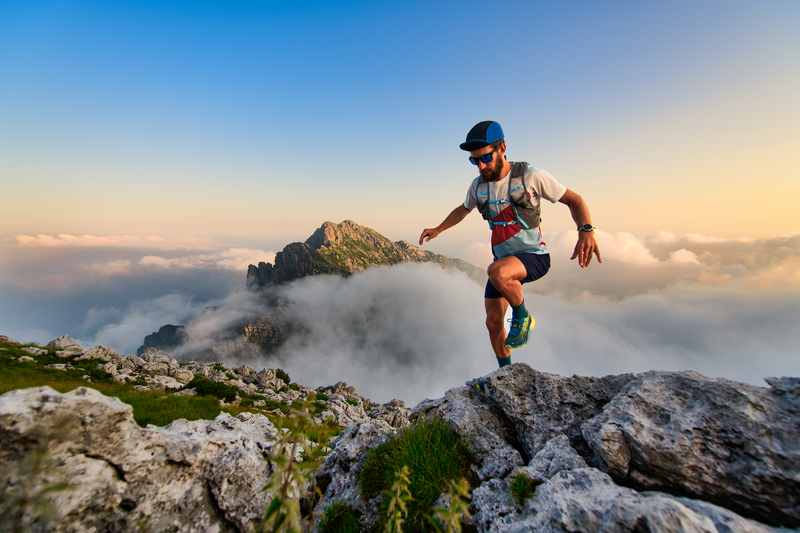
95% of researchers rate our articles as excellent or good
Learn more about the work of our research integrity team to safeguard the quality of each article we publish.
Find out more
REVIEW article
Front. Chem. , 14 February 2022
Sec. Electrochemistry
Volume 10 - 2022 | https://doi.org/10.3389/fchem.2022.827563
This article is part of the Research Topic Material and Structural Designs for Metal Ion Energy Storage Devices View all 14 articles
Flexible zinc batteries have great potential in wearable electronic devices due to their high safety, low cost, and environmental friendliness. In the past few years, a great deal of work on flexible zinc batteries has been reported, with exciting results. Therefore, many solutions have been proposed in electrode design and electrolyte preparation to ensure the desired flexibility without sacrificing the capacity. This paper reviews the recent progress of flexible zinc batteries. We discuss the differences between various anode materials, cathode materials, and electrolytes, introduce the differences of electrode preparation methods of active materials on flexible substrates and their influence on the performance of the battery. Finally, the challenges and future research trends of flexible zinc batteries in capacity and mechanical properties are pointed out.
With the development of miniaturization of electronic chips, it is possible to integrate electric devices, such as implantable medical devices, wearable health monitoring systems, flexible displays, and intelligent clothing, which have attracted the attention of researchers all over the world. (Yu et al., 2017; Liu et al., 2018; Sumboja et al., 2018; Zhang et al., 2018; Dong et al., 2021).As an indispensable part of the flexible electronic equipment, the battery needs to have good cycling and safety performance to meet the requirements. Especially when the flexible battery follows the deformation of the flexible electronic devices, the mechanical and electrochemical properties are required to be higher.
As an electrode material, zinc has the advantages of lower cost, more content in the crust and lower redox equilibrium potential than lithium. Moreover, using environmentally insensitive zinc makes zinc-based batteries manufacture easier and package cheaper than lithium-based batteries. (Fang et al., 2018). At present, conventional zinc-based batteries such as Zn-MnO2, Zn-Ni and Zn-Air have already been commercialized, but they are mainly rigid and used in non-flexible electronic devices. Many researches have been done to make these batteries flexible. In addition, Zinc ion batteries (ZIBs) because of its high energy density, low cost, environmental friendliness, safety and other advantages are gradually coming into people’s horizons, have good prospects in portable devices. ZIB, as a promising alternative to lithium ion battery, has attracted widespread attention. (Tan et al., 2017; Li et al., 2019a; Yu et al., 2019; Zhang et al., 2020a).
At present, great progresses have been made in electrode materials selection, flexible electrode preparation and electrolyte design of flexible zinc ion batteries. (Li et al., 2019a). In this review, the latest research progresses in electrode materials, electrolytes, and adhesion methods of active material are reviewed. Lastly, some concluding remarks were prospected to outline the challenges and future research trends for flexible zinc batteries.
Zinc metal anodes are of particular interest to the flexible battery market due to their low material cost, high theoretical capacity (820 mA h/g, 5,854 mA h/cm3), (Kaveevivitchai and Manthiram, 2016), good rechargeability and safe chemistry. The most common anode in flexible zinc ion battery is zinc foil, because of its good mechanical properties. However, the stiffness of heavy metal zinc foil anodes reduces the energy density, making it impossible to apply to industrial flexible, wearable energy storage systems. (Pan et al., 2016). In addition, the main challenges faced by zinc metal anodes are the mechanical stability of the electrode during long-term deformation and uncontrollable dendrite growth during cycling, resulting in poor cycle performance and coulomb efficiency of the battery, which severely limits the service life of flexible zinc ion batteries and hinders their practical application. (Lao-atiman et al., 2017; Han et al., 2018a; Li et al., 2018a; Ma et al., 2018; Tang et al., 2019; Zeng et al., 2019; Li et al., 2020a; Nguyen et al., 2020). In order to solve these problems of Zn anode, there are several strategies, including:
a) Method for constructing composite zinc anode.
Chen and his colleagues proposed a heterogeneous metal seed-mediated strategy. (Chen et al., 2021a). The basic idea is through the inkjet printing on the main chain of the three dimensional conductive printing silver nanoparticles, in order to induce the homogeneous nucleation of zinc, and avoid initial electroplating phase of the dendrite growth. Therefore, AgNPs@CC/Zn anode has excellent performance at a high current density of 10 mA/cm2, with cycle performance exceeding 480 h. (Figures 1A,B)
b) Deposition of zinc materials on a highly conductive substrate.
FIGURE 1. (A) Schematic diagram of Zn deposition on bare CC and AgNPs@CC scaffolds. (B) The capacity is 5 mA h/cm2 at 10 mA h/cm2 current density. Inset: SEM images of CC/Zn and AgNPs@CC/Zn electrodes after circulation. (C) Flexible AgNPs@CC/Zn electrode under bending.Copyright 2021 Wiley-VCH GmbH. (D) CV curves of the CC-CF@120ZnO at different cycles. SEM images of (E) CC-CF@ZnO and (F) CC-ZnO after cycling test, showing the importance of CFs for preventing the Zn dendrite growth. (G–I) Discharge curves and EIS results of solid-state batteries under different bending conditions. Copyright 2016 WILEY-VCH Verlag GmbH & Co. KGaA, Weinheim.
Zinc oxide nanoparticles are deposited on a three-dimensional layered carbon cloth-carbon nanofiber (CC-CF) substrate as an anode (CC-CF@ZnO). (Figures 1D,E) The device exhibits excellent stability, maintaining up to 91.45% initial capacity after 1,000 cycles and 72.90% initial capacity after 2,400 cycles. The significant increase in cyclic capacity is due to the uniform deposition of nano particles on the three-dimensional highly conductive nano-carbon fibers, which alleviates the shape change during the electrochemical reaction. In particular, it avoids the uneven distribution of current and zinc deposition, thus preventing the formation of zinc dendrite. (Liu et al., 2016).
Pang (Guo et al., 2021) prepared zinc nanosheets/CC (Zn/CC) by electrodeposition, and Zn nanosheets were uniformly deposited on the surface of CC fiber. Form a three-dimensional network interconnected with recent nano piece, insert for the ion-extraction process provides a rich active site. Compared with the same amount of conventional metal zinc anode foil, nanostructures zinc and CC skeleton integration enhances the flexibility of the anode, the formation of the surface of the more active, which helps the development of high performance flexible device.
c) Coating inert material on zinc anode.
For example, (Alfaruqi et al., 2018), constructed a Sn-doped NaTi2(PO4)3 (NTP/Sn) protective layer on the surface of zinc anode (Zn@NTP/Sn) to improve the cyclic stability of zinc anode. Using Sn doping NaTi2 (PO4) 3 through the structure design and high performance of zinc anode interface features, is helpful to accelerate the transfer of Zn2+, increase the interface performance. At the current density of 0.4 mA/cm2, the average voltage lag of Zn@NTP/Sn symmetric cells is only 17.4 mV, which is much lower than that of exposed Zn symmetric cells (75.8 mV) and Zn@NTP symmetric cells (43.7 mV). In addition, it has been reported that the zinc electrode surface was coated with Al2O3 coating (Lee et al., 2013) and Bi alloying (Jo et al., 2017) to improve HER overpotential and reduce self-discharge caused by hydrogen release, thus improving the cycle performance of the battery.
d) Use appropriate electrolytes.
For example, ionic liquid based zinc salt electrolyte is an effective way to solve the hydrogen evolution reaction (HER) and zinc dendrite growth of zinc ion batteries. (Ma et al., 2020) developed polyvinylidene fluoride hexafluoropropylene (PVDF-HFP)+5% poly (ethylene oxide) (PEO)+ILZE electrolyte (1-ethyl-3-methylimidazolium tetrafluoroboric acid ([EMIM]BF4) ionic liquid) using 2 M zinc tetrafluoroborate (Zn(BF4)2) as carrier. The final product is represented as PHP-ILZE. The electrolyte developed can achieve hydrogen-free, dendrite-free galvanizing/stripping in 1,500 h (3,000 cycles) at 2 mA/cm2 with a Coulomb efficiency of nearly 100%. At the same time, oxygen-induced corrosion and passivation were effectively inhibited. This is the first demonstration of an all-solid-state zinc-ion battery based on a newly developed electrolyte, which simultaneously solves the problems of deep hydrogen evolution and dendrite growth of conventional zinc-ion batteries.
Li and his colleagues have developed a polyethylene glycol 600 (PEG 600) and polysorbate 20 (Tween 20) compound additive, as the organic inhibitors in alkaline electrolyte, mainly by inhibiting the hydrogen evolution reaction to a certain extent, inhibit the corrosion of zinc. Because Tween 20 is more polar than PEG 600, it absorbs zinc better. Because of Tween 20 highly branched structure, zinc cannot be Tween 20 complete coverage, and linear polyethylene glycol (peg) can be adsorbed on the rest of the active site, thus two kind of corrosion inhibitor for zinc corrosion synergy effect, the corrosion of the composite inhibitor is better than single corrosion inhibitor. (Liang et al., 2011).
The combination of these methods enables advanced flexible zinc anodes to be better designed with less dendrite growth, higher corrosion and passivation resistance, and less hydrogen evolution, suitable for quasi-solid zinc-based aqueous batteries.
The cathode materials commonly used in flexible zinc ion battery include various transition metal compounds, such as manganese (Qiu et al., 2017; Huang et al., 2019; Zu et al., 2019; Zhang et al., 2020b; Zhang et al., 2020c), cobalt, nickel (Liu et al., 2016; Wan et al., 2018) or molybdenum-based oxides/sulfides, Prussian blue analogues and conducting polymers (Eftekhari et al., 2017; Yao et al., 2020; Cong et al., 2021). (Table 1) Here, we will focus on the last 5 years of research.
Manganese based compounds have been widely used as cathode materials for ZIB due to their advantages of abundant resources, low cost and non-toxicity. (Pan et al., 2016; Zhang et al., 2016). MnO2 is considered as the most promising cathode material among many manganese based compounds. MnO2 is a material with a polymorphic crystal shape. Under different preparation conditions, the crystal shape, morphology, particle size, porosity, specific surface area are different, and then show different capacity and charge-discharge characteristics. (Xu et al., 2012; Alfaruqi et al., 2018). Huang and his colleagues have provided new insights into the energy storage mechanism of Waterborne zinc-manganese battery with the participation of Mn2+. (Huang et al., 2019). In the first discharge process, the combination of Zn2+ and H+ insert promoted the MnO2 to ZnxMnO4, MnOOH and Mn2O3 transformation, at the same time, raised the pH of the electrolyte, formation of ZnSO4⋅3Zn(OH)2⋅5H2O (noted as “BZSP”). Later in the charging process, ZnxMnO4, MnOOH and Mn2O3 by extracting Zn2+ and H+ reduction for alpha MnO2, BZSP and Mn2+ reacts ZnMn3O7 ⋅3H2O. Besides the electrochemical reaction, Zn2+ can also be in alpha MnO2, ZnxMnO4 and ZnMn3O7⋅3H2O in the reversible insert and extract, under the participation of Mn2 +, BZSP, Zn2Mn3O8 and ZnMn2O4 reciprocal transformation (Figure 2B).
FIGURE 2. Phase evolution of cathode during the first discharge process (A) and charge process (B). Copyright 2019 Springer. (C)SEM images of the N-CC@MnO2; inset is the corresponding magnifed SEM image. (D) N-CC@MnO2//N-CC@Zn and CC@MnO2//N-CC@Zn the specific capacity and capacity retention rate of the battery as a function of current density (E) Cycling performance of the N-CC@MnO2//N-CC@Zn and CC@MnO2//N-CC@Zn batteries collected at 1 A/g for 1,000 cycles. (F) Capacity retention rate of the battery after 1,000 cycles at the current density of 1 A/g N-CC@MnO2//N-CC@Zn. The CV curves of the first and 1000th cycles are illustrated. (G) At different bending conditions N-CC@MnO2//N-CC@Zn battery capacity retention rate.Copyright 2017 Journal of Materials Chemistry A. (H) For the synthesis of MnO2-x @ VMG shell/core array. (HT, hydrothermal method; PP, phosphorization process). (I) Discharge curves of the device under flat, bending and twisting conditions at 3 A/g current density. Copyright 2020 WILEY-VCH Verlag GmbH & Co. KGaA, Weinheim.
On the other hand, the slow kinetics and rapid capacity decay caused by the strong electrostatic repulsion between the divalent zinc ions and the manganese based host crystal structure is one of the important challenges for the practical application of zinc ion batteries. Li’s team proposed in-situ growth of manganese dioxide nanorods arrays on three-dimensional porous surface nitrogen-doped carbon cloth (N-CC) (Figure 2C). It is not only better than the other nanostructures has higher specific surface area, more active site and better permeability, and can maintain the stability of the structure and electrochemical significantly improve the conductivity at the same time. In addition, it allows a strong covalent coupling based on the nanoscale active material and N-CC, leading to a fast electron transfer from the active material to the collector. Thus, Under the current density of 0.5 g/A, flexible-CC@ MnO2/N-CC@Zn battery can produce a high capacity of 353 mA h/g (Figures 2D,E). In addition, the CV curve of our assembled state N -CC@MnO2//N-CC@Zn battery hardly changes after 1,000 cycles, which also proves that the cell has good cycle stability. Further, the prepared devices can be bent and twisted in different states without affecting their electrochemical performance (Figures 2F,G). (Qiu et al., 2017)
In addition, many researchers believe that the oxygen vacancy generation and ion intercalation are another effective method to improve the electrochemical performance of Zn/MnO2 battery. There are two main reasons: On the one hand, as shallow bodies, oxygen vacancies can fundamentally improve the electrical conductivity of manganese-based materials, influence the embedding and de-embedding of metal ions in material layers, reduce the stress and electrostatic repulsion between adjacent layers, directly overcome migration and diffusion barriers, and promote the diffusion and charge transfer of ions during the embedding of metal ions; on the other hand, the electrode material with oxygen vacancy can also generate more electrochemically active sites, increase the surface energy of the system, promote the electrochemical phase transition, and thus possess better charge storage capacity. (Zu et al., 2019; Zhang et al., 2020b).
Zhang’s team reported a simple phosphorylation process that introduces oxygen vacancies into phosphate ion intercalated manganese dioxide/vertical multilayer graphene (VMG) arrays to form complete P-MnO2-x@VMG cathodes (Figure 2H). By phosphorylation, the oxygen vacancy and phosphorus ion intercalation are realized simultaneously, which improves the electrical conductivity of MnO2 and expands its layer spacing to accelerate ion transfer. In addition, the flexible VMG conductive network provides good peripheral charge transfer and imparts good mechanical strength to the cathode. Thanks to these advantages, at the current density of 0.5 A/g, P-MnO2-x@VMG cathodes shows a high capacity of 302.8 mA h/g in aqueous electrolytes, and the capacity retention rate is more than 90% after 1,000 cycles at the current density of 2 A/g, which proves its long-term cycling stability. In addition, by comparing the 3 A/g discharge curves of the battery under flat, bent and twisted conditions, it can be seen that the difference between the three curves is negligible, which proves that the battery has great potential in flexible wearable electronic devices. (Figure 2I). (Zhang et al., 2020c) Mai et al. reported the generation of oxygen vacancies in tunnel α -MnO2 via surface gradient Ti doping using defect engineering for long-life Zn-MnO2 batteries. (Lian et al., 2019). Interestingly, the introduction of surface gradient Ti doping leads to the contraction of the interlayer, but at the same time, the reduction of Mn valence state leads to the formation of oxygen vacancies compensated by electrons. In addition, Ti substitution and the resulting oxygen vacancy open the [MnO6] octahedral wall, resulting in an unbalanced charge distribution and local electric fields in the crystal structure, accelerating ion/electron migration rates. Therefore, the diffusion coefficients of Zn2+ and H+ in Ti-MnO2 nanowires are improved. Therefore, Ti-MnO2 nanowires show improved H+ and Zn2+ storage capacities in Zn/MnO2 batteries, and achieve excellent high rate capacity and ultra-long cycle stability.
Also, in ZnSO4 electrolytes widely studied, MnO2 usually suffers volume loss due to Mn3+ disambiguation dissolving Mn2+ 39. Mn2+ is added to the electrolyte to inhibit the disproportionation of Mn3+, which can effectively inhibit the dissolution of manganese base materials and improve the stability of cathode materials (Pan et al., 2016; Chamoun et al., 2018), but the mechanism is still unclear. For example, in a Zn-MnO2 cell, dissolution of α -MnO2 is alleviated when MnSO4 is added to a ZnSO4 electrolyte because it can alter the dissolution balance of Mn2+ from the α-MnO2 electrode. (Lian et al., 2019). Zhang and his colleagues reported MnO2 based anode and cathode, zinc added Mn(CF3SO3)2 additives of Zn(CF3SO3)2 electrolytic Zn-MnO2 water of high performance rechargeable batteries. It is found that Mn(CF3SO3)2 can inhibit the dissolution of Mn2+ and form uniform porous MnOx nanosheets on the cathode surface, which helps to maintain the integrity of the electrode. It is important to note that beta MnO2 has the high irreversible capacity, stable high rate capability and cycle performance. (Zhang et al., 2017). In addition, coating can also be used to alleviate the dissolution of mangan-based materials. For example, Yang et al. prepared an independent flexible film (Designated as CNT/MnO2-PPY) composed of carbon nanotubes and polypyrrole coated manganese dioxide nanowire through in-situ reactive self-assembly and vacuum filtration. The Polypyrrole (PPy) coating improves the conductivity of MnO2 NWs, alleviates the dissolution of cathode materials and provides a strong buffer to accommodate large volume changes during repeated cycles. (Zhang et al., 2020d).
Zinc-silver batteries have the following advantages: high specific energy (theoretical specific capacity: 432 mAh/g), high discharge efficiency, moderate charging efficiency, smooth discharge voltage, small self-discharge rate, long dry storage life and good mechanical properties. (Kumar et al., 2019; Tan et al., 2018). At the same time, zinc-silver batteries also have obvious shortcomings, which are as follows: very high cost, short life, poor low-temperature performance and not resistant to overcharging. The silver oxide button battery has widely used in electronic watches, calculators, small instruments and other micro electrical appliances as power supply because of their above-mentioned advantages. In recent years, a lot of research has been done to make these batteries flexible. In addition, Wang’s team has developed a printable, polymer-based zinc-silver oxide (AgO-Zn) battery with flexibility, rechargeability, high area capacity and low impedance. The redox reactiondepends on the zinc ions (Zn2+) and silver ions dissolved in alkaline electrolyte (Ag) and the supersaturated induced precipitation. In addition, due to the high oxidation state, make full use of line printing batteries can realize >54 mA h/cm2 area of high capacity (Figure 3A) while maintaining a low internal resistance (Figure 3B). The battery can still charge and discharge normally without multiple deformations affecting its rechargeability (Figures 3C,D). Overall, printed thin film AgO-Zn thin film batteries combine superior electrochemical and mechanical properties and prove to be well suited for reliably and sustainably powering a wide variety of wearable and flexible electronic devices. In addition, Meng and his colleagues used polystyrene block polyisoprene block polystyrene (SIS) as a hyperelastic adhesive for printing inks to print a Zn-Ag2O battery with high ductility and a reversible capacity density of 2.5 mA h/cm2 even after repeated 100% stretching.Two stretchable battery with “NANO” design printed directly on the seal on the spandex thermoplastic polyurethane (PU) the top of the head (Figure 3E). On the “NANO” current collector, electrodes are printed separately to form two “NA” and “NO” batteries designed to be connected in series to power the 3V wearable LED (Figure 3F). Regardless of the serious torsional strain (Figure 3G), indentation (Figure 3H), 100% uniaxial tensile (Figure 3I) and biaxial stretching, stretching “NANO” to the battery can keep constant LED brightness (Figure 3J). The battery energy provided so far reported the highest reversible capacity of the inherent scalability battery size and discharge current density. New stress wear-resisting printing inks for the wide application of flexible paved the way to the further development of the electronic products. The new printed battery based on SIS can withstand severe bending caused by the wearer’s movement. (Kumar et al., 2016).
FIGURE 3. (A) Electrochemical performance of AgO-Zn batteries as galvanic cells. The active material load is the capacity of the 2 × 2 cm2 battery with 1-8 layers. (B) Bode plot reflecting the corresponding impedance of the 2 × 2 cm2 cells with different areal loading. (C) Diagram of a cell controlled by a linear stage at a speed of 15 s/cycle under repeated 180° bending cycles, and (D) corresponding volt-time curves for charging (red) and discharging (blue) of the cell during approximately ∼2,500 repeated bending cycles. Copyright 2020 ResearchGate. (E) Screen printing steps for Zn-Ag2O cells on stretchable fabric using SIS adhesive. Illustration: REDOX charging-discharging reaction. Photographs of the sealed cell during (F) horizontal stretching, (G) distortion, (H) indentation strain, (I) 100% stretching and (J) biaxial stretching. Copyright 2016 Advanced Energy Materials.
Compared with metal oxides, conductive polymers exhibit higher conductivity due to their long π -electron conjugated system (Shi et al., 2018), and also has the advantages of low cost, easy synthesis, and excellent mechanical properties (Cong et al., 2021), are excellent cathode materials for Zn batteries, and show promising energy storage behavior. Therefore, conductive polymers have attracted wide attention in the field of electric energy storage, especially in wearable electronic devices. Polyaniline (PANI) is one of the most common type of conductive polymers. Polyaniline is easy to synthesize and has high electrical conductivity and electrochemical activity. (Eftekhari et al., 2017). It as a stream of zinc ion battery cathode material has been widely studied. However, it suffers from rapid deactivation and consequent performance deterioration due to spontaneous deprotonation during charging/discharging, and has the disadvantage of poor cycling stability in high pH solutions, which limits its further application in polyaniline zinc batteries. Therefore, Yang’s group reported a strategy to introduce [Fe (CN)6]4-, in which the nitrogen atoms on the polyaniline chain may also interact with [Fe(CN)6]3-/[Fe(CN)6]4- to affect the electrochemical stability of polyaniline. Considering these factors, [Fe(CN)6]3-/[Fe(CN)6]4- and polyaniline chain between the REDOX reaction and hydrogen bonding may be beneficial to the electrochemical properties of polyaniline, which can greatly improve the cycle stability of the polyaniline electrode, at the same time maintain its initial high specific capacity (Figure 4A). After 1,000 times charging and discharging cycle, CC–PANI–FeCN capacity retention rate of about 71%, and the capacity of the CC–PANI retention rate is only 17% (Figures 4B,C). Assembly of quasi solid flexible zinc ion battery under the condition of different bending the specific capacity and coulomb efficiency is close to 100%, suggest that the potential of as flexible energy storage device (Figure 4D). (Yao et al., 2020)
FIGURE 4. (A) REDOX mechanism of CC-PANI-FeCN. Illustration[Fe(CN)6]4− Schematic diagram of interaction with PANI. Cyclic properties of CC-PANI (B) and CC-PANI-FeCN (C) at current density 5 A/g. (D) The optical images ofquasi-solid-stateflexible batteries in different bending states. Copyright 2020 Chemical Engineering Journal. (E) Cycling performance of t-CNT-PA-PE cathode in ZIBs at current density of 10 A/g. Capacity retention of solid ZIBs under different destructive tests, including (F) bending test and (G) hammer testCopyright 2019 ACS Appl Mater Interfaces.
In addition, Huang’s team reported a method to improve the electrochemical reactivity and stability of the polyaniline cathode by constructing a pile-electron conjugate system between PEDOT: PSS on polyaniline and carbon nanotubes. The cathode can provide a high capacity of 238 mA h/g at a current density of 0.2 A/g, with good rate performance and good cycle stability (Figure 4E). Zinc ion batteries based on post-treated CNT PANI PEDOT: PSS (t-CNTs-PA-PE)showed good cycle stability and almost 100% cycle efficiency in 1,500 cycles. Moreover, Solid ZIBs in the bending and hammering process, the capacity loss is not obvious, keep in more than 90% of the original capacity (Figures 4F,G). This work demonstrates that conductive polymer cathodes can be used in high-performance ZIBs to meet the needs of flexible electronics. (Liu et al., 2019a).
PBA with the formula of AxMy [B(CN)6]zmH2O (x, y, z, m = sto-ichiometric numbers; A, B = alkaline metal; M = Zn2+, Ni2+, Cu2+, etc.) is constructed by a 3D network of zeolitic struc-ture. (Xu et al., 2017). It has open skeleton structure, sufficient REDOX active center and relatively strong structural stability (Jia et al., 2015; Zhang et al., 2015; Song et al., 2018). The open framework endows PBA to be promising candidate for Zn2+ storage. However, Prussian blue analogues have a low capacity (less than 100 mAh/g) because the active sites are underutilized and in most cases are single-atom REDOX (Ma et al., 2019a). It is still challenging to further improve the specific capacity and cycling stability of PBA cathodes.
Lu and his colleagues prepared manganese oxide coated zinc ferrite (ZnHCF) nanocubes (ZnHCF@MnO2) by in situ coprecipidation method (Lu et al., 2017). The composite has a unique structure that has a synergistic effect by combining the capacitive and intercalated properties of the two components with the REDOX reaction, thus regulating the storage of Zn ions. Therefore, ZnHCF nanocubes encapsulated by MnO2 nanocubes have a high capacity of Zn ion storage and discharge, and their working voltage can reach ∼1.7V. In order to demonstrate the practical application of zinc ion battery in the field of flexible wearable electronics, a flexible quasi-solid-state battery was prepared by coupling ZnHCF@MnO2 with Zn thin foil in ZnSO4/PVA gel electrolyte. At different bending angles, the battery’s current changes are negligible, showing excellent flexibility (Figure 5A). In addition, the discharge capacities of the flexible device at 100, 200, 400, 500 and 800 mA/g are 89, 78, 67, 58, and 53 mAh/g, respectively (Figure 5B). In addition to higher discharge capacity, quasi-solid state batteries also have higher rate capacity (Figure 5C). When the current density increases from 100 mA/g to 800 mA/g, the discharge capacity can still exceed 49 mA h/g, and the capacity retention rate is 55%. At the same time, flexible battery can be stable cycle more than 500 times, can maintain about 71 percent of the capacity. Even flexible battery folding can power LED bulbs (drive voltage is 1.8V). (Figure 5D).
FIGURE 5. Performance of flexible solid-state rechargeable zinc ion battery with ZnHCF@MnO2 nickel foil as cathode (A) CV curves under different bending conditions at 1 mV/s; (B) Charge-discharge curves under different current densities; (C) Multiplier performance of flexible cells; (D) Photographs of the flexible zinc batteries powering the LED bulbs in their original and cut state respectively Copyright 2017 J. Mater. Chem. A. Electrochemical performance of Zn/CoFe(CN)6 cells with 4 m Zn(OTf)2 electrolyte (E) constant current charge-discharge curves under different current densities; (F) Solid-state cable battery: constant current charge-discharge curve and torsional deformation of battery at different bending angles of 60° and 150° when the fixed device length is 10 cm and bending radius is 1 cm.
Zhi and his colleagues incorporated Co(II)/Co(III) and Fe(II)/Fe(III) REDOX reactions into cobalt hexocyanate (CoFe(CN)6), which is a breakthrough to realize the combination of high capacity and high voltage water-bearing zinc ion batteries. (Ma et al., 2019a). The Zn/CoFe(CN)6 battery utilizes two pairs of Co(II)/Co(III) and Fe(II)/Fe(III) REDOX reactions to provide a high operating voltage platform of 1.75 V and a high capacity of 173.4 mA h/g at 0.3 A/g. The 3D open structure of the battery provides A sufficiently high discharge capacity of 109.5 mA h/g, even at an extremely fast charge/discharge rate of 6 A/g. (Figure 5E). In addition, the Zn/CoFe(CN)6 battery achieved excellent cycle performance of 2,200 times and coulomb efficiency of nearly 100%. Furthermore, sol-gel of hydrogel electrolyte has been developed to prepare high-performance flexible cable batteries. The battery has excellent electrochemical performance, uniform torsional deformation and excellent mechanical properties at bending degrees of 60° and 150°. (Figure 5F). This strategy enables the active material to fully contact the electrolyte, thus improving the electrochemical performance (capacity increase of ≈18.73%) and mechanical stability of the solid-state device.
Vanadium cathode because of its excellent REDOX chemistry, can promote the open of insert/take off layer response the main structure and high specific capacity and aroused people’s great interest. (Wang et al., 2017; Guo et al., 2018). Among various vanadium based oxides, V2O5 has good potential as ZB cathode material due to its high theoretical capacity (589 mA h/g) based on double electron transfer. The main challenge facing V2O5 cathode is that the V2O5 cathode synthesized by traditional methods has insufficient active sites, low conductivity, making its ultra-fast charging performance and high rate performance far below expectations.
Mai and colleagues designed a novel cathode material by growing two-dimensional V2O5 nanosheets directly on a flexible titanium (Ti) substrate. (Javed et al., 2020). The resistance of 2D V2O5 nanosheets is greatly reduced and provides more active sites to facilitate electrochemical reactions in zinc ion batteries. Meanwhile, the improvement of the electrochemical performance of V2O5-Ti cathode is due to the unique structure of vertically arranged ultra-thin nanosheets (Figure 6A) which ensures that Zn2+ has a larger embedding space and a shorter diffusion length, thus improving the electrochemical performance and providing ultra-fast charging performance. An ultra-fast and flexible quasi - solid F-V2O5-Ti//Zn battery was developed. The capacity, power density and energy density parameters are better than those of the previously reported water-based/flexible zinc ion battery. (Figures 6B,C).
FIGURE 6. (A) High power FESEM images of V2O5 nanosheets grown directly on titanium substrates, (B) Charge-discharge curves of at various current densities in potential window of 0.3–1.6 V, (C) Charge-discharge profiles during different bending states at constant current density of 12 A/g. Copyright 2020 Elsevier Ltd. (D) Discharge GITT curves at a current density of 0.05 A/g,(E) Cycling stability and Coulombic efficiency at 1 A/g. Inset in (E) shows the blue LED light up by two flat or bending state flexible quasi-solid-state Zn/E-VO batteries. Copyright 2019 NANOEN 3724. (F) Charge/discharge curves of the DVOC sample at different current densities. (G) Charge/discharge curves of the DVOC sample during the initial thirty cycles. (H) High-rate long-term cycling properties of the DVOC sample at 10 A/g. Copyright 2020 ChemSusChem.
Yan and colleagues propose a simple in situ method that simultaneously introduces polyvalence to increase the interlayer water content and enlarge the interlayer distance of hydrated V2O5. (Zhao et al., 2019). These structural adjustment makes the layered expansion V2O5 2.2 H2O (E-VO) nano piece has faster charge transfer kinetics, Zn2+storage space and more than precursor V2O5 higher structural stability. (Figure 6D). As cathode of water-based ZIB, E-VO has the high irreversible capacity (450 mA h/g at 0.1 A/g), good ability of ratio (222 mA h/g at 10 A/g) and long term stability (72% of the capacity to keep 3,000 cycle, at 5 A/g). (Figure 6E).
In addition, the structure crushing and chemical dissolution during the charge-discharge cycle are the main reasons for the electrochemical instability of vanadium oxide. (Yu et al., 2015; Song et al., 2016). In order to solve these problems, regulating the V4+ and the ratio of the V5+ to enhance the electrochemical reaction of reversibility, effectively improve the capacity and stability of the vanadium oxide. An oxygen defect modulated unbonded V2O5 nanorods (PVO@C) for water/quasi-solid zinc ion batteries were constructed. (Liang et al., 2021). The structure of PVO@C electrode is stable due to oxygen deficiency and phosphorus doping, and the diffusion rate and electronic conductivity of Zn2+ ions are improved. Aqueous PVO@C//Zn cells have significantly increased capacity (362.01 mA h/g at 0.13 A/g1) and have remarkable long-term durability (86.7% capacity after 5,000 cycles and nearly 100% Coulomb efficiency) compared to the original VO//Zn or PVO//Zn cells. In addition, a stable flexible quasi-solid PVO@C//Zn cell (SS ZIB) was proposed based on flexible PVO@C cathode, elastic Zn anode and PAM gel electrolyte. This SS ZIB device achieves an impressive energy density of 10.5 mWh/cm3 at a high power density of 33.4 W/cm3, exceeding most solid-state batteries previously recorded. After 600 cycles, it has a capacity retention capacity of 81.9%.
Vanadate based materials have the advantages of various element valence states, large theoretical capacity, stable structure and abundant resources, so they are the most widely studied. (Li et al., 2020b; Liao et al., 2020). However, due to strong electrostatic interaction with divalent zinc ion the vanadate cathode has slow kinetics and poor cyclic stability. Deng and colleagues (Lin et al., 2020) constructed a novel vanadate oxide structure and successfully designed a defection-rich (V6O13-δ)/C nanovortex to prepare a fibrous flexible DVOC/SWNT@CNTF electrode (SWNT: single-walled carbon nanotube; Carbon nanotube fiber CNTF was prepared). Based on SWNT network and substrate are provided based on CNTF bicontinuous electronic path, promoted the fast dynamics and excellence rate capability (Figures 6F–H).
In addition, the pre-insertion strategy is also an effective method to improve the cycling performance of vanadium-based zinc ion batteries. Linda F. Nazar and colleagues report a vanadium oxide bronze supported by interlaminar Zn2+ ions and water (Zn0.25V2O5•nH2O) as the positive electrode of a zinc battery. (vKundu et al., 2016). A reversible Zn2+ ion (de) intercalation storage process with high speed and more than one Zn2+ per formula unit (capacity up to 300 mAh/g). The zinc battery has an energy density of 450 W/L and a capacity retention rate of more than 80 percent in 1,000 cycles. No dendrites form on the zinc electrode. In order to solve the problem, the preintercalated ions can easily lose the combination with the skeleton and deintercalated into the electrolyte, leading to the collapse of the structure again.Xu reported that the cyclic stability of a zinc ion cell can be improved by preintercalation of tetrapalent tin ions into Pyrovanadate Sn1.5V2O7(OH)2•3.3H2O (denoted as SnVO). (Xu et al., 2021). Compared with Pure vanadium pentoxide, the tetradvalent tin in SnVO can strongly bind to V2O74− layer, supporting high mechanical stability during zinc ion intercalation. In addition, the tin oxide tetrahedron in the V4O10 layer can further expand the size of the cavity between Pyrovanadate V2O74−, promoting the rapid kinetics of zinc ion diffusion, thus improving the rate performance of ZIB.
Electrolyte is another important component of flexible zinc ion battery, which is the “blood” of flexible zinc ion battery and plays an important role in determining its performance in terms of discharge operating time, cycling performance and shelf life. There are two types of electrolytes, one is liquid electrolyte (Hilder et al., 2009), which has a certain disadvantage compared with gel electrolyte because of its fluidity and is prone to side leakage when encapsulating flexible batteries. Next is the solid electrolyte, which contains three main types: hydrogel, ionic conductive inorganic solid and organic polymer. Gel polymer electrolyte is a kind of intermediate state between liquid and solid, which is composed of polymer object and liquid electrolyte. Due to its relatively high ionic conductivity, flexibility and good interfacial contact with electrodes, it has been widely used in flexible zinc ion batteries. In general, the performance of gel electrolytes depends largely on the choice of gelatin and the ratio of each element. The gelatins used to build electrolytes are generally polyvinyl alcohol (PVA), polyethylene oxide (PEO), and polyacrylic acid (PAA) (Li et al., 2019a). Polyvinyl alcohol (PVA) is a commonly used polymer matrix with good water retention capacity, which has the characteristics of high hydrophilicity and good film forming ability, excellent chemical stability, electrochemical inertness, durability, non-toxicity and ease of manufacture. (Li et al., 2019b; Fan et al., 2019).
As the evaporation of water, the electrolyte becomes more and more concentrated and the performance of battery deteriorates. To reduce water loss of the electrolyte, thereby improving the ionic conductivity of the electrolyte and the cycle life of battery, Zhong’s team has proposed a polymer electrolyte for the body that uses tetraethylammonium hydroxide (TEAOH) as an ionic conductor and polyvinyl alcohol (PVA) as a polymer, with good water retention (Figures 7A,B). The prepared polymer electrolyte maintained a high ionic conductivity of 30 mS/cm after 2 weeks. In addition, with the commonly used KOH-PVA electrolytic liquid ratio, TEAOH - PVA assembly zinc air battery has excellent discharge properties and cycle life, found no significant degradation in 2 weeks. (Li et al., 2019b).
FIGURE 7. Optical photos of (A) freshly prepared electrolytes and (B) electrolytes stored in the open ambient environment (RH = 30%, 20°C) for 72 h.Copyright 2019 Nano Energy. (C) Photographs of PHEs with polymer concentration ranging from 30% (w/w) to 50% (w/w) at varied temperatures, showing the thermoreversible gelation behavior. Copyright 2017 Angewandte Chemie International Edition. (D) Rate performance, (E) GCD curves at 0.2 A/g. Copyright 2021 Wiley-VCH GmbH.
In addition, the internal pores of the gel electrolyte play a key role in the absorption of electrolytes, electrolytes can be retained in the porous gel electrolytes, and polyethylene glycol as a pore-forming agent improves the doping ability and obtain high ionic conductivity. The prepared porous photovoltaic nanocomposite GPE has high ionic conductivity of 57.3 mS/cm, excellent water retention performance and good thermal and mechanical properties under environmental conditions, while the assembled flexible ZAB has excellent cycle stability, discharge performance and power density. (Fan et al., 2019).
In addition to the ionic conductivity and mechanical strength of the hydrogel, certain properties of the polymer electrolyte may have a particular impact on the performance of flexible zinc ion batteries. (Li et al., 2018b). For example, Cui’s team reported a novel strategy using thermally reversible polymer hydrogels as functional electrolytes, i.e., using poly (ethylene oxide)-poly (propylene oxide)-poly (ethylene oxide) (PEO-PPO-PEO), with significant structural polymorphism in polar solvents, to provide intelligent cooling recovery for flexible zinc ion batteries. When the cell system is exposed to extreme deformation, a simple cooling process can repair the fractured electrode-electrolyte interface, resulting in in-situ recovery of electrochemical performance compared to conventional flexible cells (Figure 7C). This process can be repeated even after multiple strong folding events, and this cooling-recovery strategy requires only external temperature stimulation without any side effects involving phase transitions. (Zhao et al., 2017).
Soorathep Kheawhom’s group also reported a transparent alkaline GPE film, which is polymerized by polyacrylic acid, potassium hydroxide and water as a quasi-solid electrolyteused in zinc-manganese dioxide batteries. The GPE film has high ionic conductivity, improveing the cycle life and performance of the battery. (Gaikwad et al., 2011; Lao-atiman et al., 2017).
The application of organic electrolytes in zinc ion batteries is still the least explored field in stable zinc anodes. Due to the higher thermodynamic stability of Zn to organic solvents, the application of organic solvents may reduce side reactions that may solve the lower Coulombic efficiency (CE) problem by simplifying electrochemistry at the Anode and reducing electrode passivation, thereby reducing dendrite problems.
Wang and colleagues reported that a highly stable and dendrite-free zinc anode was obtained by using triethyl phosphate (TEP) as a cosolvent in aqueous solution. In more than 3,000 h stable galvanized iron/zinc, CE value of 99.68%. The electrolyte and zinc ion battery of zinc anode and six iron acid potassium cyanide copper (KCuHCf) positive electrode showed a good compatibility. The full battery has longer cycle stability and higher rate performance. (Naveed et al., 2019).
However, compared with stream electrolytes, organic electrolytes are less safe, so stream electrolytes are the most widely studied. The flexible zinc ion battery also requires that the electrolyte can withstand the test of bending and folding, and has a certain self-healing ability.
Wong and colleagues developed a simple and economical method to construct a versatile hydrogel electrolyte using cotton as raw material, tetraethyl orthosilicate as crosslinking agent, and glycerin as antifreeze agent (Chen et al., 2021b). The resulting hydrogel electrolyte has high ionic conductivity, excellent mechanical properties (such as high tensile strength and elasticity), ultra-low freezing point, good self-healing ability, high adhesion and good heat resistance. It is worth noting that this water gel electrolyte under - 40°C can provide a record of 19.4 mS/cm high ionic conductivity. Secondly, the hydrogel electrolyte can significantly inhibit zinc dendrite growth and hydrogen evolution side reactions at -40–60°C (Figures 7D,E). Using this hydrogel electrolyte, a flexible quasi-solid Zn-MnO2 cell was assembled, which showed significant energy density in the -40–60°C range. The battery also shows excellent cycle durability, with high durability in a wide range of harsh conditions. In addition, it can be in >60% of compression deformation restored to its initial state, shows that it has good elasticity. Zhi’s team reported cathodes based on nanofibrillated cellulose (NFC)/polyacrylamide (PAM) hydrogels, electrodeposited Zinc nanoplate anodes, and carbon nanotube (CNT)/α-MnO2 sewable Zn-MnO2 cells (Wang et al., 2018a). The designed NFC/PAM hydrogel has high mechanical strength and great tensile properties. The prefabricated NFC bone network stabilizes the large pore as a channel for electrolyte diffusion. In addition, the influence of sewing on improving shear resistance of solid battery was analyzed. The sutured Zn-MnO2 battery retains 88.5% capacity after 120 stitches and can withstand a large shear force of 43 N.
In order to effectively solve the disadvantage of rapid water loss of hydrogel electrolyte, Liu and colleagues report a solid-state battery based on a self-standing gelatin-based hydrogel electrolyte (GHE) (Han et al., 2018b), whose high ionic conductivity, strong mechanical strength, and tight contact with the electrode enable reversible and stable circulation of Zn metal anodes and LiMn2O4 cathodes. Benefiting from superior stability to water, GHE’s flexibility and a carefully designed battery structure, the Zn/GHE/LiMn2O4 solid state full battery delivers a high specific capacity of 110.2 mA h/g and is resistant to cutting, flooding, bending, twisting and crimping. These characteristics of gelatin electrolyte proved beneficial to the battery components and battery performance. When heated, gelatine powder dissolved in AE, which promoted in-situ coating of GHE on the electrode. Using the fast cooling technology after cooling, gelatin solidifies into a solid layer of thin film, to provide mechanical strength to suppress the formation of zinc dendrite.
In a word, the research of flexible zinc ion battery electrolyte mainly focuses on how to alleviate the problem of zinc negative dendrite, hydrogen evolution and solve the problem of hydrogel electrolyte water loss. High performance electrolytes have great influence on the cycle stability, rate performance and energy storage capacity of batteries. (Liu et al., 2019b; Ma et al., 2019b). Therefore, it is of great significance to study electrolytes of flexible Zn-ion batteries.
In order to improve the mechanical flexibility of electrodes for wearable electronic devices so that the active material can better adhere to the flexible substrates, flexible electrodes are usually prepared by two ways: one method is to grow the active material directly on the flexible substrate, which is typically carbon cloth, polymer elastomers or textiles. Direct growth of active materials without using any binder, such as catalytic in-situ growth, electrodeposition (Liu et al., 2020; Yza et al., 2022), etc. Another method is to load active material with the help of instrument. This includes loading of electrically active material onto flexible substrates by screen printing with the help of adhesives, or loading the active material by inkjet printing, 3D printing, or laser etching with a pre-designed electrode pattern.
In the preparation of zinc ion batteries, the use of polymer binders and conductive additives results in high contact resistance, which reduces the specific capacity and diversity performance of the battery. An effective strategy to solve this problem is to directly grow the active material in-situ on flexible substrates or collectors as binder-free electrodes. (Wan et al., 2018). This strategy not only ensures fast charge transfer between the active material and the collector, but also enables a uniform distribution of the active material over the collector fluid. Therefore, the prepared flexible electrodes will provide better electrochemical performance, including high capacity, good rate performance and high capacity under deformation conditions.
Yao’s team constructed a planar flexible quasi-solid aqueous rechargeable silver-zinc battery using silver nanowires made from a metal-organic framework (MOF) as an unbonded cathode on a carbon cloth. The results show that the ag-Zn battery has a significant energy density of 1.87 mWh/cm2 due to the abundant reaction sites and short electron and ion diffusion paths provided by the MOF Silver nanowires (Figure 8A). After bending 135° and cycling 100 times, its capacity remained above 93% (Figure 8B), which further demonstrates the superb mechanical properties of the prepared devices (Figure 8C). (Li et al., 2018c) In addition, Cai and colleagues prepared three-dimensional (3D) integrated binder-free dual-functional oxygen electrodes composed of NiCo2O4@NiCoFe-hydroxide (NiCo2O4@NiCoFe-OH) nanoarrays supported by carbon cloth (Figure 8D). The three-dimensional porous structure and good hydrophilicity of the nitrate-treated carbon cloth substrate facilitated the directional growth of NiCo2O4 nanocrystals. Therefore, the nickel-iron-hydroxide can randomly anchore and cover on the NiFe surface. Using this controllable and cost-effective in-situ synthesis strategy, the core/shell structure of NiCo2O4@NiCoFe-OH nanoarrays is tightly attached to the 3D interconnected carbon microfibers. (Figure 8E).The manufactured monolithic binderless oxygen electrode is freestanding and highly flexible/bendable, inheriting a high mechanical strength carbon skeleton. (Li et al., 2020c).
FIGURE 8. (A) SEM of MOF derived Ag nanowires/CC at Ar/NH3. (B) Normalized capacity of the quasi-solid-state Ag-Zn battery bent 135°for 100 cycles. (C) The photographs of bent quasi-solid-state Ag-Zn battery.Copyright 2018 American Chemical Society. (D) Scheme of the synthetic process of NiCo2O4@NiCoFe-OH. SEM images of NiCo2O4 NWs (E) grown on CC. Copyright 2020 Journal of Materials Chemistry A.
Many flexible batteries rely on complex, low-production and high-cost manufacturing processes that hinder their transition from the lab to the marketplace, so printed high-performance batteries offer ideas for mass production of flexible batteries. Screen printing is considered to be a cost-effective, easy-to-operate and mass-productable method for the rapid construction of flexible zinc ion cells with precise control performance, flexibility and integration with printed microelectronics. (Guo et al., 2018). Screen printing allows active design control and can potentially combine deterministic and stochastic composites. In order to meet the need for flexibility and scalability while maintaining low cost and using low-cost thick film manufacturing techniques, flexible cell modules can be printed sheet by sheet or roll by roll using traditional and low-maintenance screen printing or scraper casting equipment, thus achieving low-cost mass production of flexible batteries (Figures 9A,B). (Kumar et al., 2016; Yin et al., 2021)
FIGURE 9. (A) The process of assembling the flexible, rechargeable, and high-capacity AGO-Zn battery is silkscreen printed layer by layer and vacuum sealed. Copyright 2019 ResearchGate. (B) Zn//MnO2 planar MBs was prepared by printing. Schematic diagram of printing zinc//MNO2MB by screen printing method: Blank PETsubstrate, printing graphene collector electrode, printing MnO2 cathode and Zn anode. Electrochemical performance of printed Zn//MnO2 planar MBs (C) cycling stabilities of printed Zn//MnO2 MBs with planar and sandwich-like stacked geometries, measured at a rate of 1 C; (D) long-term cycle stability of Zn//MnO2 planar MBs, with a high rate of 5C over 1,300 cycles. Copyright 2020 Natl Sci Rev.
Wu’s team reports on a cost-effective and industrially applicable screen printing strategy for the rapid and scalable production of rechargeable zinc-manganese dioxide planar batteries with high performance, superior flexibility, scalable applicability and high safety. With zinc ink as anode (6.4 μm thick) and MnO2 ink as cathode (9.8 μm thick), high-quality graphene ink was used as metal-free collector in neutral electrolyte (2 M ZnSO4 and 0.5 m MnSO4). Planar unbaffled Zn//MnO2 MBs, tested in neutral aqueous solution, has a high capacity of 19.3 mA h/cm3 (corresponding to 393 mA h/g) at a current density of 7.5 mA/cm3, and a significant volume energy density of 17.3 mWh/cm3, superior to lithium film battery (≤10 mWh/cm3). In addition, the battery has stable cycling performance, with a capacity retention rate of 83.9% after 1,300 cycles under 5C, which is better than the previously reported stacked Zn//MnO2 battery (Figures 9C,D). (Wang et al., 2020)
Screen printing solves the requirement of high volume production of flexible electrodes at low cost, but faces another difficulty, which is to select or develop the ink formulation and choose the mesh size, as well as the thickness of each layer of ink film forming the anode and cathode, according to the requirements of the amount of active material, electrical properties, electrode stability and electrolyte permeability. (Tehrani et al., 2015). The selection of the mesh is based on the particle size of the mass of active material to be printed. A mesh with too large an aperture loses the significance of screen printing, while a mesh with too small aperture leads to uneven printing of the active material, which in turn affects the electrochemical properties. The right printing template will allow the active substance to be printed more evenly and firmly and to meet the required amount of active substance.
The rheology of the ink is controlled by a complex formulation of reactive materials, binders and specific solvents. The role of the binder is to bond the ink components and the collector fluid together and to influence whether the active substance will come off. Ink with different ratios of the same active material can also have a great impact on the battery performance. Somenath Mitra’s team reported the preparation of printable composite electrodes embedded with multi-walled carbon nanotubes (CNTs) for flexible Ni-Zn batteries. (Wang et al., 2018b). Carbon nanotubes function for electron transport pathways, resulting in better performance than physical mixing of active materials. However, excessive amounts of carbon nanotubes can lead to cracking of the active material. Therefore, it is extremely important to test a suitable ink formulation, which is an inevitable requirement for the preparation of cells with excellent electrochemical properties.
Inkjet printing, as an environmentally friendly and low-cost method for direct deposition of functional nanomaterials, has received widespread attention. Non-contact printing can be achieved on various substrates by programming the print pattern to be printed and then controlling the movement of the print nozzle. The modes of operation for inkjet printing are (i) drop-on-demand (DoD) printing, which delivers droplets caused by thermal bubbles or piezoelectric actuators, and (ii) continuous inkjet (CIJ) printing, which produces a continuous stream of ink through the nozzle by means of an electrostatic field or magnetic field. (Zhang et al., 2020e; Yan et al., 2020).
Ink-jet printing provides the advantage of flexible and wearable electronics manufacturing, such as design of high-throughput, large-scale, good performance, biocompatibility, and on the infinite basal precision deposition. In addition, due to droplet deposition and programmable pattern design, inkjet printing methods greatly reduce the waste of expensive ink materials (Figure 10A).
FIGURE 10. (A) Schematic of inkjet-printed MnO2/GO-based flexible supercapacitors on A4 paper substrates using an EPSON L130 printer. Left: CV curves of the flexible supercapacitors under various bending angles. Reproduced with permission from P. Copyright 2017 American Chemical Society. (B) Use the CC/zinc and AgNPs @ CC/zinc anode NVO lots zinc battery cycle stability under the condition of 50°C.Copyright 2021 Advanced Functional Materials.
Chen and his colleagues proposed a heterogeneous metal seed-mediated strategy. The basic idea is to print silver nanoparticles on a three-dimensional conductive backbone by inkjet printing and use them as heterogeneous metal seeds to induce uniform nucleation of zinc and avoid dendrite growth during the initial electroplating stage.At the same time, the reaction of Ag with Zn produces a zinc-friendly AgZn3 alloy as a Zn resource to compensate for the irreversible loss of active Zn during the recycling process. In the first 15 cycles, the capacity of the AgNPs@CC/Zn anode cell increased from 182 mA/g to 237 mA/g and maintained a reversible capacity of 218 mA/g, corresponding to a capacity retention rate of 92% (Figure 10B). (Chen et al., 2021a)
However, at present, there are still many challenges that the technology is relatively bad. These challenges include preventing nozzle clogging, inhibiting coffee ring effect, and enhancing ink dispersion,etc. (Yan et al., 2020)
Those climb from claiming 3D printing (3DP) innovation Concerning illustration An revolutionary manufacturing system has created a great attraction for the fabrication of functional electrodes in the field of energy storage and conversion. The printing process usually combines digital programming and manufacturing procedures to rationally design a 3D functional structural model of the object, followed by the use of special software to obtain sliced layers of the model, which are printed using digital technology material printers, i.e. by adding each two-dimensional (2D) layer to the previous one, enabling the construction of a 3D structure, thus enabling precise control of the spatial geometry and architecture of the device from the macro to the nano scale (Figure 11A). (Pang et al., 2019) Yang’s team has demonstrated a 3D printing system for those development of practical electrodes for zinc-air batteries. The printed anode comprises for large portions minor zinc balls. to enhance the utilization of zinc. The self-supporting air cathode made by 3D printing has a hierarchical porous structure with high specific surface area, which allows the electrode to have high electrocatalytic activity and fast reaction diffusion channels (Figure 11B). Thanks to these advantages, the assembled zinc-air battery achieves a high releasing capacity of 670 mA h/g at a current density of 5 mA/cm2 and a long period cycle stability of at least 350 cycles. (Zhang et al., 2020f).
FIGURE 11. (A) Schematic of the 3D “drop-on-demand” ink jet printer.Copyright 2019, Elsevier. (B) Schematic illustration of 3DP-Zn-E. Copyright 2019, Advanced Functional Materials.
Laser engraving processing is based on the use of CNC technology and laser as the processing medium. The physical denaturation of the electrode material by instantaneous melting and vaporization under laser engraving irradiation enables laser engraving to achieve the processing purpose, which in turn completes the preparation of flexible electrodes.Wang’s team used a high-precision, simple and low-cost laser engraving technique to prepare finger-shaped cathodes and anodes for ZIMB. Suspended finger-like multi-walled carbon nanotube MnO2 (MWCNTs-MnO2) cathodes were prepared by laser engraving machine (JL-K3020) and MWCNTs-Zn anodes based on zinc nanosheets were prepared by laser etching with further acknowledge the full adaptability from claiming ZIMB. The flexible conductive substrate prepared by laser engraving allows ZIMB to exhibit excellent flexibility, resulting in high reliability and stability of ZIMB. The concept proves that the capacity maintenance of ZIMB is up to 96.5% of the beginning capacity at 120° bending angle, and even after the 5th self-healing cycle, the capacity retention is still up to 90.2%. This worth of effort gives new plans for outlining another era of high-efficiency, high-reliability and high-stability micro-energy storage devices. (Du et al., 2020).
In the era of electronic information technology, electronic devices are developing towards miniaturization and flexibility. Those ever-growing enthusiasm from both academic Group What’s more shopper showcase will be activating those fast advancement of new helter skelter execution adaptable vitality stockpiling gadgets. In the research of flexible power supply, flexible zinc ion batteries have been noticed by researchers and have achieved some promising results. In this paper, the research progress of flexible zinc batteries in recent years is reviewed from the following aspects: the differences between different anode, cathode materials and electrolytes in flexible zinc batteries; the method of loading active materials on flexible electrodes are discussed and compared. Although many progresses have been made in this area, it is still highly desirable to solve the following challenges and problems in time to promote the application of flexible zinc batteries.
First of all, the performance of the active materials has a critical impact on the cycling performance and flexibility of the battery. The research of zinc anodes mainly focuses on the material of metal zinc anodes. The primary goal is to reduce the generation of zinc dendrites during long-term cycling, so as to improve the lifetime and electrochemical performance of the battery. Most studies have used zinc foil directly, and some studies have cast zinc and zinc oxide particles on flexible substrates or grow zinc in situ instead of zinc foil in order to obtain better mechanical properties and stability. The research of cathodes is mainly to try to obtain materials with high capacity, high cycling performance, low cost and good mechanical properties, including ion embedding and oxygen vacancy generation for some cathode materials to improve the battery performance. Moreover, the research on the electrolyte of flexible zinc ion battery mainly focuses on the gel electrolyte. It consists of polymer body and liquid electrolyte, which has relatively excellent flexibility performance and can basically meet the needs of flexible zinc ion battery, and also has relatively good ionic conductivity to improve the multiplication performance of flexible zinc ion battery, as well as can make good interfacial contact with electrode.
Secondly, the main technologies for loading active materials onto flexible electrodes include in-situ growth method, screen printing, and inkjet printing and so on. Among them, the in-situ growth of active materials does not require binder, which ensures rapid charge transfer between active materials and collectors, makes the active material evenly distributed on the collector, so that the prepared flexible electrodes have better electrochemical performance. Screen printing is a sheet-by-sheet or roll-by-roll printing method for flexible cell, which is considered to be a cost-effective, easy-to-handle and mass-producible process. Inkjet printing can programmed according to the pattern to be printed, enabling non-contact printing on a variety of substrates, resulting in low-cost, high-precision, high-efficiency electrode printing, which can be used for artificial intelligence equipment, special-shaped battery and private customization.
With the innovation of technology, flexible zinc ion battery has a wide application prospect in flexible electronic devices due to its excellent performance. The development of wearable/implantable electronic devices such as flexible display devices, health monitors and electronic sensors has attracted more and more attention from academia and industry. One of the biggest challenges in the development of flexible electronic devices is to develop flexible, light, thin and safe portable energy storage devices. Flexible water zinc ion batteries are safe, can meet safety performance, and can be designed to fit into clothing and other wearable devices. The batteries must better fit the shape of wearable devices to avoid being bulky. Therefore, the development prospect of flexible zinc ion battery is broad.
Despite the advantages of flexible zinc batteries, there are still many problems for researchers to continue to study: (1) compared with the high theoretical capacity of the zinc metal anode, the cathode active material with higher capacity need to be explored; (2) the stability of the zinc negative electrode at high current and high capacity needs to improved; (3) in flexible batteries, with movements such as bending, the loaded active material usually cracks or flakes off, leading to a rapid decline in electrochemical performance. Therefore, it is necessary to design the ideal electrode material structure or improve the process method of loaded active material, and then further improve the mechanical properties of the electrode; (4) to further increase the actual capacity of flexible zinc batteries while improving the mechanical properties of electrodes. How to encourage the capacity of zinc ion batteries by increasing the amount of loaded active substance, which in turn has wider practicality.
All authors listed have made a substantial, direct, and intellectual contribution to the work and approved it for publication.
This work was financially supported by the National Natural Science Foundation of China (61904097), the Shandong Scientific Research Awards Foundation for Outstanding Young Scientists (No. ZR2018BEM030), Scientific Research Foundation of Shandong University of Science and Technology for Recruited Talents (No. 2017RCJJ058) and the Program for Tsingtao Al-ion Power and Energy-storage Battery Research Team in the University.
The authors declare that the research was conducted in the absence of any commercial or financial relationships that could be construed as a potential conflict of interest.
All claims expressed in this article are solely those of the authors and do not necessarily represent those of their affiliated organizations, or those of the publisher, the editors and the reviewers. Any product that may be evaluated in this article, or claim that may be made by its manufacturer, is not guaranteed or endorsed by the publisher.
Alfaruqi, M. H., Islam, S., Putro, D. Y., Mathew, V., Kim, S., Jo, J., et al. (2018). Structural Transformation and Electrochemical Study of Layered MnO2 in Rechargeable Aqueous Zinc-Ion Battery. Electrochimica Acta 276, 1–11. doi:10.1016/j.electacta.2018.04.139
Chamoun, M., Brant, W. R., Tai, C.-W., Karlsson, G., and Noréus, D. (2018). Rechargeability of Aqueous Sulfate Zn/MnO2 Batteries Enhanced by Accessible Mn2+ Ions. Energ. Storage Mater. 15, 351–360. doi:10.1016/j.ensm.2018.06.019
Chen, M., Chen, J., Zhou, W., Han, X., Yao, Y., and Wong, C. P. (2021). Realizing an All-Round Hydrogel Electrolyte toward Environmentally Adaptive Dendrite-free Aqueous Zn-MnO2 Batteries. Adv. Mater. 33 (9), e2007559. doi:10.1002/adma.202007559
Chen, T., Wang, Y., Yang, Y., Huang, F., Zhu, M., Wei Ang, B. T., et al. (2021). Heterometallic Seed‐Mediated Zinc Deposition on Inkjet Printed Silver Nanoparticles toward Foldable and Heat‐Resistant Zinc Batteries. Adv. Funct. Mater. 31 (24). doi:10.1002/adfm.202101607
Cong, Z., Guo, W., Zhang, P., Sha, W., Guo, Z., Chang, C., et al. (2021). Wearable Antifreezing Fiber-Shaped Zn/PANI Batteries with Suppressed Zn Dendrites and Operation in Sweat Electrolytes. ACS Appl. Mater. Inter. 13 (15), 17608–17617. doi:10.1021/acsami.1c02065
Dong, H., Li, J., Guo, J., Lai, F., Zhao, F., Jiao, Y., et al. (2021). Insights on Flexible Zinc-Ion Batteries from Lab Research to Commercialization. Adv. Mater. 33 (20), e2007548. doi:10.1002/adma.202170158
Du, X., Shi, J., Chen, Z., Ni, T., Li, J., Ruan, L., et al. (2020). A Laser Etched Zinc Ion Microbattery with Excellent Flexibility and Self-Healability. Sustainable Energ. Fuels 4 (9), 4713–4721. doi:10.1039/d0se00843e
Eftekhari, A., Li, L., and Yang, Y. (2017). Polyaniline Supercapacitors. J. Power Sourc. 347, 86–107. doi:10.1016/j.jpowsour.2017.02.054
Fan, X., Liu, J., Song, Z., Han, X., Deng, Y., Zhong, C., et al. (2019). Porous Nanocomposite Gel Polymer Electrolyte with High Ionic Conductivity and superior Electrolyte Retention Capability for Long-Cycle-Life Flexible Zinc–Air Batteries. Nano Energy 56, 454–462. doi:10.1016/j.nanoen.2018.11.057
Fang, G., Zhou, J., Pan, A., and Liang, S. (2018). Recent Advances in Aqueous Zinc-Ion Batteries. ACS Energ. Lett. 3 (10), 2480–2501. doi:10.1021/acsenergylett.8b01426
Gaikwad, A. M., Whiting, G. L., Steingart, D. A., and Arias, A. C. (2011). Highly Flexible, Printed Alkaline Batteries Based on Mesh-Embedded Electrodes. Adv. Mater. 23 (29), 3251–3255. doi:10.1002/adma.201100894
Guo, F., Gao, S., Ji, C., Mi, H., Li, H., Zhang, W., et al. (2021). Finely Crafted Polyaniline Cathode for High-Performance Flexible Quasi-Solid-State Zn-Ion Battery. Solid State Ionics 364, 115612. doi:10.1016/j.ssi.2021.115612
Guo, X., Fang, G., Zhang, W., Zhou, J., Shan, L., Wang, L., et al. (2018). Mechanistic Insights of Zn2+ Storage in Sodium Vanadates. Adv. Energ. Mater. 8 (27). doi:10.1002/aenm.201801819
Han, J., Wang, K., Liu, W., Li, C., Sun, X., Zhang, X., et al. (2018). Rational Design of Nano-Architecture Composite Hydrogel Electrode towards High Performance Zn-Ion Hybrid Cell. Nanoscale 10 (27), 13083–13091. doi:10.1039/c8nr03889a
Han, Q., Chi, X., Zhang, S., Liu, Y., Zhou, B., Yang, J., et al. (2018). Durable, Flexible Self-Standing Hydrogel Electrolytes Enabling High-Safety Rechargeable Solid-State Zinc Metal Batteries. J. Mater. Chem. A 6 (45), 23046–23054. doi:10.1039/c8ta08314b
Hilder, M., Winther-Jensen, B., and Clark, N. B. (2009). Paper-based, Printed Zinc–Air Battery. J. Power Sourc. 194 (2), 1135–1141. doi:10.1016/j.jpowsour.2009.06.054
Huang, Y., Mou, J., Liu, W., Wang, X., Dong, L., Kang, F., et al. (2019). Novel Insights into Energy Storage Mechanism of Aqueous Rechargeable Zn/MnO2 Batteries with Participation of Mn2+. Nano-micro Lett. 11 (1), 49. doi:10.1007/s40820-019-0278-9
Javed, M. S., Lei, H., Wang, Z., Liu, B-t., Cai, X., and Mai, W. (2020). 2D V2O5 Nanosheets as a Binder-free High-Energy Cathode for Ultrafast Aqueous and Flexible Zn-Ion Batteries. Nano Energy 70, 104573. doi:10.1016/j.nanoen.2020.104573
Jia, Z., Wang, B., and Wang, Y. (2015). Copper Hexacyanoferrate with a Well-Defined Open Framework as a Positive Electrode for Aqueous Zinc Ion Batteries. Mater. Chem. Phys. 149-150, 601–606. doi:10.1016/j.matchemphys.2014.11.014
Jo, Y. N., Prasanna, K., Kang, S. H., Ilango, P. R., Kim, H. S., Eom, S. W., et al. (2017). The Effects of Mechanical Alloying on the Self-Discharge and Corrosion Behavior in Zn-Air Batteries. J. Ind. Eng. Chem. 53, 247–252. doi:10.1016/j.jiec.2017.04.032
Kaveevivitchai, W., and Manthiram, A. (2016). High-capacity Zinc-Ion Storage in an Open-Tunnel Oxide for Aqueous and Nonaqueous Zn-Ion Batteries. J. Mater. Chem. A. 4 (48), 18737–18741. doi:10.1039/c6ta07747a
Kumar, R., Johnson, K. M., Williams, N. X., and Subramanian, V. (2019). Scaling Printable Zn–Ag2O Batteries for Integrated Electronics. Adv. Energ. Mater. 9 (13). doi:10.1002/aenm.201803645
Kumar, R., Shin, J., Yin, L., You, J. M., Meng, Y. S., and Wang, J. (2016). All‐Printed, Stretchable Zn‐Ag2O Rechargeable Battery via Hyperelastic Binder for Self‐Powering Wearable Electronics. Adv. Energ. Mater. 7 (8). doi:10.1002/aenm.201602096
Lao-atiman, W., Julaphatachote, T., Boonmongkolras, P., and Kheawhom, S. (2017). Printed Transparent Thin Film Zn-MnO2Battery. J. Electrochem. Soc. 164 (4), A859–A863. doi:10.1149/2.1511704jes
Lee, S.-M., Kim, Y.-J., Eom, S.-W., Choi, N.-S., Kim, K.-W., and Cho, S.-B. (2013). Improvement in Self-Discharge of Zn Anode by Applying Surface Modification for Zn-Air Batteries with High Energy Density. J. Power Sourc. 227, 177–184. doi:10.1016/j.jpowsour.2012.11.046
Li, C., Zhang, Q., Sun, J., Li, T., Songfeng, E., Zhu, Z., et al. (2018). High-Performance Quasi-Solid-State Flexible Aqueous Rechargeable Ag–Zn Battery Based on Metal–Organic Framework-Derived Ag Nanowires. ACS Energ. Lett. 3 (11), 2761–2768. doi:10.1021/acsenergylett.8b01675
Li, H., Han, C., Huang, Y., Huang, Y., Zhu, M., Pei, Z., et al. (2018). An Extremely Safe and Wearable Solid-State Zinc Ion Battery Based on a Hierarchical Structured Polymer Electrolyte. Energy Environ. Sci. 11 (4), 941–951. doi:10.1039/c7ee03232c
Li, M., Liu, B., Fan, X., Liu, X., Liu, J., Ding, J., et al. (2019). Long-Shelf-Life Polymer Electrolyte Based on Tetraethylammonium Hydroxide for Flexible Zinc-Air Batteries. ACS Appl. Mater. Inter. 11 (32), 28909–28917. doi:10.1021/acsami.9b09086
Li, R., Li, L., Jia, R., Jiang, K., Shen, G., and Chen, D. (2020). A Flexible Concentric Circle Structured Zinc‐Ion Micro‐Battery with Electrodeposited Electrodes. Small Methods 4 (9). doi:10.1002/smtd.202000363
Li, S., Yang, X., Yang, S., Gao, Q., Zhang, S., Yu, X., et al. (2020). An Amorphous Trimetallic (Ni–Co–Fe) Hydroxide-Sheathed 3D Bifunctional Electrode for superior Oxygen Evolution and High-Performance cable-type Flexible Zinc–Air Batteries. J. Mater. Chem. A 8 (11), 5601–5611. doi:10.1039/d0ta00888e
Li, T., Wang, J., Li, X., Si, L., Zhang, S., and Deng, C. (2020). Unlocking the Door of Boosting Biodirected Structures for High-Performance VNxOy/C by Controlling the Reproduction Mode. Adv. Sci. (Weinh). 7 (5), 1903276. doi:10.1002/advs.201903276
Li, X., Tang, Y., Lv, H., Wang, W., Mo, F., Liang, G., et al. (2019). Recent Advances in Flexible Aqueous Zinc-Based Rechargeable Batteries. Nanoscale 11 (39), 17992–18008. doi:10.1039/c9nr06721c
Li, Y., Fu, J., Zhong, C., Wu, T., Chen, Z., Hu, W., et al. (2018). Recent Advances in Flexible Zinc‐Based Rechargeable Batteries. Adv. Energ. Mater. 9 (1). doi:10.1002/aenm.201802605
Lian, S., Sun, C., Xu, W., Huo, W., Luo, Y., Zhao, K., et al. (2019). Built-in Oriented Electric Field Facilitating Durable Zn MnO2 Battery. Nano Energy 62, 79–84. doi:10.1016/j.nanoen.2019.04.038
Liang, M., Zhou, H., Huang, Q., Hu, S., and Li, W. (2011). Synergistic Effect of Polyethylene Glycol 600 and Polysorbate 20 on Corrosion Inhibition of Zinc Anode in Alkaline Batteries. J. Appl. Electrochem. 41, 991–997. doi:10.1007/s10800-011-0328-6
Liang, X., Yan, L., Li, W., Bai, Y., Zhu, C., Qiang, Y., et al. (2021). Flexible High-Energy and Stable Rechargeable Vanadium-Zinc Battery Based on Oxygen Defect Modulated V2O5 Cathode. Nano Energy 87, 106164. doi:10.1016/j.nanoen.2021.106164
Liao, M., Wang, J., Ye, L., Sun, H., Wen, Y., Wang, C., et al. (2020). A Deep-Cycle Aqueous Zinc-Ion Battery Containing an Oxygen-Deficient Vanadium Oxide Cathode. Angew. Chem. Int. Ed. Engl. 59 (6), 2273–2278. doi:10.1002/anie.201912203
Lin, Y., Zhou, F., Xie, M., Zhang, S., and Deng, C. (2020). V6O13-delta @C Nanoscrolls with Expanded Distances between Adjacent Shells as a High-Performance Cathode for a Knittable Zinc-Ion Battery. ChemSusChem 13 (14), 3696–3706. doi:10.1002/cssc.202000699
Liu, J., Guan, C., Zhou, C., Fan, Z., Ke, Q., Zhang, G., et al. (2016). A Flexible Quasi-Solid-State Nickel-Zinc Battery with High Energy and Power Densities Based on 3D Electrode Design. Adv. Mater. 28 (39), 8732–8739. doi:10.1002/adma.201603038
Liu, T., Mou, J., Wu, Z., Lv, C., Huang, J., and Liu, M. (2020). A Facile and Scalable Strategy for Fabrication of Superior Bifunctional Freestanding Air Electrodes for Flexible Zinc–Air Batteries. Adv. Funct. Mater. 30 (36). doi:10.1002/adfm.202003407
Liu, Y., Xie, L., Zhang, W., Dai, Z., Wei, W., Luo, S., et al. (2019). Conjugated System of PEDOT:PSS-Induced Self-Doped PANI for Flexible Zinc-Ion Batteries with Enhanced Capacity and Cyclability. ACS Appl. Mater. Inter. 11 (34), 30943–30952. doi:10.1021/acsami.9b09802
Liu, Z., Liang, G., Zhan, Y., Li, H., Wang, Z., Ma, L., et al. (2019). A Soft yet Device-Level Dynamically Super-tough Supercapacitor Enabled by an Energy-Dissipative Dual-Crosslinked Hydrogel Electrolyte. Nano Energy 58, 732–742. doi:10.1016/j.nanoen.2019.01.087
Liu, Z., Mo, F., Li, H., Zhu, M., Wang, Z., Liang, G., et al. (2018). Advances in Flexible and Wearable Energy-Storage Textiles. Small Methods 2 (11). doi:10.1002/smtd.201800124
Lu, K., Song, B., Zhang, Y., Ma, H., and Zhang, J. (2017). Encapsulation of Zinc Hexacyanoferrate Nanocubes with Manganese Oxide Nanosheets for High-Performance Rechargeable Zinc Ion Batteries. J. Mater. Chem. A. 5 (45), 23628–23633. doi:10.1039/c7ta07834j
Ma, L., Chen, S., Li, N., Liu, Z., Tang, Z., Zapien, J. A., et al. (2020). Hydrogen-Free and Dendrite-free All-Solid-State Zn-Ion Batteries. Adv. Mater. 32 (14), e1908121. doi:10.1002/adma.201908121
Ma, L., Chen, S., Long, C., Li, X., Zhao, Y., Liu, Z., et al. (2019). Achieving High‐Voltage and High‐Capacity Aqueous Rechargeable Zinc Ion Battery by Incorporating Two‐Species Redox Reaction. Adv. Energ. Mater. 9 (45). doi:10.1002/aenm.201902446
Ma, L., Chen, S., Wang, D., Yang, Q., Mo, F., Liang, G., et al. (2019). Super‐Stretchable Zinc–Air Batteries Based on an Alkaline‐Tolerant Dual‐Network Hydrogel Electrolyte. Adv. Energ. Mater. 9 (12). doi:10.1002/aenm.201803046
Ma, L., Chen, S., Li, H., Ruan, Z., Tang, Z., Liu, Z., et al. (2018). Initiating a Mild Aqueous Electrolyte Co3O4/Zn Battery with 2.2 V-High Voltage and 5000-cycle Lifespan by a Co(iii) Rich-Electrode. Energ. Environ. Sci. 11 (9), 2521–2530. doi:10.1039/c8ee01415a
Naveed, A., Yang, H., Yang, J., Nuli, Y., and Wang, J. (2019). Highly Reversible and Rechargeable Safe Zn Batteries Based on a Triethyl Phosphate Electrolyte. Angew. Chem. Int. Ed. Engl. 58 (9), 2760–2764. doi:10.1002/anie.201813223
Nguyen, T. T., Balamurugan, J., Kim, D. H., Kim, N. H., and Lee, J. H. (2020). Hierarchical 3D Oxygenated Cobalt Vanadium Selenide Nanosheets as Advanced Electrode for Flexible Zinc-Cobalt and Zinc-Air Batteries. Small 16 (48), e2004661. doi:10.1002/smll.202004661
Pan, H., Shao, Y., Yan, P., Cheng, Y., Han, K. S., Nie, Z., et al. (2016). Reversible Aqueous Zinc/manganese Oxide Energy Storage from Conversion Reactions. Nat. Energ. 1, 16039. doi:10.1038/nenergy.2016.39
Pang, Y., Cao, Y., Chu, Y., Liu, M., Snyder, K., Mackenzie, D., et al. (2019). Additive Manufacturing of Batteries. Adv. Funct. Mater. 30 (1). doi:10.1002/adfm.201906244
Qiu, W., Li, Y., You, A., Zhang, Z., Li, G., Lu, X., et al. (2017). High-performance Flexible Quasi-Solid-State Zn-MnO2 Battery Based on MnO2 Nanorod Arrays Coated 3D Porous Nitrogen-Doped Carbon Cloth. J. Mater. Chem. A. 5 (28), 14838–14846. doi:10.1039/c7ta03274a
Shi, H.-Y., Ye, Y.-J., Liu, K., Song, Y., and Sun, X. (2018). A Long-Cycle-Life Self-Doped Polyaniline Cathode for Rechargeable Aqueous Zinc Batteries. Angew. Chem. Int. Ed. 57 (50), 16359–16363. doi:10.1002/anie.201808886
Song, H., Liu, C., Zhang, C., and Cao, G. (2016). Self-doped V4+–V2o5 Nanoflake for 2 Li-Ion Intercalation with Enhanced Rate and Cycling Performance. Nano Energy 22, 1–10. doi:10.1016/j.nanoen.2016.02.004
Song, M., Tan, H., Chao, D., and Fan, H. J. (2018). Recent Advances in Zn-Ion Batteries. Adv. Funct. Mater. 28 (41). doi:10.1002/adfm.201802564
Sumboja, A., Liu, J., Zheng, W. G., Zong, Y., Zhang, H., and Liu, Z. (2018). Electrochemical Energy Storage Devices for Wearable Technology: a Rationale for Materials Selection and Cell Design. Chem. Soc. Rev. 3047 (15), 5919–5945. doi:10.1039/c8cs00237a
Tan, P., Chen, B., Xu, H., Cai, W., He, W., Zhang, H., et al. (2018). Integration of Zn-Ag and Zn-Air Batteries: A Hybrid Battery with the Advantages of Both. ACS Appl. Mater. Inter. 10 (43), 36873–36881. doi:10.1021/acsami.8b10778
Tan, P., Chen, B., Xu, H., Zhang, H., Cai, W., Ni, M., et al. (2017). Flexible Zn- and Li-Air Batteries: Recent Advances, Challenges, and Future Perspectives. Energ. Environ. Sci. 10 (10), 2056–2080. doi:10.1039/c7ee01913k
Tang, B., Shan, L., Liang, S., and Zhou, J. (2019). Issues and Opportunities Facing Aqueous Zinc-Ion Batteries. Energ. Environ. Sci. 12 (11), 3288–3304. doi:10.1039/c9ee02526j
Tehrani, Z., Korochkina, T., Govindarajan, S., Thomas, D. J., Mahony, J. O., Kettle, J., et al. (2015). Ultra-thin Flexible Screen Printed Rechargeable Polymer Battery for Wearable Electronic Applications. Org. Electronics 26, 386–394. doi:10.1016/j.orgel.2015.08.007
vKundu, D., Adams, B. D., Duffort, V., Vajargah, S. H., and Nazar, L. F. (2016). A High-Capacity and Long-Life Aqueous Rechargeable Zinc Battery Using a Metal Oxide Intercalation Cathode. Nat. Energ. 1 (10). doi:10.1038/nenergy.2016.119
Wan, F., Zhang, L., Dai, X., Wang, X., Niu, Z., and Chen, J. (2018). Aqueous Rechargeable Zinc/sodium Vanadate Batteries with Enhanced Performance from Simultaneous Insertion of Dual Carriers. Nat. Commun. 9 (1), 1656. doi:10.1038/s41467-018-04060-8
Wang, D., Li, H., Liu, Z., Tang, Z., Liang, G., Mo, F., et al. (2018). A Nanofibrillated Cellulose/Polyacrylamide Electrolyte-Based Flexible and Sewable High-Performance Zn-MnO2 Battery with Superior Shear Resistance. Small 14 (51), e1803978. doi:10.1002/smll.201803978
Wang, H., Bi, X., Bai, Y., Wu, C., Gu, S., Chen, S., et al. (2017). Open‐Structured V2O5·nH2O Nanoflakes as Highly Reversible Cathode Material for Monovalent and Multivalent Intercalation Batteries. Adv. Energ. Mater. 7 (14). doi:10.1002/aenm.201770077
Wang, X., Zheng, S., Zhou, F., Qin, J., Shi, X., Wang, X., et al. (2020). Scalable Fabrication of Printed Zn//MnO2 Planar Micro-batteries with High Volumetric Energy Density and Exceptional Safety. Natl. Sci. Rev. 7 (1), 64–72. doi:10.1093/nsr/nwz070
Wang, Z., Meng, X., Chen, K., and Mitra, S. (2018). Synthesis of Carbon Nanotube Incorporated Metal Oxides for the Fabrication of Printable, Flexible Nickel-Zinc Batteries. Adv. Mater. Inter. 5 (4). doi:10.1002/admi.201701036
Xu, C., Li, B., Du, H., and Kang, F. (2012). Energetic Zinc Ion Chemistry: the Rechargeable Zinc Ion Battery. Angew. Chem. Int. Ed. 51 (4), 933–935. doi:10.1002/anie.201106307
Xu, W., Sun, C., Wang, N., Liao, X., Zhao, K., Yao, G., et al. (2021). Sn Stabilized Pyrovanadate Structure Rearrangement for Zinc Ion Battery. Nano Energy 81, 105584. doi:10.1016/j.nanoen.2020.105584
Xu, Y., Zheng, S., Tang, H., Guo, X., Xue, H., and Pang, H. (2017). Prussian Blue and its Derivatives as Electrode Materials for Electrochemical Energy Storage. Energ. Storage Mater. 9, 11–30. doi:10.1016/j.ensm.2017.06.002
Yan, K., Li, J., Pan, L., and Shi, Y. (2020). Inkjet Printing for Flexible and Wearable Electronics. APL Mater. 8 (12). doi:10.1063/5.0031669
Yao, H., Li, Q., Zhang, M., Tao, Z., and Yang, Y. (2020). Prolonging the Cycle Life of Zinc-Ion Battery by Introduction of [Fe(CN)6]4− to PANI via a Simple and Scalable Synthetic Method. Chem. Eng. J. 392, 123653. doi:10.1016/j.cej.2019.123653
Yin, L., Scharf, J., Ma, J., Doux, J.-M., Redquest, C., Le, V. L., et al. (2021). High Performance Printed AgO-Zn Rechargeable Battery for Flexible Electronics. Joule 5, 228–248. doi:10.1016/j.joule.2020.11.008
Yu, M., Lin, D., Feng, H., Zeng, Y., Tong, Y., and Lu, X. (2017). Boosting the Energy Density of Carbon-Based Aqueous Supercapacitors by Optimizing the Surface Charge. Angew. Chem. Int. Ed. 56 (20), 5454–5459. doi:10.1002/anie.201701737
Yu, M., Zeng, Y., Han, Y., Chen, X., Zhao, W., Liang, C., et al. (2015). Valence-optimized Vanadium Oxide Supercapacitor Electrodes Exhibit Ultrahigh Capacitance and Super-long Cyclic Durability of 100000 Cycles. Adv. Funct. Mater. 25, 3534–3540. doi:10.1002/adfm.201501342
Yu, P., Zeng, Y., Zhang, H., Yu, M., Tong, Y., and Lu, X. (2019). Flexible Zn-Ion Batteries: Recent Progresses and Challenges. Small 15 (7), e1804760. doi:10.1002/smll.201804760
Yza, B., Gl, A., and Cz, A. (2022). Low-cost MgFe X Mn2-xO4 Cathode Materials for High-Performance Aqueous Rechargeable Magnesium-Ion Batteries. Chem. Eng. J. 392.
Zeng, Y., Zhang, X., Qin, R., Liu, X., Fang, P., Zheng, D., et al. (2019). Dendrite-Free Zinc Deposition Induced by Multifunctional CNT Frameworks for Stable Flexible Zn-Ion Batteries. Adv. Mater. 31 (36), e1903675. doi:10.1002/adma.201903675
Zhang, J., Li, X. L., Fan, S., Huang, S., Yan, D., Liu, L., et al. (2020). 3D-printed Functional Electrodes towards Zn-Air Batteries. Mater. Today Energ. 16. doi:10.1016/j.mtener.2020.100407
Zhang, L., Chao, D., and Yang, P. (2020). Flexible Pseudocapacitive Electrochromics via Inkjet Printing of Additive‐Free Tungsten Oxide Nanocrystal Ink. Adv. Energ. Mater. 10 (17). doi:10.1002/aenm.202000142
Zhang, L., Chen, L., Zhou, X., and Liu, Z. (2015). Towards High-Voltage Aqueous Metal-Ion Batteries beyond 1.5 V: The Zinc/Zinc Hexacyanoferrate System. Adv. Energ. Mater. 5 (2). doi:10.1002/aenm.201400930
Zhang, N., Cheng, F., Liu, J., Wang, L., Long, X., Liu, X., et al. (2017). Rechargeable Aqueous Zinc-Manganese Dioxide Batteries with High Energy and Power Densities. Nat. Commun. 8 (1), 405. doi:10.1038/s41467-017-00467-x
Zhang, N., Cheng, F., Liu, Y., Zhao, Q., Lei, K., Chen, C., et al. (2016). Cation-Deficient Spinel ZnMn2O4 Cathode in Zn(CF3SO3)2 Electrolyte for Rechargeable Aqueous Zn-Ion Battery. J. Am. Chem. Soc. 138 (39), 12894–12901. doi:10.1021/jacs.6b05958
Zhang, W., Zuo, C., Tang, C., Tang, W., Lan, B., Fu, X., et al. (2020). The Current Developments and Perspectives of V2O5 as Cathode for Rechargeable Aqueous Zinc‐Ion Batteries. Energ. Technology 9 (2). doi:10.1002/ente.202000789
Zhang, Y., Deng, S., Pan, G., Zhang, H., Liu, B., Wang, X. L., et al. (2020). Introducing Oxygen Defects into Phosphate Ions Intercalated Manganese Dioxide/Vertical Multilayer Graphene Arrays to Boost Flexible Zinc Ion Storage. Small Methods 4 (6). doi:10.1002/smtd.201900828
Zhang, Y., Tao, L., Xie, C., Wang, D., Zou, Y., Chen, R., et al. (2020). Defect Engineering on Electrode Materials for Rechargeable Batteries. Adv. Mater. 32 (7), e1905923. doi:10.1002/adma.201905923
Zhang, Y., Xu, G., Liu, X., Wei, X., Cao, J., and Yang, L. (2020). Scalable In Situ Reactive Assembly of Polypyrrole‐Coated MnO 2 Nanowire and Carbon Nanotube Composite as Freestanding Cathodes for High Performance Aqueous Zn‐Ion Batteries. ChemElectroChem 7 (13), 2762–2770. doi:10.1002/celc.202000253
Zhang, Z., Cui, L., Shi, X., Tian, X., Wang, D., Gu, C., et al. (2018). Textile Display for Electronic and Brain-Interfaced Communications. Adv. Mater. 30 (18), e1800323. doi:10.1002/adma.201800323
Zhao, J., Sonigara, K. K., Li, J., Zhang, J., Chen, B., Zhang, J., et al. (2017). A Smart Flexible Zinc Battery with Cooling Recovery Ability. Angew. Chem. Int. Ed. Engl. 56, 7871–7875. doi:10.1002/anie.201704373
Zhao, J., Ren, H., Liang, Q., Yuan, D., Xi, S., Wu, C., et al. (2019). High-performance Flexible Quasi-Solid-State Zinc-Ion Batteries with Layer-Expanded Vanadium Oxide Cathode and Zinc/stainless Steel Mesh Composite Anode. Nano Energy 62, 94–102. doi:10.1016/j.nanoen.2019.05.010
Keywords: flexible, zinc ion battery, zinc-air battery, mechanical property, flexible substrate, in situ growth, inkjet printing, screen printing
Citation: Xu Y, Xu X, Guo M, Zhang G and Wang Y (2022) Research Progresses and Challenges of Flexible Zinc Battery. Front. Chem. 10:827563. doi: 10.3389/fchem.2022.827563
Received: 02 December 2021; Accepted: 25 January 2022;
Published: 14 February 2022.
Edited by:
Yu Ding, University of Maryland, United StatesReviewed by:
Kangning Zhao, Swiss Federal Institute of Technology Lausanne, SwitzerlandCopyright © 2022 Xu, Xu, Guo, Zhang and Wang. This is an open-access article distributed under the terms of the Creative Commons Attribution License (CC BY). The use, distribution or reproduction in other forums is permitted, provided the original author(s) and the copyright owner(s) are credited and that the original publication in this journal is cited, in accordance with accepted academic practice. No use, distribution or reproduction is permitted which does not comply with these terms.
*Correspondence: Guoxin Zhang, emhhbmdneEBzZHVzdC5lZHUuY24=; Yaqun Wang, eXF3YW5nQHNkdXN0LmVkdS5jbg==
Disclaimer: All claims expressed in this article are solely those of the authors and do not necessarily represent those of their affiliated organizations, or those of the publisher, the editors and the reviewers. Any product that may be evaluated in this article or claim that may be made by its manufacturer is not guaranteed or endorsed by the publisher.
Research integrity at Frontiers
Learn more about the work of our research integrity team to safeguard the quality of each article we publish.