- 1Ningbo Institute of Materials Technology and Engineering, Chinese Academy of Sciences, Ningbo, China
- 2Center of Materials Science and Optoelectronics Engineering, University of Chinese Academy of Sciences, Beijing, China
The photocathodes are essential in photoelectrochemical systems for harvesting solar energy as green fuels. However, the light-absorbing p-type semiconductor in them usually suffers from carrier recombination issues. An effective strategy to address it is fabricating the p-n heterojunction to create an interfacial electric field. However, plenty of deposition process of the n-type layer for this purpose requires either sophisticated instruments or subsequent treatments, which may damage the vulnerable p-type structure. Herein, we report a mild approach for a ready-to-use n-type layer with full functionality. Structural analyses proved the successful coating of a uniform titania layer (up to 40 nm) over Cu2O without damaging its structure. Owing to the high Ti3+ content, the layer possesses excellent charge transport ability and requires no additional annealing. The heterojunction effectively facilitates the carrier separation and positively shifts the photocurrent onset potential for 0.2 V. The Mott–Schottky plot and the impedance study reveal an enhanced carrier collection with reduced charge transfer resistances. Such a nano-heterojunction can be further loaded with the hydrogen evolution catalyst, which almost doubles the photocurrent with an extended lifetime than that of the pristine Cu2O nanoarray. This approach puts forward a potentially scalable and efficient choice for fabricating photoelectrochemical devices.
Introduction
Oxide photocathodes have attracted great attention for the photoelectrocatalytic production of hydrogen fuels by solar water splitting (Li et al., 2020). Particularly, Cu2O has been demonstrated as one of the most promising choices among many light-absorbing materials. The intrinsic Cu vacancies as shallow acceptor levels lead to decent p-type characteristics (Olsen et al., 1982). However, challenges are limiting the performance of photocathodes. Specifically, many photoelectrodes suffer from surface redox instability and self-reduction by the interfacial accumulation of photoelectrons (Tilley et al., 2014). More importantly, the unsatisfactory charge separation and internal charge transport strongly degrade the practical efficiency from the theoretical expectation (Wick and Tilley, 2015; Luo et al., 2016). The short diffusion length of charge carriers in Cu2O restricted the thorough extraction of photoelectrons (Musselman et al., 2010). It has been demonstrated that various eye-of-sight deposition techniques can be used for the Cu2O structure with minor curvatures to address these challenges (Paracchino et al., 2012; Minguez-Bacho et al., 2015), such as the thermal evaporation or e-beam deposition, providing a straightforward and effective solution to load diverse functional layers (Dubale et al., 2015; Han et al., 2015). The multilayered integration of desired components greatly enhances both the efficiency and stability of Cu2O photocathodes (Zhang et al., 2013; João et al., 2016). For instance, using the rationally designed Ga2O3/TiO2/RuO2 overlayer, the photocathode exhibited an unprecedented stable high internal quantum efficiency over 120 h (Pan et al., 2018).
On the other hand, in recent years, the morphology control of the p-type light-absorbing layer has been recognized as another answer to effectively balance the surface area and charge transport pathways (Chen et al., 2012; Concina et al., 2017). The state-of-the-art efficiency has been achieved by engineering Cu2O from flat films into nanowire arrays (NWAs) to facilitate photocarrier extraction (Qu et al., 2019). By incorporating the merit of all measures, the Cu2O NWA-based photocathodes were manifested as the landmark featuring their excellent photoelectrochemical (PEC) efficiency and stability (Huang et al., 2013). Unfortunately, these three-dimensional architectures also drastically increased the difficulty of the fabrication processes. Given the great curvatures and existing shaded area on NWAs, only atomic-layer deposition is capable of realizing the uniform heterojunctions with intimate contact of components (Luo et al., 2016). Undoubtedly, the realization of the desired coating is the key to fully eliciting the potential of the complicated nanostructures.
Specifically, titanium oxide appears to be one of the essential components in the coating. It does not only prevent corrosions of Cu2O from the electrolyte solution but also rectifies the flow of photogenerated carriers (Li et al., 2015). The heterojunction between Cu2O and titanium oxide via various techniques has been demonstrated as essential for these high-performance photocathodes (Siripala et al., 2003; Lumley et al., 2019). However, as we stated, there are only extremely limited approaches that can be used for Cu2O NWAs (and certainly for other p-type nanostructures). Besides, titanium oxide has other uses (Azevedo et al., 2016; Yang et al., 2018; Wang et al., 2021). The most well-established yet almost the only route without post-treatments, the atomic-layer deposition technique, demands sophisticated instrumentation with expensive precursors (organometallic complexes) and experienced selection of conditions that delivers great impacts to the properties of the coating (Dai et al., 2014). On the other hand, there are incompatibility issues during the chemical depositions, whereas many of the bared p-type structures are instable. For instance, the hydrolysis of Ti alkoxide forms uniform titania coatings but requires following high-temperature annealing for the crystallization, which may be destructive for the bottom nanostructures (Paracchino et al., 2011). Therefore, a facile and mild titanium oxide deposition method is highly desired.
Specifically, there is no ready-to-use and uniform coating of semiconductive titanium oxides on Cu2O NWAs via chemical room temperature chemical route yet (Jang and Lee, 2019). The chemical limitation in aqueous electrolytes has been suggested by the Pourbaix diagram of the Cu element (Yang et al., 2017). The compatible conditions for Cu2O are restricted to near-neutral solutions with a narrow redox potential range. Notably, many previously reported electrodeposition of titanium oxides in harsh pH and oxidative conditions are not applicable (Eisenberg et al., 2014), for instance, the cathodic coating using the peroxide-dissolved Ti complex or anodizing Ti3+ in the strongly acidic solution (Kavan et al., 1993; Matsumoto et al., 2000). Nonetheless, titanium oxide layers from oxidizing Ti(III) over other nanostructures effectively formed various junctions, exhibiting outstanding photochemical or PEC properties (Toe et al., 2019). Unfortunately, none of these electrochemical processes is applicable to Cu2O nanostructures.
In this work, we successfully developed a new electrochemical route to realize the coating of functional titania over Cu2O NWAs. Owing to the mild environment and the deliberately controlled transient anodization, the coating thickness can be regulated with the well-maintained original NWA morphology. More importantly, the great portion of Ti3+ in the structure directly endows the coating's good charge extraction and transport ability. Specifically, the almost tripled photocurrent (from 1.17 to 3.07 mA/cm2, 0.55 V vs. RHE) and the positive shift of onset potential of the Cu2O–TiOx NWA photocathodes clearly prove the formation of a heterojunction facilitating the directional flow of photoelectrons. Moreover, combining its functionality with the assistance of the HER catalyst further raises the activity of the photocathodes. These features of the photocathodes present a new promising route of using a rationally designed electrochemical process to fabricate uniform and functioning heterostructure under mild conditions.
Results and Discussion
Figure 1 schematically illustrates the preparation process of the Cu2O/TiOx/Ni NWA photocathodes. The bottom Cu2O NWAs were prepared by a two-step method using our previously reported anodization process for uniformed Cu(OH)2 NWAs, followed by a spontaneous thermal conversion to Cu2O in an inert atmosphere (Cao et al., 2020). Morphologies of the photocathode at different fabrication stages were shown in scanning electron microscope (SEM) graphs (Figures 2A–D). The process was initiated by preparing uniform high-density Cu(OH)2 NWAs, with an average length of approximately 9 μm. After annealing in N2, Cu(OH)2 was converted into Cu2O NWAs. As seen from Figure 2B, the transformed arrays consisted of twisted and slightly shortened nanowires with an average length of 6.8 μm. The typical obtained Cu2O/TiOx NWAs are shown in Figure 2C. The thin layer of amorphous TiOx can barely be seen on the SEM micrograph, whereas some of the adjacent NWs were seized as bundles by the coating. The thickness of the TiOx layer was estimated using transmission electron microscope (TEM) (Figure 2E) and high-angle annular dark-field scanning transmission electron microscope/energy dispersive X-ray mapping (Figure 2H) as approximately 30–50 nm. The aspect ratio slightly decreased but still could provide shortened diffusion paths for the photoelectron to the solid-electrolyte interface than the flat structures. Eventually, a thin layer (∼3 nm) of Ni was deposited onto the structure as the hydrogen evolution reaction (HER) catalyst, with no evident morphological impact (Figure 2D).
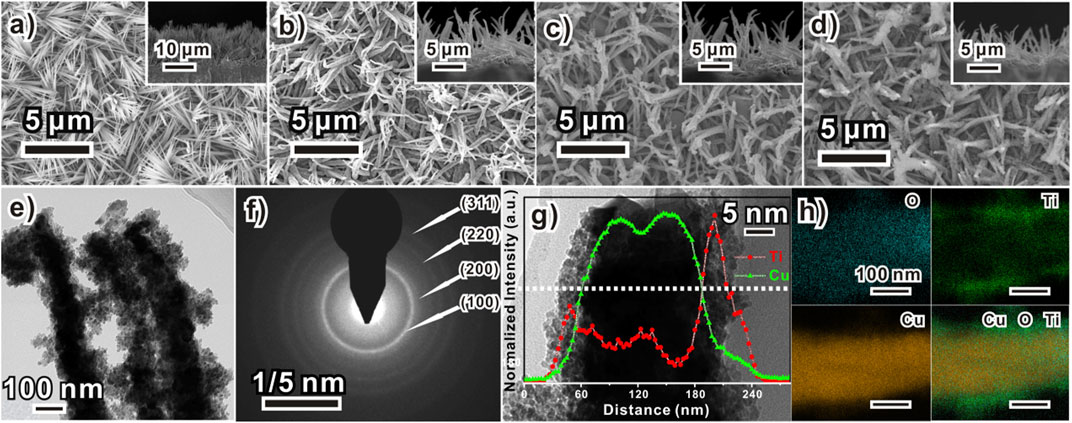
FIGURE 2. Morphology of Cu2O/TiOx/Ni composite electrode formation process of a sample (A) Cu(OH)2 NWA, (B) bare Cu2O NWA, (C) Cu2O/TiOx, and (D) Cu2O/TiOx/Ni (insets are their corresponding cross-section images) NWA; (E) TEM image and (F) selected area electron diffraction pattern of Cu2O/TiOx NWs; (G) HRTEM high magnification images of Cu2O/TiOx NW with its line-scanned element distribution profile and (H) elemental mapping of O, Ti, and Cu.
The nature of the electrodeposited titanium oxide coating layer was revealed by TEM microscopy. Figure 2E shows the typical structure of Cu2O/TiOx core-shell NWs in the array, clearly showing that Cu2O NWs were encapsulated by an amorphous layer. The titania layer was approximately 40 nm thick and assembled by primary clusters smaller than 5 nm. Micrographs showed intimate contacts of this coating layer with the inner Cu2O NW. A minor porosity and notable roughness can be found as well. The selected area electron diffraction pattern (Figure 2F) only exhibited rings corresponding to (111), (200), (220), and (311) d-spacing of Cu2O, suggesting its polycrystalline nature. No evidence of crystalized titanium oxides was observed either, which agrees with the X-ray diffraction (XRD) result (Figure 3A). High-angle annular dark-field scanning transmission electron microscope/energy dispersive X-ray mapping (Figure 2H) illustrates the elemental distribution line profile and mapping across a typical NW. The signal of oxygen sketches the overall shape of the NW, whereas Cu and Ti panels clearly confirm the spatial relationship of a core-shell structure.
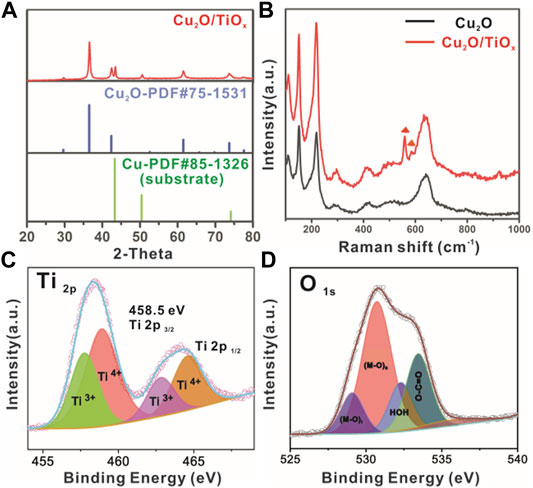
FIGURE 3. (A) XRD pattern of typical Cu2O/TiOx NWA sample; (B) Raman spectra of Cu2O and Cu2O/TiOx NWA samples; (C,D) X-ray photoelectron spectroscopy signal of Ti 2p and O 1s from Cu2O/TiOx, respectively.
The phase composition of the heterostructure was confirmed by XRD as well (Figure 3A). Despite the diffraction of 43.3 and 50.4° corresponding to (111) and (200) d-spacing of metallic Cu substrate (JCPDS 58-1326), respectively, all other peaks can be indexed to the pattern of cubic Cu2O (JCPDS 75-1531). No other diffraction peaks were found on the XRD pattern, suggesting the TiOx layer was amorphous. Raman spectroscopy was used to further study the composition regarding the existence of amorphous oxides. Figure 3B shows the Raman spectra of Cu2O and Cu2O/TiOx samples. Common features on both spectra at 109, 148, 416, 515, and 635 cm−1 can be assigned to the vibrational modes of Cu2O lattices (Maldonado-Larios et al., 2020). Different from the XRD technique, TiOx (amorphous) on the Cu2O surface is visible to the Raman spectroscopic study. The bands at 568 and 608 cm−1 that emerged on the spectrum of Cu2O/TiOx sample are associated with the deformation of out-of-plane rings (Ohsaka et al., 1978; Jumat et al., 2017). Given that no other peaks were observed, the Raman spectroscopy strongly supported the existence of titania as an amorphous form. Moreover, the successful coating was further confirmed by the ultraviolet–visible diffuse reflectance spectroscopy of Cu2O NWAs and Cu2O/TiOx NWAs samples (Supplementary Figure S1). The latter had much stronger light absorption in ultraviolet, indicating the deposition of the TiOx layer over the entire sample. The absorption in the visible range slightly decreased (in K-M expression), which is likely due to the increased scattering. Nonetheless, from the view of PEC efficiency, this is negligible on the scale of the reflection rates (Supplementary Figure S1).
X-ray photoelectron spectroscopy survey was carried out to collect the valence state and chemical environment information of elements in the Cu2O/TiOx photocathode. The full spectral range scans (Supplementary Figure S2) confirmed the elemental composition of Cu, O, and Ti. The fine scan of the Ti 2p spectrum (Figure 3C) shows the typical bands of Ti 2p3/2 and Ti 2p1/2 core levels at 458.5 and 464.9 eV, respectively. The experimental curves were well fitted with the Gaussian peaks model after Tougaard background subtraction, whereas the valence states of Ti can be identified by deconvoluting the fitting result, consisting of both Ti3+ and Ti4+. To our surprise, the percentage of Ti3+ is as high as 38% in the coating layer (Supplementary Table S1). The signal from O1s is slightly more complicated (Figure 3D), showing three different chemical environments: metal oxides (Ti-O at 529.1 and Cu-O 530.7 eV), hydroxyl (535.6 eV), and absorbed water (532.3 eV). Because the sample was not annealed, the carboxylic group should be the result of residue oxalate in the electrolyte. It is worthy to note that the signal from Cu in Cu2O is stronger and higher than the expectation of having a screening effect from the titania shell. According to TEM (Figures 2E–G), this could be from the recrystallized small Cu2O clusters during the deposition process. In short, the X-ray photoelectron spectroscopy result confirms the overall composition of the sample as oxides, but the coating layer cannot be simply regarded as TiO2, given its extremely high level of Ti3+. On the other hand, apparently, the coating does not contain a large number of hydroxyl groups. This can be beneficial to charge transport by reducing the chance of recombination (Carp et al., 2004).
The structural analysis discussed earlier proved the realization of our electrochemical route. Chemical compatibility is the essential challenge in this deposition process. Given the vulnerability of Cu2O, the electrolyte solution has to be near neutral. On the other side, Ti3+ is unstable as well, which hydrolyzes when pH > 4 and can be easily oxidized into hydrated TiO2 (Johnson et al., 2017). Hence, all reported cases were performed under very low pH with relatively stable substrates. We took several measures to stabilize Ti3+ and bring it to a workable condition for Cu2O. A water/ethylene glycol solution was used instead of a typical pure aqueous system. Hydrolysis of Ti(III) can be notably reduced with the interaction between high valent ions with the polyol environment. An optimal composition was experimentally determined as 50% (Supplementary Figure S3). Chelating/complex agents were introduced to further stabilize Ti(III) species (Lavacchi et al., 2021). The oxalic acid was selected based on both the coating coverage and photocurrent of the product.
To further achieve the uniform TiOx distribution on the Cu2O, other important parameters were carefully evaluated as well. Surfactants have been proved beneficial in many electrodeposition systems. For this system, we found that Triton X100 could moderately improve the quality of the product (Supplementary Figure S4), which likely takes effect by facilitating the wetting and diffusion of electrolytes in the NWAs (Liu et al., 2020). Again, the deposition temperature gave almost no impact on TiOx (Supplementary Figure S5), indicating that the rate-determining step in the deposition is dominated by temperature-insensitive factors.
Furthermore, the typical linear sweep voltammetry (LSV) curve (Figure 4E) of this anodic reaction demonstrates more details of the process. Despite a minor side reaction from the substrate (AI peak), anodic signals form one broad peak. Such a feature suggests the chemical inhomogeneity of Ti(III) species, as they should be a series of hydroxyl oligomers (Qin et al., 2020). Indeed, a minor Tindall effect was found with the deposition electrolyte “solution” (or should be called “sol”), although it is stable for several hours. The stability of the electrolyte is sufficient for us to prepare approximately six parallel samples at one time, so it has high reproducibility. The distance between the quartz electrolytic cell (10 × 10 cm2) and the light source is 5 cm, which needs to meet the luminous power of up to 100 mW. The working area of the electrode is 1 × 1 cm2.
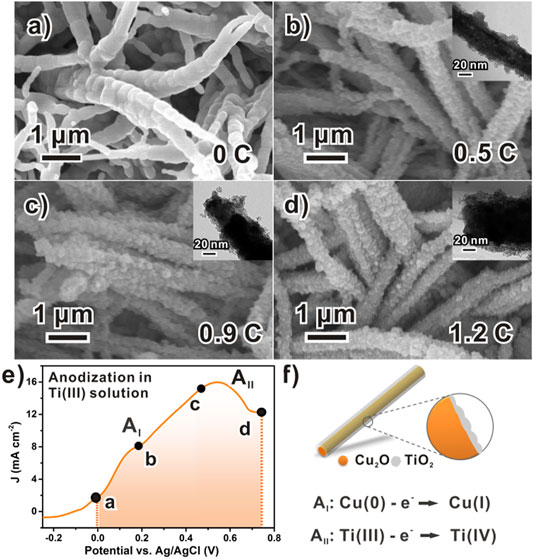
FIGURE 4. Chronology of TiOx formation in potentiodynamic anodization process: (A) Scanning electron microscope images of bare Cu2O NWAs; (B–D) Corresponding scanning electron microscope images showing morphological evolution of amorphous TiOx along with increasing anodization charge. (E) Typical LSV curve of anodizing a piece of Cu2O foil with rate of 5 mV/s, data points labeled with charges specifying samples examined in parts b–d and (F) schematic model to formation of Cu2O/TiOx NWAs.
Different electrochemical techniques were considered as well. Ideally, the electrodeposition can be performed using various techniques, such as the galvanostatic or potentiostatic process, or transient methods such as linear potential scans or AC controls. However, as we tested, none of the galvanostatic or potentiostatic processes produced reliable and reproducible results. This could be due to the large surface conductivity change during the deposition. On the contrary, directly using the LSV scan worked quite well for the deposition. In addition, the scan rate can be used as a handy tool to kinetically discriminate reactions, which reduces the redox corrosion to Cu2O. In our case, severe destruction to the NWA structure or insufficient deposition was observed with inappropriate scan rates (Supplementary Figure S6).
Moreover, fine control of the thickness of TiOx can be achieved by regulating the anodization charge during the deposition process (Figure 4). Figure 4A shows the typical relatively smooth surface of the pristine Cu2O structures before the deposition of TiOx. After that, as the anodization progressed, amorphous TiOx was gradually loaded onto the Cu2O surface (Figures 4B–D) with increasing amounts. At the anodic charge of 0.5 C cm−1 (Figure 4B), the Cu2O surface was roughened with the discrete coating of approximately 10 nm, which is not sufficient to completely hinder the photo-corrosion of Cu2O yet. Meanwhile, a minor improvement to the photocurrent was observed (Supplementary Figure S7). As the charge increased to 0.9 C cm−1, more amorphous TiOx was loaded onto Cu2O, whereas the thickness was measured as approximately 20 nm (Figure 4C). In the finishing stage, the Cu2O NWs were fully covered by the deposited TiOx layer, with the coating thickness eventually reaching 40 nm (Figure 4D). The complete deposition notably improved water reduction photocurrents of the NWA photocathode from 1.35 to 4.15 mA/cm2 at 0.55 V vs. RHE (Supplementary Figure S7). In principle, further thicker coating might be possible by optimizing conditions. However, for our current purpose in the PEC application, coating less than 50 nm is reasonable and similar to the typical thickness of reported titanium oxide layers (Musselman et al., 2010).
The PEC performance confirmed the functionality of the coating layer, as shown in Figure 5. Specifically, LSV scans of pristine-Cu2O, Cu2O/TiOx, and Cu2O/TiOx/Ni photocathodes were collected in 0.5-M sodium phosphate buffer under chopping AM1.5G illumination. The current density–potential curves clearly show that Cu2O/TiOx photocathode had a significantly enhanced photoactivity, in which photocurrent density reached −3.0 mA/cm2 at 0.55 V vs. RHE, whereas the pristine-Cu2O only had -1.1 mA/cm2 parallelly. Moreover, with Ni cocatalyst loaded onto Cu2O/TiOx photocathodes, the photocurrent density could be further enhanced to −4.7 mA/cm2 at 0.55 V vs. RHE, which is over four times higher than that of pristine Cu2O photocathode, showing a significant enhancement in photo-response that resulted from the effective heterojunction formed inside of Cu2O/TiOx. Furthermore, Cu2O/TiOx/Ni photocathode had a more positive onset potential of 0.82 V vs. RHE, 0.18 V positive to the Cu2O photocathode, as shown in Figure 5A. Both of these phenomena indicate that the Cu2O/TiOx/Ni interface could significantly improve the utilization of photocarriers with the smaller hindrance and the lower activation energy barrier in comparison with the pristine Cu2O surface.
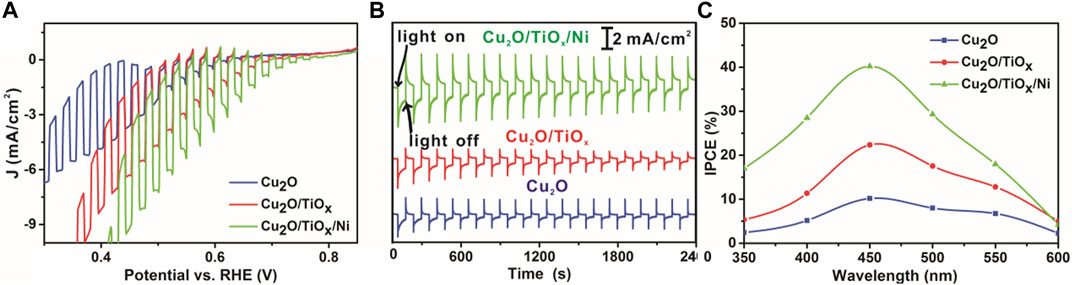
FIGURE 5. Current-potential (I–V) characteristics curve, stability, and IPCE test (A) I–V curves under chopping simulated AM 1.5G illumination (B) stability measurements with constant bias at 0.6 V vs. RHE and under simulated AM 1.5G illumination (C) IPCE of pristine Cu2O NWAs, Cu2O/TiOx NWA, and Cu2O/TiOx/Ni NWA samples (all tests performed in pH = 6 electrolytes).
As is shown in Figure 5B, the TiOx coating and Ni cocatalyst notably benefited the stability of the photocathodes. The photocurrent of pristine-Cu2O photocathode decreased by approximately 60% in 40 min. SEM (Supplementary Figure S8) image as pieces of evidence that the NWs were seriously damaged and deformed. Energy dispersive X-ray (EDX) analysis confirms the decrease of oxygen content, indicating that the Cu2O was considerably reduced. This agrees with the typical photo-corrosion mechanism of Cu2O (Toe et al., 2019). With the coating of the TiOx layer, the stability of the photocathode was notably improved. The photocurrent density decreased by approximately 40%. It is reasonable to speculate that it was still due to the self-reduction reaction, according to the EDX result. Metallic Cu nanoparticles can be distinctly discriminated after the test (Supplementary Figure S8D), implying the leakage of Cu to the electrolyte. Furthermore, the location of the speciated Cu islands indicates that the photoelectrons have been extracted to the TiOx part. Otherwise, the reduction of Cu2O should not produce Cu crystallites outside the coating. Nonetheless, HER was not the favored reaction over the surface due to lacking HER sites. Once the necessary catalyst (Ni) was integrated, the photocathodes became much more stable with better PEC efficiency. SEM and EDX (Supplementary Table S2) confirmed that the morphology and composition of Cu2O/TiOx/Ni photocathode were well maintained after the stability test. Only very few bright metallic Cu islands can be found on the surface of the photocathode, which validated the functionality of the cocatalyst. It is worthy to emphasize that the coating of this single TiOx layer is helpful for the photoelectron collection from Cu2O but still not the ultimate solution to the system. Clearly, an additional measure is necessary to fill out the pores, and the efficiency of the photocathodes can be further improved. Nonetheless, the deposition of TiOx solved one of the most important yet challenging steps for the functionalization of Cu2O.
The key advantage of the nanostructure approach is the ability to improve the charge transport and minimize the recombination by constructing heterojunction and providing a stable support structure for the cocatalyst. The incident photon-to-current efficiency (IPCE) obtained from the LSV measurement was greatly improved. As shown in Figure 5C, the IPCE for the Cu2O/TiOx/Ni photocathode is reasonably higher than that of the pristine-Cu2O across the whole wavelengths, especially in the visible region between 420 and 470 nm.
To understand the details of the charge transfer in the photocathodes, we performed an electrochemical impedance spectroscopy (EIS) study, which is a powerful frequency domain analysis technique for investigating electrical characters of interfaces (Chen et al., 2017). Figure 6A shows the impedance data of parallelly tested samples with complex coordinates as Nyquist plots. The very high-frequency region on the lower left corresponds to the series resistance and response from the counter electrode, which is approximately 5 Ω for all samples. Semicircles on the plots represent the impedance of interfaces or electrochemical processes, whereas the diameters of semicircles are equal to the corresponding resistances. Straightforwardly, a larger semicircle correlates to worse charge transportation through that interface. The linear portion of the lower frequency, so-called a Warburg element, represents the diffusion-controlled impedance (Kecsenovity et al., 2017). Visually, the charge transfer difference of Cu2O, Cu2O/TiOx, and Cu2O/TiOx/Ni photocathodes can be clearly addressed in the plots (Figure 6A). The step-by-step architecting by the TiOx coating and cocatalyst loading gradually lowered the overall charge transport impedance. The fitting result (Supplementary Table S3) indicates that the Cu2O/TiOx samples have a much smaller Rct2 in comparison with pristine-Cu2O, which benefits from the promoted charge separation and transport under the assistance of the Cu2O–TiOx heterojunction. Meanwhile, CPE1 has increased several times, implying a capacitive feature on the Cu2O–TiOx interface. All interfacial resistances were further reduced by Ni cocatalyst loading. Particularly, CPE2 is notably smaller, suggesting less charge accumulation on the photocathodes because of the expedited HER pathway.
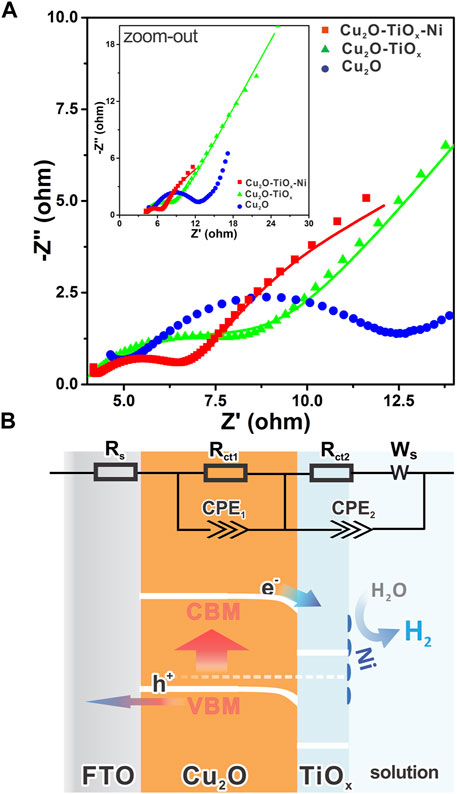
FIGURE 6. (A) Nyquist plots of different electrodes in 0.5 M phosphate buffer under light irradiation (λ = 455 nm) with electrochemical impedance spectroscopy at a DC potential of 0.5 V vs. Ag/AgCl in AC potential frequency range of 10 to 1 Hz, inset shows zoomed-out view with fewer details; (B) schematic band diagram of Cu2O/TiOx/Ni photocathode representing internal charge transfer direction, with inset showing equivalent circuit for electrochemical impedance spectroscopy analysis.
Figures 7A–C show the Mott–Schottky (M-S) measurement results of the photocathodes. The measurement can be used to determine the type of semiconductors, flat band potentials, and carrier densities (Patel et al., 2017; Sivula, 2021). Using the following equation, important semiconductor parameters were linked together:
where CSC is the space-charge capacitance, V is the applied potential, Vfb is the flat band potential, ε is the dielectric constant of Cu2O, ε0 is the permittivity of vacuum, A is the area, N is the carrier density, e is the electron charge, kB is the Boltzmann’s constant, and T is the absolute temperature.
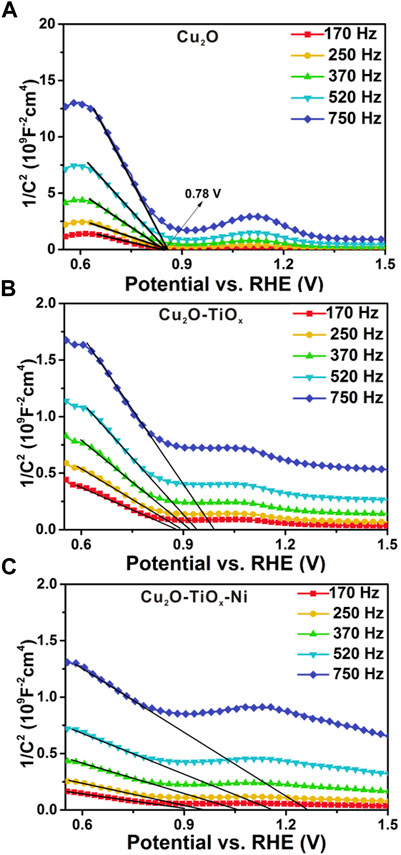
FIGURE 7. Mott–Schottky plots (A–C) for bared Cu2O, Cu2O/TiOx, and Cu2O/TiOx/Ni photocathodes, respectively. Measurements performed from 0.0 to 1.0 V vs. Ag/AgCl in pH = 6.0 0.5 M potassium phosphate buffer using multi-sine staircase potential impedance technique.
According to the equation discussed earlier, intercepts of the curve in M-S plots were used to determine Vfb. For the TiOx (Supplementary Figure S9) on metal and the bared Cu2O NWA (Figure 7A, a relatively straightforward result can be obtained indicating their n-type and p-type semiconductive nature, respectively. However, in the case of the possible frequency dispersion caused by nonideal behaviors of electrode capacitances, their M-S correlations were plotted using a series of frequencies. The dataset suggests the Vfb of the Cu2O photocathode as 0.78 V vs. RHE. For Cu2O/TiOx and Cu2O/TiOx/Ni photocathodes, large frequency dispersion was observed. A precise determination of their flat band potentials is unrealistic, although their Vfb can be speculated to be similar to pristine-Cu2O or with minor positive shifts. On the other hand, the measurements manifest the carrier density difference between the three electrodes. Specifically, the reciprocal of slopes on the tangents are proportional to N, the carrier densities in the space charge region. Despite the frequency dispersion, the slopes of Cu2O/TiOx are an order smaller than pristine-Cu2O, suggesting more responding charge carriers in the space charge region. This increment hints at a more efficient extraction of photoelectrons and less recombination in the bulk. According to the structure of the photocathodes, this beneficial effect shall be attributed to the electric field formed on the heterojunction interface, which alters the carrier distribution in Cu2O and subsequently notably facilitates the carrier extraction.
Conclusion
In short, we successfully achieved the aimed titania electrodeposition in near-neutral conditions for preparing uniform heterojunction over a vulnerable p-type semiconductor nanostructure. Due to the existence of a large portion of Ti3+, this electrodeposited layer directly exhibited a charge transport ability without any post-treatment. Both the onset potential and PEC efficiency of the NWA photocathodes were greatly improved. By further incorporating with Ni-based HER cocatalyst, the accomplished photocathodes exhibited a photocurrent approximately four times of the pristine-Cu2O NWAs, with a much longer lifetime. Electrochemical impedance spectroscopy and M-S measurements revealed that the TiOx layer facilitated the charge transport inside the space charge region. This protocol is reliable and effective but requires no sophisticated instrumentation. These merits make it easily applicable to other unstable nanostructures. Moreover, these results demonstrated the possibility of electrochemically fabricating cost-effective photoelectrodes with both earth-abundant materials and affordable preparation expenditures.
Data Availability Statement
The original contributions presented in the study are included in the article/Supplementary Material; further inquiries can be directed to the corresponding authors.
Author Contributions
YC conceived and designed the experiments. HQ assisted in experiments and the revision process. YLZ analyzed experimental data and modified articles, and NA, and YZ modified articles. All authors contributed to the article and approved the submitted version.
Funding
This work was supported by the National Natural Science Foundation of China (grant nos. 21805298 and 21905288), Ningbo's major special projects of the Plan “Science and Technology Innovation 2025” (no. 2018B10056), and Ningbo 3315 Program.
Conflict of Interest
The authors declare that the research was conducted in the absence of any commercial or financial relationships that could be construed as a potential conflict of interest.
Publisher’s Note
All claims expressed in this article are solely those of the authors and do not necessarily represent those of their affiliated organizations or those of the publisher, the editors, and the reviewers. Any product that may be evaluated in this article, or claim that may be made by its manufacturer, is not guaranteed or endorsed by the publisher.
Supplementary Material
The Supplementary Material for this article can be found online at: https://www.frontiersin.org/articles/10.3389/fchem.2022.832342/full#supplementary-material
References
Azevedo, J., Tilley, S. D., Schreier, M., Stefik, M., Sousa, C., Araújo, J. P., et al. (2016). Tin Oxide as Stable Protective Layer for Composite Cuprous Oxide Water-Splitting Photocathodes. Nano Energy 24, 10–16. doi:10.1016/j.nanoen.2016.03.022
Cao, Y., Liu, D., Ni, X., Meng, X., Zhou, Y., Sun, Z., et al. (2020). Better Charge Separation in CuO Nanowire Array Photocathodes: Micro-/nanostructure Regulation for Photoelectrochemical Reaction. ACS Appl. Energ. Mater. 3, 6334–6343. doi:10.1021/acsaem.0c00554
Carp, O., Huisman, C. L., and Reller, A. (2004). Photoinduced Reactivity of Titanium Dioxide. Prog. Solid State. Chem. 32, 33–177. doi:10.1016/j.progsolidstchem.2004.08.001
Chen, H. M., Chen, C. K., Liu, R.-S., Zhang, L., Zhang, J., and Wilkinson, D. P. (2012). Nano-Architecture and Material Designs for Water Splitting Photoelectrodes. Chem. Soc. Rev. 41, 5654–5671. doi:10.1039/C2CS35019J
Chen, W., Zhang, W., Chen, L., Zeng, L., and Wei, M. (2017). Facile Synthesis of Cu2O Nanorod Arrays on Cu Foam as a Self-Supporting Anode Material for Lithium Ion Batteries. J. Alloys Compd. 723, 172–178. doi:10.1016/j.jallcom.2017.06.153
Concina, I., Ibupoto, Z. H., and Vomiero, A. (2017). Semiconducting Metal Oxide Nanostructures for Water Splitting and Photovoltaics. Adv. Energ. Mater. 7, 1700706. doi:10.1002/aenm.201700706
Dai, P., Li, W., Xie, J., He, Y., Thorne, J., Mcmahon, G., et al. (2014). Forming Buried Junctions to Enhance the Photovoltage Generated by Cuprous Oxide in Aqueous Solutions. Angew. Chem. Int. Ed. 53, 13493–13497. doi:10.1002/anie.201408375
Dubale, A. A., Pan, C.-J., Tamirat, A. G., Chen, H.-M., Su, W.-N., Chen, C.-H., et al. (2015). Heterostructured Cu2O/CuO Decorated with Nickel as a Highly Efficient Photocathode for Photoelectrochemical Water Reduction. J. Mater. Chem. A. 3, 12482–12499. doi:10.1039/c5ta01961c
Eisenberg, D., Ahn, H. S., and Bard, A. J. (2014). Enhanced Photoelectrochemical Water Oxidation on Bismuth Vanadate by Electrodeposition of Amorphous Titanium Dioxide. J. Am. Chem. Soc. 136, 14011–14014. doi:10.1021/ja5082475
Han, J., Zong, X., Zhou, X., and Li, C. (2015). Cu2O/CuO Photocathode with Improved Stability for Photoelectrochemical Water Reduction. RSC Adv. 5, 10790–10794. doi:10.1039/c4ra13896a
Huang, Q., Kang, F., Liu, H., Li, Q., and Xiao, X. (2013). Highly Aligned Cu2O/CuO/TiO2core/shell Nanowire Arrays as Photocathodes for Water Photoelectrolysis. J. Mater. Chem. A. 1, 2418–2425. doi:10.1039/c2ta00918h
Jang, Y. J., and Lee, J. S. (2019). Photoelectrochemical Water Splitting with p‐Type Metal Oxide Semiconductor Photocathodes. ChemSusChem 12, 1835–1845. doi:10.1002/cssc.201802596
Johnson, K. E., Gakhar, S., Deng, Y., Fong, K., Risbud, S. H., and Longo, M. L. (2017). Biomembrane-Compatible Sol-Gel-Derived Photocatalytic Titanium Dioxide. ACS Appl. Mater. Inter. 9, 35664–35672. doi:10.1021/acsami.7b12673
Jumat, N. A., Wai, P. S., Ching, J. J., and Basirun, W. J. (2017). Synthesis of Polyaniline-TiO2 Nanocomposites and Their Application in Photocatalytic Degradation. Polym. Polym. Compos. 25, 507–514. doi:10.1177/096739111702500701
Kavan, L., O'Regan, B., Kay, A., and Grätzel, M. (1993). Preparation of TiO2 (Anatase) Films on Electrodes by Anodic Oxidative Hydrolysis of TiCl3. J. Electroanal. Chem. 346, 291–307. doi:10.1016/0022-0728(93)85020-H
Kecsenovity, E., Endrődi, B., Tóth, P. S., Zou, Y., Dryfe, R. A. W., Rajeshwar, K., et al. (2017). Enhanced Photoelectrochemical Performance of Cuprous Oxide/Graphene Nanohybrids. J. Am. Chem. Soc. 139, 6682–6692. doi:10.1021/jacs.7b01820
Lavacchi, A., Bellini, M., Berretti, E., Chen, Y., Marchionni, A., Miller, H. A., et al. (2021). Titanium Dioxide Nanomaterials in Electrocatalysis for Energy. Curr. Opin. Electrochem. 28, 100720. doi:10.1016/j.coelec.2021.100720
Li, C., Hisatomi, T., Watanabe, O., Nakabayashi, M., Shibata, N., Domen, K., et al. (2015). Positive Onset Potential and Stability of Cu2O-Based Photocathodes in Water Splitting by Atomic Layer Deposition of a Ga2O3buffer Layer. Energ. Environ. Sci. 8, 1493–1500. doi:10.1039/c5ee00250h
Li, C., He, J., Xiao, Y., Li, Y., and Delaunay, J.-J. (2020). Earth-abundant Cu-Based Metal Oxide Photocathodes for Photoelectrochemical Water Splitting. Energy Environ. Sci. 13, 3269–3306. doi:10.1039/D0EE02397C
Liu, Z., Wang, L., Li, L., Qiu, F., Xiong, X., Tao, C., et al. (2020). Surfactant Effect on Controllable Phase Transformation and UV-Shielding Performance of Titanium Dioxide. Mater. Chem. Phys. 240, 122079. doi:10.1016/j.matchemphys.2019.122079
Lumley, M. A., Radmilovic, A., Jang, Y. J., Lindberg, A. E., and Choi, K.-S. (2019). Perspectives on the Development of Oxide-Based Photocathodes for Solar Fuel Production. J. Am. Chem. Soc. 141, 18358–18369. doi:10.1021/jacs.9b07976
Luo, J., Steier, L., Son, M.-K., Schreier, M., Mayer, M. T., and Grätzel, M. (2016). Cu2O Nanowire Photocathodes for Efficient and Durable Solar Water Splitting. Nano Lett. 16, 1848–1857. doi:10.1021/acs.nanolett.5b04929
Maldonado-Larios, L., Mayen-Mondragón, R., Martínez-Orozco, R. D., Páramo-García, U., Gallardo-Rivas, N. V., and García-Alamilla, R. (2020). Electrochemically-assisted Fabrication of Titanium-Dioxide/polyaniline Nanocomposite Films for the Electroremediation of congo Red in Aqueous Effluents. Synth. Met. 268, 116464. doi:10.1016/j.synthmet.2020.116464
Matsumoto, Y., Ishikawa, Y., Nishida, M., Ii, S., Wang, G., and Li, Y. (2000). A New Electrochemical Method to Prepare Mesoporous Titanium(IV) Oxide Photocatalyst Fixed on Alumite Substrate. J. Phys. Chem. B 104, 4204–4209. doi:10.1021/jp9944177
Minguez-Bacho, I., Courté, M., Fan, H. J., and Fichou, D. (2015). Conformal Cu2S-Coated Cu2O Nanostructures Grown by Ion Exchange Reaction and Their Photoelectrochemical Properties. Nanotechnology 26, 185401. doi:10.1088/0957-4484/26/18/185401
Musselman, K. P., Wisnet, A., Iza, D. C., Hesse, H. C., Scheu, C., Macmanus-Driscoll, J. L., et al. (2010). Strong Efficiency Improvements in Ultra-low-cost Inorganic Nanowire Solar Cells. Adv. Mater. 22, E254–E258. doi:10.1002/adma.201001455
Ohsaka, T., Izumi, F., and Fujiki, Y. (1978). Raman Spectrum of Anatase, TiO2. J. Raman Spectrosc. 7, 321–324. doi:10.1002/jrs.1250070606
Olsen, L. C., Addis, F. W., and Miller, W. (1982). Experimental and Theoretical Studies of Cu2O Solar Cells. Solar Cel. 7, 247–279. doi:10.1016/0379-6787(82)90050-3
Pan, L., Kim, J. H., Mayer, M. T., Son, M.-K., Ummadisingu, A., Lee, J. S., et al. (2018). Boosting the Performance of Cu2O Photocathodes for Unassisted Solar Water Splitting Devices. Nat. Catal. 1, 412–420. doi:10.1038/s41929-018-0077-6
Paracchino, A., Laporte, V., Sivula, K., Grätzel, M., and Thimsen, E. (2011). Highly Active Oxide Photocathode for Photoelectrochemical Water Reduction. Nat. Mater 10, 456–461. doi:10.1038/nmat3017
Paracchino, A., Mathews, N., Hisatomi, T., Stefik, M., Tilley, S. D., and Grätzel, M. (2012). Ultrathin Films on Copper(I) Oxide Water Splitting Photocathodes: a Study on Performance and Stability. Energ. Environ. Sci. 5, 8673–8681. doi:10.1039/C2EE22063F
Patel, M., Yu, X., Kim, Y. K., and Kim, J. (2017). Facile Formation of Nanodisk-Shaped Orthorhombic SnS Layers from SnS2 Particles for Photoelectrocatalytic Hydrogen Production. ChemNanoMat 3, 591–600. doi:10.1002/cnma.201700118
Qin, Z.-M., Wang, P.-C., Yang, R., and Chen, H.-B. (2020). Fast Pyrolysis of Silicones at Low Temperatures Catalyzed by Anatase Titanium Dioxide. Polym. Degrad. Stab. 182, 109387. doi:10.1016/j.polymdegradstab.2020.109387
Qu, Y., Zhang, P., Liu, J., Zhao, L., Song, X., and Gao, L. (2019). Facile Fabrication and Hydrophobic Properties of Cu2O Nanowire Films on Cu Substrates. Mater. Chem. Phys. 226, 88–94. doi:10.1016/j.matchemphys.2019.01.004
Siripala, W., Ivanovskaya, A., Jaramillo, T. F., Baeck, S.-H., and McFarland, E. W. (2003). A Cu2O/TiO2 Heterojunction Thin Film Cathode for Photoelectrocatalysis. Solar Energ. Mater. Solar Cell 77, 229–237. doi:10.1016/S0927-0248(02)00343-4
Sivula, K. (2021). Mott–Schottky Analysis of Photoelectrodes: Sanity Checks Are Needed. ACS Energ. Lett. 6, 2549–2551. doi:10.1021/acsenergylett.1c01245
Tilley, S. D., Schreier, M., Azevedo, J., Stefik, M., and Graetzel, M. (2014). Ruthenium Oxide Hydrogen Evolution Catalysis on Composite Cuprous Oxide Water-Splitting Photocathodes. Adv. Funct. Mater. 24, 303–311. doi:10.1002/adfm.201301106
Toe, C. Y., Scott, J., Amal, R., and Ng, Y. H. (2019). Recent Advances in Suppressing the Photocorrosion of Cuprous Oxide for Photocatalytic and Photoelectrochemical Energy Conversion. J. Photochem. Photobiol. C: Photochem. Rev. 40, 191–211. doi:10.1016/j.jphotochemrev.2018.10.001
Wang, S., Yu, W., Xu, S., Han, K., and Wang, F. (2021). Ammonia from Photothermal N2 Hydrogenation over Ni/TiO2 Catalysts under Mild Conditions. ACS Sustain. Chem. Eng. 10, 115–123. doi:10.1021/acssuschemeng.1c04931
Wick, R., and Tilley, S. D. (2015). Photovoltaic and Photoelectrochemical Solar Energy Conversion with Cu2O. J. Phys. Chem. C 119, 26243–26257. doi:10.1021/acs.jpcc.5b08397
Yang, Y., Niu, S., Han, D., Liu, T., Wang, G., and Li, Y. (2017). Progress in Developing Metal Oxide Nanomaterials for Photoelectrochemical Water Splitting. Adv. Energ. Mater. 7, 1700555. doi:10.1002/aenm.201700555
Yang, J., Du, C., Wen, Y., Zhang, Z., Cho, K., Chen, R., et al. (2018). Enhanced Photoelectrochemical Hydrogen Evolution at P-type CuBi2O4 Photocathode through Hypoxic Calcination. Int. J. Hydrogen Energ. 43, 9549–9557. doi:10.1016/j.ijhydene.2018.04.066
Keywords: titanium oxide, electrochemical deposition, heterogeneous nanostructure, cuprous oxide photocathode, p-n junction
Citation: Cao Y, Qiao H, Zou Y, An N, Zhou Y, Liu D and Kuang Y (2022) Room Temperature Electrodeposition of Ready-to-Use TiOx for Uniform p-n Heterojunction Over Nanoarchitecture. Front. Chem. 10:832342. doi: 10.3389/fchem.2022.832342
Received: 09 December 2021; Accepted: 10 January 2022;
Published: 22 February 2022.
Edited by:
Jin-Young Jung, Hanwha Q Cells, South KoreaReviewed by:
Fagen Wang, Jiangsu University, ChinaMalkeshkumar Patel, Incheon National University, South Korea
Copyright © 2022 Cao, Qiao, Zou, An, Zhou, Liu and Kuang. This is an open-access article distributed under the terms of the Creative Commons Attribution License (CC BY). The use, distribution or reproduction in other forums is permitted, provided the original author(s) and the copyright owner(s) are credited and that the original publication in this journal is cited, in accordance with accepted academic practice. No use, distribution or reproduction is permitted which does not comply with these terms.
*Correspondence: Deyu Liu, bGl1ZGV5dUBuaW10ZS5hYy5jbg==; Yongbo Kuang, a3Vhbmd5b25nYm9AbmltdGUuYWMuY24=