry. Compared with the established analytical methods, the electrochemical sensing technique is a promising approach to attain dynamic analysis of H2O2 concentration for its outstanding properties, specifically high sensitivity, good selectivity, simple operation, and low cost.
Electrochemical sensors for testing H2O2 can be subdivided into natural enzymes and nanomaterial-based artificial enzymes (nanozymes)–based sensors. The natural enzymes were known as peroxidases, such as horseradish peroxidase (Sun et al., 2004). The enzyme-based electrochemical sensors have been widely utilized in electroanalysis for the advantage of specificity and fast response to the target substance. However, natural enzymes are restricted to their inherent weaknesses, including the uncontrollable deactivation and hardship in purification (Wang et al., 2018). Therefore, to overcome the shortages of natural enzymes, nanozymes have been taken into consideration as robust alternatives. Until now, a series of nanomaterials have been proved to possess intrinsic peroxidase-mimicking catalytic properties (Wu et al., 2019), including noble metals, metal oxides, and carbon-based nanomaterials (Wei and Wang, 2013).
Among various enzyme mimics, bimetallic nanoparticles (NPs) have been widely employed to fabricate electrochemical biosensors. It usually performed better electrocatalytic properties than its monometallic counterparts (Iyyamperumal et al., 2013; Li et al., 2020; Yang et al., 2021). As previously reported, the properties of electrons can be improved by the synergistic effects of each monometallic nanomaterials (Renner et al., 2006). However, few limitations are constraining the further application of these bimetallic particles-based electrochemical sensors: 1) it is usually a challenge to meet the satisfactory sensitivity of the living cells detection; and 2) their thermodynamic instability and tendency to aggregate, owing to their high surface free energy (Wang et al., 2019).
These disadvantages can be improved by immobilizing metal NPs in/on supporting structures. Recently, metal organic frameworks (MOFs) formed by the self-assembly of organic ligands and metal ions (Batten et al., 2013) have drawn extensive attention as supporting materials because of their inherent advantages (Furukawa et al., 2013). Among various MOFs, Co-based MOFs were widely used in fabricating electrochemical sensors as supporting material (Dong et al., 2019b). According to the previous research, the zeolite imidazolate framework (ZIF)–67 which compounded of the imidazole-based organic linkers and transition metal (Co) displaying a desirable 3D structure rhombic dodecahedron morphology, and it has been widely utilized for fabricating the 3D Co-MOF–modified electrodes. Lu et al. (2020) constructed a novel electrochemical sensor with the help of 3D Co-MOF to load artificial enzymes Ag nanostructures. Recently, two-dimensional (2D) Co-MOF has drawn extensive attention as supporting material in fabricating electrochemical sensors. The meso-tetra-(4-carboxyphenyl)–substituted porphyrins (TCPP) is the excellent ligand for the constructing 2D MOF due to their tetragonal symmetry and rigid structure (Zhao et al., 2018a). When the TCPP ligands connect with Co2+, 2D Co-MOF nanosheets was formed. Four Co paddle-wheel metal nodes link one TCPP ligand. These TCPP linked ultrasmall 2D metalloporphyrinic MOF nanosheets have been used to fabricating electrochemical sensors for electrochemical catalyzing and sensing applications (Zhao et al., 2015). For example, Huang et al. (2017) reported a novel sensor for catalyzing the cascade reactions. The electrochemical sensor was fabricated by peroxidase mimics material Au NPs and artificial glucose oxidase, ultrasmall 2D metalloporphyrinic MOF nanomaterial. Ma et al. (2019) reported a novel electrochemical sensor fabricated by TCPP-based 2D Cu-MOF nanosheets and Ag NPs. Because of the smaller size and more accessible active sites (Xu et al., 2016; Zhao et al., 2018b), 2D Co-MOF may combine other functionalized materials better than 3D bulk MOF crystals (Tan et al., 2017; Liu et al., 2020). However, which morphology of MOF can perform better in loading artificial enzymes that still need more exploration?
In this work, our experiments first tried to answer whether AuPt NPs are a much better catalyst for H2O2 reduction reaction than either Au or Pt NPs. Then, we evaluated the electrocatalytic activity of 3D and 2D Co-MOF–modified electrodes, figuring out the influences of morphology for a supporting material. During the electrodeposition process with a negative voltage, AuPt NPs can be easily reduced from the solution adhering to the surface of the working electrode steadily (Wirtz and Martin, 2003). After comparing a series ration of Au NPs and Pt NPs with (V:V = 1:9, 3:7, 5:5, 7:3, and 9:1), a novel sensor was constructed which bimetallic Au3Pt7 NPs decorated on the 2D Co-MOF–modified glassy carbon electrode (Au3Pt7/2D Co-MOFs/GCE) by electrodeposition. This high-performance electrochemical sensor was successfully utilized to monitor H2O2 concentration in real-time, and it performed a desirable property for tracing H2O2 in human cancer samples. Scheme 1 illustrated the detecting process of in suit analyzing of H2O2 secreted from Hela cells with drug stimulation by electrochemical sensor.
SCHEME 1. The electrochemical sensor for detecting H2O2 secreted from HeLa cells in response to drug stimulation.
The reagents and apparatus were provided in detail in the Supporting Information.
In this work, two kinds of pristine Co-MOF materials were prepared according to the published method with some improvements (Zhao et al., 2015; Sun et al., 2020).
First, 0.3 and 0.05 μM alumina slurries were used to polished the bare GCE (3 mm in diameter), until it shows mirror-like luster. Second, the electrodes were ultrasonicated in ultrapure water, ethanol, and ultrapure water. Last, nitrogen steam was used to dry the electrodes. A volume of 5 μl (2 mg/ml) of crystal ink of 2D Co-MOFs and 3D Co-MOFs were dropped on the GCE surface (the modified electrode was labeled as 2D Co-MOFs/GCE and 3D Co-MOFs/GCE), respectively. Then, the electrodes were air-dried at room temperature. After that, HAuCl4 (1.0 mM) and H2PtCl4 (1.0 mM) were mixed with the different volume ratio. The Co-MOFs/GCE was immersed in the mixed HAuCl4 and H2PtCl4 solution. AuPt NPs were grown on the surface of the modified electrodes by electrodepositing at −0.2 V (vs. Ag/AgCl). Last, two pristine Co-MOF–supported electrodes were fabricated, and these modified electrodes were washed by ultrapure water.
In the electrochemical experiments at the cellular level, 10 mM phosphate-buffered saline (PBS) with N2-saturating (pH 7.0) was utilized as the electrolyte to collect the living Hela cells. The details of Hela cells culturing condition were listed in the Supporting Information. With the help of cyclic voltammogram (CV) measurement and amperometric I-t experiment, the redox characteristics of the fabricated electrodes Au3Pt7/2D Co-MOF/GCE were fully approved and the I-t experiment potential was kept at −0.5 V.
Before the analyzing experiments, the cultured cells were gently washed twice with PBS. Then, 3 ml of PBS was added into the Hela cells culture dish, and 400 μM ascorbic acid (AA) was injected into the dish to stimulate the cells releasing H2O2. With the amperometric technique, the current response for H2O2 detection was recorded.
Here, we fabricated a bimetallic electrochemical sensor on the basis of Au3Pt7 NPs for H2O2 detection by CV measurements. Scanning electron microscopy (SEM) were employed to characterize the Au NPs, Pt NPs, and Au3Pt7 NPs. Figure 1A showed the SEM image of Au NPs that dispersedly immobilizing on the electrode. The Au NPs were of spheroidal nature, the average diameter of Au NPs was almost 20 nm. As recorded in the CV curves of Figure 1B, with different time of electrodeposition, the Au NP–modified electrodes showed an increased catalytic ability to H2O2, and the best catalytic response was obtained at 120 s. Figure 1C exhibited the histograms of the reduction peak current value of different electrodeposition time (t = 30, 60, 90, 120, and 150 s). The detection signal of H2O2 increased following the electrodeposition time, increasing until the electrochemical signal achieves a peak value of 120 s. Then, the current signal declined with continually increasing the electrodeposition time. The SEM image of Pt NPs is shown in Figure 1D, and the Pt NPs were of spheroidal nature, showing an average size of ∼50 nm. Figures 1E,F demonstrate the CV curves with different electrodeposition time of Pt NPs. The peak current increased from 30 to 120 s and dropped down until 150 s. The result demonstrated that, with the electrodeposition time increased to 120 s, the detection signal of H2O2 reduction achieved a peak value. Therefore, 120 s was chosen to be the suitable electrodeposition time of synthesized Au3Pt7 NPs. As shown in Figure 1G, the SEM image of Au3Pt7 NPs was distributed sparsely on the surface of GCE electrode, where a uniform NP structure with nearly 100 nm exists. Figures 1H,I depict the volume ratio of AuPt NPs (3:7), presenting the best catalytic ability to H2O2 with the different volume ratio of AuPt NPs (V:V = 1:9, 3:7, 5:5, 7:3, and 9:1). Au3Pt7 NPs was selected as the optimum nanozyme for fabricating the novel electrochemical sensors. From the results above, metal NPs all gain high catalytic abilities to H2O2, especially the Au3Pt7 bimetallic NPs, and the phenomenon may come from the several reasons as follows: 1) electrodeposition provided a considerable route for its low cost, immediate modification, stable current signal, and simple construction; and 2) Au3Pt7 NPs combining the advantages the high catalytic ability of the Pt NPs and the stability of Au NPs. Therefore, the electrochemical catalytic capability and the selectivity of Au3Pt7 have been improved (Sun et al., 2017; Yu et al., 2018).
FIGURE 1. (A) SEM images of Au NPs. (B,C) The CV curves and histograms of reduction peak current responses of Au/GCE for 2 mM H2O2 with different electrodeposition time (t = 30, 60, 90, 120, and 150 s) (n = 3). (D) SEM images of Pt NPs. (E,F) The CV curves and histograms of reduction peak current responses of Pt/GCE for 2 mM H2O2 with different electrodeposition time (t = 30, 60, 90, 120, and 150 s) (n = 3). (G) SEM images of Au3Pt7 NPs. (H,I) The CV curves and histograms of reduction peak current responses of Au3Pt7/GCE for 2 mM H2O2 with different volume ratio of Au NPs : Pt NPs (1:9, 3:7, 5:5, 7:3, and 9:1).
The morphology analysis of 2D and 3D Co-MOF was investigated by SEM, transmission electron microscopy (TEM), powder X-ray diffraction (XRD), X-ray photoelectron spectroscopy (XPS), and Fourier transform infrared (FT-IR) spectroscopy. As Supplementary Figure S1 shown, the collected products of pristine Co-MOF exhibited a powder-like 3D Co-MOF that was purple and 2D Co-MOF that was brown, matching with the previous report. Figure 2A shows the SEM image of 3D Co-MOF, which displays a 3D structure rhombic dodecahedron crystalline morphology with the uniform size of nearly 500 nm. Figure 2B reveals the 2D Co-MOF with a sheet-like structure. The crumpled and wrinkled surface indicates its ultrathin property. The TEM image supported 2D Co-MOF with a thin nanosheet structure and confirmed the structure shown in the SEM image. Figures 2C,D reveal the XRD patterns of the 3D Co-MOF and 2D Co-MOF. Figure 2E shows the chemical composition and states of the Co-MOF. As indicated from the two lines of XPS data, there were two main peaks of approximately 780 and 796 eV for 3D and 2D Co-MOF products, respectively, corresponding to Co 2p3/2 and Co 2p1/2 that are derived from Co2+. The two broad peaks at 786.0 and 802.8 eV were satellite peaks, matching well with the reported data. Then, for further investigating of the prepared pristine MOFs, FT-IR spectroscopic studies are shown in Figure 2F, and the characteristic absorption peak of 3D Co-MOF and 2D Co-MOF nanosheet appeared at nearly 716, 770, and 1,025 cm−1. These three peaks can prove the existing of skeleton vibration absorption peak of the porphyrin ring (Bai et al., 2019). The peak approximately 1,396 cm−1 is related to the stretching vibration of C=N. Besides, the peaks at 1,128 and 1,610 cm−1 are generated by the vibration of the benzene ring skeleton outside the pyrrole ring. The absorption peak at 1,700 cm−1 can be attributed to the C=O vibration of the carboxyl group on the benzene ring. These results demonstrated that the pristine Co-MOF products had been successfully synthesized.
FIGURE 2. SEM images of (A) 3D Co-MOF and (B) 2D Co-MOF. (C) TEM images of 2D Co-MOF. (D) XRD patterns of 3D Co-MOF and 2D Co-MOF. (E) XPS survey scan of 3D Co-MOF and 2D Co-MOF. (F) The FTIR spectra of 3D Co-MOF and 2D Co-MOF. (G,H) CV curves and EIS curves of GCE, 3D Co-MOF/GCE, and 2D Co-MOF/GCE in 5.0 mM K3Fe (CN)6/K4Fe (CN)6 solution containing 0.1 M KCl (amplitude 10 mV, impedance spectral frequency 0.1∼105 Hz). (I) CV curves of GCE, 3D Co-MOF/GCE and 2D Co-MOF/GCE in 100 mM PBS solution containing 10 mM H2O2 at a scan rate of 100 mV/s.
The examination of CV and electrochemical impedance spectroscopy (EIS) characterized that the pristine Co-MOF of two morphologies was decorated on the surface of the surface of GCE. The electrochemical study of the fabricated sensor in 5 mM K3Fe (CN)6/K4Fe (CN)6. As shown in Figures 2G,H, the electrochemical performances of the interfacial of the modified electrodes have been tested by CV and EIS. For the bare GCE, a couple of reversible redox peaks was observed, and the current signals of 3D Co-MOF and 2D Co-MOF–modified electrodes (2D Co-MOF/GCE) were decreased. Moreover, the diffusion-limited process of the electrons was showed in the Nyquist plots. The semicircle diameter displaying the charge transfer resistance (Rct) of the modified GCE electrodes. The picture of EIS showed the bad conductivity of the pristine 3D Co-MOF. The Rct of 2D Co-MOF/GCE was close to 800 Ω, and the Rct of 3D Co-MOF/GCE was close to 1,700 Ω. Three-dimensional Co-MOF performed worse than the bare GCE in electrical conductivity properties. Without interlayer interactions and electron confinement, 2D Co-MOF performed better than 3D Co-MOF and bare GCE. As shown in Figure 2I by using the CV technique, the electrocatalytic activity of the selected two pristine Co-MOF for H2O2 reduction was evident, indicating the current signal of the 2D Co-MOF/GCE closed to 50 μA, the 3D Co-MOF/GCE closed to 23 μA, and the bare GCE was 8 μA. These results illustrated that Co-MOF materials had little influence in the catalytic activity for H2O2 reduction in 100 mM PBS solution containing 10 mM H2O2, which can be ignored after the noble metal depositing on the Co-MOF–modified electrodes.
After figuring out the optimum volume ratio of Au3Pt7/GCE for H2O2 detection, the influence of the supporting materials was investigated. Electrodepositing Au3Pt7 NPs on Co-MOF/GCE, the morphology of Au3Pt7/3D Co-MOF/GCE and Au3Pt7/2D Co-MOF/GCE was observed by SEM measurements. Moreover, the CV measurement results exhibited that 2D Co-MOF performed better in supporting that Au3Pt7 NPs showed the best electrocatalytic activity to reduce H2O2. As Figures 3A,B reveal, Au3Pt7 NPs immobilized on the 3D Co-MOF sparsely with an average size of 100 nm. The corresponding EDS spectrum verified the existence of Co, Au, and Pt elements, as shown in Figure 3C and Supplementary Figure S2. The pictures of SEM clarified that the Au3Pt7 NPs located on the 2D Co-MOF/GCE uniformly (Figures 3D,E). The result of EDS element analysis clearly characterized the Au3Pt7 NPs combining on the nanosheet 2D Co-MOF. The Co, Au, and Pt elements can be observed distinctly in Figure 3F and Supplementary Figure S3. The result supported that the Au3Pt7/2D Co-MOF/GCE was synthesized successfully. The small Au3Pt7 NPs attached better to the 2D Co-MOF nanosheet layers than these were attached to 3D Co-MOF. The redox peak current was increased due to the excellent conductivity of the Au3Pt7 NPs.
FIGURE 3. (A,B) SEM images of 3D Co-MOF. (C) EDS mapping analysis of Au3Pt7/3D Co-MOF/GCE. (D,E) SEM images of Au3Pt7/2D Co-MOF/GCE. (F) EDS mapping analysis of Au3Pt7/2D Co-MOF/GCE. (G) CV curves of GCE, Au3Pt7/3D Co-MOF/GCE, and Au3Pt7/2D Co-MOF/GCE in 5.0 mM K3Fe (CN)6/K4Fe (CN)6 solution containing 0.1 M KCl (amplitude 10 mV, impedance spectral frequency 0.1∼105 Hz). (H) CV curves of GCE, 2D Co-MOF/GCE, Au3Pt7/3D Co-MOF/GCE, and Au3Pt7/2D Co-MOF/GCE in 100 mM PBS solution containing 10 mM H2O2 at a scan rate of 100 mV/s. (I) Histograms of GCE, 3D Co-MOF/GCE, 2D Co-MOF/GCE, Au3Pt7/GCE, Au3Pt7/3D Co-MOF/GCE, and Au3Pt7/2D Co-MOF/GCE in 0.1 M PBS containing 10 mM H2O2.
Operating in the solution of 5 mM K3Fe (CN)6/K4Fe (CN)6, in Figure 3G, after Au3Pt7 NPs depositing on two morphologies of pristine Co-MOF–modified electrodes, the gap between the cathodic and anodic peaks of the CV curves has been narrower, and the peak current of Au3Pt7 Co-MOF/GCE and Au3Pt7 2D Co-MOF/GCE was increased. Comparing with the conventional 3D Co-MOF, the 2D Co-MOF nanosheets possessed a larger surface area, smaller diffusion barrier, and more accessible active sites for the substrate molecules (Li et al., 2018). As shown in Figure 3H, compared with the peak current of the pristine 2D Co-MOF–modified electrode, the current signal of the Au3Pt7/Co-MOF/GCE prominent increased, indicating that the H2O2 can be catalyzed effectively by the presence of Au3Pt7 NPs. Preliminarily, the 2D Co-MOF with the better affinity for locating the Au3Pt7 NPs was inferred. Two-dimensional MOF nanosheets possess unique advantages, such as their large surface area can facilitate the contact of substrate molecules with the active sites on their surface with minimal diffusion barriers (Huang et al., 2017), thus improving their performance in catalysis and sensing applications (Wang et al., 2016). Figure 3I illustrates the peak current of 10 mM H2O2 to electrodes modified by different materials. The sequence of the peak current increases as follows: GCE < 3D Co-MOF/GCE < 2D Co-MOF/GCE < Au3Pt7/3D Co-MOF/GCE < Au3Pt7/GCE < Au3Pt7/2D Co-MOF/GCE. The sequence indicated the 2D Co-MOF as supporting materials combining the Au3Pt7 NPs as the compound nanozymes can help get the best electrocatalytic activity to reduce H2O2.
These results are mainly attributed by the 2D Co-MOF with a highly porous structure and more accessible electroactive sites for adsorbing more Au3Pt7 NPs than the 3D Co-MOF. On the basis of the Randled–Sevcik equation, the electroactive surface area of different modified electrodes was calculated Ip = (2.69 × 105) AD1/2n3/2γ1/2C, where Ip is the peak current (A); A refers to the effective surface area of the electrode (cm2), D means the diffusion coefficient for [Fe (CN)6]3-/4-(6.7 × 10−6 cm2s−1), n is the number of transition electrons of [Fe (CN)6]3-/4- (n = 1), γ means the scan rate (V/s), and C is the concentration of the redox reactant (5 × 10−6 mol cm−3). The effective surface area of Au3Pt7/2D Co-MOF/GCE was determined to be 0.143 cm2, which is nearly 1.5 times higher than that of bare GCE (0.09 cm2). However, the effective surface area of Au3Pt7/3D Co-MOF/GCE was determined to be 0.085 cm2, indicating the lousy conductivity of the 3D Co-MOF. Therefore, Au3Pt7/2D Co-MOF/GCE was selected to carry on the further electrocatalytic performance.
The sensitivity of Au3Pt7/2D Co-MOF/GCE was verified in 10 ml of PBS solution with different concentrations of H2O2 (2, 4, 6, 8, and 10 mM) (Supplementary Figure S4A). The reduction peak current increased following the increase of H2O2 concentrations from 0 to 10 mM. The electrochemical signal was plotted, and a good linear relationship was revealed in Supplementary Figure S4B. These consequences preliminarily illuminated that the fabricated electrode possessed a good response for H2O2 reduction. Supplementary Figure S5A displays the effect of scan rates and the charge transport behavior of electrode Au3Pt7/2D Co-MOF/GCE that have been investigated, with the help of the [Fe (CN)6]3−/4−redox probe. The CV curves revealed that both the catholic and anodic peak currents were enhanced with increasing scan rates from 50 to 700 mV/s. Furthermore, the current signal responses exhibited a proportional to the square root of the scan rates (Supplementary Figure S5B). The good linear relationship illustrated fast electron transfer kinetics with a typical diffusion-controlled process.
For meeting the sensitivity of demonstrating H2O2 concentration at the cellular level, the suitable detecting potential of the fabricated electrode Au3Pt7/2D Co-MOFs/GCE needs to be obtained. The amperometric responses were recorded in different potentials from −0.2 to −0.6 V. With the potential increase, the current responses were enhanced under successive injection of 0.4 mM H2O2, and the solution was stirred continuously at 250 rpm. The highest response showed in the potentials −0.6 V, but the signal noise of the background currents was too larger to be ignored. On the basis of the above results, −0.5 V was selected as the optimal working potential in the following determination (Supplementary Figure S6).
The amperometric measurement was employed to evaluate the detection sensitivity of Au3Pt7/2D Co-MOFs/GCE for the reduction of H2O2. With the successive injection of different H2O2 concentrations into 10 ml of PBS at the optimum potential of −0.5 V. Figure 4A describes the current response for the low concentration of H2O2. A typical amperometric plot was exhibited of injecting H2O2 per 50 s. The insets of Figure 4A show that the current response was achieved at 0.1 μM. The result means that the electrochemical sensor had a good sensitivity response for H2O2 at a low concentration. Figure 4B reveals the linear regions from 0.1 μM to 5 mM and the linear regression was I (μA) = −31.77C (mM) −0.16695 (R2 = 0.999) with the sensitivities of 236.1 μA mM−1 cm−2. The amperometric plot of 5–60 mM was shown in Figures 4C,D, discovering the liner regression of high concentration of H2O2, which was I (μA) = −24.98C (mM) −73.26 (R2 = 0.997) with the sensitivities of 174.6 μA mM−1 cm−2 (the sensitivities were calculated by dividing the slope of the linear regression equation by the geometric surface area of the bare GCE). In addition, the low detection limit (LOD) was calculated to be 0.02 μM (S/N = 3). The Au3Pt7/2D Co-MOF/GCE presented a good electrochemical catalytic activity for H2O2 with an extended linear range and a lower LOD.
FIGURE 4. (A) Amperometric responses of Au3Pt7/2D Co-MOF/GCE with the successive addition of low concentration of H2O2. (B) The linear relationships of the response current of Au3Pt7/2D Co-MOF/GCE with the H2O2 concentrations from 0.1 μM to 5 mM (R2 = 0.999) and (C) amperometric responses of Au3Pt7/2D Co-MOF/GCE with the successive addition of high concentration of H2O2. (D) The linear relationships of from 5 to 60 mM (R2 = 0.997). (E) Amperometric responses of 5 mM L-ascorbic acid, DL-alanine, ethanol, glucose, lactic acid, citric acid, lysine acid, KCl, NaCl, Na2SO4, and 0.5 mM H2O2 in PBS solution at an applied potential of −0.5 V. (F) Histograms of different substances.
The selectivity of Au3Pt7/2D Co-MOF/GCE for H2O2 was evaluated by injecting the relevant species successively. As shown in Figures 4E,F, there was no obvious current signal when following adding different substances, including 5 mM L-AA, 5 mM DL-alanine, 5 mM glucose, 5 mM lactic acid, 5 mM citric acid, 5 mM Lysine acid, and 5 mM KCl, NaCl, and Na2SO4. However, an apparent electrochemical signal was observed when a low concentration of H2O2 (0.5 μM) was added into the same solvent system. These results indicated that the H2O2 sensor possessed high selectivity for H2O2.
The stability of the H2O2 sensor was investigated. The peak current of CV curves was displayed in Supplementary Figures S7A,B after 7 days, the signal of peak currents only decreased to 93.3%, which presented the excellent stability of the Au3Pt7/2D Co-MOF/GCE. The same sensor recorded five successive CV measurements. The response current signals shown in Supplementary Figure S8 were almost unchanged with a relative standard deviation of 3.5%, which suggested the excellent repeatability of the electrode. The relevant electrode materials for the electrochemical sensing of H2O2 displayed in Table 1. The novel electrode Au3Pt7/2D Co-MOF/GCE exhibited an acceptable electrochemical catalytic activity for H2O2 with an extended linear range and a lower LOD.
Hela cells have been elected as the model cell to estimate weather the sensitivity of the novel electro-sensor Au3Pt7/Co-TCPP/GCE could meet the need of evaluating H2O2 secreted from actual samples. Before the electrochemical detecting, dehydrogenate (DHE) staining was applied for preliminary analyzing the intracellular H2O2 of Hela cells. As the fluorescence image depicting, the intracellular concentration of H2O2 was kept at a low level before AA stimulation (Figure 5A). After the treatment with AA (400 μM), the level of endogenous H2O2 concentration increased apparently (Figure 5B). The DHE fluorescence photographs proved that H2O2 of Hela cells indeed motivated by AA.
FIGURE 5. (A) DHE fluorescence image of Hela cells without stimulation by AA (400 μM). (B) Hela cells stimulated by AA (400 μM). (C) Current responses of Hela cells without and with AA (400 μM) stimulations at an applied potential of −0.5 V. (D) Bright images of Hela cells after electrochemical detection.
The amperometric technique was utilized to analyze H2O2 at the cellular level with the potential of −0.5 V. AA was injected into the dish as a stimulant to boost the H2O2 secreted from the Hela cells. After the injection of 400 μM AA solution into the system without cell, there was a negligible current response. However, an obvious electrochemical current signal was observed when the same drug injected into the system contained the Hela cells at the same concentration. The current value was calculated to be 204 nA, which corresponded to 0.12 μM H2O2 (Figure 5C). Bright images of Hela cells revealed that the modified electrodes and the electrochemical detection were harmless to the cells, and the morphology and viability of the living cells were similar to those without stimulating (Figure 5D). These results supported that Au3Pt7/2D Co-MOF/GCE could meet the need of the intracellular H2O2 in situ detection.
In conclusion, we have fabricated an enzyme-free electrochemical sensor for the real-time detection of H2O2 released from cells. We discovered that bimetallic Au3Pt7 NPs showed much higher electrocatalytic activity than pure Au NPs or Pt NPs toward H2O2 detection. Because of the ultrathin nanosheet structural features, 2D Co-MOF showed a better supporting ability than the 3D Co-MOF. Benefitting from the microplate structures of 2D Co-MOF and the high catalytic ability of Au3Pt7 NPs, we constructed a novel electrochemical biosensor on the basis of the Au3Pt7 NPs deposited on the 2D Co-MOF/GCE for the first time. The fabricated Au3Pt7/2D Co-MOF/GCE exhibited high electrocatalytic activity, fast response, and good sensitivity toward H2O2 reduction. The linear range of the Au3Pt7/2D Co-MOFs/GCE provided to H2O2 appealing in 0.1 μM–5 mM and 5–60 mM and exhibited a LOD of 0.02 μM (S/N = 3). These characters enable the real-time quantification of H2O2 secreted from Hela cancer cells under drug stimulation. This work provided a new method for improving the detection performance by changing the supporting materials of different crystalline morphologies. For future electrochemical sensors, more efforts will be made in cell adhesion/growth on the surface of the electrodes to get excellent sensitivity in situ detection of H2O2 or other disease biomarkers.
The original contributions presented in the study are included in the article/Supplementary Material, further inquiries can be directed to the corresponding authors.
This study was conceived and supervised by DS, XL, and LZ. Synthesis, structural characterization, and electrochemical measurements were performed by YX, XS, and LC. The analysis of all data was done by YX and XS. The original draft was written by YX. The manuscript was revised by DS, JL, and LZ. All authors contributed to the article and approved the submitted version.
This work was supported by the National Natural Science Foundation of China (82003710), the Natural Science Foundation of Guangdong Province (2020A1515010075), the Project of Educational Commission of Guangdong Province (2021ZDZX 2012), and the Foundation from Guangdong Traditional Medicine Bureau (20201194).
The authors declare that the research was conducted in the absence of any commercial or financial relationships that could be construed as a potential conflict of interest.
All claims expressed in this article are solely those of the authors and do not necessarily represent those of their affiliated organizations or those of the publisher, the editors, and the reviewers. Any product that may be evaluated in this article, or claim that may be made by its manufacturer, is not guaranteed or endorsed by the publisher.
We thank all members of laboratory for their technical support and academic discussions.
The Supplementary Material for this article can be found online at: https://www.frontiersin.org/articles/10.3389/fchem.2022.856003/full#supplementary-material
Bai, W., Li, S., Ma, J., Cao, W., and Zheng, J. (2019). Ultrathin 2D Metal-Organic Framework (Nanosheets and Nanofilms)-Based xD-2D Hybrid Nanostructures as Biomimetic Enzymes and Supercapacitors. J. Mater. Chem. A. 7 (15), 9086–9098. doi:10.1039/c9ta00311h
Batten, S. R., Champness, N. R., Chen, X.-M., Garcia-Martinez, J., Kitagawa, S., Öhrström, L., et al. (2013). Terminology of Metal-Organic Frameworks and Coordination Polymers (IUPAC Recommendations 2013). Pure Appl. Chem. 85 (8), 1715–1724. doi:10.1351/pac-rec-12-11-20
Dong, Y., Duan, C., Sheng, Q., and Zheng, J. (2019b). Preparation of Ag@zeolitic Imidazolate Framework-67 at Room Temperature for Electrochemical Sensing of Hydrogen Peroxide. Analyst 144 (2), 521–529. doi:10.1039/c8an01641k
Dong, Z., Yang, Z., Hao, Y., and Feng, L. (2019a). Fabrication of H2O2-Driven Nanoreactors for Innovative Cancer Treatments. Nanoscale 11 (35), 16164–16186. doi:10.1039/c9nr04418c
Furukawa, H., Cordova, K. E., O’Keeffe, M., and Yaghi, O. M. (2013). The Chemistry and Applications of Metal-Organic Frameworks. Science 341 (6149), 1230444. doi:10.1126/science.1230444
Gimeno, P., Bousquet, C., Lassu, N., Maggio, A.-F., Civade, C., Brenier, C., et al. (2015). High-performance Liquid Chromatography Method for the Determination of Hydrogen Peroxide Present or Released in Teeth Bleaching Kits and Hair Cosmetic Products. J. Pharm. Biomed. Anal. 107, 386–393. doi:10.1016/j.jpba.2015.01.018
Giorgio, M., Trinei, M., Migliaccio, E., and Pelicci, P. G. (2007). Hydrogen Peroxide: a Metabolic By-Product or a Common Mediator of Ageing Signals? Nat. Rev. Mol. Cel Biol. 8 (9), 722–728. doi:10.1038/nrm2240
Griendling, K. K. (2004). Novel NAD(P)H Oxidases in the Cardiovascular System. Heart 90 (5), 491–493. doi:10.1136/hrt.2003.029397
Hayyan, M., Hashim, M. A., and AlNashef, I. M. (2016). Superoxide Ion: Generation and Chemical Implications. Chem. Rev. 116 (5), 3029–3085. doi:10.1021/acs.chemrev.5b00407
Huang, Y., Zhao, M., Han, S., Lai, Z., Yang, J., Tan, C., et al. (2017). Growth of Au Nanoparticles on 2D Metalloporphyrinic Metal‐Organic Framework Nanosheets Used as Biomimetic Catalysts for Cascade Reactions. Adv. Mater. 29 (32), 1700102. doi:10.1002/adma.201700102
Iyyamperumal, R., Zhang, L., Henkelman, G., and Crooks, R. M. (2013). Efficient Electrocatalytic Oxidation of Formic Acid Using Au@Pt Dendrimer-Encapsulated Nanoparticles. J. Am. Chem. Soc. 135 (15), 5521–5524. doi:10.1021/ja4010305
Jorgenson, T. C., Zhong, W., and Oberley, T. D. (2013). Redox Imbalance and Biochemical Changes in Cancer. Cancer Res. 73 (20), 6118–6123. doi:10.1158/0008-5472.Can-13-1117
Li, S., Liu, X., Chai, H., and Huang, Y. (2018). Recent Advances in the Construction and Analytical Applications of Metal-Organic Frameworks-Based Nanozymes. Trac Trends Anal. Chem. 105, 391–403. doi:10.1016/j.trac.2018.06.001
Li, Y., Hou, L., Liu, Z., Lu, W., Zhao, M., Xiao, H., et al. (2020). A Sensitive Electrochemical MUC1 Sensing Platform Based on Electroactive Cu-MOFs Decorated by AuPt Nanoparticles. J. Electrochem. Soc. 167(7), 2158–2161. doi:10.1149/1945-7111/ab88b9
Li, Y., Lu, Q., Wu, S., Wang, L., and Shi, X. (2013). Hydrogen Peroxide Sensing Using Ultrathin Platinum-Coated Gold Nanoparticles with Core@shell Structure. Biosens. Bioelectron. 41, 576–581. doi:10.1016/j.bios.2012.09.027
Liu, C.-S., Li, J., and Pang, H. (2020). Metal-organic Framework-Based Materials as an Emerging Platform for Advanced Electrochemical Sensing. Coord. Chem. Rev. 410, 213222. doi:10.1016/j.ccr.2020.213222
Liu, Y., Zhang, Y., Hua, H., and Li, Y. (2017). Fabrication of Single Pt@Au Nanowire Electrodes for Monitoring Hydrogen Peroxide Released from Living Cells. RSC Adv. 7 (70), 44552–44558. doi:10.1039/c7ra08085a
Lu, J., Hu, Y., Wang, P., Liu, P., Chen, Z., and Sun, D. (2020). Electrochemical Biosensor Based on Gold Nanoflowers-Encapsulated Magnetic Metal-Organic Framework Nanozymes for Drug Evaluation with In-Situ Monitoring of H2O2 Released from H9C2 Cardiac Cells. Sensors Actuators B: Chem. 311, 127909. doi:10.1016/j.snb.2020.127909
Ma, J., Bai, W., and Zheng, J. (2019). Non-enzymatic Electrochemical Hydrogen Peroxide Sensing Using a Nanocomposite Prepared from Silver Nanoparticles and Copper (II)-porphyrin Derived Metal-Organic Framework Nanosheets. Microchim. Acta 186 (7), 482. doi:10.1007/s00604-019-3551-1
Ma, Y., Cen, Y., Sohail, M., Xu, G., Wei, F., Shi, M., et al. (2017). A Ratiometric Fluorescence Universal Platform Based on N, Cu Codoped Carbon Dots to Detect Metabolites Participating in H2O2-Generation Reactions. ACS Appl. Mater. Inter. 9 (38), 33011–33019. doi:10.1021/acsami.7b10548
Markesbery, W. R. (1997). Oxidative Stress Hypothesis in Alzheimer's Disease. Free Radic. Biol. Med. 23 (1), 134–147. doi:10.1016/s0891-5849(96)00629-6
Peng, Y., Yan, Z., Wu, Y., and Di, J. (2015). AgAuPt Nanocages for Highly Sensitive Detection of Hydrogen Peroxide. RSC Adv. 5 (11), 7854–7859. doi:10.1039/c4ra13653e
Ren, M., Deng, B., Wang, J.-Y., Kong, X., Liu, Z.-R., Zhou, K., et al. (2016). A Fast Responsive Two-Photon Fluorescent Probe for Imaging H2O2 in Lysosomes with a Large Turn-On Fluorescence Signal. Biosens. Bioelectron. 79, 237–243. doi:10.1016/j.bios.2015.12.046
Renner, F. U., Stierle, A., Dosch, H., Kolb, D. M., Lee, T.-L., and Zegenhagen, J. (2006). Initial Corrosion Observed on the Atomic Scale. Nature 439 (7077), 707–710. doi:10.1038/nature04465
Rhee, S. G., Kang, S. W., Jeong, W., Chang, T.-S., Yang, K.-S., and Woo, H. A. (2005). Intracellular Messenger Function of Hydrogen Peroxide and its Regulation by Peroxiredoxins. Curr. Opin. Cel Biol. 17 (2), 183–189. doi:10.1016/j.ceb.2005.02.004
Sun, D., Cai, C., Li, X., Xing, W., and Lu, T. (2004). Direct Electrochemistry and Bioelectrocatalysis of Horseradish Peroxidase Immobilized on Active Carbon. J. Electroanalytical Chem. 566 (2), 415–421. doi:10.1016/j.jelechem.2003.11.055
Sun, D., Yang, D., Wei, P., Liu, B., Chen, Z., Zhang, L., et al. (2020). One-step Electrodeposition of Silver Nanostructures on 2D/3D Metal-Organic Framework ZIF-67: Comparison and Application in Electrochemical Detection of Hydrogen Peroxide. ACS Appl. Mater. Inter. 12 (37), 41960–41968. doi:10.1021/acsami.0c11269
Sun, Y., Luo, M., Meng, X., Xiang, J., Wang, L., Ren, Q., et al. (2017). Graphene/Intermetallic PtPb Nanoplates Composites for Boosting Electrochemical Detection of H2O2 Released from Cells. Anal. Chem. 89 (6), 3761–3767. doi:10.1021/acs.analchem.7b00248
Tan, C., Cao, X., Wu, X.-J., He, Q., Yang, J., Zhang, X., et al. (2017). Recent Advances in Ultrathin Two-Dimensional Nanomaterials. Chem. Rev. 117 (9), 6225–6331. doi:10.1021/acs.chemrev.6b00558
Trachootham, D., Alexandre, J., and Huang, P. (2009). Targeting Cancer Cells by ROS-Mediated Mechanisms: a Radical Therapeutic Approach? Nat. Rev. Drug Discov. 8 (7), 579–591. doi:10.1038/nrd2803
Ushio-Fukai, M., Alexander, R. W., Akers, M., Yin, Q., Fujio, Y., Walsh, K., et al. (1999). Reactive Oxygen Species Mediate the Activation of Akt/protein Kinase B by Angiotensin II in Vascular Smooth Muscle Cells. J. Biol. Chem. 274 (32), 22699–22704. doi:10.1074/jbc.274.32.22699
Wang, H., Chen, W., Chen, Q., Liu, N., Cheng, H., and Li, T. (2021). Metal-organic Framework (MOF)-Au@Pt Nanoflowers Composite Material for Electrochemical Sensing of H2O2 in Living Cells. J. Electroanalytical Chem. 897, 115603. doi:10.1016/j.jelechem.2021.115603
Wang, N., Sun, Q., and Yu, J. (2019). Ultrasmall Metal Nanoparticles Confined within Crystalline Nanoporous Materials: a Fascinating Class of Nanocatalysts. Adv. Mater. 31 (1), 1803966. doi:10.1002/adma.201803966
Wang, Q., Wei, H., Zhang, Z., Wang, E., and Dong, S. (2018). Nanozyme: an Emerging Alternative to Natural Enzyme for Biosensing and Immunoassay. Trac Trends Anal. Chem. 105, 218–224. doi:10.1016/j.trac.2018.05.012
Wang, Y., Zhao, M., Ping, J., Chen, B., Cao, X., Huang, Y., et al. (2016). Bioinspired Design of Ultrathin 2D Bimetallic Metal-Organic-Framework Nanosheets Used as Biomimetic Enzymes. Adv. Mater. 28 (21), 4149–4155. doi:10.1002/adma.201600108
Wei, H., and Wang, E. (2013). Nanomaterials with Enzyme-like Characteristics (Nanozymes): Next-Generation Artificial Enzymes. Chem. Soc. Rev. 42 (14), 6060–6093. doi:10.1039/c3cs35486e
Wirtz, M., and Martin, C. R. (2003). Template-fabricated Gold Nanowires and Nanotubes. Adv. Mater. 15 (5), 455–458. doi:10.1002/adma.200390106
Wu, J., Wang, X., Wang, Q., Lou, Z., Li, S., Zhu, Y., et al. (2019). Nanomaterials with Enzyme-like Characteristics (Nanozymes): Next-Generation Artificial Enzymes (II). Chem. Soc. Rev. 48 (4), 1004–1076. doi:10.1039/c8cs00457a
Xie, J., and Huang, Y. (2011). Co3O4 Nanoparticles-Enhanced Luminol Chemiluminescence and its Application in H2O2 and Glucose Detection. Anal. Methods 3 (5), 1149–1155. doi:10.1039/c1ay05103b
Xu, R., Wang, Y., Duan, X., Lu, K., Micheroni, D., Hu, A., et al. (2016). Nanoscale Metal-Organic Frameworks for Ratiometric Oxygen Sensing in Live Cells. J. Am. Chem. Soc. 138 (7), 2158–2161. doi:10.1021/jacs.5b13458
Yang, J.-C., Lu, M.-C., Lee, C.-L., Chen, G.-Y., Lin, Y.-Y., Chang, F.-R., et al. (2011). Selective Targeting of Breast Cancer Cells through ROS-Mediated Mechanisms Potentiates the Lethality of Paclitaxel by a Novel Diterpene, Gelomulide K. Free Radic. Biol. Med. 51 (3), 641–657. doi:10.1016/j.freeradbiomed.2011.05.012
Yang, S., Han, G., Chen, Q., Yu, L., Wang, P., Zhang, Q., et al. (2021). Au-Pt Nanoparticle Formulation as a Radiosensitizer for Radiotherapy with Dual Effects. Ijn Vol. 16, 239–248. doi:10.2147/ijn.S287523
Yu, M., Zhao, K., Zhu, X., Tang, S., Nie, Z., Huang, Y., et al. (2017). Development of Near-Infrared Ratiometric Fluorescent Probe Based on Cationic Conjugated Polymer and CdTe/CdS QDs for Label-free Determination of Glucose in Human Body Fluids. Biosens. Bioelectron. 95, 41–47. doi:10.1016/j.bios.2017.03.065
Yu, Y., Yu, C., Niu, Y., Chen, J., Zhao, Y., Zhang, Y., et al. (2018). Target Triggered Cleavage Effect of DNAzyme: Relying on Pd-Pt Alloys Functionalized Fe-MOFs for Amplified Detection of Pb2+. Biosens. Bioelectron. 101, 297–303. doi:10.1016/j.bios.2017.10.006
Yuan, J., and Shiller, A. M. (1999). Determination of Subnanomolar Levels of Hydrogen Peroxide in Seawater by Reagent-Injection Chemiluminescence Detection. Anal. Chem. 71 (10), 1975–1980. doi:10.1021/ac981357c
Zhao, M., Huang, Y., Peng, Y., Huang, Z., Ma, Q., and Zhang, H. (2018a). Two-dimensional Metal-Organic Framework Nanosheets: Synthesis and Applications. Chem. Soc. Rev. 47 (16), 6267–6295. doi:10.1039/c8cs00268a
Zhao, M., Wang, Y., Ma, Q., Huang, Y., Zhang, X., Ping, J., et al. (2015). Ultrathin 2D Metal-Organic Framework Nanosheets. Adv. Mater. 27 (45), 7372–7378. doi:10.1002/adma.201503648
Zhao, W., Peng, J., Wang, W., Liu, S., Zhao, Q., and Huang, W. (2018b). Ultrathin Two-Dimensional Metal-Organic Framework Nanosheets for Functional Electronic Devices. Coord. Chem. Rev. 377, 44–63. doi:10.1016/j.ccr.2018.08.023
Zhou, J., Zhao, Y., Bao, J., Huo, D., Fa, H., Shen, X., et al. (2017). One-step Electrodeposition of Au-Pt Bimetallic Nanoparticles on MoS 2 Nanoflowers for Hydrogen Peroxide Enzyme-free Electrochemical Sensor. Electrochimica Acta 250, 152–158. doi:10.1016/j.electacta.2017.08.044
Keywords: electroanalysis, bimetallic nanoparticles, electrochemical sensor, hydrogen peroxide, cancer cells
Citation: Xie Y, Shi X, Chen L, Lu J, Lu X, Sun D and Zhang L (2022) Direct Electrodeposition of Bimetallic Nanostructures on Co-Based MOFs for Electrochemical Sensing of Hydrogen Peroxide. Front. Chem. 10:856003. doi: 10.3389/fchem.2022.856003
Received: 16 January 2022; Accepted: 09 February 2022;
Published: 11 March 2022.
Edited by:
Junjie Zhu, Nanjing University, ChinaCopyright © 2022 Xie, Shi, Chen, Lu, Lu, Sun and Zhang. This is an open-access article distributed under the terms of the Creative Commons Attribution License (CC BY). The use, distribution or reproduction in other forums is permitted, provided the original author(s) and the copyright owner(s) are credited and that the original publication in this journal is cited, in accordance with accepted academic practice. No use, distribution or reproduction is permitted which does not comply with these terms.
*Correspondence: Xiange Lu, bHhlXzAxMDNAc2luYS5jb20=; Duanping Sun, c3VuZHBAZ2RwdS5lZHUuY24=; Luyong Zhang, bHl6aGFuZ0BjcHUuZWR1LmNu
Disclaimer: All claims expressed in this article are solely those of the authors and do not necessarily represent those of their affiliated organizations, or those of the publisher, the editors and the reviewers. Any product that may be evaluated in this article or claim that may be made by its manufacturer is not guaranteed or endorsed by the publisher.
Research integrity at Frontiers
Learn more about the work of our research integrity team to safeguard the quality of each article we publish.