- 1Department of Chemistry, Nelson Mandela University, Gqeberha (Port Elizabeth), South Africa, Nelson Mandela University, Gqeberha, South Africa
- 2Research and Development Division, Sasol Technology (Pty) Ltd, Sasolburg, South Africa
Reliance on crude oil remains high while the transition to green and renewable sources of fuel is still slow. Developing and strengthening strategies for reducing sulfur emissions from crude oil is therefore imperative and makes it possible to sustainably meet stringent regulatory sulfur level legislations in end-user liquid fuels (mostly less than 10 ppm). The burden of achieving these ultra-low sulfur levels has been passed to fuel refiners who are battling to achieve ultra-deep desulfurization through conventional hydroprocessing technologies. Removal of refractory sulfur-containing compounds has been cited as the main challenge due to several limitations with the current hydroprocessing catalysts. The inhibitory effects of nitrogen-containing compounds (especially the basic ones) is one of the major concerns. Several advances have been made to develop better strategies for achieving ultra-deep desulfurization and these include: improving hydroprocessing infrastructure, improving hydroprocessing catalysts, having additional steps for removing refractory sulfur-containing compounds and improving the quality of feedstocks. Herein, we provide perspectives that emphasize the importance of further developing hydroprocessing catalysts and pre-treating feedstocks to remove nitrogen-containing compounds prior to hydroprocessing as promising strategies for sustainably achieving ultra-deep hydroprocessing.
Introduction
Crude oil, and fossil fuels in general, were formed when large amounts of dead organisms buried under sedimentary rock were subjected to intense heat and pressure (Höök et al., 2010). It is predominantly composed of hydrocarbons that are desirable for energy needs (Sami and Hatch, 2001). Demand for petroleum is especially high in motorized transport, particularly long haul air and sea transport, due to its high volumetric density (Saleh, 2015). Consumption of crude oil rose from around 70 million barrels per day (Mb/d) in 1995 to 80 and 95 Mb/d in 2005 and 2015, and to over 100 Mb/d in 2019 (Statistical Review of World Energy, 2022). The overall share of renewable energy sources remains relatively low at 5.7% (Statistical Review of World Energy, 2022). To bridge the gap in adoption of renewables, legislation is being implemented to minimize fossil fuel based emissions, reduce their carbon footprint, and to develop strategies for CO2 capture, storage and utilization.
The hydrocarbon composition of crude oil is accompanied by several other elements such as oxygen, sulfur, nitrogen, and metals which give rise to most of the challenges associated with its handling, storage and use (Théodet, 2010). Sulfur and nitrogen pose the biggest challenges (Hansmeier et al., 2011). As a result, sweet crude oils (crude oils with lower contamintants, especially sulfur and nitrogen), are generally preferred compared to sour crude oils (crude oils with higher concentrations of contaminants). At the projected demands, the preferred sweet crude sources will soon be depleted forcing the switch to more sour crude sources (Campbell and Laherrère, 1998; Dincer and Zamfirescu, 2018; Bardi, 2019). More than 70% of the world’s oil reserves are classified to be of heavier and sourer composition (World Energy Review, 2020).
Due to the rising demand for “clean” energy, pressure is mounting for the world to adopt cleaner energy technologies and improve the use of fossil fuels. Crude oil refining will be with us for a while to come so it is crucial that we continue to develop the technology. Conventionally, hydroprocessing is used to remove sulfur-containing compounds. Several other methods such as oxidative desulfurization, biodesulfurization, extractive desulfurization, and adsorptive desulfurization are also being pursued. It has been difficult to replace hydroprocessing as the industrially preferred method. As such, this work provides perspectives on the advances that are being made in improving the hydroprocessing technology to achieve ultra-deep desulfurization. Emphasis is placed on hydroprocessing catalyst development and improvement of feedstock through pre-treatment to remove nitrogen-containing compounds.
Sulfur- and nitrogen-containing compounds in crude oil
Sulfur and nitrogen content of crude oil is known to vary significantly depending on the source and there is no relationship between the concentrations of the two (Prado et al., 2017). The sulfur- and nitrogen-containing compounds are predominantly in aromatic form and generally increase with the heaviness of the crude source (Wiwel et al., 2000; Zeuthen et al., 2001). In addition to other factors, the sulfur and nitrogen content of crude oils determine their acceptability as feedstock in the refinery industry (Choudhary et al., 2008).
After carbon and hydrogen, sulfur is the next most abundant element in most crude oils (Javadli and de Klerk, 2012). Sulfur levels in crude oils are usually in the range 0.1–5.0 wt% and most have more than 1.0 wt% sulfur and are classified as sour; 0.5–1.0 wt%S is considered medium sour while <0.5 wt%S is considered sweet (World Energy Review, 2020). Sulfur-containing compounds are present in two forms; inorganic (e.g. suspended or dissolved elemental sulfur, hydrogen sulfide and pyrite) and organic (thiols and thiophenic compounds), the latter being the more abundant form (Javadli and de Klerk, 2012). Benzothiophenes and dibenzothiophenes are the most dominant sulfur-containing compounds in heavier fuels. Sulfur can also be found in combination with other heteroatoms and some sulfur-containing compounds may have more than one sulfur atoms. Sulfones and sulfoxides can also be found due to naturally occurring oxidation (Javadli and de Klerk, 2012).
Nitrogen levels in crude oil are generally lower compared to sulfur levels. About 90% of crude oils are classified as nitrogen poor, having less than 0.25 wt% nitrogen content while most are below 0.1 wt% (Prado et al., 2017). Four chemical classes of nitrogen-containing compounds are found in fuel oils: aliphatic amines, anilines, and two heterocyclic aromatic compound groups (five-membered pyrrolic and six-membered pyridinic compounds). Aliphatic amines and anilines are not present in significant amounts in most fuel oils (Wiwel et al., 2000). Aliphatic amines, anilines and six-membered pyridinic compounds are classified as basic nitrogen-containing compounds (pKa ≥ 2) while the five-membered compounds are classified as neutral, non-basic or acidic (pKa < 2) (Richter et al., 1952; Wiwel et al., 2000; Prado et al., 2017). The ratio of basic nitrogen to non-basic nitrogen remains within the range 0.25–0.35 irrespective of the origin of the crude oil (Prado et al., 2017). The most common basic nitrogen-containing compounds in fuel oils are pyridine, acridine, quinoline and their derivatives, and the most common neutral nitrogen-containing compounds are pyrrole, indole, carbazole and their derivatives (Asumana et al., 2011). In most cases, 75% of the total nitrogen content in the straight run gas oil and light cycle oil used as feed stocks for diesel fuel production consist of indole and carbazole type compounds while the 25% is constituted mainly of quinoline derivatives (Laredo et al., 2002; Prado et al., 2017). Heavier crudes (e.g. bitumen and tar sands) are associated with very high nitrogen content, mostly basic nitrogen-containing compounds, particularly quinoline derivatives (Wiwel et al., 2000; Javadli and de Klerk, 2012).
Challenges associated with sulfur and nitrogen containing-compounds in liquid fuels
The primary concern of having sulfur-containing compounds in fuel oil is that upon combustion they produce sulfur oxides (especially sulfur dioxide) that have devastating effects on the environment, biodiversity and human health (Schlesinger and McQueen, 2010; Hansmeier et al., 2011; Thurston, 2017). Diesel engines operate at higher temperatures and pressures producing more sulfur oxide emissions than gasoline engines (Zolotareva et al., 2019). The gases aggravate the contribution of motorized transport to global warming as they poison catalysts required in vehicle emission control systems to minimize the escape of carbon monoxide (Hansmeier et al., 2011).
Sulfur- and nitrogen-containing compounds affect fuel storage and handling (Abro et al., 2016). Nitrogen-containing compounds cause storage problems due to instability and degradation which leads to formation of gums. Five-membered nitrogen-containing heterocycles such as pyrroles can easily undergo oxidative or thermal free-radical addition reactions to form heavy polymeric products commonly observed as gums or red tars (Abro et al., 2016). Sulfur-containing compounds are mostly responsible for promoting gum formation, stabilizing gum and may also react with some components of fuel oil to form materials of high molecular weight (Thompson et al., 1949). The gums formed are undesirable in storage tanks and tanks of vehicles, and negatively impact refinery processes and engine performance.
To make matters worse, sulfur and nitrogen-containing compounds interfere with the processes that are used to remove them from fuel oil. The compounds cause fouling of process equipment (Prado et al., 2017). They also inhibit the catalysts used during fuel refining processes such as hydrocracking and hydrotreatment, of which nitrogen-containing compounds are the main culprits (Sau et al., 2005). That makes sourer crudes difficult to process using conventional methods. High concentrations of refractory sulfur-containing compounds in sour crudes also persistently remain in the final product after most treatment procedures (Hansmeier et al., 2011; Forte et al., 2014).
It is evident that nitrogen-containing compounds are the ones that are mostly associated with the numerous challenges around the processing, handling, and storage of fuel. However, they are often “ignored” in favour of sulfur-containing compounds as they are usually much lower that sulfur-containing compounds and are removed simultaneously during hydrotreatment (Wiwel et al., 2000; Hansmeier et al., 2011; Prado et al., 2017). Considering the drive to achieve ultra-low sulfur levels of sulfur and also pave way for the utilization of sourer crudes, it is now imperative to find ways of removing even the small amounts of nitrogen-containing compounds from crude oil to prevent the inhibition of catalysts used in downstream processes such as hydrocracking and hydrotreatment.
Regulation of sulfur and nitrogen levels in liquid fuels
Most governments have come up with strict legislations to curb the release of sulfur oxides into the environment, by primarily monitoring the amounts of sulfur in carburant fuels. The monitoring started as early as 1990 with specifications for different types of vehicles and designated areas (e.g. urban, highway, off-road) and the policies now apply across all vehicle types and areas (US: Fuels: Diesel and Gasoline, 2021; Fuel Regulation, 2022a; Fuel Regulation, 2022b). The most recent Clean Air Act (2021) from the United States Environmental Protection Agency requires liquid fuels to have less that 15 ppm sulfur content (Environmental Protection Agency, 2021). Euoropean Union sulfur limits are set under Euro VI at 10 ppm (ICCT, 2020). Brazil has standards equivalent to the Euro V but additionally banning the sale of diesel cars in the country while promoting ethanol and biofuel blending (Zolotareva et al., 2019; ICCT, 2022). From 2013, major metropolitan areas and selected stations around the country were able to supply 10 ppm diesel to trucks (ICCT, 2022). China’s vehicle emissions standards are also equivalent to the Euro V, being China V for light-duty vehicles and China V for heavy-duty vehicles (Zolotareva et al., 2019).
Ships have been lagging behind although some marine areas have been declared to be designated emission-controlled areas (along the shorelines) where ships are required to use light distillate fuels such as marine gas oil to reduce pollution levels. USA and Canada applied to the International Maritime Organization to establish emission control areas along their shorelines where the sulfur limit was 10,000 ppm from 2010 to 1000 ppm from 2015, marking the beginning of international regulation of sulfur limits in marine fuels (IMO, 2022). In 2020 the MARPOL convention introduced the so-called “IMO-2020” regulation which cut the sulfur content in the marine fuel from 3.5% m/m down to 0.5% m/m outside designated emission control areas. The sulfur specification is even lower at 0.1% m/m for designated emission-control areas.
African countries have also been lagging behind. Morocco was the pioneering country in Africa starting with 50 ppm diesel in 2009 and then 10 ppm diesel becoming available from 2011 (UNO, 2022). Other leading countries in adopting legislation for low sulfur levels in fuel Africa are Mauritius, Kenya, Uganda, Tanzania, Rwanda, Burundi, Ghana, Mozambique, Malawi and Zimbabwe (UNO, 2022). In Mauritius 50 ppm diesel had become a standard across all fuel stations from 2012. Mozambique implemented 50 ppm limit for gasoline in 2017. Zimbabwe implemented the 50 ppm limit for diesel in 2018 with a goal to progressively phase out 500 ppm diesel. However, despite African countries adopt the legislation for low sulfur diesel implementation remains a challenge as can be seen from Figure 1 (Global Fuel Specification, 2022).
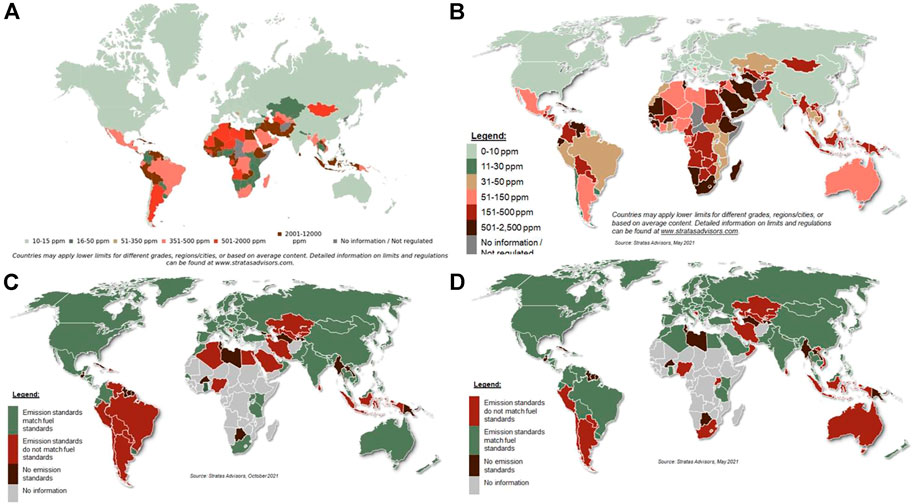
FIGURE 1. Maximum sulfur limits in (A) gasoline 2021 and (B) on-road diesel 2021, and gap between current vehicle emission standards and (C) gasoline quality and (D) on-road diesel quality (Global Fuel Specification, 2022).
Most of the discussed legislations such as the Clean Air Act of USA and the Euro 5/6 do not directly minitor the amounts of nitrogen-containing compounds in the curbarent fuel but rather control the emission levels of nitrogen oxides, particularly NO2. Nevertheless, it is important to have nitrogen levels below 10 ppm (Abro et al., 2016).
Overview of crude oil processing
The crude oil refinery process is primarily meant to separate the hydrocarbons according to their boiling points (viz. molecular weight) through fractional distillation into fractions suitable for specific applications such as fuels, lubricants, and feedstock for other downstream petrochemical industries. Product improvement is also important during refinery and encompasses processes applied to adjust the boiling range of the products to target more valuable cuts or to introduce special properties that improve the product performance and to ensure adherence to given specifications (Speight, 2018). Common refinery boiling point adjustment technologies include alkylation (increase in boiling point) as well as fluid catalytic cracking and hydrocracking (boiling point reduction). Naphtha reforming and isomerization is used primarily to improve gasoline octane numbers, while hydrotreatment is typically used to remove heteroatoms and improve product stability (Speight, 2018). The main categories of fuel oils from the distillation of crude oil are heavy gas oil, diesel/light gas oil, kerosene and they serve as different carburants in different forms of motorized transport.
Removal of sulfur- and nitrogen-containing compounds is complicated by the complexity of the fuel oil matrix. (Hansmeier et al., 2011) Reductive or oxidative techniques are primarily used in industry to remove sulfur and nitrogen-containing compounds (Forte et al., 2014). The techniques can be applied before the use of the fuel oil or on exhaust fumes (Zolotareva et al., 2019). However, removal of sulfur- and nitrogen-containing compounds from exhaust fumes is more applicable to industrial plants. Practically, the techniques cannot be applied to motorized transport as a primary method for removing the noxious gases that come from the sulfur and nitrogen compounds. Catalytic converters can only sufficiently manage small quantities of sulfur oxides that come from low sulfur fuel oils. That still leaves the removal of sulfur- and nitrogen-containing compounds from oil fuel before use as the most preferred technique. In that regard, hydroprocessing has proven to be the more practical route due to how easily it can be applied on an industrial scale and has been the conventional technique (Huh et al., 2009). However, the technique has limitations and challenges that keep on motivating researchers to find alternative techniques or techniques to aid hydrotreatment.
Conventional hydroprocessing
Hydroprocessing covers a range of catalytic processes such as hydrotreating, hydropyrrolysis and hydrocracking processes (Figure 2). This process is carried out prior to most processes (e.g. catalytic reforming) to alleviate the challenges associated with the heteroatoms found in fuel oil such as sulfur, oxygen, metals and nitrogen (Saleh, 2015). The removal of these unwanted heteroatoms is referred to as hydrodesulfurization (HDS) for removal of sulfur, hydrodenitrogenation (HDN) for removal of nitrogen, hydrodeoxygenation (HDO) for removal of oxygen, and hydrodemetalation (HDM) for removal of metals. The processes occur simultaneously (Hansmeier et al., 2011). During hydroprocessing, the sulfur and nitrogen in sulfur- and nitrogen-containing compounds react with hydrogen under high pressure (typically 15–90 bar) and high heat (typically 300–350°C) in the presence of catalysts to produce H2S and NH3, respectively, leaving behind the corresponding hydrocarbons (Huh et al., 2009; Hansmeier et al., 2011; Javadli and de Klerk, 2012; Zolotareva et al., 2019). Additional steps, such as the Claus process, are required to remove and convert H2S into elemental sulfur (Hansmeier et al., 2011; Saleh, 2015; Zolotareva et al., 2019).
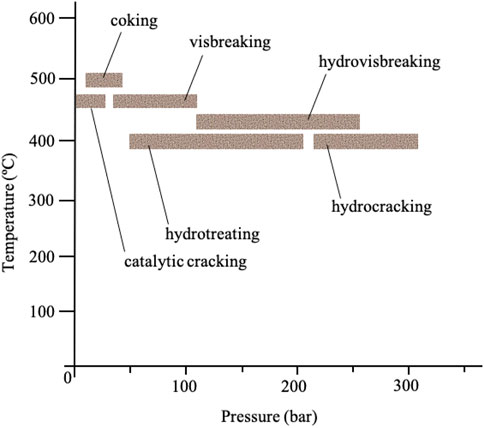
FIGURE 2. Hydroprocessing examples based on the process conditions (temperature and pressure) (Bose, 2015).
Hydrotreating catalysts consist of two or more metals, at least one being the active metal and the other one a promoter (Brunet et al., 2005). The most common hydrotreating catalysts contain the active metal as Molybdenum (Mo) or Tungsten (W), and Nickel (Ni) or Cobalt (Co) as the promoter metal. The most common hydrotreating catalysts are alumina supported Cobalt-Molybdenum (CoMo) and Nickel-Molybdenum (NiMo), being mainly used for HDS and HDN respectively. This is due to NiMo catalysts having higher hydrogenation activity than CoMo catalysts. The Nickel-Tungsten (NiW) catalyst has a higher denitrogenation activity than NiMo but is more expensive (Prado et al., 2017; Kaiser et al., 2020). The catalysts are commonly supplied in their air-stable oxide form and require sulfidation for activation (Figure 3). Sulfidation forms a metal-sulfide bond between the active metal sites in the catalysts and the sulfur compound used for sulfidation, usually carbon disulfide (CS2) or dimethyldisulfide. (Chowdari et al., 2020; Tanimu et al., 2021). The role of sulfidation is to form coordinatively unsaturated sites (CUS) or sulfur vacancies on the active metal where sulfur- and nitrogen-containing compounds are preferentially adsorbed by binding via the sulfur and nitrogen providing a driving force for their removal (Harris and Chianelli, 1986; Chianelli et al., 2020). In other words, the active forms of the hydroprocessing catalysts are actually transition metal sulfides.
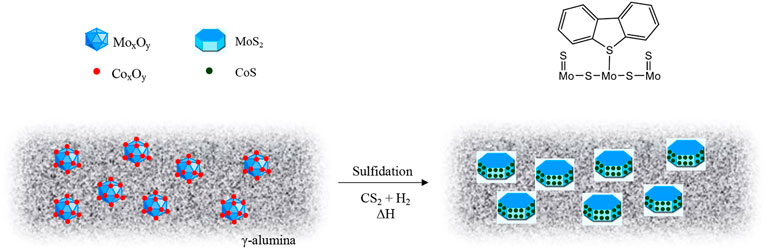
FIGURE 3. Schematic of the sulfidation process for CoMo catalyst showing DBT binding on a coordinatively unsaturated Mo site (Moqadam and Mahmoudi, 2013).
Conventional hydroprocessing challenges
Hydrotreatment does not completely remove sulfur- and nitrogen-containing compounds from fuel (Gray et al., 1995; Ma et al., 1996; Whitehurst et al., 1998). Reactivity is dependent on the molecular structure; paraffinic components, i.e. aliphatic acyclic sulfides (thioethers), cyclic sulfides (thiolanes), aliphatic amines and anilines can be easily removed while sulfurs and nitrogens in heterocycles are more resistant. The performance of hydroprocessing catalysts is negatively affected by steric and electronic factors of the heterocyclic compounds. Sulfur-containing compounds remaining in hydrotreated diesel fuels at sulfur levels lower than 500 ppm are predominantly dibenzothiophenes with alkyl substituents at the 4- and/or 6-position. Carbazole and its alkyl-substituted analogues are the most refractory nitrogen-containing compounds (van Looij et al., 1998; Wiwel et al., 2000; Zeuthen et al., 2001). The more the substituents in pyrrole benzologues the less their reactivity (Zeuthen et al., 2001). Carbazoles with methyl substituents at positions 1- and 8- were found to have extraordinarily low reactivity similar to 4,6-substituted dibenzothiophene. Interestingly, alkyl substitution at positions 4- and 5- also reduces reactivity significantly, inconsistent with the normally used steric restriction reasoning (Wiwel et al., 2000). Indoles and quinolines are very reactive compared to carbazoles (Zeuthen et al., 2001). For equivalent sulfur- and nitrogen-containing compounds (e.g. carbazole and dibenzothiophene), nitrogen-containing compounds have much lower reactivity (Zeuthen et al., 2001).
Small amounts of aromatic nitrogen-containing compounds in the fuel can easily inhibit the catalysts due to preferential adsorption (Zeuthen et al., 1991; Whitehurst et al., 2000; Koltai et al., 2002; Turaga et al., 2003; Wiwel et al., 2010; Hidalgo-Vivas et al., 2022). This has a serious negative effect on the kinetics of hydroprocessing, particularly removal of refractory sulfur-containing compounds. Basic nitrogen-containing compounds are considered the biggest problem in that regard as they severely inhibit hydrogenolysis and hydrogenation, steps that are crucial for hydroprocessing of refractory sulfur-containing compounds (Zeuthen et al., 1991; Whitehurst et al., 2000; Zeuthen et al., 2001; Koltai et al., 2002; Turaga et al., 2003; Wiwel et al., 2010; Prado et al., 2017; Hidalgo-Vivas et al., 2022). It has always been feared that even neutral nitrogen-containing compounds have a similar inhibitory effect since strongly basic intermediates (amines) are formed during hydrotreatment of neutral nitrogen-containing compounds (Forte et al., 2014; Prado et al., 2017; Wen et al., 2017). The final product of hydrodenitrogenation (NH3) is also strongly basic (Nakamura et al., 2005). An investigation by van Looij et al. (van Looij et al., 1998) shows that trace amounts of nitrogen compounds (0–30 ppm) have a significant inhibition effect to the reaction rate in deep hydrodesulfurization and are a key factor in achieving deep hydrodesulfurization of fuel oil while the influence of compositional changes e.g. content polycyclic aromatics is negligible. Sulfur-containing compounds and their hydrotreatment product H2S are also capable of the same, albeit to much a lesser extent (Nakamura et al., 2005; Kent, 2010). Neutralization depends on the equilibrium of the acid-base reaction which is determined by the strength of the acidic sites, the basicity of the compounds and temperature. Inhibitory effects are also higher at low temperatures (>350°C) (Prado et al., 2017). The more basic the compounds, the greater the inhibition.
Additionally, hydrodenitrogenation is an unfavourable process, it is a kinetically slow process, consumption of hydrogen is not stoichiometric (much higher quantities are required), and high amounts of ammonia affect the gas cleaning step and complicate other process aspects (Prado et al., 2017).
Strategies for achieving ultra-deep hydroprocessing
Achieving ultra-low sulfur levels using conventional hydroprocessing is still a work in progress and a cause for concern for refiners when it comes to striking a balance between producing such products at reasonable market prices. There is now an increasing need for drastic improvements to the process to meet the required low sulfur specifications as well as accommodate the increasing heavier poorer feeds. Severe hydrotreatment conditions (higher temperature, residence time, pressure and more hydrogen) have been applied to try and remove the persistent sulfur- and nitrogen-containing compounds and also minimize inhibition of catalysts (Hansmeier et al., 2011; Prado et al., 2017). For example, hydrotreating of creosote distillate to produce a distillate blending material for diesel fuel is industrially performed at 280–380°C and 18.5 MPa (Prado et al., 2017). However, the use of severe operating conditions means: consumption of hydrogen is much higher, hydrodearomatization increases, and there is an adiabatic temperature increase since hydrodenitrogenation and hydrodearomatization are very exothermic reactions (Hansmeier et al., 2011; Prado et al., 2017). Often additives need to be added to manage the fuel specifications. Excessive use of hydrogen is also of concern due to increasing demand for hydrogen over the years, especially in green fuel technologies (Lima et al., 2019). Besides, the current production of hydrogen already has a huge carbon footprint (Javadli and de Klerk, 2012).
Better bets have been on catalyst development, better reactor designs and introducing new processing systems. (Lima et al., 2019). With regards to new processing systems, placement of different catalysts in multibed configurations so that they can work synergistically in what is known as catalyst stacking technology has been showing promise in improving hydroprocessing activity and reducing hydrogen consumption and other operating costs (Leal et al., 2022). The technology also allows expensive catalysts to be easily incorporated into the systems. However, the technology is still at its infancy, how it works is not yet fully understood and there are many factors to consider. Each optimal configuration is on a case-to-case basis as there is no established predictive method. However, just slight increamental gains are achieved at this stage.
Several complementary methods that can aid hydroprocessing to achieve ultra-low sulfur levels are widely reported in literature. These are mainly meant to target the refractory sulfur compounds and they oxidative desulfurization, biodesulfurization, extractive desulfurization and adsorptive desulfurization are the most popular. However, in this work our perspectives are only centered around further improvements that can be made on hydroprocessing catalysts and improving feedstock through pre-treatment to remove nitrogen-containing compounds.
Improvements in conventional hydroprocessing catalysts
The development of novel hydroprocessing catalysts with excellent hydroprocessing performance is theoretically the most effective strategy as it can be easily adopted into existing fuel processing plants with minimal capital requirements (Zhang et al., 2019a). Hydroprocessing catalysts can be improved through new catalytic systems such as engineering new supports and/or new active phases (Huirache-Acuña et al., 2021). For example, replacement of transition metal sulfide active phases with noble metals has been successfully used. (Zhang et al., 2019a; Majodina et al., 2021). Ru and Pt as additives result in enhanced C-S cleavage activity as was demonstrated when they were added to a Rh-P catalyst and tested on 4,6-DMDBT (Kanda et al., 2022). Superior direct desulfurization selectivity has also been reported for Pt2Si/CNTs, RhxSi/CNTs and RuSi/CNTs catalysts, with Pt2Si/CNTs having the highest activity, excellent stability and sulfur resistance (Yang et al., 2021). Small amounts of Ru were also shown to cause a significant increase in activity and selectivity for the products of the hydrogenation desulfurization pathways (Topalian et al., 2021). Highly active Re and Pd phases in Re/Pd-TiO2/SiO2 aerogel and xerogel catalysts have also been reported to result in higher catalytic activity compared to conventional CoMo catalysts (Prokić-Vidojević et al., 2021). However, the high cost and stability of platinum group metals discourages their industrial applications.
Even with the several hydrotreating catalyst improvements, refractory sulfur containing compounds continue to pose several challenges that make it difficult to achieve ultra-deep hydroprocessing. It is therefore important to take catalyst improvement strategies with an understanding that improved catalytic activity does not necessarily translate to completely treating refractory compounds in an economical way. Therefore, there is need for foundational changes to how the improvement of catalysts and the removal of sulfur- and nitrogen-containing compounds are looked at. Considering the several years of development of hydrotreatment catalysts, it has become more and more difficult to find major breakthroughs in catalyst improvement. Continous changes to the catalysts also means continuous changes to the industrial process conditions and these changes can be expensive, e.g. pressure increases/new reactors and increased saturation result in high exotherms and requirement for heat management or more hydrogen (cost). As such, the playing field still remains open for other potential strategies and research in those areas has been gaining traction in the past few decades. Considering that catalyst performance is also impeded by nitrogen-containing compounds, particularly basic nitrogen-containing compounds, there is also a need to develop hydrotreatment catalysts that take into cognizance those aspects. It is also important to explore the option of removing nitrogen containing compounds before hydrotreatment is carried out.
In this section, we explore the potential improvements that can be made on hydroprocessing catalysts to improve their catalytic activity and ability to hydrotreat refractory compounds. Inferrences can be made from groups of catalysts incorporating some of the proposed catalyst activity and selectivity strategies (Table 1).
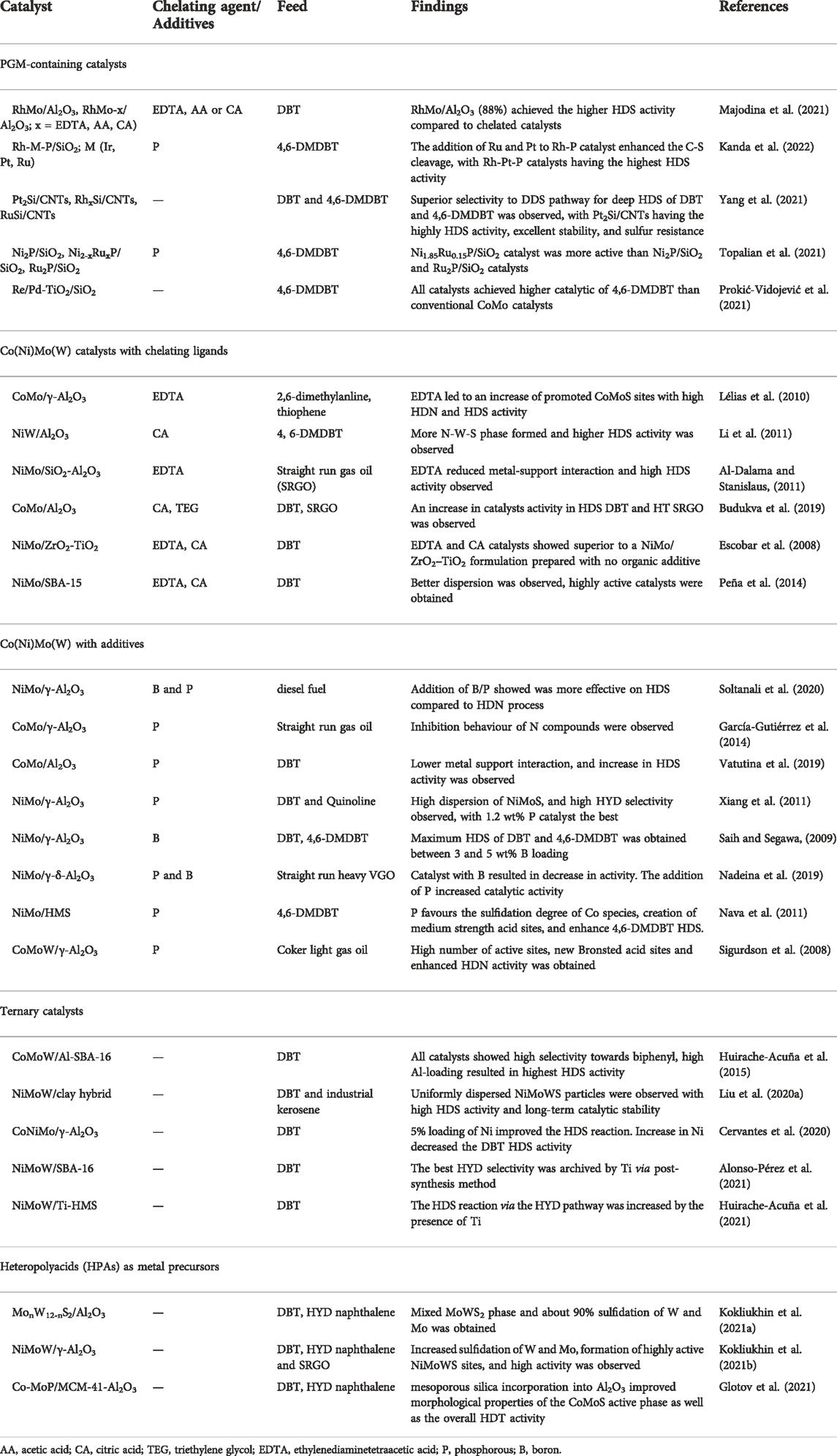
TABLE 1. Typical improvements that are being made to improve the performance of hydroprocessing catalysts.
Catalyst support considerations
The primary use of the support is for dispersing the active phase of the catalyst and to stabilize the catalysts. However, supports also influence the catalytic activity in various ways: by influencing the morphology of the active phase, modifying the electronic properties, and participating in bifunctional reactions with acid sites. A good support material has high surface area, optimum pore diameter (5–15 nm), optimum acidity, and moderate metal-support interaction (Shi et al., 2020). Different support materials have different effects on catalytic activity due to their distinct textural and structural properties, and the extent to which they interact with the active metal. Several studies have been conducted on a variety of supports such as TiO2, ZrO2, silica-alumina and mixed oxides (Duchet et al., 1991; Grzechowiak et al., 2001). It was discovered that TiO2 and ZrO2 show high activity (Caero et al., 2003). However, their low surface areas and small pore sizes make it difficult to disperse active phases and prevent diffusion of large bulky molecules onto the active sites leading to pore blockage and a decrease in catalytic activity (Michaud et al., 1998). The textural properties (morphology), affordability, and thermal and chemical stability, high tolerance for longevity, and good regeneration capacity of alumina supports (mainly ɣ-Al2O3) make them more advantageous and they are the most popular in hydrotreatment catalysts.
The chemical nature of the support material dictates the nature of the active phase. Strong metal-support interaction on the alumina support in Co(Ni)Mo-Al2O3 catalysts results in the formation of tetrahedral Mo oxides impeding full sulfidation. Due to this, research has focused on obtaining the optimum metal-support interaction, and this can be achieved by developing new supports for HDS and HDN application, or it can be engineered by introducing chelating ligands (Huirache-Acuna et al., 2013; Zhang et al., 2019b). The support needs not to be too acidic or too basic, since too much of either might lead to poor metal-support interactions resulting in poor Mo dispersion (Valencia and Klimova, 2011). However, it is important to note that high acidity leads to other undesirable side reactions such as coke poisoning and blockage of the active phase by basic nitrogen compounds (Alsolami, 2022). The support also has basic sites that contribute to the activity of the catalyst. Basic sites result in better dispersion of MoO4 and lead to low coke formation. Therefore, optimum metal-support interaction is desired to obtain proper sulfidation and dispersion of active species and to generate more Type II active phases. Type II active phases are the more active CoMoS phases formed from weak metal-support interactions. Weaker tetrahedral metal-support interactions allow for easier reduction and sulfidation process of molybdenum (tungsten) oxide unlike strong interactions that result in the formation of octahedral metal oxides which are difficult to reduce and sulfide resulting in the formation of the less active Type I phases.
The morphology of the alumina support is crucial in determining the physicochemical properties that influence the catalytic behavior of the catalyst. The acidity of the alumina support is one of the most important features that controls the dispersion of Co(Ni)Mo and also affects the metal-support interaction (Chen et al., 2013). Brönsted acid sites found in the support material impart electronic effects on the active sites making it easier for coordinatively unsaturated sites to be formed when sulfur is released as H2S. They also act as a source of H2 to push towards the hydrogenation pathway through the S-H group found on the S-edge sites located on the active site of the catalyst (Figure 4). (Wu et al., 2014; Cao et al., 2020) Brönsted acid sites help to overcome the steric hindrance of alkyl DBTs through isomerization thus enhancing HDS activity (Breysse et al., 2003; Breysse et al., 2008). They also enhance C-N cleavage during denitrogenation since the denitrogenation process is linked to the S-H groups around the active site. Therefore, increased hydrogenation activity also results in an overall increased in HDS and HDN activity.
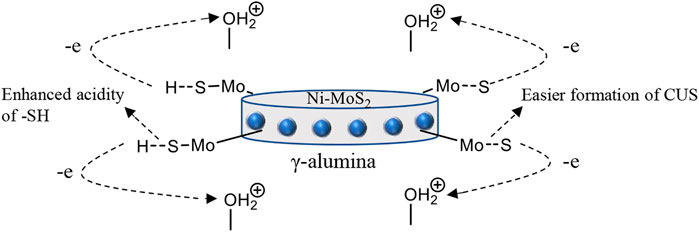
FIGURE 4. A representation of an alumina supported catalyst showing the movement of electrons on the active sites.
Bimetallic vs. ternary catalyst considerations
Conventional bimetallic catalysts are susceptible to thermal, chemical, or mechanical degradation and metal coke poisoning, which often leads to inefficient ways to treat feedstock (Cervantes et al., 2020). This has motivated the development of trimetallic catalysts such as NiMoW, CoNiW, CoNiMo to increase the versatility of catalysts such as working in various harmful conditions and increased activity due to the additional active phase (Cervantes et al., 2020). These trimetallic catalysts have the advantage of having mixed phases [Ni(Co)MoWS] and their systems can be easily tailored compared to bimetallic and monometallic catalysts (Tanimu and Alhooshani, 2019). However, the catalyst performance results from different literature sources are contradictory. Severino et al. (Severino et al., 2000) and Sadeghbeigi (Sadeghbeigi, 2020) concluded that CoNiMo catalysts are less active as compared to bimetallic catalytic systems when they tested the HDS of thiophene and of 4,6-DMDBT, respectively. Mozhaev et al. (Mozhaev et al., 2016) and Cervantes et al. (Cervantes et al., 2020) obtained higher HDS activity for DBT when using CoNiMo catalysts compared to bimetallic catalysts. Higher catalytic activity for the trimetallic catalysts was also obtained for the desulfurization of heavy gasoline (Badoga, 2015). Monodispersed γ-Al2O3 supported NiMoW nanocatalysts also showed higher activity compared to NiMo and NiW bimetallic nanocatalysts when tested by Singh et al. (Tanimu and Alhooshani, 2019) With such contradictory results, it is important to note that the rational interpretation of the positive or negative role devoted to the addition of a second promoter has not been proposed yet (Cervantes et al., 2020). Therefore, more research can be focused on this work to improve the performance of the catalyst (Singh et al., 2021).
Unsupported versions of trimettalic catalysts have also been explored in what is known as NEBULA technology (Mendoza-Nieto et al., 2013). NEBULA catalysts predominantly contain metal sulfides in forms that incorporate porosity and avoid the use of catalyst supports to circumvent limitations in catalyst activity that comes from the limit in the amount of active metal that can be deposited on the pore walls of the support material. The catalysts show more superior HDS and HDN activities (about three times higher) than conventional alumina supported catalysts (Stanislaus et al., 2010). This is attributed to the metallic character, i.e. the formation of new mixed NiMoWS active phase which has better properties compared to conventional bimetallic NiWS, NiMoS, and CoMoS active phases. They also have better porosity character which is a limiting issue in supported catalyst. They are not limited by base interactions from the support and they contain a greater proportion of the formation of more Type II active phases. They contain more active metal per given volume with greater concentration of highly active Type II phases. The main disadvantage with Nebula catalysts is their high cost due to high metal content (Mendoza-Nieto et al., 2013). A study by Liu et al. (Liu et al., 2020b) using unsupported NiMoW catalyst on DBT showed a significant improvement of HDS activity but it was difficult to maintain the overall layered structure of the catalyst at high temperatures.
The success of NEBULA catalysts inspired the Albemarle-ExxonMobil research team to discover a new range of catalysts which they named Celestia catalysts (Leliveld and Slettenhaar, 2022). Celestia catalysts have higher catalytic activity than NEBULA catalysts. They have improved HDS performance and increased diesel yield enabled by turning down the cracking activity while still meeting product sulfur targets. The distillate and gasoline product have improved qualities and overall volume swell due to its exceptional saturation ability because it provides a higher exotherm. Celestia catalysts also provide the option to process more challenging feeds in hydrocracking pre-treatment without sacrificing unit length through increased catalyst deactivation. However, celestia catalysts require longer hold time to sulfide the catalyst fully. Their efficieny is best realized in high pressure applications. They are also typically very expensive. They are heavier (denser) than normal catalysts which is sometimes prohibitive when the equipment design limits are exceeded.
Fine-tuning the active phases of the catalysts
The use of chelating ligands
The catalytic activity mostly depends on the formation of the active phase and its structure. Research has shown that during the activation of the catalyst the promoter sulfides first, e.g., Co or Ni (50–150°C) and then the Mo sulfides later from 175°C (Kishan et al., 2000; Coulier et al., 2001). The formation of the active phase requires the presence of a promoter (Co or Ni). Considering that the promoter sulfides first to form inactive phases (CoxSy or NixSy), it is important to create a way of preserving promoter ions for the formation of the active MoS2 phase which only happens after the sulfidation of Mo (Kibsgaard et al., 2010). When there are fewer promoter ions remaining after the sulfidation of Mo there will be less chances of the promoter ions locating the MoS2 resulting in fewer CoMoS active phases forming and ultimately the formation of a catalyst with poor activity. Addition of chelating ligands during catalyst synthesis decreases the interaction of the promoter and the support by forming a promoter ion-chelating ligand complex which later decomposes to react with MoS2 to form the CoMoS active phases (Sundaramurthy et al., 2005). Most promoter ion-chelating ligand complexes only start to decompose above 200°C preventing early sulfidation of the promoter ions. Chelating ligands that have been applied for improving the formation of active phases include citric acid (CA), ethylenediaminetetraacetic acid (EDTA), glycol, nitriloacetic acid (NTA) (Sun et al., 2003). The addition of ligands also leads to the formation of polymolybdates in the oxide phase which are easily reducible, and metal dispersion is favorable during sulfidation of both cobalt and molybdenum oxides as they will start sulfiding at similar temperatures, thus resulting in the formation of more active phases (Badoga et al., 2012). The nature of the chelating ligand also imposes changes on the catalyst such as thermal stability, modulate the metal-support interaction, improve the dispersion of metal sulfides, and acidity leading to the formation of more CUS in the catalyst (de León et al., 2010; Rashidi et al., 2013; Zhang et al., 2016). Some of the advances that have been made in the use of chelating ligands to improve catalyst activity were summarized in Table 1.
A comparative study was carried out on HDS with catalysts synthesized on SBA-15 support with and without EDTA as a chelating ligand (Badoga, 2015). The sulfided catalysts were CAT 0 (NiMo/SBA-15), CAT 1 (NiMo/SBA-15, EDTA/Ni molar ratio 1), and CAT 2 (NiMo/SBA-15, EDTA/Ni molar ratio 2). A NiMo/Al2O3 catalyst (with no EDTA) was used for benchmarking. It was observed that all chelated catalysts had greater HDS catalytic activity compared to the standard NiMo/Al2O3 catalyst. The sulfur conversion followed the order: CAT2 (82%) > CAT1 (75%) > CAT 0 (70%) > NiMo/γ-Al2O3 (68%). Pena et al. (Peña et al., 2014) conducted a study on CoMo-SBA-15 catalysts with different amounts of MoO3 and CoO3 (Table 2). Benchmarking with CoMo6 and CoMo12, the chelated catalysts showed higher activity in the hydrotreatment of DBT in the order EDTA chelated catalysts > CA chelated catalysts > non-chelated catalysts.
Majodina et al. (Majodina et al., 2021) recently tested a series of catalysts with and without chelating ligands tested for DBT HDS (RhMox/Al2O3, x = EDTA, acetic acid, citric acid) and observed the following: RhMo/Al2O3 (88%) > RhMo-AA/Al2O3 (73%) > RhMo-CA/Al2O3 (72%) > RhMo-EDTA/Al2O3 (68%). The observed trend is contrary to the previous studies carried out on base metal catalysts where the importance of delaying sulfidation of the promoter (Co or Ni) sulfide, using chelating liagnds, was demonstrated. This opens up more questions around where chelation can be advantageous or how it can be applied for the different metals.
The use of additives
The addition of additives such as boron and phosphsorus also greatly improves the catalytic activity of conventional HDS and HDN catalysts (Table 1) (Rashidi et al., 2013; Vatutina et al., 2019; Soltanali et al., 2020). The addition of boron or phosphorous helps improve the acidity of the catalyst and the dispersion of the active phase. The acidity of the catalyst helps sterrically hindered refractory compounds to access the active sites. When Soltanani et al. (Soltanali et al., 2020) used phosphorus as an additive they additionally found that phosphorous can act as a promoter atom, helping with the distribution of MoS2 as it reduces Mo(VI) to Mo(IV). They also realized that phosphorous encourages the formation of octahedral Ni and Co structures vs. tetrahedral structures that cause polymerization of Mo-S bonds. Phosphorous also improves the degree of sulfidation of Co or Ni particles by reducing the metal support interaction. Additionally, phosphorous improves the formation of Brönsted acid sites on the catalyst, hydrogenation of aromatic rings, stability of the impregnation solution resulting in better metal distribution on the support, and the thermal stability of alumina. It also prevents the formation of inactive NiAl2O4 particles during catalyst synthesis and coke formation during hydrotreatment (Poulet et al., 1993; Jian and Prins, 1998; Maity et al., 2008; Rayo et al., 2012; Rashidi et al., 2013; Soltanali et al., 2020). The same properites can also be obtained using boron; increasing dispersion of the active phase, modifying the acidity of the support and reducing metal-support interaction.
Changing synthesis strategies (using different precursor compounds)
Replacement of conventional active metal precursors with precursors having two or more metals in the form of heteropolyacids (HPAs) in the preparation of oxidic precursors of metal-sulfide catalysts enhances catalyst activity (Ramírez et al., 2012; Minaev et al., 2015). Starting with such multimetal precursor compounds results in all metals being in close proximity allowing them to interact better with each other. Keggin-type HPAs such as B-H4[SiMo3W9O40] have been used as precursors for alumina supported catalysts giving the advantage to introduce simultaneously both metals in the same compound allowing to maintain a Mo-W nanoscape proximity (Nikulshina et al., 2018; Nikulshina et al., 2020) obtained MoxW1-xS2 active phases using SiMonW12-n HPA precursors and observed higher catalytic activity for DBT (Nikulshina et al., 2018). Higher hydrodrogenation activity for naphthalene using the same catalyst was also observed by Nikulshina et al. (Nikulshina et al., 2020) Monometallic HPAs have also been investigated and it was found that the use of a mixture of H4SiMo12O40 and H4SiW12O40 leads to preferential formation of corresponding MoS2 and WS2 active phases, respectively (Kokliukhin et al., 2021a). Thomazeau et al. (Thomazeau et al., 2007) reported that the formation of mixed MoWS2 crystallites is possible only from a precursor which contains both closely related metals in the structure at once. The introduction of HPAs as precursors has also inspired a new method for preparing trimetallic catalysts (Kokliukhin et al., 2021a).
Metal borides, phosphides, carbides, and nitrides have also been investigated as metal precursors (Alexander and Hargreaves, 2010; Prins and Bussell, 2012; Carenco et al., 2013; Topalian et al., 2021). Transition metal phosphides (Ni2P, Co2P, MoP) have been found to produce highly active phases that lead to high HDS and HDN activity (Cecilia et al., 2009; Shamanaev et al., 2021). Ni2P gives the highest activity and performs better when it comes to hydroprocessing refractory compounds (Miles et al., 2020; Jiang et al., 2021). Metal phosphides optimize the acidity of the material support, positively affecting the dispersion of the active phase resulting in improved catalytic performance (Cecilia et al., 2009). Metal phosphides have high thermal and electrical conductivity, high thermal stability, and are resistant to the inhibition of sulfur- and nitrogen-containing compounds under standard hydrotreating conditions (Yun and Lee, 2014; Topalian et al., 2021). However, Jiang et al. (Jiang et al., 2021) investigated Ni2P catalysts and found contradictory results that showed challenges with dispersion, limited total HDS activity and instability. Transition metal carbides and nitrides such as molybdenum carbides and molybdenum nitrides have simple crystal structures that possess similar properties to those of transitions metals normally used for hydroprocessing catalysts, e.g. desirable electronic and magnetic properties, extreme hardness, and ionic charactericsts (Chen, 1996). However, they have additional advantages of being resistant to sintering at high temperatures, having strong interactions with nitrogen, sulfur and other heteroatoms in fuel and not causing C-C bond scission which leads to undesirable saturation of aromatics (Lin et al., 2021; Yue et al., 2021). They also have noble metal-like properties that make them effective hydrogenation catalysts (Yue et al., 2021). However, transition metal carbides and nitrides do not sustain long-term stability, they are easily deactivate due to their strong affinity with the adsorbates, especially at high temperatures (Lin et al., 2021).
The catalyst selection for ultra-deep desulfurization would be pre-mature at this stage as there is a lot of research work being undertaken. It is possible that a mix of catalysts, including those containing PMGs, may be a way to go in the application of the catalyst stacking technology.
Removal of nitrogen-containing compounds prior to hydrotreatment
It is clear from the discussion in Section 1.6 that nitrogen-containing compounds make it difficult for hydroprocessing to be efficiently carried out. In summary, the compounds act as temporary poisons and inhibit hydroprocessing catalysts, they are precursors to coke formation and their hydroprocessing is not economical. Overall, they are one of the biggest hurdles to achieving ultra-low sulfur levels using standard hydroprocessing conditions and catalysts. Intensifying hydroprocessing conditions to overcome some of the challenges is expensive, increases coke formation and affects the quality of the product.
García-Gutiérrez et al. (García-Gutiérrez et al., 2014) demonstrated how hydroprocessing significantly improves with the ability to easily obtain ultra-low sulfur diesel after nitrogen-containing compounds were removed from straight run gas oil. They investigated the kinetics of hydroprocessing feedstock of varying nitrogen and sulfur concentrations in straight run gas oil: (0.0020–0.0357 wt%) and (1.3985–1.2130 wt%), respectively, using a commercial CoMoP/γ-Al2O3 catalyst at 5.49 MPa, liquid hourly space velocity of 2.5 h−1, and a temperature range of 622–643 K. The rate of desulfurization varied proportionally with sulfur concentration and inversely with the nitrogen concentration. From their experimental work, they developed a model that showed the sulfur levels that can be achieved as nitrogen levels are varied. The model shows that it is possible to reach 0.0010 wt% sulfur levels if nitrogen compounds are removed prior to hydrodesulfurization. Sau et al. (Sau et al., 2005) also showed that hydroprocessing catalyst activity increases by at least 60% and hydrogen consumption is significantly reduced when they carried out a similar investigation of removing nitrogen-containing compounds from straight run gas oil and vacuum gas oil prior to hydroprocessing.
Zeuthen et al. (Zeuthen et al., 2001) demonstrated that even though carbazoles are not very reactive and are among the predominant nitrogen compounds, it is the basic nitrogen-containing compounds that play a major inhibitory effect during hydrotreatment of fuel oil. The inhibitory effect of basic nitrogen compounds is magnitudes higher than that of neutral nitrogen compounds while carbazoles are not very inhibiting. Similar studies using different sets of neutral and basic nitrogen compounds are reported throughout literature (Zeuthen et al., 1991; van Looij et al., 1998; Whitehurst et al., 2000; Koltai et al., 2002; Turaga et al., 2003; Wiwel et al., 2010; Hidalgo-Vivas et al., 2022). The behaviour of even trace amounts of basic nitrogen compounds strongly resembles the effect of high concentrations of nitrogen compounds (van Looij et al., 1998; Whitehurst et al., 2000; Zeuthen et al., 2001). The more basic the nitrogen compounds the more the inhibitory effect (Wiwel et al., 2010; Hidalgo-Vivas et al., 2022). Zeuthen and Blom (Zeuthen et al., 1991) characterized the nitrogen on aged hydroprocessing catalysts using temperature-programmed oxidation. They concluded that the nitrogen compounds were attached to the catalyst surface and were not necessarily in the bulk of the coke that was on the surface of the catalyst providing further evidence of the inhibitory effect of the nitrogen compounds on the catalysts.
With that in mind, removing nitrogen-containing compounds (especially the basic ones) as a primary step should improve the efficacy of hydroprocessing and all the other upstream and downstream processes that are affected by these compounds. It is possible that pre-treating to remove nitrogen compounds may be more economical compared to applying more intense hydroprocessing conditions (higher hydrogen pressure, temperature and residence time), improved infrastructure and process design, reduced catalyst lifespan requiring constant replenishment, and obtaining quality product which is not compromized by harsh processing conditions. More importantly, high nitrogen and sulfur content feedstocks such as creosote that are usually shunned by industry can also be pre-treated to remove nitrogen-containing compounds and be economically hydroprocessed to achieve ultra-deep desulfurization. Key to the success of this approach is achieving high selectivity for the nitrogen-containing compounds since the fuel matrix is complex. The fuel matrix is composed of other components (e.g. moisture, aromatics, metals and oxygenates) that affect the performance of methods that target nitrogen-containing compounds (Khan et al., 2013; Ahmed and Jhung, 2016a). Some of the compounds in fuel that are important for the quality of the fuel (e.g. octane and cetane numbers) also have physichochemical properties close to those of the target nitrogen-containing compounds, e.g. aromatics and other heteroatomic compounds (Ghosh et al., 2006). Most approaches can be considered as either 1) modification of the nitrogen-containing compounds so that extractive or adsorptive techniques can be easily applied or 2) improvements of the solvents or materials used for extractive or adsorptive separation. Oxidative, extractive, and adsorptive techniques have also been widely researched for desulfurization and the process may also contribute to simultaneous removal of the harsher refractory sulfur compounds. Extraction or adsorption using affordable solvents or materials are more practical approaches and the purified compounds obtained as by-products can also become feedstock for other industries, e.g. quinoline and carbazole which are obtained exclusively from coal tar (Fetzner, 1998). We consider it much more beneficial to fine-tune extractive and adsorptive techniques for the selective removal of nitrogen-containing compounds as a complementary technique to hydrodesulfurization.
Denitrogenation by chemical modification followed by separation
Nitrogen-containing compounds can be derivatized via the nitrogen using addition or substitution reagents to precipitate them out of fuel or to make them more polar so that they can be preferentially separated from the rest of the fuel oil using extractive or adsorptive techniques due to their increased relative polarity. Alkylation and oxidation have been extensively investigated in that regard. Alkylating agents usually lead to precipitation. Shiraishi et al. (Shiraishi et al., 2001a; Shiraishi et al., 2001b; Shiraishi et al., 2001c) investigated the denitrogenation of aniline, indole and carbazole from xylene (representing light oil feedstocks) using CH3I and AgBF4 for alkylation. N-methylation and subsequent precipitation of the compounds occurred under moderate conditions. The nitrogen content was reduced to less than 20% of the feed concentrations and application on light oil feedstocks was also demonstrated. Bromoacetic acid has been extensively tested for alkylation of nitrogen compounds (Prado et al., 2017). Metallic salts (e.g. CuCl2, SnCl2 and FeCl3) have also been used to precipitate basic nitrogen compounds through Lewis acid-base complexation (Prado et al., 2017). N-alkylation and precipitation is lucrative as it also simultaneously removes sulfur-containing compounds. However, the method struggles with refractory compounds such as carbazole as electron density on the nitrogen decreases with increasing carbon number of the alkyl substituents. Alkylation of other aromatics is also possible and it is especially difficult to selectively apply the method on high oxygenate content oils.
Catalysts based on strong Lewis acid transition metals (titanium, tungsten, platinum group metals, polyoxometalates, molybdenum and vanadium) have proven to be efficient for the oxidation of nitrogen-containing compounds (Dembaremba et al., 2019a). Various support materials have also been used for the catalysts, e.g. silica, alumina, zeolites and organic polymers, and these also have an influence in the performance of the catalysts (Dembaremba et al., 2019a; Dembaremba et al., 2019b). Selective oxidants such as nitric acid and/or nitrogen oxides, organic hydroperoxides, peroxyacids, and hydrogen peroxide are usually employed as oxidants (Dembaremba et al., 2019a; Dembaremba et al., 2019b). Some of the by-products generated by the organic oxidants influence the quality of the fuels; for example, tert-butyl alcohol is obtained from tert-butylhydroperoxide and improves the octane number (Karas et al., 2008). Hydrogen peroxide is much cheaper but is difficult to use since it is aqueous. Research has been carried out around the introduction of surfactants which help to disperse oxidant in the form of small spherical droplets in fuel oil, a process described as an emulsion catalysis system (Jiang et al., 2011).
Other methods such as autooxidation (using atmospheric oxygen), photochemical oxidation and ultrasound oxidation have also been attempted to cut costs (Shiraishi et al., 2000; Whitehurst et al., 2000). Photochemical oxidation is based on free radical formation and although promising results have been obtained in model samples it is difficult to predict the various reactions that can occur in a complex medium such as fuel oil (Forte et al., 2014). Photodecomposition of nitrogen-containing compounds has been reported by Shiraishi et al. (Shiraishi et al., 2000) in model fuel oils with hydrogen peroxide being required in an oil/water biphase to enhance photoreactivity of the nitrogen-containing compounds.
The advantage of the oxidative process is that, unlike hydrotreatment, it operates at lower reaction temperatures and pressure, and in the absence of hydrogen which is expensive. However, large amounts of expensive oxidants are required to completely oxidize the compounds. Our previous work was on improving the catalysts for oxidation in order to reduce the amount of oxidant required but even with good catalysts the amounts of oxidant required still remain high. (Dembaremba et al., 2019a). Using this already expensive process prior to hydrotreatment will result in an expensive product. The capital investment for the additional units is also significant. Product selectivity is also a major challenge (Ishihara et al., 2005). A study by Ogunlaja et al. (Ogunlaja et al., 2017) shows the complexity around the oxidation of nitrogen-containing compounds, attributing the array of products to reactions that occur following the formation of hydroxy radicals. Formation of this vast array of products further complicates removal of products after oxidation.
Extractive denitrogenation
Choice of solvent for the extraction of nitrogen-containing compounds from fuel oils is critical, it must be thermodynamically compatible with the compounds (Dembaremba et al., 2020). Polar solvents with low boiling points such as ethanol, ethanol and acetonitrile have been tested for denitrogenation of fuel oil but these solvents are too volatile for practical application on an industrial scale (Campos-Martin et al., 2010; Mokhtar et al., 2014). Polyalkyleneglycol, polyalkylene glycol ether, imidazolidinones, pyrrolidones, pyrimidinones, dimethyl sulfoxide and dimethyl formamide which have much higher boiling points have shown higher extraction levels of nitrogen-containing compounds (Mokhtar et al., 2014; Abro et al., 2016). However, the boiling points of these solvents are in the same range as some nitrogen-containing compounds, and thus may not be easily separated from the extracted compounds, discouraging solvent recovery by distillation. Besides boiling points, poor selectivity still stands as the major deterrent in the application of solvent extraction.
Considering the poor selectivity witnessed with the use of organic solvents, researchers redirected their efforts towards the use of ionic liquids (ILs) to try and selectively remove nitrogen-containing compounds from fuel. Ionic liquids are described as liquids composed entirely of ions at or below 100°C - more like an ambient temperature molten salt (Abro et al., 2016; Ibrahim et al., 2017). The cations are normally organic and are a basis upon which much of their physicochemical properties are derived, and the anions can be organic or inorganic. Extraction of nitrogen-containing compounds using ILs is based on their solubility that is determined by the chemical interactions involving the acid proton and steric factors in the IL. The use of ionic liquids gained momentum over the past decade with researchers attempting to use various combinations of cations and anions, dialkylimidazolium salts being the most common due to their desirable physical properties and ease of synthesis (Abro et al., 2016).
The chemical structures of ILs are now being tailor made to target certain physicochemical properties to obtain what are termed task specific or designer solvents which allow for better selectivity. In some cases, ILs have been designed to act as both oxidation catalysts and extractants. The ILs catalyse the oxidation of the sulfur- and nitrogen-containing compounds, improving their solubility in the ILs which also act as the extractant (Bhutto et al., 2016). The performance of the ILs in the presence of oxidant is remarkably higher, although efforts are needed to reduce the amounts of oxidant (Abro et al., 2016). Normally polyoxometalates such as vanadium and tungsten are used as the catalytic cations and H2O2 as oxidant (Lu et al., 2007). Researchers have also explored the use of supported ionic liquid phase (SILP) systems where the ionic liquid is dispersed on a support such as silica to increase surface area, reduce mass transfer limitations and for ease of use (Kuhlmann et al., 2009; Kohler et al., 2010). Leaching is still a challenge, an area that still requires more attention. The development of these SILPs demonstrate the importance of ease of separation in the fuel refinery process which leads use to adsorptive separation (Forte et al., 2014).
Overall, the biggest limit for solvent extraction is poor selectivity. The use of ILs is also mired by the presence of potent complex-forming agents in the fuel matrix. The presence of water, acids, halide ion and other impurities is known to significantly affect their structure and ultimately their physicochemical properties e.g., ILs based on AlCl3 and AlCl4 form precipitates when exposed to moisture (Dembaremba et al., 2020). The fuel matrix is a complex mixture that contains such components that may incapacitate the ILs over a number of cycles.
Adsorptive denitrogenation
The use of adsorbents to selectively adsorb nitrogen-containing compounds from fuel oil is the most lucrative option and a lot of research has been revolving around this technique. This is because of the advantages offered by adsorbents that make upscaling feasible and affordable: ease of separation after use, lower risk of noxious sub-products and easy to set-up and operate with the possibility of having a continuous flow system (Putra et al., 2009). However, the challenge has been on finding suitable adsorbents with high capacities and selectivity, and these are dependent on the mechanisms of adsorption.
Adsorption in primitive materials is mainly based on trapping molecules in their cavities based on their sizes (critical diameters and volumes) while in more advanced materials interactive forces that are important for improved selectivity are involved, e.g. acid-base interactions, coordination bond formation, π-complexation, hydrogen-bonding and van der Waals forces (Khan et al., 2013; Hasan and Jhung, 2015; Ahmed and Jhung, 2016a). Van der Waals forces are the most popular with hydrogen bonding being more relevant to adsorption of nitrogen-containing compounds. However, van der Waals forces are very weak in nature. Adsorption via π-complexation requires the donation of electrons from the π-orbitals of the adsorbates to vacant s-orbitals of the metal and back donation of d-elections of the metal to the empty π antibonding orbitals of the adsorbates. Transition metals in the d-block such as Cu+ and Ag+ and Ni2+ are good candidates for π-complexation, Cu+ being more prominent since it is cheaper and more readily available. Basic nitrogen-containing compounds can also undergo a special type of acid-base interaction that forms coordination bonds. The adsorbent serves as the Lewis acid while the nitrogen-containing compounds are the Lewis bases. Based on the Pearson’s hard and soft acid-base theory, basic nitrogen-containing compounds are in the hard to intermediate category and prefer hard Lewis acid sites (e.g. Fe3+, Cr3+, and Al3+) (Pearson, 1963). Suitable materials are those with coordinatively unsaturated sites (Kim et al., 2011; Maes et al., 2011; Van de Voorde et al., 2013a).
The drive is now on refining the materials through designing and testing to achieve high selectivity for nitrogen-containing compounds. Research contributions on this subject have been increasing and materials summarized in Table 3 are meant to provide a guide in that regard.
Adsorptive denitrogenation using traditional adsorbents
Several primitive general adsorbents have been tested as potential adsorbents for removing nitrogen-containing compounds from fuel and these include clays (Mambrini et al., 2013; Baia et al., 2017), silicas and aluminosilicates (Kim et al., 2006; Kwon et al., 2008; Zhang et al., 2010; Rashidi et al., 2015; Laredo et al., 2017; Suganuma et al., 2020), zeolites (Hernández-Maldonado and Yang, 2004; Tian et al., 2020; Ofoghi et al., 2021), activated carbon (Sano et al., 2004; Kim et al., 2006; Ahmed and Jhung, 2015a; Anisuzzamana et al., 2018; Suganuma et al., 2020) and ion exchange resins (Marcelin et al., 1986; XiE et al., 2010) (Table 3). Composite materials that include these materials have also been widely tested (Song et al., 2017; Wang et al., 2019). The biggest advantage is that most of these materials are cheap and readily available. Althought most model fuel studies have yielded wonderful results in terms of loading capacities and recoverability of the adsorbents selectivity remains a challenge when they are applied on real fuel samples. Nevertheless, testing of these materials has provided a lot of information that has inspired the development of various tailor made adsorbents for the removal of nitrogen compounds.
Adsorptive denitrogenation using tailored organic polymers
Organic polymers can be effective adsorbents through hydrogen bonding, π-π interactions, van der Waals forces and electrostatic interactions. They can be tactically synthesized to achieve high selectivity for nitrogen-containing compounds through these adsorption mechanisms by creating selective binding sites that recognize and have a higher affinity for template molecules of interest. One approach is to polymerize monomers in the presence of template molecules of interest which are then removed after polymerization through leaching to obtain molecularly imprinted polymers (MIPs) (Awokoya et al., 2021). Several molecularly imprinted polymer materials in different forms (beads, nanofibers etc.) have been synthesized and tested for the removal of nitrogen-containing compounds. Typical MIPs and their performances have been summarized in Table 3. (Cao et al., 2014; Niu et al., 2014; Abdul-Quadir et al., 2018a; Abdul-Quadir et al., 2018b; Abdul-quadir et al., 2019; Mathidala and Ogunlaja, 2019; Awokoya et al., 2021). These include Fe3O4 nanoparticles equipped MIPs that are magnetic for ease of separation (Niu et al., 2014). A method for removing nitrogen compounds from gasoline or diesel fuel using molecularly imprinted polymers has been patented (Rinne and Risto, 2022). Literature search revealed limited study on the use of molecularly imprinted polymer for the selective adsorption of organonitrogen compounds in real fuel samples.
Adsorptive denitrogenation using coordination polymers
Research around the refinement of materials to achieve high selectivity has been tending towards coordination polymers (CPs), particularly metal organic frameworks (MOFs) due to the several provisions they offer. CPs are classes of crystalline repeating coordination entities between transition-metal clusters or ions and multidentate organic linkers extending in 1, 2 or 3 dimensions (Kitagawa et al., 2004; Batten et al., 2013). Three-dimensional CPs (MOFs) are the most popular for adsorption work as they contain uniformly sized pores, high total pore volume and very high surface areas (Kitagawa et al., 2004; Jiang, 2015). Due to the ability to tap into both the inorganic and organic aspects, MOFs are ideal candidates for tailoring good selectivity. The design strategies used to obtain particular pore sizes and modify pore properties are also simple, and these include control of their composition, structure, scale and the bulk properties (Schneemann et al., 2014; Kenta, 2017). There is a also a vast range of transition metal clusters/ions and organic building blocks to choose from.
Due to the preferential adsorption of nitrogen-containing compounds, especially on coordinatively unsaturated sites, adsorptive denitrogenation using MOFs is prominent (Ahmed and Jhung, 2016a). Nuzhdin et al. (Nuzhdin et al., 2010) pioneered the use of MOFs in adsorptive denitrogenation by testing the performance of the common MIL-101 (Cr3+) MOF using indole, carbazole and their alkyl substituted derivatives. They realized an uptake of 9.0 mg nitrogen per gram of MIL-101 from straight run gas oil and 19.6 mg nitrogen per gram of MIL-101 from light cycle oil. They attributed the high sorption capacity to coordination of the nitrogen-containing compounds (via nitrogen) to the unsaturated Cr3+ centres. Selectivity for nitrogen-containing compounds decreased in the order indole > indole derivatives > carbazole > carbazole derivatives which they explained in terms of indole and derivatives having better steric accessibility of the nitrogen atom to the Cr3+ centre while the alkyl substituents cause steric hindrance. Simultaneous adsorption of other aromatic molecules was observed due to π-π stacking interactions with terephthalate bridges of metal-organic framework.
Maes et al. (Maes et al., 2011) screened a wide variety of MOFs with and without open metal sites for the selective adsorption of indole (IND), 2-methylindole (2MI), 1,2-dimethylindole (1,2DMI), carbazole (CBZ), and N-methyl carbazole (NMC) over TP, BT, and DBT (Table 3). They concluded that the availability and nature of open sites were crucial in the adsorption of nitrogen-containing compounds in the presence of other aromatic compounds. However, uptake was greatly reduced when the solvent system was switched from heptane/toluene (H/T, ratio 80:20 v/v) to toluene/heptane (T/H, 80:20 v/v). The reduction was attributed to the contribution of the aromatic rings in the MOFs and co-adsorption of competing toluene molecules.
Van de Voorde et al. (Van de Voorde et al., 2013a) found an Fe terephthlate MOF MIL100(Fe) to be efficient for the selective removal of N-heterocyclic compounds (Table 3). They extended their studies to isostructural terephthalate MOFs by varying the metal centre, MIL-100 (Al3+, Cr3+, Fe3+, V3+) and investigated the role of the metal centre in the adsorptive removal of nitrogen-containing compounds from fuel. Their calorimetric observations were coherent with the trend in the initial slopes of the isotherms, decreasing in the order: V > Cr > Fe > Al. They confirmed the formation of coordinated species for indole and dimethyl indole. The results for the polarizing power of the Lewis acid centres were inconclusive, similar with most other studies in literature, but it was clear that Fe3+ possesses the weakest Lewis acidity in perfect agreement with the observed lack of stable indole-Fe3+ coordination complexes in MIL-100(Fe). Al3+ in MIL-100(Al) had the lowest affinity for indole. Investigations on the adsorption of pyridine on MIL-101(Cr3+) by Kim et al. (Kim et al., 2011) also confirmed the formation of a coordination bond between the pyridine N and the coordinatively unsaturated sites in Cr3+. Wang et al. (Wang et al., 2013) showed that the stronger the basicity of the nitrogen-containing compounds the better the adsorption on MIL-101 (Cr3+). They also clarified the importance of pore shape and size in adsorption using MIL-53(Al) and MIL-96(Al). MIL-101(Cr3+) which is the best performing MOF in the MIL series was also shown to outperform silica gel, Selexsorb® CD, Selexsorb® CDX and activated carbon by far in the adsorption of nitrogen-containing compounds from straight run gas and light cycle oil with exceptional adsorption capacity and the fastest kinetics (Laredo et al., 2016). Adsorption studies on IL-53(Fe) which contains no coordinatively unsaturated metal sites showed that the material relies on adsorbates being good hydrogen bond acceptors (Van de Voorde et al., 2013b).
Following these revelations on what matters regarding adsorption of nitrogen-containing compounds using MOFs researchers went on a drive to improve the adsorption performance of other MOFs through modifications which include incorporation of functional groups (e.g. –SO3H, amino group) (Hasan et al., 2014; Ahmed et al., 2015; Ahmed and Jhung, 2015b) and Lewis acid sites (e.g. CuCl, phosphotungstic acid and AlCl3) (Ahmed et al., 2013a; Ahmed et al., 2014; Ahmed and Jhung, 2014) (Table 3). Composites between MOFs, and MOFs with other materials such as graphene oxide have also been tested (Table 3) (Ahmed et al., 2013b; Ahmed and Jhung, 2016b; Bhadra and Jhung, 2020). Lewis acid sites are also used to add functional moieties inside the pores of the MOFs (Van de Voorde et al., 2013b). She et al. (She et al., 2018) reported a highly selective metal organic framework with immobilized Ag(I) sites, (Cr)-MIL-101-SO3Ag, which nitrogen-containing compounds to interact with adsorption sites through multiple ways simultaneously (Figure 5).
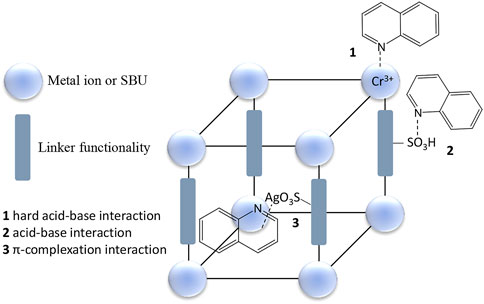
FIGURE 5. Schematic representation of the multiple interaction sites in the highly selective MOF, (Cr)-MIL-101-SO3Ag, reported by She et al. (She et al., 2018).
The approach of using MOFs is not impeccable. Firstly, MOFs are generally expensive due to the types of ligands or synthesis protocols used to obtain them. It can be capital intensive in industries such as the fuel industry where bulk quantities are required. In that regard, development of MOFs is being carried out with ease of separation and reusability in mind. Magnetism has been applied in some MOFs for ease of separation from the fuel matrix (Jin et al., 2014). Carrying out adsorption under continuous flow conditions can also be helpful. Secondly, synthesizing the desired MOF in a short period of time when scaling up remains a challenge. Sometimes the bulk powder form does not always match the structure of the target product. Bulk quantities are also usually obtained in a fine powder form with poor mechanical strength which makes re-use difficult. Aggregation during use or recycling may compromise the performance of the MOFs. Thirdly, some MOFs are sensitive to the environment, e.g., temperature and moisture. Lastly, adsorption in MOFs is based on small pore apertures which result in high diffusion resistance due to steric and dynamic hindrance limiting the movement of sulfur- and nitrogen-containing compounds into the inner pores. Nevertheless, if adsorptive denitrogenation is to be achieved it will most likely be through coordination polymer based-materials due to the several advantages they offer.
Perspectives and advances
Hydrotreament remains the main process used for removing sulfur- and nitrogen-containing compounds from crude oil. It is easier for industry to adopt improvements around this process compared to introducing new processes. The limits of the technique (remaining economically viable and the ability to process harsh feedstocks) are now being challenged by the recently imposed legislations that require ultra-low sulfur levels (<10 ppm) in fuel oil products. This has prompted major reconsiderations into process optimizations, catalyst designs, use of additives, blending with cleaner streams, and possible complimentary or alternative desulfurization methods. To date, none of the proposed alternative desulfurization methods such as biodesulfurization, extractive desulfurization, oxidative desulfurization and adsorptive desulfurization has resulted in a commercially viable process. The main inhibiting factor is the additional processing units required to incorporate the alternative technologies, such as the oxidation unit and extraction unit which require a high capital investment. It may be cheaper to add an adsorptive denitrogenation pre-treatment step since pre-treatment steps already exist in industry for example towards demulsifying, desalting, deasphalting and demetallization.
Improvements around the hydroprocessing technique has reached its apex, especially process optimization, and major changes will be difficult to achieve unless fundamental changes are made to the catalysts. There are still gaps that can be addressed in the design and use of catalysts, an area that was extensively covered in this review. Catalyst improvements range from perfecting the support materials, fine-tuning the active phases of standard catalysts by using chelating agents and additives, design of PMs-containing catalysts and trimetallic systems, and other synthesis strategies. From the works cited, catalyst activity improves with these changes. However, the efficiency of the improved catalysts on refractory compounds still remains a challenge. Even with those improvements, the catalysts are still not immune to the inhibitory effects that mostly come of nitrogen containing compounds. The inhibitory effects of nitrogen-containing compounds also have a serious impact on achieving ultra-deep hydrodesulfurization. As a result, there is huge motivation for pre-treatment of feedstocks to remove nitrogen-containing compounds prior to hydroprocessing in order to achieve deep hydrotreatment.
Despite the lack of industrial application of adsorbents currently for removal of nitrogen-containing compounds from crude oil, research in this direction is promising. Several strides have been made in the refinement of liquid-liquid partitioning techniques, with ionic liquids addressing most of the challenges that limit the selectivity of extraction solvents. The use of traditional adsorbents such as zeolites and carbon materials suffer lack of specificity for the nitrogen-containing compounds but nanoarchitectured coordination polymers (CPs) have recently been overcoming most of the challenges. It was identified that lack of synergy among the key features during adsorption, especially the metal centre and linker properties, is the biggest shortfall in most of the CPs from achieving good selectivity. It is assumed that an entity is either adsorbed based on its interaction with the linker or through interaction with the metal centre, but not effectively with both at the same time. That could also explain the lack of coherence in the results reported by authors so far with change in linkers, target molecules and when toluene was added as no resolute trend can be interpreted from the data. It was also noted that when looked at independently, coordination via coordinatively unsaturated sites provides a better chance to achieve good selectivity compared to any other mechanism. As considerations for the key features to work together are being made, it is important to have coordinatively unsaturated sites as the primary focus and exclude the other adsorption mechanisms as much as possible if good selectivity is to be achieved. This is particularly more important for crude oil since it is a very complex mixture with several competing molecules. Recent work from She et al. (Ahmed et al., 2015) has clearly demonstrated how focusing on design strategies can lead to highly remarkable selectivity for nitrogen-containing compounds. The governing factors should also be affordability and reusability of the coordination polymers.
Pre-treatment of crude oil to remove nitrogen-containing compounds undoubtedly helps to achieve ultra-deep hydrodesulfurization using conventional hydroprocessing. Coupled with the successes being made with coordination polymers, as well as as other adsorbents and solvents for liquid-liquid partitioning, it warrants research to put more effort in this direction. Removal of nitrogen-containing compounds from feedstock can also result in significant improvements of other downstream processes. High nitrogen content feedstock such as creosote are becoming more readily available syncrude oil sources and this approach will make it possible to process them sustainably. Besides, hydrodenitrogenation in itself is not an economical process hence pre-treatment of feedstock to remove nitrogen-containing compounds should improve the efficiency of hydroprocessing and also reduce operational costs. With concerted efforts on adsorbent and catayst development/catalyst stacking technology, it should be possible to study the application of these techniques in tandem for ultra-deep desulfurization. It will be important to consider the economical aspects for the implementation of this technique, of which there is no literature that clearly provides answers in that regard. It is crucial to provide more literature on the total cost and benefits of adding the pre-treatment step compared to intensifying hydroprocessing conditions and continuously replacing catalyst.
Author contributions
TD and SM: writing—original draft preparation; RW, AO, and ZT: Review and editing; ZT—Conceptualization.
Funding
This work is funded through the Sasol-NRF University Collaborative Research Grants (UID 138605).
Conflict of interest
Ryan Walmsley is employed by Sasol Technology (Pty) Ltd. All authors declare no other competing interests.
The remaining authors declare that the research was conducted in the absence of any commercial or financial relationships that could be construed as a potential conflict of interest.
Publisher’s note
All claims expressed in this article are solely those of the authors and do not necessarily represent those of their affiliated organizations, or those of the publisher, the editors and the reviewers. Any product that may be evaluated in this article, or claim that may be made by its manufacturer, is not guaranteed or endorsed by the publisher.
References
Abdul-Quadir, M. S., Ferg, E. E., Tshentu, Z. R., and Ogunlaja, A. S. (2018). Remarkable adsorptive removal of nitrogen-containing compounds from hydrotreated fuel by molecularly imprinted poly-2-(1H -imidazol-2-yl)-4-phenol nanofibers. RSC Adv. 8 (15), 8039–8050. doi:10.1039/c8ra00543e
Abdul-Quadir, M. S., van Der Westhuizen, R., Welthagen, W., Ferg, E. E., Tshentu, Z. R., Ogunlaja, A. S., et al. (2018). Adsorptive denitrogenation of fuel over molecularly imprinted poly-2-(1H-imidazol-2-yl)-4-phenol microspheres. New J. Chem. 42 (15), 13135–13146. doi:10.1039/c8nj02818d
Abdul-quadir, M. S., van der Westhuizen, R., Welthagen, W., Ferg, E. E., Tshentu, Z. R., Ogunlaja, A. S., et al. (2019). Selective removal of organonitrogen compounds in fuel using functional polybenzimidazole nanofibres: A combined experimental and theoretical study. S. Afr. J. Chem. 72, 88–100. doi:10.17159/0379-4350/2019/v72a12
Abro, R., Abro, M., Gao, S., Bhutto, A. W., Ali, Z. M., Shah, A., et al. (2016). Extractive denitrogenation of fuel oils using ionic liquids: A review. RSC Adv. 6, 93932–93946. doi:10.1039/c6ra09370a
Ahmed, I., and Jhung, S. H. (2014). Adsorptive denitrogenation of model fuel with CuCl-loaded metal–organic frameworks (MOFs). Chem. Eng. J. 251, 35–42. doi:10.1016/j.cej.2014.04.044
Ahmed, I., and Jhung, S. H. (2016). Adsorptive desulfurization and denitrogenation using metal-organic frameworks. J. Hazard. Mat. 301, 259–276. doi:10.1016/j.jhazmat.2015.08.045
Ahmed, I., and Jhung, S. H. (2015). Effective adsorptive removal of indole from model fuel using a metal-organic framework functionalized with amino groups. J. Hazard. Mat. 283, 544–550. doi:10.1016/j.jhazmat.2014.10.002
Ahmed, I., and Jhung, S. H. (2016). Remarkable adsorptive removal of nitrogen-containing compounds from a model fuel by a graphene oxide/MIL-101 composite through a combined effect of improved porosity and hydrogen bonding. J. Hazard. Mat. 314, 318–325. doi:10.1016/j.jhazmat.2016.04.041
Ahmed, I., and Jhung, S. H. (2015). Remarkable improvement in adsorptive denitrogenation of model fossil fuels with CuCl/activated carbon, prepared under ambient condition. Chem. Eng. J. 279, 327–334. doi:10.1016/j.cej.2015.05.035
Ahmed, I., Jun, J. W., Jung, B. K., and Jhung, S. H. (2014). Adsorptive denitrogenation of model fossil fuels with Lewis acid-loaded metal-organic frameworks (MOFs). Chem. Eng. J. 255, 623–629. doi:10.1016/j.cej.2014.06.099
Ahmed, I., Khan, N. A., Hasan, Z., and Jhung, S. H. (2013). Adsorptive denitrogenation of model fuels with porous metal-organic framework (MOF) MIL-101 impregnated with phosphotungstic acid: Effect of acid site inclusion. J. Hazard. Mat. 250–251, 37–44. doi:10.1016/j.jhazmat.2013.01.024
Ahmed, I., Khan, N. A., and Jhung, S. H. (2013). Graphite oxide/Metal−Organic framework (MIL-101): Remarkable performance in the adsorptive denitrogenation of model fuels. Inorg. Chem. 52, 14155–14161. doi:10.1021/ic402012d
Ahmed, I., Tong, M., Jun, J. W., Zhong, C., and Jhung, S. H. (2015). Adsorption of nitrogen-containing compounds from model fuel over sulfonated metal-organic framework: Contribution of hydrogen-bonding and acid-base interactions in adsorption. J. Phys. Chem. C 120 (1), 407–415. doi:10.1021/acs.jpcc.5b10578
Al-Dalama, K., and Stanislaus, A. (2011). Temperature programmed reduction of SiO2–Al2O3 supported Ni, Mo and NiMo catalysts prepared with EDTA. Thermochim. Acta 520 (1-2), 67–74. doi:10.1016/j.tca.2011.03.017
Alexander, A. M., and Hargreaves, J. S. (2010). Alternative catalytic materials: Carbides, nitrides, phosphides and amorphous boron alloys. Chem. Soc. Rev. 39 (11), 4388. doi:10.1039/b916787k
Alonso-Pérez, M. O., Pawelec, B., Zepeda, T. A., Alonso-Núñez, G., Nava, R., Navarro, R. M., et al. (2021). Effect of the titanium incorporation method on the morphology and HDS activity of supported ternary Ni–Mo–W/SBA-16 catalysts. Microporous Mesoporous Mat. 312, 110779. doi:10.1016/j.micromeso.2020.110779
Alsolami, B. H. (2022). HDS for fuel cell applications. AvaliableAt: http://resolver.tudelft.nl/uuid:1d9702c6-5365-49a3-93db-70e350688093 (Accessed on April 07, 2022).
Anisuzzamana, S. M., Krishnaiah, D., and Alfred, D. (2018). Adsorption potential of a modified activated carbon for the removal of nitrogen containing compounds from model fuel. AIP Conf. Proc. 1930, 020013. doi:10.1063/1.5022907
Asumana, C., Yu, G., Guan, Y., Yang, S., Zhou, S., Chen, X., et al. (2011). Extractive denitrogenation of fuel oils with dicyanamide-based ionic liquids. Green Chem. 13, 3300. doi:10.1039/c1gc15747g
Awokoya, K. N., Okoya, A. A., and Elujulo, O. (2021). Preparation, characterization and evaluation of a styrene-based molecularly imprinted polymer for capturing pyridine and pyrrole from crude oil. Sci. Afr. 13, e00947. doi:10.1016/j.sciaf.2021.e00947
Badoga, S., Mouli, K. C., Soni, K. K., Dalai, A. K., and Adjaye, J. (2012). Beneficial influence of EDTA on the structure and catalytic properties of sulfided NiMo/SBA-15 catalysts for hydrotreating of light gas oil. Appl. Catal. B Environ. 125, 67–84. doi:10.1016/j.apcatb.2012.05.015
Badoga, S. (2015). Synthesis and characterization of NiMo supported mesoporous materials with EDTA and phosphorus for hydrotreating of heavy gas oil. University of Sakatchewan. Available at: https://central.bac-lac.gc.ca/.item?id=TC-SSU-2015042019&op=pdf&app=Library&oclc_number=1033227891 (Accessed July 10, 2022).
Baia, L. V., Souza, W. C., de Souza, R. J. F., Veloso, C. O., Chiaro, S. S. X., Figueiredo, M. A. G., et al. (2017). Removal of sulfur and nitrogen compounds from diesel oil by adsorption using clays as adsorbents. Energy fuels. 31 (11), 11731–11742. doi:10.1021/acs.energyfuels.7b01928
Bardi, U. (2019). Peak oil, 20 years later: Failed prediction or useful insight? Energy Res. Soc. Sci. 48, 257–261. doi:10.1016/j.erss.2018.09.022
Batten, S. R., Champness, N. R., Chen, X., Garcia-Martinez, J., Kitagawa, S., Öhrström, L., et al. (2013). Terminology of metal–organic frameworks and coordination polymers (IUPAC Recommendations 2013. Pure Appl. Chem. 85 (8), 1715–1724. doi:10.1351/pac-rec-12-11-20
Bhadra, B. N., and Jhung, S. H. (2020). Remarkable metal–organic framework composites for adsorptive removal of nitrogenous compounds from fuel. Chem. Eng. J. 398 (15), 125590. doi:10.1016/j.cej.2020.125590
Bhutto, A. W., Abro, R., Gao, S., Abbas, T., Chen, X., Yu, G., et al. (2016). Oxidative desulfurization of fuel oils using ionic liquids: A review. J. Taiwan Inst. Chem. Eng. 62, 84–97. doi:10.1016/j.jtice.2016.01.014
Bose, D. (2015). Design parameters for a hydro desulfurization (HDS) unit for petroleum naphtha at 3500 barrels per day. World Sci. News 3, 99–111.
Breysse, M., Afanasiev, P., Geantet, C., and Vrinat, M. (2003). Overview of support effects in hydrotreating catalysts. Catal. Today 86 (1-4), 5–16. doi:10.1016/s0920-5861(03)00400-0
Breysse, M., Geantet, C., Afanasiev, P., Blanchard, J., and Vrinat, M. (2008). Recent studies on the preparation, activation and design of active phases and supports of hydrotreating catalysts. Catal. Today 130 (1), 3–13. doi:10.1016/j.cattod.2007.08.018
Brunet, S., Mey, D., Pérot, G., Bouchy, C., and Diehl, F. (2005). On the hydrodesulfurization of FCC gasoline: A review. Appl. Catal. A General 278 (2), 143–172. doi:10.1016/j.apcata.2004.10.012
Budukva, S. V., Klimov, O. V., Uvarkina, D. D., Chesalov, Y. A., Prosvirin, I. P., Larina, T. V., et al. (2019). Effect of citric acid and triethylene glycol addition on the reactivation of CoMo/γ-Al2O3 hydrotreating catalysts. Catal. Today 329, 35–43. doi:10.1016/j.cattod.2018.10.017
Caero, L. C., Romero, A. R., and Ramirez, J. (2003). Niobium sulfide as a dopant for Mo/TiO2 catalysts. Catal. Today 78 (1-4), 513–518. doi:10.1016/s0920-5861(02)00342-5
Campbell, C. J., and Laherrère, J. H. (1998). The end of cheap oil: Global production of conventional oil will begin to decline sooner than most people think, probably within 10 years. Sci. Am. 278 (3), 78–83.
Campos-Martin, J. M., Capel-Sanchez, M. C., Perez-Presas, P., and Fierro, J. L. G. (2010). Oxidative processes of desulfurization of liquid fuels. J. Chem. Technol. Biotechnol. 85, 879–890. doi:10.1002/jctb.2371
Cao, Y., Liu, L., Xu, W., Wu, X., and Huang, W. (2014). Surface molecularly imprinted polymer prepared by reverse atom transfer radical polymerization for selective adsorption indole. J. Appl. Polym. Sci. 131, 40473. doi:10.1002/app.40473
Cao, Z., Zhang, X., Guo, R., Ding, S., Zheng, P., Fan, J., et al. (2020). Synergistic effect of acidity and active phases for NiMo catalysts on dibenzothiophene hydrodesulfurization performance. Chem. Eng. J. 400, 125886. doi:10.1016/j.cej.2020.125886
Carenco, S., Portehault, D., Boissiere, C., Mezailles, N., and Sanchez, C. (2013). Nanoscaled metal borides and phosphides: Recent developments and perspectives. Chem. Rev. 113 (10), 7981–8065. doi:10.1021/cr400020d
Cecilia, J. A., Infantes-Molina, A., Rodríguez-Castellón, E., and Jiménez-López, A. (2009). A novel method for preparing an active nickel phosphide catalyst for HDS of dibenzothiophene. J. Catal. 263 (1), 4–15. doi:10.1016/j.jcat.2009.02.013
Cervantes, J. M., Huirache-Acuna, R., de Leon, J. D., Moyado, S. F., Paraguay-Delgado, F., Berhault, G., et al. (2020). CoNiMo/Al2O3 sulfide catalysts for dibenzothiophene hydrodesulfurization: Effect of the addition of small amounts of nickel. Microporous Mesoporous Mat. 309, 110574. doi:10.1016/j.micromeso.2020.110574
Chen, J. G. (1996). Carbide and nitride overlayers on early transition metal surfaces: Preparation, characterization, and reactivities. Chem. Rev. 96 (4), 1477–1498. doi:10.1021/cr950232u
Chen, W., Mauge, F., van Gestel, J., Nie, H., Li, D., Long, X., et al. (2013). Effect of modification of the alumina acidity on the properties of supported Mo and CoMo sulfide catalysts. J. Catal. 304, 47–62. doi:10.1016/j.jcat.2013.03.004
Chianelli, R. R., Berhault, G., Raybaud, P., Kasztelan, S., Hafner, J., Toulhoat, H., et al. (2020). Periodic trends in hydrodesulfurization: In support of the sabatier principle. Appl. Catal. A General 227 (1-2), 83–96. doi:10.1016/s0926-860x(01)00924-3
Choudhary, T. V., Parrott, S., and Johnson, B. (2008). Understanding the hydrodenitrogenation chemistry of heavy oils. Catal. Commun. 9, 1853–1857. doi:10.1016/j.catcom.2008.03.002
Chowdari, R. K., de León, J. N. D., and Fuentes-Moyado, S. (2020). Single step and template-free synthesis of Dandelion flower-like core-shell architectures of metal oxide microspheres: Influence of sulfidation on particle morphology & hydrodesulfurization performance. Appl. Catal. B Environ. 277, 119213. doi:10.1016/j.apcatb.2020.119213
Coulier, L., De Beer, V. H. J., Van Veen, J. A. R., and Niemantsverdriet, J. W. (2001). Correlation between hydrodesulfurization activity and order of Ni and Mo sulfidation in planar silica-supported NiMo catalysts: The influence of chelating agents. J. Catal. 197 (1), 26–33. doi:10.1006/jcat.2000.3068
de León, J. D., Picquart, M., Villarroel, M., Vrinat, M., Llambias, F. G., Murrieta, F., et al. (2010). Effect of gallium as an additive in hydrodesulfurization WS2/γ-Al2O3 catalysts. J. Mol. Catal. A Chem. 323 (1-2), 1–6. doi:10.1016/j.molcata.2010.03.008
Dembaremba, Tendai O., Ogunlaja, Adeniyi S., and Tshentu, Zenixole R. (2020). “Coordination polymers and polymer nanofibers for effective adsorptive desulfurization,” in Nanocomposites for the desulfurization of fuels (Hershey, Pennsylvania, United States: IGI Global), 168–234. AvaliableAt: https://www.igi-global.com/book/nanocomposites-desulfurization-fuels/235700. doi:10.4018/978-1-7998-2146-5
Dembaremba, T. O., Correa, I., Hosten, E. C., Kuznetsov, M. L., Gerber, W. J., Pessoa, J. C., et al. (2019). New VIVO-complexes for oxidative desulfurization of refractory sulfur compounds in fuel: Synthesis, structure, reactivity trend and mechanistic studies. Dalton Trans. 48, 16687–16704. doi:10.1039/c9dt02505g
Dembaremba, T. O., van Der Westhuizen, R., Welthagen, W., Ferg, E., Ogunlaja, A. S., Tshentu, Z. R., et al. (2019). Comparing the catalytic activity of silica-supported vanadium oxides and the polymer nanofiber-supported oxidovanadium(IV) complex toward oxidation of refractory organosulfur compounds in hydrotreated diesel. Energy fuels. 33 (8), 7595–7603. doi:10.1021/acs.energyfuels.9b01579
Dincer, I., and Zamfirescu, C. (2018). 1.4 sustainability dimensions of energy. Compr. Energy Syst. 1, 101–152. doi:10.1016/b978-0-12-809597-3.00104-8
Duchet, J. C., Tilliette, M. J., Cornet, D., Vivier, L., Perot, G., Bekakra, L., et al. (1991). Catalytic properties of nickel molybdenum sulphide supported on zirconia. Catal. Today 10 (4), 579–592. doi:10.1016/0920-5861(91)80040-g
Environmental Protection Agency (2021). Air plan approval; New Hampshire; sulfur content limitations for fuels. AvaliableAt: https://www.govinfo.gov/content/pkg/FR-2021-02-08/pdf/2021-02535.pdf.
Escobar, J., Barrera, M. C., De los Reyes, J. A., Toledo, J. A., Santes, V., Colín, J. A., et al. (2008). Effect of chelating ligands on Ni-Mo impregnation over wide-pore ZrO2-TiO2. J. Mol. Catal. A Chem. 287 (1-2), 33–40. doi:10.1016/j.molcata.2008.02.022
Fetzner, S. (1998). Bacterial degradation of pyridine, indole, quinoline, and their derivatives under different redox conditions. Appl. Microbiol. Biotechnol. 49, 237–250. doi:10.1007/s002530051164
Forte, P., Sachse, A., Maes, M., Galarneau, A., and De Vos, D. (2014). Selective continuous flow extractive denitrogenation of oil containing S- and N- heteroaromatics using metal-containing ionic liquids supported on monolithic silica with hierarchical porosity. RSC Adv. 4, 1045–1054. doi:10.1039/c3ra43585g
Fuel Regulation (2022a). Diesel fuel grades. AvaliableAt: https://dieselnet.com/standards/us/fuel.php (Accessed on April 07, 2022).
Fuel Regulation (2022b). EU: Fuels: Automotive diesel fuel. AvaliableAt: https://dieselnet.com/standards/eu/fuel_automotive.php#:∼:text=EN%20590%3A2004%E2%80%94Sulfur%20limits (Accessed on April 07, 2022).
García-Gutiérrez, J. L., Laredo, G. C., Fuentes, G. A., García-Gutiérrez, P., and Jiménez-Cruz, F. (2014). Effect of nitrogen compounds in the hydrodesulfurization of straight-run gas oil using a CoMoP/δ-Al2O3 catalyst. Fuel 138, 98–103. doi:10.1016/j.fuel.2014.08.008
Ghosh, P., Hickey, K. J., and Jaffe, S. B. (2006). Development of a detailed gasoline composition-based octane model. Ind. Eng. Chem. Res. 45, 337–345. doi:10.1021/ie050811h
Global Fuel Specification (2022). Diesel sulfur reduction remains critical as prerequisite for cleaner vehicle sales. AvaliableAt: https://stratasadvisors.com/insights/2021/10282021-global-on-road-diesel-quality-outlook (Accessed on April 07, 2022).
Glotov, A. P., Vutolkina, A. V., Vinogradov, N. A., Pimerzin, A. A., Vinokurov, V. A., Pimerzin, A. A., et al. (2021). Enhanced HDS and HYD activity of sulfide Co-PMo catalyst supported on alumina and structured mesoporous silica composite. Catal. Today 377, 82–91. doi:10.1016/j.cattod.2020.10.010
Gray, M. R., Ayasse, A. R., Chan, E. W., and Veljkovic, M. (1995). Kinetics of hydrodesulfurization of thiophenic and sulfide sulfur in Athabasca bitumen. Energy fuels. 9, 500–506. doi:10.1021/ef00051a015
Grzechowiak, J. R., Rynkowski, J., and Wereszczako-Zielinska, I. (2001). Catalytic hydrotreatment on alumina-titania supported NiMo sulphides. Catal. Today 65 (2-4), 225–231. doi:10.1016/s0920-5861(00)00562-9
Han, W., Nie, H., Long, X., Li, M., Yang, Q., Li, D., et al. (2017). Effects of the support Brønsted acidity on the hydrodesulfurization and hydrodenitrogention activity of sulfided NiMo/Al2O3 catalysts. Catal. Today 292, 58–66. doi:10.1016/j.cattod.2016.11.049
Hansmeier, A. R., Meindersma, G. W., and de Haan, A. B. (2011). Desulfurization and denitrogenation of gasoline and diesel fuels by means of ionic liquids. Green Chem. 13, 1907. doi:10.1039/c1gc15196g
Harris, S., and Chianelli, R. R. (1986). Catalysis by transition metal sulfides: A theoretical and experimental study of the relation between the synergic systems and the binary transition metal sulfides. J. Catal. 98 (1), 17–31. doi:10.1016/0021-9517(86)90292-7
Hasan, Z., and Jhung, S. H. (2015). Removal of hazardous organics from water using metal-organic frameworks (MOFs): Plausible mechanisms for selective adsorptions. J. Hazard. Mat. 283, 329–339. doi:10.1016/j.jhazmat.2014.09.046
Hasan, Z., Tong, M., Jung, B. K., Ahmed, I., Zhong, C., Jhung, S. H., et al. (2014). Adsorption of pyridine over amino-functionalized Metal−Organic frameworks: Attraction via hydrogen bonding versus Base−Base repulsion. J. Phys. Chem. C 118, 21049–21056. doi:10.1021/jp507074x
Hernández-Maldonado, A. J., and Yang, R. T. (2004). Denitrogenation of transportion fuels by zeolites at ambient temperature and pressure. Angew. Chem. 116, 1022.
Hidalgo-Vivas, A., Wiwel, P., Hinnemann, B., Zeuthen, P., Petersen, B. O., and Duus, J. Ø. (2022). Refractory nitrogen compounds in hydrocracking pretreatment identification and characterization. AvaliableAt: www.researchgate.net/publication/268362629 (Accessed on April 07, 2022).
Höök, M., Bardi, U., Feng, L., and Pang, X. (2010). Development of oil formation theories and their importance for peak oil. Mar. Pet. Geol. 27 (9), 1995–2004. doi:10.1016/j.marpetgeo.2010.06.005
Huh, E. S., Zazybin, A., Palgunadi, J., Ahn, S., Hong, J., Kim, H. S., et al. (2009). Zn-containing ionic liquids for the extractive denitrogenation of a model oil: A mechanistic consideration. Energy fuels. 23, 3032–3038. doi:10.1021/ef900073a
Huirache-Acuna, R., Nava, R., Peza-Leddesma, C. L., Lara-Romero, J., Alonso-Nuez, G., Pawelec, B., et al. (2013). SBA-15 mesoporous silica as catalytic support for hydrodesulfurization catalysts—review. Materials 6 (9), 4139–4167. doi:10.3390/ma6094139
Huirache-Acuña, R., Pérez-Ayala, E., Cervantes-Gaxiola, M. E., Alonso-Núñez, G., Zepeda, T. A., Rivera-Muñoz, E. M., et al. (2021). Dibenzothiophene hydrodesulfurization over ternary metallic NiMoW/Ti-HMS mesoporous catalysts. Catal. Commun. 148, 106162. doi:10.1016/j.catcom.2020.106162
Huirache-Acuña, R., Zepeda, T. A., Rivera-Muñoz, E. M., Nava, R., Loricera, C. V., Pawelec, B., et al. (2015). Characterization and HDS performance of sulfided CoMoW catalysts supported on mesoporous Al-SBA-16 substrates. Fuel 149, 149–161. doi:10.1016/j.fuel.2014.08.045
Ibrahim, M. H., Hayyan, M., Hashima, M. A., and Hayyan, A. (2017). The role of ionic liquids in desulfurization of fuels: A review. Renew. Sustain. Energy Rev. 76, 1534–1549. doi:10.1016/j.rser.2016.11.194
ICCT (2020). Diesel sulfur content impacts on euro VI soot-free vehicles: Considerations for emerging markets. AvaliableAt: https://theicct.org/publication/diesel-sulfur-content-impacts-on-euro-vi-soot-free-vehicles-considerations-for-emerging-markets/#:∼:text=As%20the%20major%20vehicle%20markets,known%20as%20ultralow%2Dsulfur%20diesel.
ICCT (2022). Technical and economic analysis of the transition to ultra-low sulfur fuels in Brazil, China, India and Mexico. AvaliableAt: https://theicct.org/sites/default/files/publications/ICCT_ULSF_refining_Oct2012.pdf (Accessed on April 07, 2022).
IMO (2022). Imo 2020 – cutting sulphur oxide emissions. AvaliableAt: https://www.imo.org/en/MediaCentre/HotTopics/Pages/Sulphur-2020.aspx (Accessed on April 07, 2022).
Ishihara, A., Wang, D., Dumeignil, F., Amano, H., Qian, E. W., Kabe, T., et al. (2005). Oxidative desulfurization and denitrogenation of a light gas oil using an oxidation/adsorption continuous flow process. Appl. Catal. A General 279, 279–287. doi:10.1016/j.apcata.2004.10.037
Javadli, R., and de Klerk, A. (2012). Desulfurization of heavy oil. Appl. Petrochem. Res. 1, 3–19. doi:10.1007/s13203-012-0006-6
Jian, M., and Prins, R. (1998). Reaction Kinetics of the hydrodenitrogenation of decahydroquinoline over NiMo (P)/Al2O3 catalysts. Ind. Eng. Chem. Res. 37 (3), 834–840. doi:10.1021/ie9703292
Jiang, J. (2015). Metal-organic frameworks materials modeling towards engineering applications. Newton, Singapore: Jenny Stanford Publishing. AvaliableAt: www.crcpress.com/9789814613453.
Jiang, N., Jiang, B., Wang, S., and Song, H. (2021). Efficient Ni2P/Al2O3 hydrodesulfurization catalysts from surface hybridization of Al2O3 particles with graphite-like carbon. J. Taiwan Inst. Chem. Eng. 121, 139–146. doi:10.1016/j.jtice.2021.03.053
Jiang, Z., Lu, H., Zhang, Y., and Li, C. (2011). Oxidative desulfurization of fuel oils. Chin. J. Catal. 32, 707–715. doi:10.1016/s1872-2067(10)60246-x
Jin, T., Yang, Q., Meng, C., Xu, J., Liu, H., Hu, J., et al. (2014). Promoting desulfurization capacity and separation efficiency simultaneously by the novel magnetic Fe3O4@PAA@MOF-199. RSC Adv. 4, 41902–41909. doi:10.1039/c4ra06515h
Kaiser, M. J., de Klerk, A., Gary, J. H., and Handwerk, G. E. (2020). Petroleum refining technology, economics, and markets. 6th edition. Boca Raton, Florida, United States: CRC Press.
Kanda, Y., Saito, R., Ono, T., Kon, K., Toyao, T., Furukawa, S., et al. (2022). Enhancement of the hydrodesulfurization and C–S bond cleavage activities of rhodium phosphide catalysts by platinum addition. J. Catal. 408, 294–302. doi:10.1016/j.jcat.2022.03.001
Kent, J. A. (2010). Kent and riegel’s handbook of industrial Chemistry and biotechnology. New York: Springer.
Kenta, K. (2017). Network polymers derived from the integration of flexible organic polymers and rigid metal–organic frameworks. Polym. J. 49, 345–353. doi:10.1038/pj.2016.122
Khan, N. A., Hasan, Z., and Jhung, S. H. (2013). Adsorptive removal of hazardous materials using metal-organic frameworks (MOFs): A review. J. Hazard. Mat. 244–245, 444–456. doi:10.1016/j.jhazmat.2012.11.011
Kibsgaard, J., Tuxen, A., Knudsen, K. G., Brorson, M., Topsoe, H., Laegsgaard, E., et al. (2010). Comparative atomic-scale analysis of promotional effects by late 3d-transition metals in MoS2 hydrotreating catalysts. J. Catal. 272 (2), 195–203. doi:10.1016/j.jcat.2010.03.018
Kim, J. H., Ma, X., Zhou, A., and Song, C. (2006). Ultra-deep desulfurization and denitrogenation of diesel fuel by selective adsorption over three different adsorbents: A study on adsorptive selectivity and mechanism. Catal. Today 111, 74–83. doi:10.1016/j.cattod.2005.10.017
Kim, M. J., Park, S. M., Song, S., Won, J., Lee, J. Y., Yoon, M., et al. (2011). Adsorption of pyridine onto the metal organic framework MIL-101. J. Colloid Interface Sci. 361, 612–617. doi:10.1016/j.jcis.2011.05.067
Kishan, G., Coulier, L., De Beer, V. H. J., Van Veen, J. A. R., and Niemantsverdriet, J. W. (2000). Sulfidation and thiophene hydrodesulfurization activity of nickel tungsten sulfide model catalysts, prepared without and with chelating agents. J. Catal. 196 (1), 180–189. doi:10.1006/jcat.2000.3013
Kitagawa, S., Kitaura, R., and Noro, S. (2004). Functional porous coordination polymers. Angew. Chem. Int. Ed. 43, 2334–2375. doi:10.1002/anie.200300610
Kohler, F., Roth, D., Kuhlmann, E., Wasserscheid, P., and Haumann, M. (2010). Continuous gas-phase desulfurisation using supported ionic liquid phase (SILP) materials. Green Chem. 12, 979. doi:10.1039/c004883f
Kokliukhin, A., Nikulshina, M., Mozhaev, A., Lancelot, C., Blanchard, P., Briois, V., et al. (2021). Study of hydrotreating performance of trimetallic NiMoW/Al2O3 catalysts prepared from mixed MoW Keggin heteropolyanions with various Mo/W ratios. J. Catal. 403, 141–159. doi:10.1016/j.jcat.2021.02.019
Kokliukhin, A., Nikulshina, M., Mozhaev, A., Lancelot, C., Lamonier, C., Nuns, N., et al. (2021). Bulk hydrotreating MonW12-nS2 catalysts based on SiMonW12-n heteropolyacids prepared by alumina elimination method. Catal. Today 377, 26–37. doi:10.1016/j.cattod.2020.07.018
Koltai, T., Macaud, M., Guevara, A., Schulz, E., Lemaire, M., Bacaud, R., et al. (2002). Comparative inhibiting effect of polycondensed aromatics and nitrogen compounds on the hydrodesulfurization of alkyldibenzothiophenes. Appl. Catal. A General 231, 253–261. doi:10.1016/s0926-860x(02)00063-7
Kuhlmann, E., Haumann, M., Jess, A., Seeberger, A., and Wasserscheid, P. (2009). Ionic liquids in refinery desulfurization: Comparison between biphasic and supported ionic liquid phase suspension processes. ChemSusChem 2, 969–977. doi:10.1002/cssc.200900142
Kwon, J. M., Moon, J. H., Bae, Y. S., Lee, D. G., Sohn, H. C., Lee, C. H., et al. (2008). Adsorptive desulfurization and denitrogenation of refinery fuels using mesoporous silica adsorbents. ChemSusChem 1, 307–309. doi:10.1002/cssc.200700011
Laredo, G. C., Leyva, S., Alvarez, R., Mares, M. T., Castillo, J., and Cano, J. L. (2002). Nitrogen compounds characterization in atmospheric gas oil and light cycle oil from a blend of Mexican crudes. Fuel 81, 1341–1350. doi:10.1016/s0016-2361(02)00047-9
Laredo, G. C., Vega-Merino, P. M., Fuente, J. A. M., Mora-Vallejo, R. J., Meneses-Ruiz, E., Castillo, J. J., et al. (2016). Comparison of the metal–organic framework MIL-101 (Cr) versus four commercial adsorbents for nitrogen compounds removal in diesel feedstocks. Fuel 180, 284–291. doi:10.1016/j.fuel.2016.04.038
Laredo, G. C., Vega-Merino, P. M., Pérez-Romo, P., Navarrete-Bolaños, J., and Trejo-Zárraga, F. (2017). Adsorption of nitrogen compounds from diesel fuels over alumina-based adsorbent towards ULSD production. Pet. Sci. Technol. 35, 392–398. doi:10.1080/10916466.2015.1021012
Leal, E., Torres-Mancera, P., and Ancheyta, J. (2022). Catalyst stacking technology as a viable solution to ultralow sulfur diesel production. Energy fuels. 36 (6), 3201–3218. doi:10.1021/acs.energyfuels.1c04038
Lélias, M. A., Le Guludec, E., Mariey, L., Van Gestel, J., Travert, A., Oliviero, L., et al. (2010). Effect of EDTA addition on the structure and activity of the active phase of cobalt–molybdenum sulfide hydrotreatment catalysts. Catal. Today 150 (3-4), 179–185. doi:10.1016/j.cattod.2009.07.107
Leliveld, B., and Slettenhaar, B. (2022). CELESTIA™: A revolutionary new hydroprocessing catalyst providing ultra-high activity. AvaliableAt: https://www.albemarle.com/storage/wysiwyg/cat_catalyst_courier_89_celestia_final_article.pdf (Accessed on April 07, 2022).
Li, H., Li, M., Chu, Y., Liu, F., and Nie, H. (2011). Essential role of citric acid in preparation of efficient NiW/Al2O3 HDS catalysts. Appl. Catal. A General 403 (1-2), 75–82. doi:10.1016/j.apcata.2011.06.015
Lima, F., Dave, M., Silvestre, A. J. D., Branco, L. C., and Marrucho, I. M. (2019). Concurrent desulfurization and denitrogenation of fuels using deep eutectic solvents. ACS Sustain. Chem. Eng. 7, 11341–11349. doi:10.1021/acssuschemeng.9b00877
Lin, Z., Denny, S. R., and Chen, J. G. (2021). Transition metal carbides and nitrides as catalysts for thermochemical reactions. J. Catal. 404, 929–942. doi:10.1016/j.jcat.2021.06.022
Liu, X., Zhang, C., Liu, T., Jiang, Z., and Li, C. (2020). Effect of Zr on catalytic performance of unsupported Ni (Zr) Mo and Ni (Zr) W sulfide catalysts for quinoline hydrodenitrogenation. Appl. Catal. A General 604, 117744. doi:10.1016/j.apcata.2020.117744
Liu, Y., Xu, B., Qin, B., Tao, C., Cao, L., Shen, Y., et al. (2020). Novel NiMoW-clay hybrid catalyst for highly efficient hydrodesulfurization reaction. Catal. Commun. 144, 106086. doi:10.1016/j.catcom.2020.106086
Lu, L., Cheng, S., Gao, J., Gao, G., and He, M. (2007). Deep oxidative desulfurization of fuels catalyzed by ionic liquid in the presence of H2O2. Energy fuels. 21 (1), 383–384. doi:10.1021/ef060345o
Ma, X., Sakanishi, K., and Mochida, I. (1996). Hydrodesulfurization reactivities of various sulfur compounds in vacuum gas oil. Ind. Eng. Chem. Res. 35, 2487–2494. doi:10.1021/ie960137r
Maes, M., Trekels, M., Boulhout, M., Schouteden, S., Vermoortele, F., Alaerts, L., et al. (2011). Selective removal of N-heterocyclic aromatic contaminants from fuels by Lewis acidic metal-organic frameworks. Angew. Chem. Int. Ed. 50, 4210–4214. doi:10.1002/anie.201100050
Maity, S. K., Flores, G. A., Ancheyta, J., and Rana, M. S. (2008). Effect of preparation methods and content of phosphorus on hydrotreating activity. Catal. Today 130 (2-4), 374–381. doi:10.1016/j.cattod.2007.10.100
Majodina, S., Tshentu, Z. R., and Ogunlaja, A. S. (2021). Effect of adding chelating ligands on the catalytic performance of Rh-promoted MoS2 in the hydrodesulfurization of dibenzothiophene. Catalysts 11 (11), 1398. doi:10.3390/catal11111398
Mambrini, R. V., Saldanha, A. L. M., Ardisson, J. D., Araujo, M. H., and Moura, F. C. C. (2013). Adsorption of sulfur and nitrogen compounds on hydrophobic bentonite. Appl. Clay Sci. 83–84, 286–293. doi:10.1016/j.clay.2013.08.030
Marcelin, G., Cronauer, D. C., Vogel, R. F., Prudich, M. E., and Solash, J. (1986). Shale oil denitrogenation with ion exchange. 2. Evaluation of ion-exchange adsorbents and resin treatment procedures. Ind. Eng. Chem. Proc. Des. Dev. 25 (3), 747–756. doi:10.1021/i200034a026
Mathidala, S., and Ogunlaja, A. S. (2019). Selective removal of pyridine in fuel by imprinted polymer (poly 4-vinyl aniline-co-DVB) as adsorbent. Pet. Sci. Technol. 37, 1691–1703. doi:10.1080/10916466.2019.1602641
Mendoza-Nieto, J. A., Vera-Vallejo, O., Escobar-Alarcón, L., Solís-Casados, D., and Klimova, T. (2013). Development of new trimetallic NiMoW catalysts supported on SBA-15 for deep hydrodesulfurization. Fuel 110, 268–277. doi:10.1016/j.fuel.2012.07.057
Michaud, P., Lemberton, J. L., and Pérot, G. (1998). Hydrodesulfurization of dibenzothiophene and 4, 6-dimethyldibenzothiophene: Effect of an acid component on the activity of a sulfided NiMo on alumina catalyst. Appl. Catal. A General 169 (2), 343–353. doi:10.1016/s0926-860x(98)00021-0
Miles, C. E., Carlson, T. R., Morgan, B. J., Topalian, P. J., Schare, J. R., Bussell, M. E., et al. (2020). Hydrodesulfurization properties of nickel phosphide on boron‐treated alumina supports. ChemCatChem 12 (19), 4939–4950. doi:10.1002/cctc.202000755
Minaev, P. P., Nikulshin, P. A., Kulikova, M. S., Pimerzin, A. A., and Kogan, V. M. (2015). NiWS/Al2O3 hydrotreating catalysts prepared with 12-tungstophosphoric heteropolyacid and nickel citrate: Effect of Ni/W ratio. Appl. Catal. A General 505, 456–466. doi:10.1016/j.apcata.2015.05.012
Mokhtar, W. N. A. W., Bakar, W. A. W. A., Ali, R., and Abdul-Kadir, A. A. (2014). Deep desulfurization of model diesel by extraction with N, N-dimethylformamide: Optimization by Box–Behnken design. J. Taiwan Inst. Chem. Eng. 45, 1542–1548. doi:10.1016/j.jtice.2014.03.017
Moqadam, S. I., and Mahmoudi, M. (2013). Advent of nanocatalysts in hydrotreating process: Benefits and developments. Am. J. Oil Chem. Technol. 1 (2), 13–21.
Mozhaev, A. V., Nikulshin, P. A., Pimerzin, A. A., Maslakov, K. I., and Pimerzin, A. A. (2016). Investigation of co-promotion effect in NiCoMoS/Al2O3 catalysts based on Co2Mo10-heteropolyacid and nickel citrate. Catal. Today 271, 80–90. doi:10.1016/j.cattod.2015.11.002
Nadeina, K. A., Kazakov, M. O., Danilova, I. G., Kovalskaya, A. A., Stolyarova, E. A., Dik, P. P., et al. (2019). The influence of B and P in the impregnating solution on the properties of NiMo/γ-δ-Al2O3 catalysts for VGO hydrotreating. Catal. Today 329, 2–12. doi:10.1016/j.cattod.2018.12.035
Nakamura, H., Amemiya, M., and Ishida, K. (2005). Inhibition effect of hydrogen sulfide and ammonia on NiMo/Al2O3 CoMo/Al2O3, NiCoMo/Al2O3 catalysts in hydrodesulfurization of dibenzothiophene and 4, 6-dimethyldibenzothiophene. J. Jpn. Pet. Inst. 48 (5), 281–289. doi:10.1627/jpi.48.281
Nava, R., Infantes-Molina, A., Castaño, P., Guil-López, R., and Pawelec, B. (2011). Inhibition of CoMo/HMS catalyst deactivation in the HDS of 4, 6-DMDBT by support modification with phosphate. Fuel 90 (8), 2726–2737. doi:10.1016/j.fuel.2011.03.049
Nikulshina, M., Blanchard, P., Lancelot, C., Griboval-Constant, A., Marinova, M., Briois, V., et al. (2020). Genesis of active phase in MoW/Al2O3 hydrotreating catalysts monitored by HAADF and in situ QEXAFS combined to MCR-ALS analysis. Appl. Catal. B Environ. 269, 118766. doi:10.1016/j.apcatb.2020.118766
Nikulshina, M. S., Blanchard, P., Mozhaev, A., Lancelot, C., Griboval-Constant, A., Fournier, M., et al. (2018). Molecular approach to prepare mixed MoW alumina supported hydrotreatment catalysts using H4SiMon W12-nO40 heteropolyacids. Catal. Sci. Technol. 8 (21), 5557–5572. doi:10.1039/c8cy00672e
Niu, D., Zhou, Z., Wu, C., and Xu, W. (2014). Preparation and properties of magnetic molecularly imprinted polymers and their use as adsorbents for selective adsorption of indole. Adsorp. Sci. Technol. 32 (7), 509–519. doi:10.1260/0263-6174.32.7.509
Nuzhdin, A. L., Kovalenko, K. A., Dybtsev, D. N., and Bukhtiyarova, G. A. (2010). Removal of nitrogen compounds from liquid hydrocarbon streams by selective sorption on metal-organic framework MIL-101. Mendeleev Commun. 20, 57–58. doi:10.1016/j.mencom.2010.01.022
Ofoghi, S., Soleimani, M., and Ravanchi, M. T. (2021). Quinoline adsorption from organic phase on X-type zeolites: Experimental and DFT study. Can. J. Chem. Eng. 100 (4), 838–848. doi:10.1002/cjce.24201
Ogunlaja, A. S., Abdul-quadir, M. S., Kleyi, P. E., Ferg, E. E., Watts, P., Tshentu, Z. R., et al. (2017). Towards oxidative denitrogenation of fuel oils: Vanadium oxide-catalysed oxidation of quinoline and adsorptive removal of quinoline-N-oxide using 2, 6-pyridine-polybenzimidazole nanofibers. Arab. J. Chem. 12 (2), 198–214. doi:10.1016/j.arabjc.2017.05.010
Pearson, R. G. (1963). Hard and soft acids and bases. J. Am. Chem. Soc. 85 (22), 3533–3539. doi:10.1021/ja00905a001
Peña, L., Valencia, D., and Klimova, T. (2014). CoMo/SBA-15 catalysts prepared with EDTA and citric acid and their performance in hydrodesulfurization of dibenzothiophene. Appl. Catal. B Environ. 147, 879–887. doi:10.1016/j.apcatb.2013.10.019
Poulet, O., Hubaut, R., Kasztelan, S., and Grimblot, J. (1993). Modification of the site structure of MoS2/γ-Al2O3 catalysts by addition of P, F and alkaline elements. Stud. Surf. Sci. Catal. 75, 2075–2078.
Prado, G. H. C., Rao, Y., and de Klerk, A. (2017). Nitrogen removal from oil: A review. Energy fuels. 31, 14–36. doi:10.1021/acs.energyfuels.6b02779
Prins, R., and Bussell, M. E. (2012). Metal phosphides: Preparation, characterization and catalytic reactivity. Catal. Lett. 142 (12), 1413–1436. doi:10.1007/s10562-012-0929-7
Prokić-Vidojević, D., Glišić, S. B., Krstić, J. B., and Orlović, A. M. (2021). Aerogel Re/Pd-TiO2/SiO2 and Co/Mo-Al2O3/SiO2 catalysts for hydrodesulphurisation of dibenzothiophene and 4, 6-dimethyldibenzothiophene. Catal. Today 378, 10–23. doi:10.1016/j.cattod.2020.11.022
Putra, E., Pranowo, R., Sunarso, J., Indraswati, N., and Ismadji, S. (2009). Performance of activated carbon and bentonite for adsorption of amoxicillin from wastewater: Mechanisms, isotherms and kinetics. Water Res. 43, 2419–2430. doi:10.1016/j.watres.2009.02.039
Ramírez, J., Gutiérrez-Alejandre, A., Sánchez-Minero, F., MacÍas-Alcántara, V., Castillo-Villalón, P., Oliviero, L., et al. (2012). HDS of 4, 6-DMDBT over NiMoP/(x) Ti-SBA-15 catalysts prepared with H3PMo12O40. Energy fuels. 26 (2), 773–782. doi:10.1021/ef201590g
Rashidi, F., Sasaki, T., Rashidi, A. M., Kharat, A. N., and Jozani, K. J. (2013). Ultradeep hydrodesulfurization of diesel fuels using highly efficient nanoalumina-supported catalysts: Impact of support, phosphorus, and/or boron on the structure and catalytic activity. J. Catal. 299, 321–335. doi:10.1016/j.jcat.2012.11.012
Rashidi, S., Nikou, M. R. K., and Anvaripour, B. (2015). Adsorptive desulfurization and denitrogenation of model fuel using HPW and NiO-HPW modified aluminosilicate mesostructures. Microporous Mesoporous Mat. 211, 134–141. doi:10.1016/j.micromeso.2015.02.041
Rayo, P., Ramírez, J., Torres-Mancera, P., Marroquín, G., Maity, S. K., Ancheyta, J., et al. (2012). Hydrodesulfurization and hydrocracking of Maya crude with P-modified NiMo/Al2O3 catalysts. Fuel 100, 34–42. doi:10.1016/j.fuel.2011.12.004
Richter, F. P., Caesar, P. D., Meisel, S. L., and Offenhauer, R. D. (1952). Distribution of nitrogen in petroleum according to basicity. Ind. Eng. Chem. 44 (11), 2601–2605. doi:10.1021/ie50515a037
Rinne, J., and Risto, A. (2022). Scienceon. AvaliableAt: https://scienceon.kisti.re.kr/srch/selectPORSrchPatent.do?cn=USP2010087771587 (Accessed on April 07, 2022).
Sadeghbeigi, R. (2020). Fluid catalytic cracking handbook: An expert guide to the practical operation, design, and optimization of FCC units. Oxford, United Kingdom: Butterworth-Heinemann.
Saih, Y., and Segawa, K. (2009). Catalytic activity of CoMo catalysts supported on boron-modified alumina for the hydrodesulphurization of dibenzothiophene and 4, 6-dimethyldibenzothiophene. Appl. Catal. A General 353 (2), 258–265. doi:10.1016/j.apcata.2008.10.037
Saleh, T. A. (2015). Applying nanotechnology to the desulfurization process in petroleum engineering, for the book series "the advances in chemical and materials engineering (ACME). United States of America: IGI Global, 281–336. AvaliableAt: http://www.igi-global.com/book/applying-nanotechnology-desulfurization-process-petroleum/134808.
Sami, M., and Hatch, L. F. (2001). Chemistry of petrochemical processes. Houston, Texas, United States: Gulf Professional Publishing. doi:10.1016/B978-0-88415-315-3.X5000-7
Sano, Y., Choi, K. H., Korai, Y., and Mochida, I. (2004). Adsorptive removal of sulfur and nitrogen species from a straight run gas oil over activated carbons for its deep hydrodesulfurization. Appl. Catal. B Environ. 49, 219–225. doi:10.1016/j.apcatb.2003.12.007
Sau, M., Basak, K., Manna, U., Santra, M., and Verma, R. P. (2005). Effects of organic nitrogen compounds on hydrotreating and hydrocracking reactions. Catal. Today 109, 112–119. doi:10.1016/j.cattod.2005.08.007
Schlesinger, R. B. (2010). “Sulfur oxides,”. Editor C. A. McQueen. Second Edition (Amsterdam, Netherlands: Elsevier Science), 8, 277–290. 9780080468846. doi:10.1016/B978-0-08-046884-6.00915-5Compr. Toxicol.
Schneemann, A., Bon, V., Schwedler, I., Senkovska, I., Kaskel, S., Fischer, R. A., et al. (2014). Flexible metal–organic frameworks. Chem. Soc. Rev. 43, 6062–6096. doi:10.1039/c4cs00101j
Severino, F., Laine, J., and Lopez-Agudo, A. (2000). Compensation effect and dual promotion effect in activated carbon-supported CoNiMo hydrodesulfurization catalysts. J. Catal. 189 (1), 244–246. doi:10.1006/jcat.1999.2683
Shamanaev, I. V., Suvorova, A. O., Gerasimov, E. Y., Pakharukova, V. P., Panafidin, M. A., Yakovlev, I. V., et al. (2021). SRGO hydrotreating over Ni-phosphide catalysts on granulated Al2O3. Catal. Today 378, 24–32. doi:10.1016/j.cattod.2021.04.001
She, H., Ma, X., and Chang, G. (2018). Highly efficient and selective removal of N-heterocyclic aromatic contaminants from liquid fuels in a Ag(I) functionalized metal-organic framework: Contribution of multiple interaction sites. J. Colloid Interface Sci. 518 (15), 149–155. doi:10.1016/j.jcis.2018.01.114
Shi, Y., Wang, G., Mei, J., Xiao, C., Hu, D., Wang, A., et al. (2020). The Influence of pore structure and acidity on the hydrodesulfurization of dibenzothiophene over nimo-supported catalysts. ACS omega 5 (25), 15576–15585. doi:10.1021/acsomega.0c01783
Shiraishi, Y., Hirai, T., and Komasawa, I. (2001). A novel desulfurization process for fuel oils based on The formation and subsequent precipitation of S-alkylsulfonium salts. 4. Desulfurization and simultaneous denitrogenation of vacuum gas oil. Ind. Eng. Chem. Res. 40, 3398–3405. doi:10.1021/ie001091b
Shiraishi, Y., Hirai, T., and Komasawa, I. (2000). Photochemical denitrogenation processes for light oils effected by a combination of UV irradiation and liquid-liquid extraction. Ind. Eng. Chem. Res. 39, 2826–2836. doi:10.1021/ie990917a
Shiraishi, Y., Tachibana, K., Hirai, T., and Komasawa, I. (2001). A novel desulfurization process for fuel oils based on The formation and subsequent precipitation of S-alkylsulfonium salts. 3. Denitrogenation behavior of light oil feedstocks. Ind. Eng. Chem. Res. 40, 3390–3397. doi:10.1021/ie001090j
Shiraishi, Y., Tachibana, K., Hirai, T., and Komasawa, I. (2001). A novel desulfurization process for fuel oils based on The formation and subsequent precipitation of S-alkylsulfonium salts. 5. Denitrogenation reactivity of basic and neutral nitrogen compounds. Ind. Eng. Chem. Res. 40, 4919–4924. doi:10.1021/ie0104565
Sigurdson, S., Sundaramurthy, V., Dalai, A. K., and Adjaye, J. (2008). Phosphorus promoted trimetallic NiMoW/γ-Al2O3 sulfide catalysts in gas oil hydrotreating. J. Mol. Catal. A Chem. 291 (1-2), 30–37. doi:10.1016/j.molcata.2008.05.011
Singh, R., Kunzru, D., and Sivakumar, S. (2021). Enhanced catalytic activity of ultrasmall NiMoW trimetallic nanocatalyst for hydrodesulfurization of fuels. Fuel 288, 119603. doi:10.1016/j.fuel.2020.119603
Soltanali, S., Mashayekhi, M., and Mohaddecy, S. R. S. (2020). Comprehensive investigation of the effect of adding phosphorus and/or boron to NiMo/γ-Al2O3 catalyst in diesel fuel hydrotreating. Process Saf. Environ. Prot. 137, 273–281. doi:10.1016/j.psep.2020.02.033
Song, H., You, J., Liab, B., Chen, C., Huang, J., Zhang, J., et al. (2017). Synthesis, characterization and adsorptive denitrogenation performance of bimodal mesoporous Ti-HMS/KIL-2 composite: A comparative study on synthetic methodology. Chem. Eng. J. 327 (1), 406–417. doi:10.1016/j.cej.2017.06.055
Speight, J. G. (2018). Petroleum refinery processes. Hoboken, New Jersey, United States: John Wiley & Sons Inc., 1–46. doi:10.1002/0471238961.1805060919160509
Stanislaus, A., Marafi, A., and Rana, M. S. (2010). Recent advances in the science and technology of ultra-low sulfur diesel (ULSD) production. Catal. Today 153 (1-2), 1–68. doi:10.1016/j.cattod.2010.05.011
Statistical Review of World Energy. (2022). British petroleum statistical review of world energy 2021. London, United Kingdom: BP plc, AvaliableAt: https://www.bp.com/en/global/corporate/energy-economics/statistical-review-of-world-energy.html (Accessed on 07 April 2022).
Suganuma, S., Arita, K., Nakano, F., Tsuji, E., and Katada, N. (2020). Adsorption kinetics in removal of basic nitrogen-containing compounds from practical heavy oils by amorphous silica-alumina. Fuel 266, 117055. doi:10.1016/j.fuel.2020.117055
Sun, M., Nicosia, D., and Prins, R. (2003). The effects of fluorine, phosphate and chelating agents on hydrotreating catalysts and catalysis. Catal. Today 86 (1-4), 173–189. doi:10.1016/s0920-5861(03)00410-3
Sundaramurthy, V., Dalai, A. K., and Adjaye, J. (2005). Effect of EDTA on hydrotreating activity of CoMo/γ-Al2O3 catalyst. Catal. Lett. 102 (3), 299–306. doi:10.1007/s10562-005-5872-4
Tanimu, A., and Alhooshani, K. (2019). Advanced hydrodesulfurization catalysts: A review of design and synthesis. Energy fuels. 33 (4), 2810–2838. doi:10.1021/acs.energyfuels.9b00354
Tanimu, A., Ganiyu, S. A., Kozhevnikov, I., and Alhooshani, K. (2021). In-situ activation of NiMo catalyst based on support surface-bound thiols: A green approach to catalyst sulfidation and improved activity. Arab. J. Chem. 14 (4), 103030. doi:10.1016/j.arabjc.2021.103030
Théodet, M. (2010). New generation of “bulk” catalyst precursors for hydrodesulfurization synthesized in supercritical fluids. Doctoral dissertation.
Thomazeau, C., Geantet, C., Lacroix, M., Danot, M., Harlé, V., Raybaud, P., et al. (2007). Predictive approach for the design of improved HDT catalysts: γ-Alumina supported (Ni, Co) promoted Mo1− xWxS2 active phases. Appl. Catal. A General 322, 92–97. doi:10.1016/j.apcata.2007.01.016
Thompson, R. B., Druge, L. W., and Chenicek, J. A. (1949). Stability of fuel oils in storage: Effect of sulfur compounds. Ind. Eng. Chem. 41, 2715–2721. doi:10.1021/ie50480a016
Thurston, G. D. (2017). Stella R. Quah, international encyclopedia of public health. Second Edition. Cambridge, Massachusetts, United States: Academic Press, 367–377. 9780128037089. doi:10.1016/B978-0-12-803678-5.00320-9
Tian, F., Sun, X., Liu, X., Zhang, H., Liu, J., Guo, H., et al. (2020). Effective adsorptive denitrogenation from model fuels over yttrium ion-exchanged Y zeolite. Chin. J. Chem. Eng. 28 (2), 414–419. doi:10.1016/j.cjche.2019.05.014
Topalian, P. J., Carrillo, B. A., Cochran, P. M., Takemura, M. F., and Bussell, M. E. (2021). Synthesis and hydrodesulfurization properties of silica-supported nickel-ruthenium phosphide catalysts. J. Catal. 403, 173–180. doi:10.1016/j.jcat.2021.02.006
Turaga, U. T., Ma, X., and Song, C. (2003). Influence of nitrogen compounds on deep hydrodesulfurization of 4, 6-dimethyldibenzothiophene over Al2O3- and MCM-41-supported Co-Mo sulfide catalysts. Catal. Today 86, 265–275. doi:10.1016/s0920-5861(03)00464-4
UNO (2022). Four more countries in Africa switch to low sulfur fuels. AvaliableAt: https://www.unep.org/news-and-stories/blogpost/four-more-countries-africa-switch-low-sulfur-fuels#:∼:text=Morocco%20is%20actually%20the%20first,low%20sulphur%20fuels%20as%20well (Accessed on April 07, 2022).
US: Fuels: Diesel and Gasoline (2021). Transportpolicy. AvaliableAt: https://www.transportpolicy.net/standard/us-fuels-diesel-and-gasoline/#:∼:text=Sulfur%20Content&text=500%20ppm%20%E2%80%93%20Sulfur%20limit%20of,for%20heavy%2Dduty%20highway%20engines (Accessed 02 26, 21).
Valencia, D., and Klimova, T. (2011). Effect of the support composition on the characteristics of NiMo and CoMo/(Zr)SBA-15 catalysts and their performance in deep hydrodesulfurization. Catal. Today 166 (1), 91–101. doi:10.1016/j.cattod.2010.08.006
Van de Voorde, B., Boulhout, M., Vermoortele, F., Horcajada, P., Cunha, D., Lee, J. S., et al. (2013). N/S-Heterocyclic contaminant removal from fuels by the mesoporous Metal−Organic framework MIL-100: The role of the metal ion. J. Am. Chem. Soc. 135, 9849–9856. doi:10.1021/ja403571z
Van de Voorde, B., Munn, A. S., Guillou, N., Millange, F., De Vos, D. E., Walton, R. I., et al. (2013). Adsorption of N/S heterocycles in the flexible metal–organic framework MIL-53(FeIII) studied by in situ energy dispersive X-ray diffraction. Phys. Chem. Chem. Phys. 15, 8606. doi:10.1039/c3cp44349c
van Looij, F., van der Laan, P., Stork, W. H. J., Di Camillo, D. J., and Swain, J. (1998). Key parameters in deep hydrodesulfurization of diesel fuel. Appl. Catal. A General 170 (1), 1–12. doi:10.1016/s0926-860x(98)00028-3
Vatutina, Y. V., Klimov, O. V., Stolyarova, E. A., Nadeina, K. A., Danilova, I. G., Chesalov, Y. A., et al. (2019). Influence of the phosphorus addition ways on properties of CoMo-catalysts of hydrotreating. Catal. Today 329, 13–23. doi:10.1016/j.cattod.2019.01.005
Wang, F., Li, J., Xu, L., Jiang, F., and Zhang, J. (2019). Magnetic composites Fe3O4@SiO2@PILs as sorbents for efficient denitrogenation of fuel oil. Micro Nano Lett. 14 (13), 1287–1292. doi:10.1049/mnl.2019.0245
Wang, Z., Sun, Z., Kong, L., and Li, G. (2013). Adsorptive removal of nitrogen-containing compounds from fuel by metal-organic frameworks. J. Energy Chem. 22, 869–875. doi:10.1016/s2095-4956(14)60266-7
Wen, J., Lin, H., Han, X., Zheng, Y., and Chu, W. (2017). Physicochemical studies of adsorptive denitrogenation by oxidized activated carbons. Ind. Eng. Chem. Res. 56, 5033–5041. doi:10.1021/acs.iecr.6b05015
Whitehurst, D. D., Isoda, T., and Mochida, I. (1998). Present state of the art and future challenges in the hydrodesulfurization of polyaromatic sulfur compounds. Adv. Catal. 42, 345.
Whitehurst, D. D., Knudsen, K. G., Nielsen, I. V., Wiwel, P., and Zeuthen, P. (2000). The influence of trace amounts of nitrogen compounds on the achievement of future sulfur specifications in diesel fuels - Part 1: HDS inhibition studies with model N-compounds. Am. Chem. Soc. Div. Pet. Chem. Prepr. 45 (4), 692–696.
Wiwel, P., Hinnemann, B., Hidalgo-Vivas, A., Zeuthen, P., Petersen, B. O., Duus, J. Ø., et al. (2010). Characterization and identification of the most refractory nitrogen compounds in hydroprocessed vacuum gas oil. Ind. Eng. Chem. Res. 49 (7), 3184–3193. doi:10.1021/ie901473x
Wiwel, P., Knudsen, K., Zeuthen, P., and Whitehurst, D. (2000). Assessing compositional changes of nitrogen compounds during hydrotreating of typical diesel range gas oils using a novel preconcentration technique coupled with gas chromatography and atomic emission detection. Ind. Eng. Chem. Res. 39, 533–540. doi:10.1021/ie990554e
World Energy Review (2020). Eni World Oil, Gas and Renewables Review, annual statistical report. Report. AvaliableAt: https://www.eni.com/assets/documents/eng/scenari-energetici/WORLD-OIL-REVIEW-2020-vol1.pdf (Accessed on April 07, 2022).
Wu, H., Duan, A., Zhao, Z., Qi, D., Li, J., Liu, B., et al. (2014). Preparation of NiMo/KIT-6 hydrodesulfurization catalysts with tunable sulfidation and dispersion degrees of active phase by addition of citric acid as chelating agent. Fuel 130, 203–210. doi:10.1016/j.fuel.2014.04.038
Xiang, C. E., Chai, Y. M., and Liu, C. G. (2011). Effect of phosphorus on the hydrodesulfurization and hydrodenitrogenation performance of presufided NiMo/Al2O3 catalyst. J. Fuel Chem.Technol. 39 (5), 355–360. doi:10.1016/S1872-5813(11)60026-1
XiE, L., Favre-Reguillon, A., Wang, X., Fu, X., and Lemaire, M. (2010). Selective adsorption of neutral nitrogen compounds from fuel using ion-exchange resins. J. Chem. Eng. Data 55 (11), 4849–4853. doi:10.1021/je100446p
Yang, K., Chen, X., Bai, Z., and Liang, C. (2021). Noble metal silicides catalysts with high stability for hydrodesulfurization of dibenzothiophenes. Catal. Today 377, 205–212. doi:10.1016/j.cattod.2020.08.027
Yue, S., Xu, D., Sheng, Y., Yin, Z., Zou, X., Wang, X., et al. (2021). One-step synthesis of mesoporous alumina-supported molybdenum carbide with enhanced activity for thiophene hydrodesulfurization. J. Environ. Chem. Eng. 9 (4), 105693. doi:10.1016/j.jece.2021.105693
Yun, G. N., and Lee, Y. K. (2014). Dispersion effects of Ni2P catalysts on hydrotreating of light cycle oil. Appl. Catal. B Environ. 150, 647–655. doi:10.1016/j.apcatb.2014.01.010
Zeuthen, P., Blom, P., and Massoth, F. (1991). Characterization of nitrogen on aged hydroprocessing catalysts by temperature-programmed oxidation. Appl. Catal. 78, 265–276. doi:10.1016/0166-9834(91)80111-9
Zeuthen, P., Knudsen, K. G., and Whitehurst, D. D. (2001). Organic nitrogen compounds in gas oil blends, their hydrotreated products and the importance to hydrotreatment. Catal. Today 65, 307–314. doi:10.1016/s0920-5861(00)00566-6
Zhang, C., Brorson, M., Li, P., Liu, T., Jiang, Z., and Li, C. (2019). CoMo/Al2O3 catalysts prepared by tailoring the surface properties of alumina for highly selective hydrodesulfurization of FCC gasoline. Appl. Catal. A General 570, 84–95. doi:10.1016/j.apcata.2018.10.039
Zhang, H., Li, G., Jia, Y., and Liu, H. J. (2010). Adsorptive removal of nitrogen-containing compounds from fuel. J. Chem. Eng. Data 55, 173–177. doi:10.1021/je9003004
Zhang, P., Mu, F., Zhou, Y., Long, Y., Wei, Q., Liu, X., et al. (2019). Synthesis of highly ordered TiO2-Al2O3 and catalytic performance of its supported NiMo for HDS of 4, 6-dimethyldibenzothiophene. Appl. Catal. B Environ. 268, 118428.
Zhang, Y., Han, W., Long, X., and Nie, H. (2016). Redispersion effects of citric acid on CoMo/γ-Al2O3 hydrodesulfurization catalysts. Catal. Commun. 82, 20–23. doi:10.1016/j.catcom.2016.04.012
Keywords: desulfurization, denitrogenation, crude oil, fuel refinery, hydroprocessing, hydroprocessing catalysts, adsorptive denitrogenation, metal-organic frameworks
Citation: Dembaremba TO, Majodina S, Walmsley RS, Ogunlaja AS and Tshentu ZR (2022) Perspectives on strategies for improving ultra-deep desulfurization of liquid fuels through hydrotreatment: Catalyst improvement and feedstock pre-treatment. Front. Chem. 10:807225. doi: 10.3389/fchem.2022.807225
Received: 01 November 2021; Accepted: 29 June 2022;
Published: 22 July 2022.
Edited by:
Neil Coville, University of the Witwatersrand, South AfricaReviewed by:
Rafael Huirache Acuña, Michoacana University of San Nicolás de Hidalgo, MexicoXinying Liu, University of South Africa, South Africa
Copyright © 2022 Dembaremba, Majodina, Walmsley, Ogunlaja and Tshentu. This is an open-access article distributed under the terms of the Creative Commons Attribution License (CC BY). The use, distribution or reproduction in other forums is permitted, provided the original author(s) and the copyright owner(s) are credited and that the original publication in this journal is cited, in accordance with accepted academic practice. No use, distribution or reproduction is permitted which does not comply with these terms.
*Correspondence: Tendai O. Dembaremba, czIxMjM4NTY3NEBtYW5kZWxhLmFjLnph; Siphumelele Majodina, czIxNzU1MzUzMkBtYW5kZWxhLmFjLnph; Zenixole R. Tshentu, emVuaXhvbGUudHNoZW50dUBtYW5kZWxhLmFjLnph