- Institute of Chemistry, University of São Paulo - USP, São Paulo, Brazil
Silver-gold nanoalloys were prepared from their metal salts precursors through bottom-up mechanochemical synthesis, using one-pot or galvanic replacement reaction strategies. The nanostructures were prepared over amorphous SiO2 as an inert supporting material, facilitating their stabilization without the use of any stabilizing agent. The nanomaterials were extensively characterized, confirming the formation of the bimetallic nanostructures. The nanoalloys were tested as catalysts in the hydrogenation of 2-nitroaniline and exhibited up to 4-fold the rate constant and up to 37% increased conversion compared to the respective single metal nanoparticles. Our approach is advantageous to produce nanoparticles with clean surfaces with available catalytic sites, directly in the solid-state and in an environmentally friendly manner.
1 Introduction
The interest in designing metal nanoparticles (NPs) is in constant increase, resulting from the exceptional properties of the nanostructures, which are relevant in catalysis (Araujo et al., 2019), energy (Califano et al., 2021), biomedicine (Li et al., 2017), and sensing (Jeon et al., 2016; Ma et al., 2020). Many advances have been made in tuning the properties of single metal NPs by controlling their size and shape. Nonetheless, a material containing a single element hardly satisfies all the requirements for a certain application. In catalysis field, for example, an ideal material should be highly active, selective, chemically, and structurally stable. Additionally, for industrial purposes the material should also be available at scale. These technological demands can be accomplished by the association of two or more elements into the final nanostructure. The combination of different metals in a single nanostructure is an opportunity to integrate the properties emerging from all individual contributors in a synergistic fashion (Gilroy et al., 2016).
Solution-based and thermal-annealing protocols have succeeded in the preparation of multimetallic structures containing two, eventually three elements (da Silva et al., 2016; Bhunia et al., 2018; Quiroz et al., 2018; Rodrigues et al., 2021). The solution-based techniques are, however, considerably sensitive to the reaction conditions, and are solvent- and/or energy-intensive. In addition, classical routes still face challenges for the preparation of nanostructures at larger scales (Paliwal et al., 2014). In the past decades, environmentally friendly syntheses have been developed in order to overcome these issues, including the use of bio-based reducing agents and alternative energy inputs (Iravani, 2011; Baláž et al., 2017; Ahmad et al., 2019; Jorge de Souza et al., 2019). In such contexts, mechanochemistry and mechanically-induced transformations in the solid-state (Boldyrev, 2006; Boldyreva, 2013) have gained prominence as an alternative to solvent-based protocols in the preparation of a diversity of chemicals (James et al., 2012) and materials (Xu et al., 2015; Muñoz-Batista et al., 2018). In fact, the use of mechanical energy to induce transformations in multimetallic systems through milling, the so-called mechanical alloying (Suryanarayana, 2001), is one of the its most explored applications. The early works were mostly dedicated to investigate the alloying of non-noble transition metals via top-down approaches, i.e., by milling the elemental powders (Suryanarayana, 2001).
More recently, noble metal nanoparticles have been more investigated under mechanochemical conditions, however, similarly to previous works, the studies have used the top-down methodology with elements such as Au, Ag and Pd to produce the final multicomponent nanomaterials (Delogu, 2008; Danielis et al., 2018; Schreyer et al., 2019; De Bellis et al., 2021). The greatest advantage offered by the technique is related to the simplicity of the treatment, dismissing any post-synthetic workup. On the other hand, top-down approaches are limited in terms of size and shape control. The alternative is to start from the metal salt precursors and construct the nanostructure by the chemical reduction or decomposition, known as bottom-up approach (Moores, 2018; de Oliveira et al., 2020b). The reduction or decomposition processes enable the control of reaction rate, nucleation and generation of growing species, leading to nanoparticles with tunable sizes and shape. Previous works have demonstrated that mechanochemical synthesis in ball milling devices is a promising strategy for the preparation of catalytically relevant noble single metal nanoparticles from their metal precursors (Debnath et al., 2009; Rak et al., 2014; De Oliveira et al., 2019; de Oliveira et al., 2020a; Baláž et al., 2021; Jin et al., 2021; Quiroz et al., 2021). On the other hand, few works associate more than one metal in the final structure totally through bottom-up mechanochemical synthesis. The examples include Pd-Ag nanoalloys (Ruiz-Ruiz et al., 2018, 2022), and Ag-Au nanoclusters (Murugadoss et al., 2012).
In the present report, we describe different approaches for the bottom-up mechanochemical synthesis of noble metal AgAu nanoalloys. Ag and Au were chosen due to their high miscibility and the possibility of a galvanic replacement according to the standard redox potentials of Ag and Au species. Additionally, Ag and Au NPs displays their localized surface plasmon resonance (LSPR) in the visible range, making the alloyed NPs suitable candidates for further studies as plasmonic materials (Liu et al., 2018). For the preparation of the nanoalloys, we adopted two strategies; 1) one-pot/co-reduction of Ag and Au metallic precursors and, 2) two-step galvanic replacement reaction (GRR), using Ag NPs as templates. The use of amorphous SiO2 as solid support enabled the stabilization of the nanoalloys in sizes smaller than 10 nm without using any surfactant or capping agent. The clean surfaces are an advantage for catalytic applications. Thus, the final nanoalloys were tested in the catalytic reduction of 2-nitroaniline (2-NA) to o-phenylenediamine (o-PDA), and exhibited superior activities compared to their monometallic Ag and Au NPs counterparts.
2 Materials and Methods
2.1 Chemicals
Flash silica (high-purity grade, Sigma-Aldrich), HAuCl4.3H2O (hydrogen tetrachloroaurate trihydrate, 48% in gold, Sigma-Aldrich), AgNO3 (silver nitrate, ≥99%, Sigma-Aldrich), C6H6N2O2 (2-nitroaniline, 98%, Sigma-Aldrich), C6H8N2 (o-phenylenediamine, ≥99%, Sigma-Aldrich), NaBH4 (sodium borohydride, powder, ≥98%, Sigma-Aldrich). All chemicals were analytical grade reagents. Deionized water (18.2 MΩ) was used throughout the experiments.
2.2 Instrumentation
Transmission electron microscopy (TEM) and high-resolution TEM (HRTEM) images were obtained using a JEOL JEM 2100 operated at 200 kV. A Talos F200S microscope (Thermo Scientific) operating at 200 kV was used for high-angle annular dark-field imaging scanning transmission electron microscopy (HAADF-STEM) and STEM-energy dispersive x-ray analysis (STEM-EDX). Samples were prepared by drop casting 5 μL from a well dispersed isopropanol suspension of each sample on a Formvar/Carbon Film coated, 200 Mesh, copper grid. UV-VIS and diffusive reflectance spectra (DRS) of the solid samples were obtained using a Shimadzu UV-2600 UV-VIS spectrophotometer. For catalytic experiments in water a quartz cuvette with an optical path of 1 cm was used. Powder X-ray diffraction (XRD) patterns were recorded in Shimadzu 7,000 Maxima diffractometer with Cu Kα radiation (λCuKα = 1.5418 Å for combined Kα1 and Kα2) in a Bragg-Brentano geometry. XPS analyses were performed with a UNI-SPECS UHV at pressure below 5·10–7 Pa (MgKα hν = 1,253.6 eV). A carbon tape was used as substrate to fix the samples. To determine the metal composition in the samples, inductively coupled plasma–optical emission spectrometry (ICP-OES) Spectro Arcos equipment was used. Aqua regia or HNO3 was used for the digestion of the samples. Then, a 10-fold dilution with distilled water was performed before each measurement. Further details can be found in the Supplementary Material.
2.3 Bottom-Up Mechanochemical Synthesis of Supported Metal Nanoparticles
2.3.1 Silver Nanoparticles–Ag/SiO2
Typically, Ag NPs/SiO2 were synthesized using AgNO3 (19.6 mg) as precursor and NaBH4 (19.6 mg) as reducing agent in presence on SiO2 (250 mg). The reactants and the support were placed in a PMMA jar containing one single ZrO2 milling ball (ø = 10 mm, m = 3.14 g) and milled at 50 Hz for 60 min in a vibratory ball-mill Pulversitte 23 Fritsch®. After the milling period the powder underwent several cycles of redispersion in water and centrifugation to eliminate the byproducts formed.
2.3.2 Gold Nanoparticles–Au/SiO2
Au/SiO2 nanoparticles were prepared similarly to Ag/SiO2. HAuCl4.3H2O (25 mg) was used as gold precursor and NaBH4 (25 mg) as reducing agent. SiO2 (250 mg) was utilized as solid support.
2.3.3 AgAu Nanoalloy - Galvanic Replacement Reaction
HAuCl4.3H2O (7.5 mg) and cleaned and dried AgNPs/SiO2 (250 mg) were added to the milling jar and milled for 60 min at 50 Hz. Note that no reducing agent was used and the Au3+ reduction to metallic gold was induced only by the galvanic replacement of Ag0 according to Eq. 1. To eliminate the byproduct, i.e., AgCl, the powder was dispersed in a supersaturated NaCl solution, to generate (AgClx)−(x−1) (x > 1) soluble species, and centrifuged. A final washing cycle was performed with water.
2.3.4 AgAu Nanoalloy - One-Pot Synthesis
The one-pot synthesis of AgAu NAs was performed by the simultaneously addition of AgNO3 (9.8 mg). HAuCl4.3H2O (12.5 mg) (Ag: Au ratio 1:1 m/m) and NaBH4 (22.3 mg) into the milling jar containing SiO2 (250 mg).
2.4 Catalytic Reduction of 2-Nitroaniline
Hydrogenation of 2-nitroaniline (0.75 μmol) were performed with NaBH4 (0.26 μmol) in triplicates in a quartz cuvette and monitored using a UV-Vis spectrophotometer ranging from 500 to 250 nm. The total load of metal used was 1.6 wt%, considering the catalyst/substrate ratio. (Details in the Supplementary Material).
3 Results and Discussion
3.1 Materials Synthesis and Characterization
The specific combination of Ag and Au as noble metals in bimetallic systems offers the opportunity to minimize the cost of Au catalysts and, at the same time, enhance the material catalytic performance through use synergistic effects (Slater et al., 2014). Typically, Ag and Au are combined either in core-shell or in alloys to applications in biosensors (Jia et al., 2014), SERS (Chen et al., 2013; Girão et al., 2017), catalysis (Wang et al., 2014; Kisukuri et al., 2016; Rodrigues et al., 2016) or electrocatalysis (Nazir et al., 2017; Balasubramanian et al., 2020; da Silva et al., 2021). They may be either blended in nanocrystals comprised of the individual elements or in an alloyed or intermetallic atomic ordering (Gilroy et al., 2016). Most of the experimental protocols requires capping agents to keep the colloidal nanoparticle dispersion stable. Capping agents, however, usually act as a physical barrier hindering the catalytic surface of nanoparticles from the access of reactants. In addition, they can act poisoning the catalytic sites (Niu and Li, 2014).
In the present work, two different approaches were used to synthesize AgAu bimetallic alloys supported over amorphous SiO2, without any addition of capping agents, as depicted in Figure 1. The first (Figure 1A) is a two-step reaction, where the first step led to the reduction of Ag NPs on amorphous SiO2 through the addition of Ag precursor and NaBH4 as the reducing agent, leading to Ag/SiO2. After washing steps to remove remaining NaBH4 and other byproducts, the Ag NPs acted as templates for the mechanochemical GRR. In this second step, due to the difference in the reduction potential of AuCl4− and Ag0 (Eq. (1)), the Ag atoms quickly oxidize with the addition of Au precursor salt, driving the formation of the nanoalloys, also supported on SiO2, named AgAu/SiO2 galvanic. The second strategy (Figure 1B) was based in a one-pot co-reduction, where both Ag and Au precursors salts were added simultaneously with NaBH4 as a reducing agent with SiO2 in a ball mill, leading to AgAu/SiO2 one-pot. Besides, monometallic Ag/SiO2 and Au/SiO2 nanostructures were prepared for the subsequent comparison of the catalytic activity. The metal content of the final materials was determined by ICP-OES (Supplementary Table S1) and the reaction yield for each metal for 1-h milling is given on Supplementary Table S2.
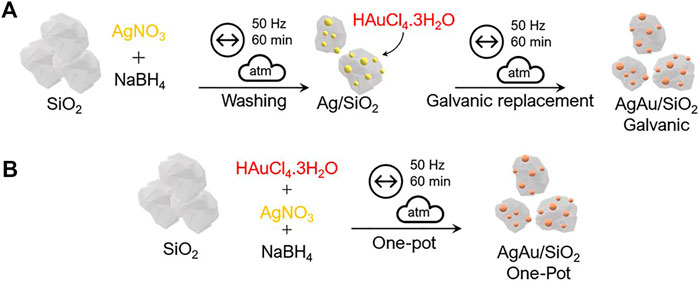
FIGURE 1. Scheme of the bottom-up mechanochemical synthesis routes for the preparation of AgAu/SiO2 galvanic (A) and AgAu/SiO2 one-pot (B) nanoalloys. The symbols over the arrows follow the recommendation of ref. (Michalchuk et al., 2021).
TEM micrographs of the synthesized samples are depicted in Figures 2A–D. TEM images show an even distribution of rounded nanoparticles throughout the supporting material. Particle-size distribution for each sample was determined through averaging the diameter of at least 150 nanoparticles, measured one-by-one (Supplementary Figure S1). Single metal Ag/SiO2 (Figure 2A) and Au/SiO2 (Figure 2B) present relatively polydisperse particle sizes of 5.9 ± 1.7 nm and 6.4 ± 3.3 nm, respectively. On the other hand, the generated bimetallic nanoalloys displayed a relatively narrow size distribution of 6.1 ± 1.7 nm for AgAu/SiO2 galvanic (Figure 2C) and 4.0 ± 1.0 nm for AgAu/SiO2 one-pot samples (Figure 2B). When comparing the size of the Ag NPs templates for GRR (5.9 nm) and the final bimettalic nanoalloys (6.1 nm), we see no significant difference, which suggests a constant rate of Ag0 oxidation and Au0 deposition. Interestingly, the one-pot route generated smaller nanoparticles (4.0 nm). We believe that this is probably due to the concurrent reactions that could take place: reduction of Ag+, reduction of AuCl4−, GRR of Ag0 by Au3+ and redeposition/reduction of displaced Ag+. Each one of these reactions can occur in different rates but, at the end, there are a number of nucleation events that hampers further particle growth. It was already demonstrated the bottom-up mechanochemical synthesis of NP may not follow the yet known solution chemistry for the construction of similar architectures, making difficult further discussions over the mechanism of formation of the bimetallic systems at this stage (de Oliveira et al., 2020a).
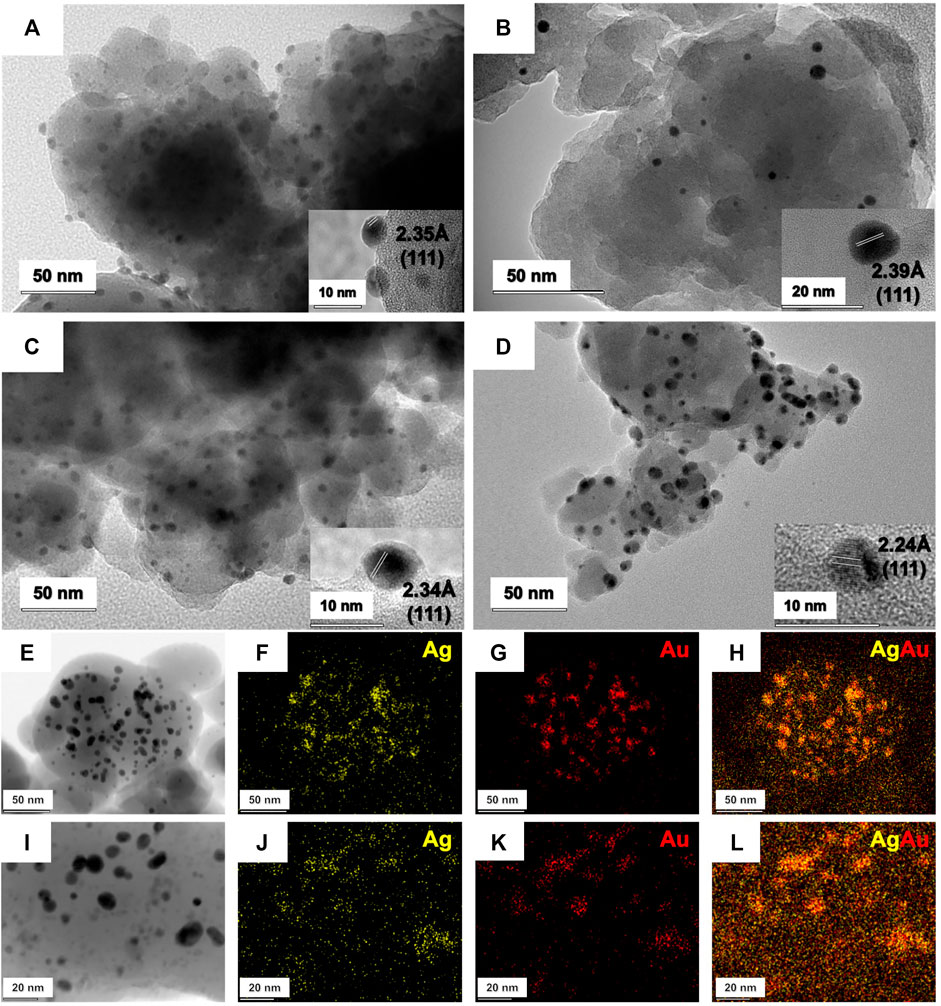
FIGURE 2. TEM, HRTEM and STEM of the mechanochemically prepared nanoparticles. TEM and HRTEM of AgNP (A), AuNP (B), AgAu/SiO2 galvanic (C) and AgAu/SiO2 one-pot (D). STEM of AgAu/SiO2 galvanic (E–H) and AgAu/SiO2 one-pot (I–L).
The insets of Figures 2A–D show HRTEM and the lattice spacing of the nanocrystals. All distances found were 2.35 Å (Ag/SiO2), 2.39 Å (Au/SiO2), 2.34 Å (AgAu/SiO2 galvanic) and 2.24 Å (AgAu/SiO2 one-pot), which are consistent with the (111) facet of metallic fcc Ag and Au (ICSD 44382 and 44362). STEM of Ag and Au in AgAu/SiO2 galvanic and AgAu/SiO2 one-pot are displayed in Figures 2E–H and Figures 2I–L, respectively. The images show the colocalization of Ag and Au in both nanostructures. This pattern is plausible due to the high miscibility among the two metals (Guisbiers et al., 2016). Supplementary Figure S2 shows HAADF-STEM of Ag, Au, Si and O for AgAu/SiO2 galvanic and AgAu/SiO2 one-pot.
UV-VIS diffusive reflectance spectra (DRS) spectra after Kubelka-Munk function of the synthesized materials are presented in Figure 3A. Both monometallic materials present a narrower localized surface plasmon resonance (LSPR) peak in comparison to the bimetallic alloys. LSPR peak referent to Ag/SiO2 has its maximum at 422 nm, whereas the maximum of Au/SiO2 is at 528 nm, corresponding to the values previously reported for mechanochemical syntheses of these metallic nanoparticles (De Oliveira et al., 2019; Baláž et al., 2021). The bimetallic samples present broader LSPR spectra, composed by overlapped LSPR contributions of shifted Ag and Au NPs and are liable to deconvolution. AgAu/SiO2 galvanic LSPR maximum is comprehended at 512 nm and a shoulder peak can be seen at 450 nm. For AgAu/SiO2 one-pot, these values are 452 and 501 nm, respectively. This indicates that although there is the formation of alloy through both GRR and one-pot strategies indicated by the redshift in both samples, some remaining monometallic particles could have been formed, as well as regions of higher density of single element within the bimetallic nanostructure. Accordingly, in solution-based synthesis alloyed AgAu nanoparticles present a LSPR broadening and a redshift, varying their maxima starting from the AgNP used as templates to the near infrared (Vongsavat et al., 2011; Gilroy et al., 2016; da Silva et al., 2021).
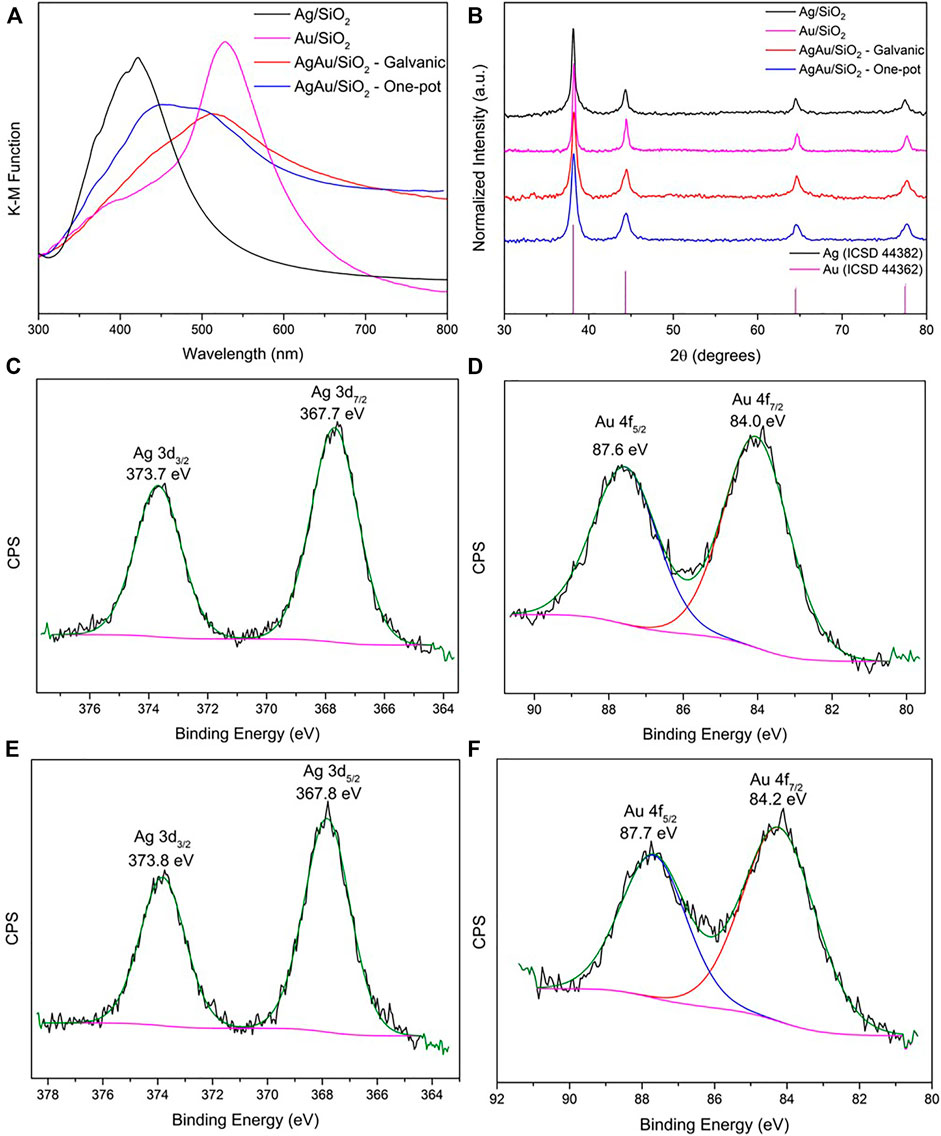
FIGURE 3. Characterization of the nanoparticles supported on SiO2. UV-VIS DRS spectra (A), XRD patterns (B) and XPS spectra of AgAu/SiO2 galvanic (C,D) and AgAu/SiO2 one-pot (E,F) for the Ag 3d and Au 4f core levels.
Regarding the PXRD data presented in Figure 3B, we see that all the samples are crystalline, however displaying a broad diffraction pattern, which indicates the presence of nanoparticles. No significant shifts in the PXRD reflections for the nanoalloys are noteworthy relative to the monometallic Ag/SiO2 and Au/SiO2 sanples. For example, the reflections relative to (111) crystalline planes represented in 2θ (λCuKα) for single Ag/SiO2 and Au/SiO2 are 2θ = 38.15° and 38.24°, respectively. Similarly, for bimetallic AgAu/SiO2 galvanic and AgAu/SiO2 one-pot samples this occurs at 2θ = 38.24° and 38.20°. This is because, both Ag and Au have the same fcc crystal structure and similar lattices constants (
To gain further insights in the structure and surface composition of the supported nanoparticles, the samples were also characterized by XPS (Figures 3C–F and Supplementary Figure S3). In the case of single metal Ag/SiO2 composite, the XPS spectrum shows two intense photoelectron peaks with binding energies (BE) at the maxima in 368.1 and 374.1 eV, ascribed to Ag 3d5/2 and 3d3/2 doublet, respectively (Supplementary Figure S3) (Liu et al., 1994). These BE values and the splitting value between them (6.0 eV) are characteristics of metallic Ag, indicating the efficiency of the mechanochemical route in the reduction of the Ag+, and that the final material is stable on the support, not undergoing any oxidation when stored in room conditions. Similarly, the XPS spectrum of single metal Au/SiO2 demonstrates the formation of metallic Au according to the Au 4f7/2 and 4f5/2 doublet with BE of 84.1 and 87.6 eV, respectively and a splitting value of 3.5 eV (Supplementary Figure S3) (Mansour, 1994).
Concerning the XPS spectra of the nanoalloys (Figures 3C–F), they demonstrate the metallic character of both metals present in the sample. However, small shifts in the BE can be considered when compared to the respective single metal NPs. In the XPS spectra of AgAu/SiO2 galvanic sample (Figure 3C), the peaks related to the Ag 3d5/2 and 3d3/2 spin orbit coupling doublet, shifts in −0.4 eV while for AgAu/SiO2 one-pot sample (Figure 3E) this shift is around −0.3 eV. The shifted BE to lower values is consistent with the formation of a Ag alloyed structure which is, in the present case, with Au (Tyson et al., 1992; Sun et al., 2018). For the BE values of Au 4f7/2 and 4f5/2 doublet in the samples of alloyed NPs (Figures 3D,F), the shifts compared to Au in the Au/SiO2 sample are meaningless considering the precision of ±0.1 eV in determining the BE.
3.2 Catalytic Reduction of 2-Nitroaniline
The successful mechanochemical preparation of bimetallic AgAu nanoalloys, without using any stabilizing surface agent, make them suitable materials for catalytic applications. We employed the catalytic reduction of 2-nitroaniline (2-NA) to probe the catalytic activity of the nanostructures. Nitrobenzenes’ reductions are largely investigated in order to assess the catalytic activity of noble metal nanomaterials due to the simplicity in extracting kinetic data from spectroscopic measurements (Le et al., 2014; Hajfathalian et al., 2015; Menumerov et al., 2016; Naseem et al., 2017; Saire-Saire et al., 2019). In our case, we used 2-NA, which is an environmental pollutant and is the by-product of several anthropogenic chemical processes, such as the production of dyes, explosives and pharmaceutical products (Naseem et al., 2017). The product of its catalytic reduction is the ortho-phenylenediamine (o-PDA), a precursor for the synthesis of antioxidants, polymers, surfactants, and for products of food and fine chemistry industries. Therefore, the conversion of 2-NA into o-PDA is an environmental solution and a chemical opportunity.
The scheme of the catalytic reduction of 2-NA by BH4− is illustrated in Figure 4A. The same amount of total metal load was used in each catalytic run of the different materials. The metal load was determined by ICP-OES (Supplementary Table S1). The conversion of 2-NA was followed by UV-VIS spectroscopy through the decay of 412 nm band, as demonstrated by Supplementary Figure S4. A shift in the 283—288 nm range was also observed throughout the catalytic reaction, and it is assigned to the formation of o-PDA (Supplementary Figure S5). As the catalytic reaction progresses, the yellowish starting solution characteristic of 2-NA becomes transparent with its conversion to o-PDA. Figure 4B show the triplicate of conversion curves for the different mechanochemically synthesized catalytic materials. The first points of these curves represent the induction period necessary for restructuring the surface of the catalyst with the substrate, just as observed for others nitrobenzenes (Hervés et al., 2012). This induction period plays a major role for Ag/SiO2, slowing up the 412 nm band decay for this material in the first minutes, whereas Au/SiO2 and both alloys show a shorter induction period. For the same period of reaction (∼25 min), the conversions were around 60% for both Ag/SiO2 and Au/SiO2. These values are much lower in comparison to the nanoalloys, which reached 2-NA conversions of 87 and 97%, for AgAu/SiO2 galvanic and AgAu/SiO2 one-pot, respectively.
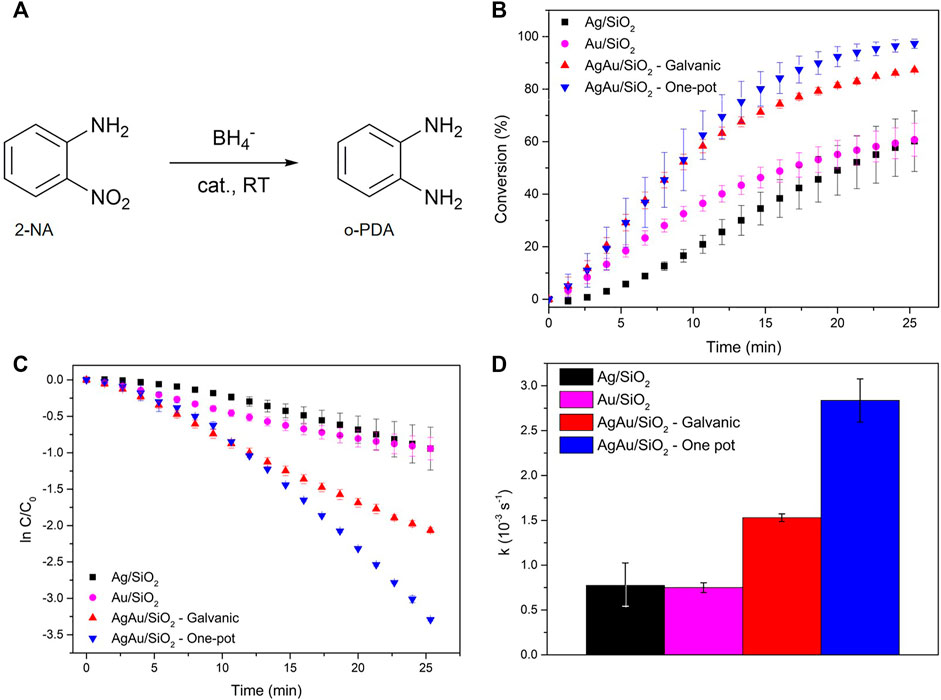
FIGURE 4. Catalytic reduction of 2-NA. Reaction scheme of 2-NA reduction to o-PDA (A). Reduction of 2-NA analyzed by the decay of 412 nm band (B). Linearization considering a pseudo first-order reaction (C). Reaction pseudo first-order rate constant values obtained from C (D).
The apparent reaction rate constants (k) were obtained after linearization of the conversion data as presented in Figure 4C, considering a typical pseudo-first order reaction (high excess of NaBH4) (Figure 4D). The induction periods were not considered for this calculation since they do not follow a linear trend. Similar apparent rate constants were shown by Ag/SiO2 (kAg = 7.75 10–4 s−1) and Au/SiO2 (kAu = 7.50 10–4 s−1), values that are consistent with previously reported studies for monometallic Ag and Au nanomaterials in the catalytic reduction of 2-NA (Nalawade et al., 2013; Dong et al., 2014). However, AgAu/SiO2 galvanic and AgAu/SiO2 one-pot showed twice and four-fold the rate constant values of kGalvanic = 1.53 10–3 s−1 and kone-pot = 2.84 10–3 s−1, respectively.
It is worth discussing the better performance shown by the AgAu/SiO2 one-pot material. One can suggest that the composition, i.e., the Ag/Au ratio, can affect the catalytic activity. The metal content determined by ICP-OES (Supplementary Table S1) indicates that AgAu/SiO2 galvanic is composed by 58 wt% of Ag and 42 wt% of Au, whereas the one-pot sample consists of 36 wt% of Ag and 64 wt% of Au. However, the similar activities for single metal NPs indicate no significant effect of the metal in our experimental conditions. Another possibility for such difference of the catalytic activity between the nanoalloys could be the surface composition, as the studied catalytic reaction strongly depends on the surface chemistry. The surface composition estimated by XPS (Supplementary Table S3) shows the same fraction of Ag (62 wt%) and Au (38 wt%) for both galvanic and one-pot prepared nanostructures. Thus, this possibility solely can also be dismissed. A final aspect that must be considered is simply the difference of particle size. AgAu/SiO2 one-pot material presents smaller particles (4.0 nm) compared to the material prepared by the galvanic route (6.1 nm). It is of wide knowledge that smaller sizes are related to higher specific surface areas, which can be available for catalysis, and consequently increase the reaction rate.
Conversion, induction period and reaction rate constant strongly suggest that the bimetallic nanoalloys benefit from the synergy between silver and gold, just as previously reported (Wang et al., 2014; Kisukuri et al., 2016; da Silva et al., 2021). Although many examples of enhanced catalytic properties of bimetallic systems have been reported, the synergistic effect between metals in these systems is yet to be fully comprehended. Among reaction conditions such as pH, temperature and diffusion rates, catalytic rate constants are related to adsorption energy of reactants on active sites. In its turn, adsorption energy is dependent on the crystallite size, facets and composition, properties that are susceptible of changes by the formation of alloys in bimetallic systems. Besides, the rearranged atomic ordering admitted by alloys may change the surface electronic structure of the nanoparticles and, thus, affect their catalytic properties (Sha et al., 2019).
4 Conclusion
Bimetallic nanoparticles offer the possibility to combine the different properties in a single structure. We demonstrated different possibilities to prepare crystalline AgAu nanoalloys through bottom-up mechanochemical synthesis. Both galvanic and one-pot/co-reduction strategies led to the formation of nanoalloys with diameters of 6.1 ± 1.7 nm and 4.0 ± 1.0 nm, respectively. STEM-EDX images and XPS confirmed the formation of these alloys. The LSPR in the UV-VIS DRS, however, demonstrated that some monometallic NPs or regions of higher density of a single element can also be formed. This shows that further investigation into elementary steps of the nanostructure formation is necessary if one aims an effective control of the final materials, and therefore, their properties. Our results reinforces that we need to understand the specific rules for solid-state mechanochemical synthesis of nanomaterial from their respective precursors. This includes the different rates of reactions, and therefore, the nucleation and the formation of feeding species to the growing sites.
Additionally, in our work, the mechanochemical route was able to generate materials with efficient distribution over the support. Besides, the direct synthesis over SiO2 dismisses the use of capping agents and eliminate impregnation steps. The catalytic activity of our AgAu/SiO2 nanoalloys were tested in the reduction of 2-NA. The alloyed nanoparticles displayed higher catalytic activity in terms of reaction rate constants (kGalvanic = 1.53 10–3 s−1 and kone-pot = 2.84 10–3 s−1) and conversion (87 and 97%, respectively) when compared to the single metal NPs (kAg = 7.75 10–4 s−1 and kAu = 7.50 10–4 s−1 with 60% conversion for both materials). The higher reaction rate and conversion were obtained for the AgAu/SiO2 one-pot material, showing the dominant effect of the particle size. It still worth mentioning that the LSPR displayed by the nanoalloys are very interesting for further studies on light-enhanced plasmonic catalysis.
The mechanochemical route for the preparation of multicomponent catalysts with active surfaces is highly envisioned to improve catalytic activity and minimize costly processes. This also includes the possibility of scaling up the process to meet industrial requirements, in addition of being a powerful tool for a sustainable design of new materials.
Data Availability Statement
The raw data supporting the conclusion of this article will be made available by the authors, without undue reservation.
Author Contributions
RTPS and PFMO conceived the project. RTPS performed the catalytic assays and wrote the manuscript. PFMO developed the synthetic approaches, synthesized the materials, and wrote the manuscript. PFMO and SICT supervised the work.
Funding
This work was supported by FAPESP (Grants 2015/26308–7 and 2018/16219–5). RTPS was funded by a PhD fellowship from CAPES (Grant 88882.328241/2019–01). PFMO thanks FAPESP for the Grants 2020/14955-6 and 2021/12899-4.
Conflict of Interest
The authors declare that the research was conducted in the absence of any commercial or financial relationships that could be construed as a potential conflict of interest.
Publisher’s Note
All claims expressed in this article are solely those of the authors and do not necessarily represent those of their affiliated organizations, or those of the publisher, the editors and the reviewers. Any product that may be evaluated in this article, or claim that may be made by its manufacturer, is not guaranteed or endorsed by the publisher.
Acknowledgments
The authors thank the work of C. Prinz, M. Heilmann and F. Emmerling from the Federal Institute for Materials Research and Testing (BAM–Berlin) for HRTEM and STEM images and Peter Hammer from LEFE, Physical Chemistry department, IQ-UNESP for XPS data and analysis.
Supplementary Material
The Supplementary Material for this article can be found online at: https://www.frontiersin.org/articles/10.3389/fchem.2022.836597/full#supplementary-material
References
Ahmad, S., Munir, S., Zeb, N., Ullah, A., Khan, B., Ali, J., et al. (2019). Green Nanotechnology: a Review on green Synthesis of Silver Nanoparticles - an Ecofriendly Approach. Ijn 14, 5087–5107. doi:10.2147/IJN.S200254
Araujo, T. P., Quiroz, J., Barbosa, E. C. M., and Camargo, P. H. C. (2019). Understanding Plasmonic Catalysis with Controlled Nanomaterials Based on Catalytic and Plasmonic Metals. Curr. Opin. Colloid Interf. Sci. 39, 110–122. doi:10.1016/j.cocis.2019.01.014
Balasubramanian, P., He, S.-B., Jansirani, A., Peng, H.-P., Huang, L.-L., Deng, H.-H., et al. (2020). Bimetallic AgAu Decorated MWCNTs Enable Robust Nonenzyme Electrochemical Sensors for In-Situ Quantification of Dopamine and H2O2 Biomarkers Expelled from PC-12 Cells. J. Electroanalytical Chem. 878, 114554. doi:10.1016/j.jelechem.2020.114554
Baláž, M., Bedlovičová, Z., Daneu, N., Siksa, P., Sokoli, L., Tkáčiková, Ľ., et al. (2021). Mechanochemistry as an Alternative Method of green Synthesis of Silver Nanoparticles with Antibacterial Activity: A Comparative Study. Nanomaterials 11, 1139. doi:10.3390/nano11051139
Baláž, M., Daneu, N., Balážová, Ľ., Dutková, E., Tkáčiková, Ľ., Briančin, J., et al. (2017). Bio-mechanochemical Synthesis of Silver Nanoparticles with Antibacterial Activity. Adv. Powder Technol. 28, 3307–3312. doi:10.1016/j.apt.2017.09.028
Banin, U., Waiskopf, N., Hammarström, L., Boschloo, G., Freitag, M., Johansson, E. M. J., et al. (2021). Nanotechnology for Catalysis and Solar Energy Conversion. Nanotechnology 32 (4), 042003. doi:10.1088/1361-6528/abbce8
Bhunia, K., Khilari, S., and Pradhan, D. (2018). Monodispersed PtPdNi Trimetallic Nanoparticles-Integrated Reduced Graphene Oxide Hybrid Platform for Direct Alcohol Fuel Cell. ACS Sustain. Chem. Eng. 6, 7769–7778. doi:10.1021/acssuschemeng.8b00721
Boldyrev, V. V. (2006). Mechanochemistry and Mechanical Activation of Solids. Russ. Chem. Rev. 75, 177–189. doi:10.1070/RC2006v075n03ABEH001205
Boldyreva, E. (2013). Mechanochemistry of Inorganic and Organic Systems: what Is Similar, what Is Different? Chem. Soc. Rev. 42, 7719–7738. doi:10.1039/c3cs60052a
Chen, L., Chabu, J. M., and Liu, Y. (2013). Bimetallic AgM (M = Pt, Pd, Au) Nanostructures: Synthesis and Applications for Surface-Enhanced Raman Scattering. RSC Adv. 3, 4391–4399. doi:10.1039/c3ra23137b
da Silva, A. G. M., Rodrigues, T. S., Haigh, S. J., and Camargo, P. H. C. (2017). Galvanic Replacement Reaction: Recent Developments for Engineering Metal Nanostructures towards Catalytic Applications. Chem. Commun. 53, 7135–7148. doi:10.1039/C7CC02352A
da Silva, R. T. P., de Souza Rodrigues, M. P., Davilla, G. F. B., da Silva, A. M. R. P., Dourado, A. H. B., and Córdoba de Torresi, S. I. (2021). AgAu Hollow Nanoshells on Layered Graphene Oxide and Silica Submicrospheres as Plasmonic Nanozymes for Light-Enhanced Electrochemical H2O2 Sensing. ACS Appl. Nano Mater. 4, 12062–12072. doi:10.1021/acsanm.1c02611
Danielis, M., Colussi, S., de Leitenburg, C., Soler, L., Llorca, J., and Trovarelli, A. (2018). Outstanding Methane Oxidation Performance of Palladium-Embedded Ceria Catalysts Prepared by a One-step Dry Ball-Milling Method. Angew. Chem. Int. Ed. 57, 10212–10216. doi:10.1002/anie.201805929
da Silva, A. G. M., Rodrigues, T. S., Correia, V. G., Alves, T. V., Alves, R. S., Ando, R. A., et al. (2016). Plasmonic Nanorattles as Next-Generation Catalysts for Surface Plasmon Resonance-Mediated Oxidations Promoted by Activated Oxygen. Angew. Chem. Int. Ed. 55, 7111–7115. doi:10.1002/anie.201601740
De Bellis, J., Felderhoff, M., and Schüth, F. (2021). Mechanochemical Synthesis of Supported Bimetallic Catalysts. Chem. Mater. 33, 2037–2045. doi:10.1021/acs.chemmater.0c04134
de Oliveira, P. F. M., Michalchuk, A. A. L., Marquardt, J., Feiler, T., Prinz, C., Torresi, R. M., et al. (2020a). Investigating the Role of Reducing Agents on Mechanosynthesis of Au Nanoparticles. CrystEngComm 22, 6261–6267. doi:10.1039/D0CE00826E
De Oliveira, P. F. M., Quiroz, J., De Oliveira, D. C., and Camargo, P. H. C. (2019). A Mechano-Colloidal Approach for the Controlled Synthesis of Metal Nanoparticles. Chem. Commun. 55, 14267–14270. doi:10.1039/c9cc06199a
de Oliveira, P. F. M., Torresi, R. M., Emmerling, F., and Camargo, P. H. C. (2020b). Challenges and Opportunities in the Bottom-Up Mechanochemical Synthesis of noble Metal Nanoparticles. J. Mater. Chem. A. 8, 16114–16141. doi:10.1039/D0TA05183G
Debnath, D., Kim, S. H., and Geckeler, K. E. (2009). The First Solid-phase Route to Fabricate and Size-Tune Gold Nanoparticles at Room Temperature. J. Mater. Chem. 19, 8810. doi:10.1039/b905260g
Delogu, F. (2008). A Mechanistic Study of Ag50Cu50 Solid Solution Formation by Mechanical Alloying. Acta Materialia 56, 2344–2352. doi:10.1016/j.actamat.2008.01.024
Dong, Z., Le, X., Li, X., Zhang, W., Dong, C., and Ma, J. (2014). Silver Nanoparticles Immobilized on Fibrous Nano-Silica as Highly Efficient and Recyclable Heterogeneous Catalyst for Reduction of 4-nitrophenol and 2-nitroaniline. Appl. Catal. B: Environ. 158-159, 129–135. doi:10.1016/j.apcatb.2014.04.015
Gilroy, K. D., Ruditskiy, A., Peng, H.-C., Qin, D., and Xia, Y. (2016). Bimetallic Nanocrystals: Syntheses, Properties, and Applications. Chem. Rev. 116, 10414–10472. doi:10.1021/acs.chemrev.6b00211
Girão, A. V., Pinheiro, P. C., Ferro, M., and Trindade, T. (2017). Tailoring Gold and Silver Colloidal Bimetallic Nanoalloys towards SERS Detection of Rhodamine 6G. RSC Adv. 7, 15944–15951. doi:10.1039/c7ra00685c
Guisbiers, G., Mendoza-Cruz, R., Bazán-Díaz, L., Velázquez-Salazar, J. J., Mendoza-Perez, R., Robledo-Torres, J. A., et al. (2016). Electrum, the Gold-Silver alloy, from the Bulk Scale to the Nanoscale: Synthesis, Properties, and Segregation Rules. ACS Nano 10, 188–198. doi:10.1021/acsnano.5b05755
Hajfathalian, M., Gilroy, K. D., Yaghoubzade, A., Sundar, A., Tan, T., Hughes, R. A., et al. (2015). Photocatalytic Enhancements to the Reduction of 4-Nitrophenol by Resonantly Excited Triangular Gold-Copper Nanostructures. J. Phys. Chem. C 119, 17308–17315. doi:10.1021/acs.jpcc.5b04618
Hervés, P., Pérez-Lorenzo, M., Liz-Marzán, L. M., Dzubiella, J., Lu, Y., and Ballauff, M. (2012). Catalysis by Metallic Nanoparticles in Aqueous Solution: Model Reactions. Chem. Soc. Rev. 41, 5577–5587. doi:10.1039/c2cs35029g
Iravani, S. (2011). Green Synthesis of Metal Nanoparticles Using Plants. Green. Chem. 13, 2638–2650. doi:10.1039/c1gc15386b
James, S. L., Adams, C. J., Bolm, C., Braga, D., Collier, P., Friščić, T., et al. (2012). Mechanochemistry: Opportunities for New and Cleaner Synthesis. Chem. Soc. Rev. 41, 413–447. doi:10.1039/C1CS15171A
Jeon, T. Y., Kim, D. J., Park, S.-G., Kim, S.-H., and Kim, D.-H. (2016). Nanostructured Plasmonic Substrates for Use as SERS Sensors. Nano Convergence 3. doi:10.1186/s40580-016-0078-6
Jia, K., Khaywah, M. Y., Li, Y., Bijeon, J. L., Adam, P. M., Déturche, R., et al. (2014). Strong Improvements of Localized Surface Plasmon Resonance Sensitivity by Using Au/Ag Bimetallic Nanostructures Modified with Polydopamine Films. ACS Appl. Mater. Inter. 6, 219–227. doi:10.1021/am403943q
Jin, T., Liu, X., Su, Y.-Q., Pan, F., Han, X., Zhu, H., et al. (2021). Mesoporous Carbon-Supported Ultrasmall Metal Nanoparticles via a Mechanochemical-Driven Redox Reaction: A "Two-In-One" Strategy. Appl. Catal. B: Environ. 294, 120232. doi:10.1016/j.apcatb.2021.120232
Jorge de Souza, T. A., Rosa Souza, L. R., and Franchi, L. P. (2019). Silver Nanoparticles: An Integrated View of green Synthesis Methods, Transformation in the Environment, and Toxicity. Ecotoxicology Environ. Saf. 171, 691–700. doi:10.1016/j.ecoenv.2018.12.095
Kisukuri, C. M., Palmeira, D. J., Rodrigues, T. S., Camargo, P. H. C., and Andrade, L. H. (2016). Bimetallic Nanoshells as Platforms for Metallo- and Biometallo-Catalytic Applications. ChemCatChem 8, 171–179. doi:10.1002/cctc.201500812
Le, X., Dong, Z., Zhang, W., Li, X., and Ma, J. (2014). Fibrous Nano-Silica Containing Immobilized Ni@Au Core-Shell Nanoparticles: A Highly Active and Reusable Catalyst for the Reduction of 4-nitrophenol and 2-nitroaniline. J. Mol. Catal. A: Chem. 395, 58–65. doi:10.1016/j.molcata.2014.08.002
Li, F., Lu, J., Kong, X., Hyeon, T., and Ling, D. (2017). Dynamic Nanoparticle Assemblies for Biomedical Applications. Adv. Mater. 29, 1605897. doi:10.1002/adma.201605897
Liu, J., He, H., Xiao, D., Yin, S., Ji, W., Jiang, S., et al. (2018). Recent Advances of Plasmonic Nanoparticles and Their Applications. Materials 11, 1833. doi:10.3390/ma11101833
Liu, Y., Jordan, R. G., and Qiu, S. L. (1994). Electronic Structures of Ordered Ag-Mg Alloys. Phys. Rev. B 49, 4478–4484. doi:10.1103/PhysRevB.49.4478
Ma, J., Liu, X., Wang, R., Zhang, J., Jiang, P., Wang, Y., et al. (2020). Bimetallic Core-Shell Nanostars with Tunable Surface Plasmon Resonance for Surface-Enhanced Raman Scattering. ACS Appl. Nano Mater. 3, 10885–10894. doi:10.1021/acsanm.0c02144
Mansour, A. N. (1994). Gold Mg Kα XPS Spectra from the Physical Electronics Model 5400 Spectrometer. Surf. Sci. Spectra 3, 197–201. doi:10.1116/1.1247747
Menumerov, E., Hughes, R. A., and Neretina, S. (2016). Catalytic Reduction of 4-Nitrophenol: A Quantitative Assessment of the Role of Dissolved Oxygen in Determining the Induction Time. Nano Lett. 16, 7791–7797. doi:10.1021/acs.nanolett.6b03991
Michalchuk, A. A. L., Boldyreva, E. V., Belenguer, A. M., Emmerling, F., and Boldyrev, V. V. (2021). Tribochemistry, Mechanical Alloying, Mechanochemistry: What Is in a Name? Front. Chem. 9, 1–29. doi:10.3389/fchem.2021.685789
Moores, A. (2018). Bottom up, Solid-phase Syntheses of Inorganic Nanomaterials by Mechanochemistry and Aging. Curr. Opin. Green Sustain. Chem. 12, 33–37. doi:10.1016/j.cogsc.2018.05.004
Muñoz-Batista, M. J., Rodriguez-Padron, D., Puente-Santiago, A. R., and Luque, R. (2018). Mechanochemistry: Toward Sustainable Design of Advanced Nanomaterials for Electrochemical Energy Storage and Catalytic Applications. ACS Sustain. Chem. Eng. 6, 9530–9544. doi:10.1021/acssuschemeng.8b01716
Murugadoss, A., Kai, N., and Sakurai, H. (2012). Synthesis of Bimetallic Gold-Silver alloy Nanoclusters by Simple Mortar Grinding. Nanoscale 4, 1280. doi:10.1039/c2nr11727d
Nalawade, P., Mukherjee, T., and Kapoor, S. (2013). Green Synthesis of Gold Nanoparticles Using Glycerol as a Reducing Agent. Anp 02, 78–86. doi:10.4236/anp.2013.22014
Naseem, K., Begum, R., and Farooqi, Z. H. (2017). Catalytic Reduction of 2-nitroaniline: a Review. Environ. Sci. Pollut. Res. 24, 6446–6460. doi:10.1007/s11356-016-8317-2
Nazir, R., Fageria, P., Basu, M., and Pande, S. (2017). Decoration of Carbon Nitride Surface with Bimetallic Nanoparticles (Ag/Pt, Ag/Pd, and Ag/Au) via Galvanic Exchange for Hydrogen Evolution Reaction. J. Phys. Chem. C 121, 19548–19558. doi:10.1021/acs.jpcc.7b04595
Niu, Z., and Li, Y. (2014). Removal and Utilization of Capping Agents in Nanocatalysis. Chem. Mater. 26, 72–83. doi:10.1021/cm4022479
Paliwal, R., Babu, R. J., and Palakurthi, S. (2014). Nanomedicine Scale-Up Technologies: Feasibilities and Challenges. AAPS PharmSciTech 15, 1527–1534. doi:10.1208/s12249-014-0177-9
Quiroz, J., Barbosa, E. C. M., Araujo, T. P., Fiorio, J. L., Wang, Y.-C., Zou, Y.-C., et al. (2018). Controlling Reaction Selectivity over Hybrid Plasmonic Nanocatalysts. Nano Lett. 18, 7289–7297. doi:10.1021/acs.nanolett.8b03499
Quiroz, J., de Oliveira, P. F. M., Shetty, S., Oropeza, F. E., de la Peña O’Shea, V. A., Rodrigues, L. C. V., et al. (2021). Bringing Earth-Abundant Plasmonic Catalysis to Light: Gram-Scale Mechanochemical Synthesis and Tuning of Activity by Dual Excitation of Antenna and Reactor Sites. ACS Sustain. Chem. Eng. 9, 9750–9760. doi:10.1021/acssuschemeng.1c02063
Rak, M. J., Friščić, T., and Moores, A. (2014). Mechanochemical Synthesis of Au, Pd, Ru and Re Nanoparticles with Lignin as a Bio-Based Reducing Agent and Stabilizing Matrix. Faraday Discuss. 170, 155–167. doi:10.1039/C4FD00053F
Rodrigues, M. P. d. S., Dourado, A. H. B., Cutolo, L. d. O., Parreira, L. S., Alves, T. V., Slater, T. J. A., et al. (2021). Gold-Rhodium Nanoflowers for the Plasmon-Enhanced Hydrogen Evolution Reaction under Visible Light. ACS Catal. 11, 13543–13555. doi:10.1021/acscatal.1c02938
Rodrigues, T. S., da Silva, A. G. M., de Moura, A. B. L., Freitas, I. G., and Camargo, P. H. C. (2016). Rational Design of Plasmonic Catalysts: Matching the Surface Plasmon Resonance with Lamp Emission Spectra for Improved Performance in AgAu Nanorings. RSC Adv. 6, 62286–62290. doi:10.1039/C6RA11362A
Ruiz-Ruiz, V.-F., Betancourt, I., Zumeta-Dubé, I., Díaz-Pardo, R., and Díaz, D. (2022). Magnetic Properties of Pd-Ag Nanoalloys Obtained by Liquid-Assisted Mechanochemical Pathway. J. Phys. Chem. Sol. 161, 110427. doi:10.1016/j.jpcs.2021.110427
Ruiz-Ruiz, V.-F., González-Olvera, R., Díaz-Pardo, R., Betancourt, I., Zumeta-Dubé, I., Díaz, D., et al. (2018). Mechanochemically Obtained Pd-Ag Nanoalloys. Structural Considerations and Catalytic Activity. Materialia 4, 166–174. doi:10.1016/j.mtla.2018.09.031
Saire-Saire, S., Barbosa, E. C. M., Garcia, D., Andrade, L. H., Garcia-Segura, S., Camargo, P. H. C., et al. (2019). Green Synthesis of Au Decorated CoFe2O4 Nanoparticles for Catalytic Reduction of 4-nitrophenol and Dimethylphenylsilane Oxidation. RSC Adv. 9, 22116–22123. doi:10.1039/C9RA04222A
Schreyer, H., Eckert, R., Immohr, S., de Bellis, J., Felderhoff, M., and Schüth, F. (2019). Milling Down to Nanometers: A General Process for the Direct Dry Synthesis of Supported Metal Catalysts. Angew. Chem. Int. Ed. 58, 11262–11265. doi:10.1002/anie.201903545
Sha, J., Paul, S., Dumeignil, F., and Wojcieszak, R. (2019). Au-based Bimetallic Catalysts: How the Synergy between Two Metals Affects Their Catalytic Activity. RSC Adv. 9, 29888–29901. doi:10.1039/c9ra06001d
Slater, T. J. A., Macedo, A., Schroeder, S. L. M., Burke, M. G., O’Brien, P., Camargo, P. H. C., et al. (2014). Correlating Catalytic Activity of Ag-Au Nanoparticles with 3D Compositional Variations. Nano Lett. 14, 1921–1926. doi:10.1021/nl4047448
Sun, L., Lv, P., Li, H., Wang, F., Su, W., and Zhang, L. (2018). One-step Synthesis of Au-Ag alloy Nanoparticles Using Soluble Starch and Their Photocatalytic Performance for 4-nitrophenol Degradation. J. Mater. Sci. 53, 15895–15906. doi:10.1007/s10853-018-2763-9
Suryanarayana, C. (2001). Mechanical Alloying and Milling. Prog. Mater. Sci. 46, 1–184. doi:10.1016/S0079-6425(99)00010-9
Tyson, C. C., Bzowski, A., Kristof, P., Kuhn, M., Sammynaiken, R., and Sham, T. K. (1992). Charge Redistribution in Au-Ag Alloys from a Local Perspective. Phys. Rev. B 45, 8924–8928. doi:10.1103/PhysRevB.45.8924
Vongsavat, V., Vittur, B. M., Bryan, W. W., Kim, J.-H., and Lee, T. R. (2011). Ultrasmall Hollow Gold-Silver Nanoshells with Extinctions Strongly Red-Shifted to the Near-Infrared. ACS Appl. Mater. Inter. 3, 3616–3624. doi:10.1021/am2008322
Wang, J. L., Ando, R. A., and Camargo, P. H. C. (2014). Investigating the Plasmon-Mediated Catalytic Activity of AgAu Nanoparticles as a Function of Composition: Are Two Metals Better Than One? ACS Catal. 4, 3815–3819. doi:10.1021/cs501189m
Keywords: mechanochemical synthesis, bottom-up approach, ball-milling, bimetallic nanoparticle, nanoalloys, nanocatalysis, galvanic replacement reaction, nitrobenzene reduction
Citation: da Silva RTP, Córdoba De Torresi SI and de Oliveira PFM (2022) Mechanochemical Strategies for the Preparation of SiO2-Supported AgAu Nanoalloy Catalysts. Front. Chem. 10:836597. doi: 10.3389/fchem.2022.836597
Received: 15 December 2021; Accepted: 05 January 2022;
Published: 02 February 2022.
Edited by:
Elena Vladimirovna Boldyreva, Novosibirsk State University, RussiaReviewed by:
Matej Baláž, Slovak Academy of Sciences, SlovakiaVasile-Dan Hodoroaba, Federal Institute for Materials Research and Testing (BAM), Germany
Copyright © 2022 da Silva, Córdoba De Torresi and de Oliveira. This is an open-access article distributed under the terms of the Creative Commons Attribution License (CC BY). The use, distribution or reproduction in other forums is permitted, provided the original author(s) and the copyright owner(s) are credited and that the original publication in this journal is cited, in accordance with accepted academic practice. No use, distribution or reproduction is permitted which does not comply with these terms.
*Correspondence: Paulo F. M. de Oliveira, cGF1bG9mbW9AdXNwLmJy