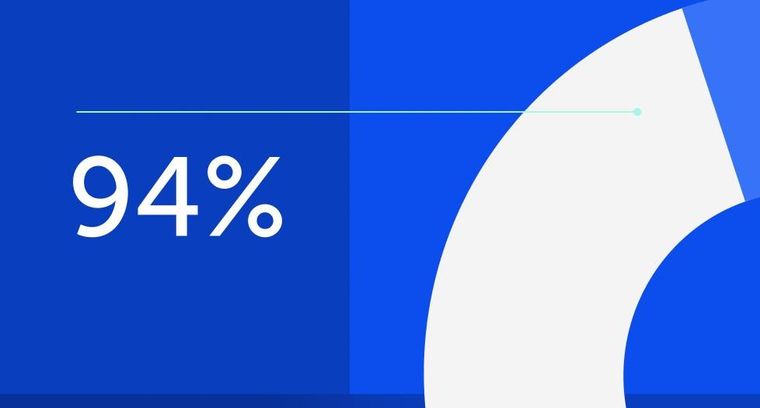
94% of researchers rate our articles as excellent or good
Learn more about the work of our research integrity team to safeguard the quality of each article we publish.
Find out more
ORIGINAL RESEARCH article
Front. Chem., 07 February 2022
Sec. Electrochemistry
Volume 10 - 2022 | https://doi.org/10.3389/fchem.2022.813008
This article is part of the Research TopicFrontiers in Chemistry: Editors Showcase 2021View all 50 articles
Reinforced concrete is among the most multifaceted materials used in the construction field. Maintaining the resistance of reinforced concrete to weathering, abrasion, and chemical attack, particularly in aggressive natural conditions such as seawater environments, is challenging. The main factor in the degradation of reinforced-concrete durability is chloride penetration, which accelerates iron alloy corrosion and facilitates structural degradation. In this study, calcium-iron-based layered double hydroxides (CaFe-LDHs) were fabricated at room temperature, followed by structural modulation, and their effectiveness in mitigating iron alloy corrosion due to chloride ions (in 3.5 wt% of NaCl) was investigated. The synthesized CaFe-LDHs with phase transfer notably improved the Cl− removal capacity (Qmax) to 881.83 mg/g, which is more than three times that reported based on previous studies. The novelty of this research lies in the sophisticated structural and phase transformations of the as-synthesized CaFe-LDHs, determination of crucial factors for chloride ion removal, and suggestion of calcium-iron-based layered double oxide (CaFe-LDO)-based chloride ion removal mechanisms considering chemical and ion-exchange reactions. Moreover, when the phase-transformed LDHs, C-700 LDOs, were applied to inhibit iron alloy corrosion, a noticeable inhibition efficiency of 98.87% was obtained, which was an 11-fold improvement compared to the case of iron alloys without LDOs. We believe this work can provide new insights into the design of CaFe-LDOs for the enhancement of the lifespan of reinforced concrete structures.
• Structural transformation of CaFe-based LDOs in crystallinity and morphology
• Novel reconstruction mechanism of structure-modulated CaFe-LDOs
• Cl- removal capacity of C-700 LDOs, higher than previous reports.
• Inhibition efficiency of 98.87% was obtained comparing with iron alloys without LDOs.
• Potential in the construction industries to enhance the durability of buildings.
Corrosion damage to reinforcement and prestressed steel has been identified as the primary cause of a significant number of structural failures over the past centuries. It also entails a significant cost in terms of the repair, monitoring, and replacement of structures (Berrocal et al., 2016). Reinforced concrete structures are continually exposed to destructive elements that decrease their durability and lifespan. In general, concrete has high alkalinity (pH > 13) and causes iron alloys to develop a passivation layer to prevent corrosion (Ke et al., 2017). The corrosion of reinforcement steel in concrete can only be initiated when the passivation layer is destroyed by anions, particularly chloride ions (Cl−). The penetration of chloride into reinforced concrete is a significant threat, specifically for structures near marine environments that are exposed to high concentrations of salts. Such a scenario allows chloride ions to easily penetrate concrete structures through capillary adsorption, hydrostatic pressure, or diffusion into the iron alloys (Pradhan and Bhattacharjee, 2011; Muthulingam and Rao, 2015). Poor concrete quality or insufficient covering of concrete is deleterious to the durability of iron alloys and can lead to substantial economic losses and serious safety disasters (Bassuoni and Nehdi, 2008; Chen et al., 2016). Therefore, the selective and prompt removal of anions that cause deterioration immediately upon their penetration is desirable to maintain the durability and lifespan of concrete structures.
Layered double hydroxides (LDHs), also known as anionic clays, capture anions in aqueous solutions. The structure of LDHs is similar to the regular hexagonal structure of brucite [M(OH)2], and their general chemical formula is [M2+1-xM3+x(OH)2]x+[An-x/n] x−∙yH2O, where M2+ (e.g., Ca, Mg, Zn, Co, Ni, Cu) and M3+ (e.g., Fe, Al, Cr) are divalent and trivalent metal cations, respectively, and An-is an n-valent anion. Owing to the substitution of M2+ with M3+ in the brucite-like metallic hydroxide M(OH)2, the layered structures have positive charges that are balanced by interlayer anions (An−) (Yang et al., 2013; Mishra et al., 2018; Zuo et al., 2019). A unique property of LDHs is that their original structure can be obtained upon rehydration after calcination treatment (Erickson et al., 2005; Santos R. M. M. et al., 2017; Ivánová et al., 2018). In addition, it is well known that various anions can be selectively exchanged without altering the original structure, a feature referred to as the memory effect (Lv et al., 2015). Other advantageous features of LDHs, including their high specific surface area, non-toxicity, and low cost, expand their utilization in the fields of hazardous material adsorption, catalysis, biomedicine, and agriculture (Evans and Duan, 2006; Benício et al., 2015; Lv et al., 2015).
Among the published reports on chloride adsorption with LDHs, a maximum removal capacity of 257 mg/g was reported by Yoon et al. (2014), who compared the adsorption capabilities of various LDHs with different metal hydroxide layers. CaFe-LDHs also show high potential for removing condensed phosphate (Wu et al., 2012). However, the few existing studies are insufficient to understand the adsorption mechanism of CaFe-LDHs, and studies on the anion adsorption capacity are limited.
In this paper, we report the synthesis of structure-modulated calcium-iron-based layered double oxides (CaFe-LDOs) and their outstanding performance in terms of both a high chloride ion removal capacity and iron alloy corrosion protection. To evaluate the effectiveness of CaFe-LDOs in enhancing the durability of concrete, the chloride removal performance and mitigation of iron alloy corrosion by CaFe-LDOs were examined using an aqueous solution of 3.5 wt% NaCl, which corresponds to the concentration of chloride in seawater. In addition, the mechanisms of chloride ion exchange and corrosion protection were investigated.
CaFe-LDH powders were prepared using calcium nitrate tetrahydrate (Ca(NO3)2·4H2O, 99%), iron nitrate nonahydrate (Fe(NO3)3·9H2O, 98%), sodium hydroxide (NaOH, 99%), and sodium chloride (NaCl, 99.5%), all of which were purchased from Sigma-Aldrich, Inc. and Daejung, Inc. without further purification. Distilled deionized water was used to prepare the standard aqueous ionic solutions.
The CaFe-LDHs (pristine LDHs) were prepared via a one-step co-precipitation method (Wu et al., 2012; Gupta et al., 2020; Kong et al., 2021; Romero Ortiz et al., 2021). In particular, the optimized conditions for CaFe-LDH synthesis were determined by varying the Ca2+:Fe3+ molar ratio in the precursor solutions. First, an aqueous 3 M NaOH solution (300 ml) was rapidly injected at a rate of 300 ml/min into a second solution (300 ml) containing 66.7–80.0 mmol of Ca(NO3)2·4H2O and 33.3–20.0 mmol of Fe(NO3)3·9H2O at room temperature for 1 min to induce supersaturation. This was followed by the aging of the solution for 18 h. The concentration of each salt was balanced according to the Ca2+:Fe3+ molar ratio, which ranged from 2:1 to 4:1. During this process, the pH was controlled from 12 to 14, and all reactions were performed at room temperature. Upon completion of the reaction, the as-formed precipitates were filtered (200 nm), and the synthesized CaFe-LDHs were washed with excess quantities of ethanol to remove any undesirable nitrate salt residues. The obtained crystals were stored at room temperature in a desiccator to prevent contact with moisture and carbon dioxide in the air.
Structural transformations were induced in the as-prepared CaFe-LDHs by thermal treatment. The specimens were heat-treated in a furnace at 400°C (C-400 LDOs) and 700°C (C-700 LDOs) for 3 h in air at a heating rate of 10°C/min.
A chloride solution was prepared by dissolving NaCl in deionized water. The initial concentration of the chloride ions was 200 ppm. Chloride removal was conducted in 200 ml of the aqueous ionic solution after adding 1.89 g of LDHs and LDO under magnetic stirring at 250 rpm.
The type of steel used in the corrosion experiments was SS400 with a chemical composition (in wt%) of 0.27 C, 0.90 Mn, 0.20 Cu, 0.05 S, 0.04 Si, and 0.04 P (Fe balance). Iron alloys (1.5 mm) were first sanded and then washed with ethanol. Iron alloy specimens for the corrosion tests were prepared using a simple microstopper coating. The surface on one side of the iron alloys was exposed to perform the corrosion experiment (1 cm × 1 cm), and the remaining area was sealed with a microstopper. An electrolyte solution of 3.5 wt% NaCl, which corresponds to the typical concentration of NaCl in seawater, was prepared. Solution-based chloride adsorption with LDHs and LDOs (pristine LDHs, C-400 LDOs, and C-700 LDOs) and without LDHs and LDOs (bare iron alloys) was monitored. The reaction was initiated by adding 1 g of CaFe-LDH and LDO powder to 250 ml of the corrosion solution. A saturated calomel electrode (SCE) and platinum electrode (PE) were employed as the reference and auxiliary electrodes, respectively.
The solution pH was measured using a FE20 pH meter (Mettler Toledo, United States) equipped with a glass electrode (LE438, Mettler Toledo, United States). Thermogravimetric (TGA) measurements were performed using a Shimadzu TGA-50 thermogravimetric analyzer. The ion concentrations in the liquids after ion exchange were determined by ion chromatography (METROSEP A SUPP 5-250) at a flow rate of 0.7 ml/min with an eluent mixture of 3.2 mM Na2CO3/1.0 mM NaHCO3. Solid samples were characterized by Fourier transform infrared spectroscopy (FT-IR, Thermo Scientific 380 FT-IR) in the range of 4,000 to 500 cm−1 with a resolution of 4 cm−1; analysis was conducted on a 32 mm disc. X-ray diffraction (XRD) patterns of the solids were acquired using a D/max2200 diffractometer (Rigaku Co., Japan), operating at 40 kV and 100 mA, with Cu Kα radiation (λ = 0.1541 nm) in the range of 2θ = 10–80° at a scan rate of 4°/min. The morphology was characterized using a scanning electron microscope (SEM, S-4800, Hitachi Ltd. Japan) equipped with an energy-dispersive X-ray spectroscopy (EDX) detector. The morphology and size of the as-obtained samples were observed by transmission electron microscopy (TEM, JEM-2100F, JEOL, Japan) at an accelerating voltage of 200 kV. For corrosion testing, the anti-corrosive properties and electrical conductivity of the iron alloys were determined by electrochemical measurements, including potentiodynamic polarization (PDP) and electrochemical impedance spectroscopy (EIS) measurements. The EIS measurements were performed by applying a sinusoidal potential perturbation of 10 mV over a frequency range of 100 kHz to 100 MHz. The obtained EIS spectra were analyzed with an equivalent circuit using the Zman software. All electrochemical measurements were performed under quiescent conditions. No attempt was made to aerate or de-aerate the test solutions.
CaFe-LDHs with nitrate
where K is the shape factor, λ is the X-ray wavelength, β is the full width at half maximum for a given diffraction peak after correcting for instrumental broadening, and θ is the Bragg angle of reflection.
TABLE 1. Structural parameters from XRD analysis of CaFe-LDHs with different Ca2+:Fe3+ molar ratios.
As shown in Supplementary Figure S1B, when the co-precipitation reaction was performed at a pH less than 13, no CaFe-LDH crystallites were formed. With an increase in the initial pH to 14, CaFe-LDHs interacted with the (OH)- anion, exhibiting a lower crystallinity owing to an increase in the formation of impurities, including Ca(OH)2 and Fe(OH)3. The obtained results demonstrate that well-crystallized single-phase CaFe-LDHs can be synthesized at a pH of 13.
Figure 1A shows the XRD patterns after the thermal treatment. In the CaFe-LDHs calcined at different temperatures, remarkable phase changes were observed. Specifically, the original structure of pristine LDHs was completely destroyed and transformed into Ca2Fe2O5 (JCPDS No. 47-1744) with an orthorhombic structure. A CaCO3 (JCPDS No. 86-2339) impurity phase, indexed to a rhombohedral structure with a main diffraction peak at 2θ = 29.36°, was subsequently generated. As evident from the XRD patterns in Figure 1A, the CaCO3 phase only appeared when pristine LDHs were treated at 400°C under ambient conditions. However, when the C-400-LDO specimen was treated in Ar, no CaCO3 phase was formed (Supplementary Figure S2). This finding suggests that CaCO3 formation is related to CO2 dissolution from the air during the calcination process. As the calcination temperature increased, the CaCO3 phase disappeared, and the crystallization of the CaO phase increased. When the temperature exceeded 550°C, decomposition occurred (Babou-Kammoe et al., 2012; Yamaguchi et al., 2015). The disappearance of CaCO3 can be explained by the following reaction:
FIGURE 1. (A) XRD patterns of Pristine LDHs, C-400 LDOs, and C-700 LDOs with different structural transformations and (B) TGA spectrum.
TGA data were acquired to explore the thermal decomposition of the synthesized CaFe-LDHs. The results are shown in Figure 1B. Four major weight loss stages were observed. The first weight loss stage (11.7 wt%) occurred from room temperature to 200°C and corresponds to the loss of weakly adsorbed water molecules on the crystallite surface. During the second stage of weight loss (9.9 wt%) between 200 and 300°C, the intercalated crystallized water between the cationic layers was removed. The third stage of weight loss (15.86 wt%), which occurred from 300 to 550°C, was associated with the thermal decomposition of hydroxyl (OH−) groups anchored to transition metal oxides and anionic (NO3−) species (Mahjoubi et al., 2017). Finally, the last stage of weight loss (3.7 wt%), which appeared at temperatures above 550°C, was attributed to the decomposition of calcium carbonate (CaCO3), as displayed in Supplementary Figure S3.
The FT-IR spectra of the CaFe-LDH samples were analyzed to identify structural transformations via comparisons of chemical bonding involving various functional groups (Supplementary Figure S4). The characteristic peaks appearing at 1,640 cm−1 are assigned to the H-O-H bending vibrations of interlayer molecular water, whereas the characteristic sharp absorption band around 1,350 cm−1 is associated with the antisymmetric stretching mode of NO3− in the interlayer (Supplementary Figure S4B). The intense broad bands observed at approximately 3,585 cm−1 are associated with the stretching vibrations of the structural (OH−) groups in Ca(OH)2 and Fe(OH)2 (Supplementary Figure S4C). The peaks at 749 and 580 cm−1 are attributed to the stretching vibrations of either Ca-O or Fe-O (Metal-O) in the lattice, as shown in Supplementary Figure S4D (Wu et al., 2012; Mahjoubi et al., 2017). The band at 1,640 cm−1 disappeared completely after calcination in the case of C-400 LDO because the crystallized water molecules were removed. In addition, the gradual decrease in the NO3− stretching vibration intensity at 1,350 cm−1 implies the decomposition of NO3− anions due to an increase in the calcination temperature; complete decomposition occurred in the C-700 LDO sample. A noticeable decrease in peaks corresponding to the OH− group in the case of C-400 LDO implies that the OH− species of the Ca(OH)2 and Fe(OH)2 cationic layers were decomposed when the thermal treatment temperature exceeded 400°C. The subsequent increase in the intensity of the metal-O peaks at 749 and 580 cm−1 indicates that the structure of the pristine LDHs was transformed into that of the respective metal oxides. Peaks corresponding to commercial CaCO3 appeared at 1,394 cm−1 (υ3 asymmetric CO32−) and 871 cm−1 (υ2 asymmetric CO32−) (Butto et al., 2018). The intensity of these bands decreased with increasing calcination temperature. Thus, according to the XRD and TGA findings, a structural transformation occurred in the C-400 LDO specimen.
Figure 2 shows SEM images of the CaFe-LDHs for different thermal treatment conditions. A comparison of the CaFe-LDHs with pristine LDHs revaled transformed morphology in C-400 LDOs. Such a phenomenon is influenced by the destruction of anion layers with the removal of crystalline water molecules. The C-700 LDOs specimen presented a morphology consisting primarily of individual spherical particles.
Figure 3 displays the TEM images and the associated selected area electron diffraction (SAED) patterns obtained for the CaFe-LDH samples. The pristine LDHs showed the typical crystallographic layered structure of CaFe-LDHs, including (300) and (103) planes with lattice constants of 1.70 Å and 2.50 Å, respectively. For C-400 LDOs, the presence of the (006), (110), and (116) planes corresponding to rhombohedral CaCO3 was detected after thermal treatment at 400°C. In the case of calcination at 700°C, only the CaO phase was identified in the XRD pattern (Figure 1A). Here, CaO is a unique by-product formed as a result of thermal treatment. The obtained SAED patterns confirm the occurrence of gradual structural and crystallographic transformations as a function of temperature from 400 to 700°C.
Experiments were conducted to investigate the removal of chloride ions, which may shorten the lifespan of building structures. Figures 4A,B show the results obtained for the removal of chloride at different adsorption times and a fixed ion adsorption time of 60 min, respectively. The percentage removal efficiency was estimated according to Equation (3).
where
FIGURE 4. Ion chromatography of CaFe-LDHs chloride removal (%) at (A) different adsorption times and (B) a fixed adsorption time of 60 min.
As shown in Figure 4A, the removal percentages of pristine LDHs, C-400 LDOs, and C-700 LDOs were 11.49, 16.98, and 57.90%, respectively, for an adsorption time of 5 min at the initial stage of the reaction. The amount of adsorbed chloride increased rapidly over 5 min, with a gradual increase up to 60 min before reaching steady-state equilibrium. The removal percentages for a 60 min adsorption time were 13.41% for pristine LDHs, 29.25% for C-400 LDOs, and 79.62% for C-700 LDOs. Based on the obtained findings, the removal efficiency with C-700 LDOs was almost six times higher than that of pristine LDHs, suggesting outstanding performance in terms of chloride removal.
The FT-IR spectra of the sample upon the adsorption of chloride ions are illustrated in Supplementary Figure S5. The characteristic sharp absorption band at approximately 1,360 cm−1 is associated with the antisymmetric stretching mode of chloride in the interlayer. In addition, in the cases of C-400 LDOs and C-700 LDOs without anion adsorption, typical H-O-H peaks corresponding to bending vibrations (1,640 cm−1) and stretching vibrations (3,585 cm−1) induced by (OH)- groups were removed (see comment in Supplementary Figure S4). However, the adsorption peaks reappeared after chloride adsorption due to the recovery of (OH)- groups and crystalline water molecules during the reconstruction process in an aqueous atmosphere. Hence, the results provide evidence for both the reconstruction processes and the successful adsorption of chloride ions by CaFe-LDOs.
The reconstructed CaFe-LDHs and LDOs after 60 min of chloride adsorption were characterized by XRD. The results are shown in Figure 5. Characteristic peaks that were typical of CaFe-LDHs were indexed to the rhombohedral structure for all LDH samples, including those from the (006) and (0012) planes after the adsorption of chloride ions. Upon chloride adsorption, the XRD patterns of the crystalline LDHs corresponded to a structure with a chemical formula Ca2Fe(OH)6(Cl)∙2H2O (JCPDS No.44-445). The crystallinity of the CaFe-LDHs simultaneously decreased with anion adsorption due to the reconstruction phenomenon (i.e., memory effect). Furthermore, the basal spacing increased, and the 2θ angle associated with the (006) XRD peak increased from 10.36° (NO3−) to 11.32° (chloride) because the ionic radius of the chloride ion (1.75 Å) is smaller than that of NO3− (1.79 Å). The chloride ion was partially substituted with NO3− present in the interlayer, which led to the formation of two types of interstratified pristine LDHs. The pre-adsorption data are shown in Figure 1A. The XRD pattern of the post-adsorption C-400 LDOs also included secondary crystalline phases of CaCO3 (2θ = 29.36°) even after particle reconstruction because CaCO3 is a basic salt with very low solubility. Therefore, the layered CaFe- LDOs reconstructed via refolding in aqueous solution could exchange chloride anions due to the reconstruction of Ca2Fe2O5 as follows (4):
FIGURE 5. XRD patterns of before the chloride adsorption (Raw LDHs), Pristine LDHs, C-400 LDOs, and C-700 LDOs after chloride adsorption.
In particular, the CaFe-LDOs prepared after thermal treatment at 700°C showed the pure crystalline structure of CaFe-LDHs with individual interlayer anions of Cl−, Ca(OH)2, and CaCl2. This was because the secondary crystalline phase of CaO (see Figure 1A) after anion adsorption was transformed to Ca(OH)2 via hydrolysis in solution. The results suggest adsorption from partial Ca(OH)2 phases to CaCl2. Therefore, the C-700 LDOs sample undergoes the following chemical reaction when reconstructed in water containing chloride (5):
This mechanism was induced by the following factors: 1) anion capture by reconstruction, 2) capture by chemical reaction with metal oxide, and 3) physical adsorption. The C-700 LDOs can capture 4 mol of Cl− (see Eq. (5)). Here, 1 mol of Cl− was captured by intercalation between cationic layers during the refolding process, and additional 3 mol of Cl− were captured by chemical reaction with CaO, which was generated during thermal treatment at 700°C. This metal oxide moiety, initiated by pristine-LDHs, also captures Cl− by chemical reactions other than capture by the refolding process, which cannot be realized even after the calcination of typical LDHs (Wu et al., 2012; Halajnia et al., 2013; Zhou et al., 2015). Supplementary Figure S6 shows SEM images of the reconstructed pristine LDHs, C-400 LDOs, and C-700 LDOs before (top) and after anion adsorption (bottom). Although no significant changes were observed for the reconstructed LDHs, differences in morphology between the pristine LDHs and C-700 LDOs are evident. Although the morphologies of pristine LDHs before and after anion adsorption appear to be similar, the post-anion adsorption C-700 LDOs exhibit larger plate-like particles aggregated into flower-like shapes. Such a microstructure may be attributed to the change in the crystallinity of the remaining Ca(OH)2 phases, as expressed by Eq. 9. The removal of chloride ions by CaFe-LDOs particles could be influenced by structural tuning. In particular, the CaFe-LDOs exhibited different efficiencies (%) for anion removal, which could be enhanced by using CaFe-LDOs rather than pristine LDHs.
To investigate the difference in anion adsorption by the CaFe-LDHs and LDOs, the adsorption kinetics of chloride ions onto the CaFe-LDHs and LDOs were fitted to a linearized form of pseudo-first-order (Eq. 6) and pseudo-second-order (Eq. 7) models based on experimental data (Calisto et al., 2019; Rathee et al., 2019):
where
FIGURE 6. (A) Pseudo-first-order and (B) pseudo-second-order kinetic plots for the sorption of chloride on Pristine LDHs, C-400 LDOs and C-700 LDOs.
TABLE 2. Coefficients of pseudo-first-order and pseudo-second-order parameters for chloride adsorption by pristine LDHs, C- 400 LDOs, and C-700 LDOs.
The aim of adsorption isotherms is to correlate the adsorbate concentration in the solution with the adsorbed content of metal ions at the adsorbate-to-adsorbent interface. Langmuir (Figure 7A) and Freundlich (Figure 7B) isotherm models are crucial for the design of sorption systems. The adsorption of chloride ions onto CaFe-LDH and LDO adsorbents was investigated, and the applicability of the aforementioned isotherms was examined. The Langmuir model assumes a homogenous surface via monolayer adsorption between the adsorbed ions, and the Langmuir isotherm represents the equilibrium distribution of sorbate between the solid and liquid phases. This isotherm is often expressed as Equation 8 (Yan et al., 2016; Mu’azu et al., 2018):
where
FIGURE 7. (A) Langmuir and (B) Freundlich plots for the sorption of chloride onto Pristine LDHs, C-400 LDOs, and C-700 LDOs.
The Freundlich equation can be utilized to estimate the sorption intensity of the sorbent toward the sorbate, and is expressed by Equation 9 (Peng et al., 2015; Santos R. M. M.d. et al., 2017):
where
TABLE 3. Langmuir and Freundlich isotherm parameters for chloride adsorption by pristine LDHs, C- 400 LDOs, and C-700 LDOs.
TABLE 4. Comparison of LDHs removal capacity for chloride anions obtained in this work and in previous reports.
To study the anti-corrosion effects of CaFe-LDHs and LDOs on iron alloys from destructive chloride anions, potentiodynamic measurements of the exponential dependence of the current on voltage deviations were conducted in an aqueous 3.5 wt% NaCl solution; the results are depicted in Figure 8. The variations in the electrochemical kinetic parameters, such as the corrosion current (Icorr) and corrosion rate (CR), were determined from the curves using the extrapolation method (as plotted in Figure 8 and supplemented by Table 5).
FIGURE 8. Potentiodynamic polarization (PDP) curves obtained in a 3.5 wt% NaCl solution without LDHs or LDOs and with Pristine LDHs, C-400 LDOs, and C-700 LDOs.
TABLE 5. Electrochemical polarization parameters and calculated corrosion rates (mm/year) in a 3.5 wt% NaCl solution with and without LDHs or LDOs.
The corrosion rates (mm year−1) obtained from the polarization were calculated using Equation 10:
Here, EW denotes the equivalent weight of Fe (27 g), K represents the corrosion rate constant (3,272 mm year−1A−1 cm−1), denotes the density of Fe (7.874 gcm−3), and A (cm2) denotes the surface area of the electrode.
The CR for iron alloys with the application of C-700 LDHs was 89.28 × 10–3 mm/year, whereas the CR in the case without LDHs and LDOs was 2390.69 × 10–3 mm/year. These findings indicate that when iron alloys are placed in a severe atmosphere with a high concentration of chlorides in solution, the speed of corrosion is accelerated to a maximum of 26.7 times over a year unless the iron alloys are not protected. As can be seen from the results of the Nyquist plot for iron alloys upon the application of CaFe-LDHs and LDOs (Figure 9A), the mitigation of corrosion led to a clear increase in the diameter of the capacitive loop. The diameter of the semicircle was also significantly enlarged for the protective LDH and LDO samples containing more charge-transfer resistance, as confirmed by the Bode plots in Figure 9B. To quantitatively determine the effects of various factors, including the addition of CaFe-LDHs and LDOs, immersion time, and chloride content, a numerical simulation must be employed to fit the obtained EIS results. Because the degree of chloride removal from the solution containing the iron alloy specimen is an indicator of corrosion protection, the equivalent circuit model constants in Figure 9D were applied. In the equivalent circuit model, iron alloys are considered to have a porous structure and exhibit capacitive behavior (Hou et al., 2019; Imanieh and Afshar, 2019; Liu et al., 2019; Xu et al., 2020). The relevant parameters are listed in Table 6, where Rs denotes the electrolyte resistance, Rf denotes the electrolyte resistance inside the pores, Cf represents the film capacitance, Rct represents the charge transfer resistance of the electrochemical processes occurring inside the pores, and Cdl denotes the double-layer capacitance. The Cdl value for pristine LDHs was 3.26 × 10–3 (µF·cm−2), and this parameter generally decreased with higher-temperature heat treatment; the Cdl values of C-400 LDOs and C-700 LDOs were 2.65 × 10–3 (µF·cm−2) and 2.45 × 10–3 (µF·cm−2), respectively. This phenomenon can be explained according to the Helmholtz model, where Cdl is expressed as follows (11) [15]:
where S denotes the exposed surface area of the steel specimen, d represents the thickness of the double layer, εr denotes the dielectric constant of the electrolyte inside the capacitor, and ε0 denotes the vacuum dielectric constant. Here, an increase in Cdl indicates an increase in the exposed surface area of the bare iron alloys by corrosion. The addition of LDHs and LDOs decreases the aggressive chlorination reaction (see Equations 1–4 and corrosion of the steel specimen is significantly alleviated. Hence, the reduction in the Cdl value is mainly attributed to a decrease in the exposed iron alloy surface area due to the presence of the LDHs. It is well known that the corrosion rate of steel is inversely proportional to the Rct value. In a solution without LDHs, the Rct value was 39.57 Ω cm2. However, the Rct value with pristine LDHs was 159.62 Ω cm2, and this parameter gradually increased to a maximum of 3,520.01 Ω cm2 for C-700 LDOs. To more clearly illustrate the corrosion mitigation effects of CaFe-LDHs and LDOs, the inhibition efficiency (IE%) of the CaFe-LDHs and LDOs was calculated according to the following Equation 12:
where Rct, with LDHs and LDOs, and Rct, without LDHs, represent the charge transfer resistances of the steel specimens in the presence and absence of CaFe-LDHs and LDOs, respectively. From Table 6, it is evident that the solution containing both the iron alloy specimen and CaFe-LDHs and LDOs showed an inhibitory effect, whereas no effect was observed in the test solution without LDHs. In particular, the highest inhibition efficiency of 98.87% was achieved with the C-700 LDOs.
FIGURE 9. (A) Nyquist plots, (B) Bode plots, and (C) phase angle plots collected at corrosion potentials in a 3.5 wt% NaCl solution without LDHs or LDOs and with Pristine LDHs, C-400 LDOs, and C-700 LDOs. (D) Equivalent electrical circuit (EEC) used to fit the electrochemical impedance data.
TABLE 6. Impedance parameters and calculated inhibition efficiencies (IE%) in a 3.5 wt% NaCl solution with and without LDHs or LDOs.
Structure-modulated CaFe-LDHs were fabricated at room temperature using a modified co-precipitation method. A high Cl− adsorption capability of 881.83 mg/g was achieved with C-700 LDOs (LDHs heat treated at 700°C). The chloride removal capability exceeded three times the maximum performance in previous studies (257 mg/g) on chloride removal by LDHs. It was also confirmed that the kinetics were well fitted by a pseudo-second-order model, suggesting dominant chemical adsorption on CaFe-LDHs and LDOs. The Langmuir isotherm models confirmed that the LDHs and LDOs effectively removed the target anions, primarily via monolayer sorption. Moreover, it was also confirmed that crystalline CaO was formed as a C-700 LDO moiety during the heat treatment of pristine CaFe-LDHs, and this phase served as another source to enhance the Cl− removal by undergoing a chemical reaction. In particular, the highest inhibition efficiency of 98.87% was achieved with C-700 LDOs compared to iron alloys without LDHs. Based on the results obtained in this study, CaFe-LDOs demonstrate considerable potential for use as concrete fillers or additives in the construction industry to enhance the durability/sustainability of iron alloy reinforced buildings.
The original contributions presented in the study are included in the article/Supplementary Material, further inquiries can be directed to the corresponding author.
JP conceived of the experiments and prepared the manuscript. SY helped perform the analysis and discussed the results. H-BC and H-SL contributed to editing. Y-HC contributed to the manuscript, accepted responsibility for the conducted research, and provided final approval. All authors have given approval to the final version of the manuscript.
This work was supported by a National Research Foundation of Korea (NRF) grant funded by the Korean government (MSIT) (No. 2015R1A5A1037548); the Industrial Strategic Technology Development Program (20010460, Developing the Ceramic ALD Precursors with High Corrosion Resistance and Core Parts of Deposition Etching Equipment for High Density Semiconductors) funded by the Ministry of Trade, Industry and Energy (MOTIE, Korea); and the Technology Innovation Program (20002694, Gas sensor) funded by the Ministry of Trade, Industry and Energy (MOTIE, Korea).
Author SY was employed by the company Park Systems Corporation.
The remaining authors declare that the research was conducted in the absence of any commercial or financial relationships that could be construed as a potential conflict of interest.
All claims expressed in this article are solely those of the authors and do not necessarily represent those of their affiliated organizations, or those of the publisher, the editors and the reviewers. Any product that may be evaluated in this article, or claim that may be made by its manufacturer, is not guaranteed or endorsed by the publisher.
The Supplementary Material for this article can be found online at: https://www.frontiersin.org/articles/10.3389/fchem.2022.813008/full#supplementary-material
Babou-Kammoe, R., Hamoudi, S., Larachi, F., and Belkacemi, K. (2012). Synthesis of CaCO3 Nanoparticles by Controlled Precipitation of Saturated Carbonate and Calcium Nitrate Aqueous Solutions. Can. J. Chem. Eng. 90, 26–33. doi:10.1002/cjce.20673
Bassuoni, M. T., and Nehdi, M. L. (2008). Durability of Self-Consolidating concrete to Different Exposure Regimes of Sodium Sulfate Attack. Mater. Struct. 42, 1039–1057. doi:10.1617/s11527-008-9442-2
Benício, L. P. F., Silva, R. A., Lopes, J. A., Eulálio, D., Santos, R. M. M. d., Aquino, L. A. d., et al. (2015). LAYERED DOUBLE HYDROXIDES: NANOMATERIALS FOR APPLICATIONS IN AGRICULTURE. Rev. Bras. Ciênc. Solo 39, 1–13. doi:10.1590/01000683rbcs2015081
Berrocal, C. G., Lundgren, K., and Löfgren, I. (2016). Corrosion of Steel Bars Embedded in Fibre Reinforced concrete under Chloride Attack: State of the Art. Cement Concrete Res. 80, 69–85. doi:10.1016/j.cemconres.2015.10.006
Butto, N., Cabrera-Barjas, G., and Neira-Carrillo, A. (2018). Electrocrystallization of CaCO3 Crystals Obtained through Phosphorylated Chitin. Crystals 8, 82. doi:10.3390/cryst8020082
Calisto, J. S., Pacheco, I. S., Freitas, L. L., Santana, L. K., Fagundes, W. S., Amaral, F. A., et al. (2019). Adsorption Kinetic and Thermodynamic Studies of the 2, 4 – Dichlorophenoxyacetate (2,4-D) by the [Co–Al–Cl] Layered Double Hydroxide. Heliyon 5, e02553. doi:10.1016/j.heliyon.2019.e02553
Chen, Y., Gao, J., Tang, L., and Li, X. (2016). Resistance of concrete against Combined Attack of Chloride and Sulfate under Drying-Wetting Cycles. Construction Building Mater. 106, 650–658. doi:10.1016/j.conbuildmat.2015.12.151
Dorn, J. E. (1953). “Structure of Metals: Crystallographic Methods, Principles, and Data,”. Editor S. B. Charles. 2nd (New York-London: McGraw-Hill), 117, 421–422. Science 1952. 661 pp. $10.00. doi:10.1126/science.117.3042.421-a
Erickson, K. L., Bostrom, T. E., and Frost, R. L. (2005). A Study of Structural Memory Effects in Synthetic Hydrotalcites Using Environmental SEM. Mater. Lett. 59, 226–229. doi:10.1016/j.matlet.2004.08.035
Evans, D. G., and Duan, X. (2006). Preparation of Layered Double Hydroxides and Their Applications as Additives in Polymers, as Precursors to Magnetic Materials and in Biology and Medicine. Chem. Commun. 37, 485–496. doi:10.1039/b510313b
Gupta, N. K., Saifuddin, M., Kim, S., and Kim, K. S. (2020). Microscopic, Spectroscopic, and Experimental Approach towards Understanding the Phosphate Adsorption onto Zn–Fe Layered Double Hydroxide. J. Mol. Liquids 297, 111935. doi:10.1016/j.molliq.2019.111935
Halajnia, A., Oustan, S., Najafi, N., Khataee, A. R., and Lakzian, A. (2013). Adsorption-desorption Characteristics of Nitrate, Phosphate and Sulfate on Mg-Al Layered Double Hydroxide. Appl. Clay Sci. 80-81, 305–312. doi:10.1016/j.clay.2013.05.002
Hou, L., Li, Y., Sun, J., Zhang, S. H., Wei, H., and Wei, Y. (2019). Enhancement Corrosion Resistance of Mg Al Layered Double Hydroxides Films by Anion-Exchange Mechanism on Magnesium Alloys. Appl. Surf. Sci. 487, 101–108. doi:10.1016/j.apsusc.2019.05.048
Imanieh, I., and Afshar, A. (2019). Corrosion protection of Aluminum by Smart Coatings Containing Layered Double Hydroxide (LDH) Nanocontainers. J. Mater. Res. Technology 8, 3004–3023. doi:10.1016/j.jmrt.2018.05.030
Ivánová, D., Albert, P., and Kavuličová, J. (2018). Nitrate Removal from Model Aqueous Solutions and Real Water by Calcined Mg/Al Layered Double Hydroxides. Appl. Clay Sci. 152, 65–72. doi:10.1016/j.clay.2017.10.033
Ke, X., Bernal, S. A., and Provis, J. L. (2017). Uptake of Chloride and Carbonate by Mg-Al and Ca-Al Layered Double Hydroxides in Simulated Pore Solutions of Alkali-Activated Slag Cement. Cement Concrete Res. 100, 1–13. doi:10.1016/j.cemconres.2017.05.015
Kong, X., Ge, R., Liu, T., Xu, S., Hao, P., Zhao, X., et al. (2021). Super-stable Mineralization of Cadmium by Calcium-Aluminum Layered Double Hydroxide and its Large-Scale Application in Agriculture Soil Remediation. Chem. Eng. J. 407, 127178. doi:10.1016/j.cej.2020.127178
Liu, A., Ju, X., Tian, H., Yang, H., and Li, W. (2019). Direct Synthesis of Layered Double Hydroxides Monolayer Nanosheets for Co-assembly of Nanobrick wall Hybrid Film with Excellent Corrosion Resistance. Appl. Surf. Sci. 493, 239–249. doi:10.1016/j.apsusc.2019.06.295
Lv, W., Yang, L., Fan, B., Zhao, Y., Chen, Y., Lu, N., et al. (2015). Silylated MgAl LDHs Intercalated with MnO2 Nanowires: Highly Efficient Catalysts for the Solvent-free Aerobic Oxidation of Ethylbenzene. Chem. Eng. J. 263, 309–316. doi:10.1016/j.cej.2014.11.009
Mahjoubi, F. Z., Khalidi, A., Abdennouri, M., and Barka, N. (2017). Zn-Al Layered Double Hydroxides Intercalated with Carbonate, Nitrate, Chloride and Sulphate Ions: Synthesis, Characterisation and Dye Removal Properties. J. Taibah Univ. Sci. 11, 90–100. doi:10.1016/j.jtusci.2015.10.007
Mishra, G., Dash, B., and Pandey, S. (2018). Layered Double Hydroxides: A Brief Review from Fundamentals to Application as Evolving Biomaterials. Appl. Clay Sci. 153, 172–186. doi:10.1016/j.clay.2017.12.021
Mu'azu, N. D., Jarrah, N., Kazeem, T. S., Zubair, M., and Al-Harthi, M. (2018). Bentonite-layered Double Hydroxide Composite for Enhanced Aqueous Adsorption of Eriochrome Black T. Appl. Clay Sci. 161, 23–34. doi:10.1016/j.clay.2018.04.009
Muthulingam, S., and Rao, B. N. (2015). Non-uniform Corrosion States of Rebar in concrete under Chloride Environment. Corrosion Sci. 93, 267–282. doi:10.1016/j.corsci.2015.01.031
Peng, C., Dai, J., Yu, J., and Yin, J. (2015). Calcined Mg-Fe Layered Double Hydroxide as an Absorber for the Removal of Methyl orange. AIP Adv. 5, 057138. doi:10.1063/1.4921455
Pradhan, B., and Bhattacharjee, B. (2011). Rebar Corrosion in Chloride Environment. Construction Building Mater. 25, 2565–2575. doi:10.1016/j.conbuildmat.2010.11.099
Rathee, G., Awasthi, A., Sood, D., Tomar, R., Tomar, V., and Chandra, R. (2019). A New Biocompatible Ternary Layered Double Hydroxide Adsorbent for Ultrafast Removal of Anionic Organic Dyes. Sci. Rep. 9, 16225. doi:10.1038/s41598-019-52849-4
Romero Ortiz, G., Lartundo-Rojas, L., Samaniego-Benítez, J. E., Jiménez-Flores, Y., Calderón, H. A., and Mantilla, A. (2021). Photocatalytic Behavior for the Phenol Degradation of ZnAl Layered Double Hydroxide Functionalized with SDS. J. Environ. Manage. 277, 111399. doi:10.1016/j.jenvman.2020.111399
Santos, R. M. M. d., Gonçalves, R. G. L., Constantino, V. R. L., Santilli, C. V., Borges, P. D., Tronto, J., et al. (2017b). Adsorption of Acid Yellow 42 Dye on Calcined Layered Double Hydroxide: Effect of Time, Concentration, pH and Temperature. Appl. Clay Sci. 140, 132–139. doi:10.1016/j.clay.2017.02.005
Santos, R. M. M., Tronto, J., Briois, V., and Santilli, C. V. (2017a). Thermal Decomposition and Recovery Properties of ZnAl-CO3 Layered Double Hydroxide for Anionic Dye Adsorption: Insight into the Aggregative Nucleation and Growth Mechanism of the LDH Memory Effect. J. Mater. Chem. A. 5, 9998–10009. doi:10.1039/c7ta00834a
Wu, Y., Yu, Y., Zhou, J. Z., Liu, J., Chi, Y., Xu, Z. P., et al. (2012). Effective Removal of Pyrophosphate by Ca-Fe-LDH and its Mechanism. Chem. Eng. J. 179, 72–79. doi:10.1016/j.cej.2011.10.053
Xu, J., Tan, Q., and Mei, Y. (2020). Corrosion protection of Steel by Mg-Al Layered Double Hydroxides in Simulated concrete Pore Solution: Effect of SO42-. Corrosion Sci. 163, 108223. doi:10.1016/j.corsci.2019.108223
Yamaguchi, N., Masuda, Y., Yamada, Y., Narusawa, H., Han-Cheol, C., Tamaki, Y., et al. (2015). Synthesis of CaO-SiO2 Compounds Using Materials Extracted from Industrial Wastes. Ojinm 05, 1–10. doi:10.4236/ojinm.2015.51001
Yan, Z., Zhu, B., Yu, J., and Xu, Z. (2016). Effect of Calcination on Adsorption Performance of Mg-Al Layered Double Hydroxide Prepared by a Water-In-Oil Microemulsion Method. RSC Adv. 6, 50128–50137. doi:10.1039/c6ra05253c
Yang, Z., Fischer, H., and Polder, R. (2013). Modified Hydrotalcites as a New Emerging Class of Smart Additive of Reinforced concrete for Anticorrosion Applications: A Literature Review. Mater. Corrosion 64, 1066–1074. doi:10.1002/maco.201206915
Yoon, S., Moon, J., Bae, S., Duan, X., Giannelis, E. P., and Monteiro, P. M. (2014). Chloride Adsorption by Calcined Layered Double Hydroxides in Hardened Portland Cement Paste. Mater. Chem. Phys. 145, 376–386. doi:10.1016/j.matchemphys.2014.02.026
Zaghouane-Boudiaf, H., Boutahala, M., Tiar, C., Arab, L., and Garin, F. (2011). Treatment of 2,4,5-trichlorophenol by MgAl-SDBS Organo-Layered Double Hydroxides: Kinetic and Equilibrium Studies. Chem. Eng. J. 173, 36–41. doi:10.1016/j.cej.2011.07.032
Zhou, J., Li, X., Li, W., Wei, F., Su, Y., Zhang, J., et al. (2015). Multi-step Removal Mechanism of Pyrophosphate Using CaFe-Layered Double Hydroxide at High pH. Appl. Clay Sci. 105-106, 21–26. doi:10.1016/j.clay.2014.12.001
Keywords: layered double hydroxide, cafe, corrosion, chloride, iron alloy
Citation: Park JY, Yoo SB, Cho H-, Lee H- and Choa Y- (2022) CaFe-Based Layered Double Oxides With Superior Iron Alloy Corrosion Inhibition Behaviors in Aggressive Seawater Environment. Front. Chem. 10:813008. doi: 10.3389/fchem.2022.813008
Received: 11 November 2021; Accepted: 12 January 2022;
Published: 07 February 2022.
Edited by:
Cheng Zhong, Tianjin University, ChinaReviewed by:
Tamara Posati, National Research Council (CNR), ItalyCopyright © 2022 Park, Yoo, Cho, Lee and Choa. This is an open-access article distributed under the terms of the Creative Commons Attribution License (CC BY). The use, distribution or reproduction in other forums is permitted, provided the original author(s) and the copyright owner(s) are credited and that the original publication in this journal is cited, in accordance with accepted academic practice. No use, distribution or reproduction is permitted which does not comply with these terms.
*Correspondence: Yong-Ho Choa, Y2hvYTE1QGhhbnlhbmcuYWMua3I=
Disclaimer: All claims expressed in this article are solely those of the authors and do not necessarily represent those of their affiliated organizations, or those of the publisher, the editors and the reviewers. Any product that may be evaluated in this article or claim that may be made by its manufacturer is not guaranteed or endorsed by the publisher.
Research integrity at Frontiers
Learn more about the work of our research integrity team to safeguard the quality of each article we publish.