- 1Department of Biotechnology, University of Sargodha, Sargodha, Pakistan
- 2Institute of Zoology, University of the Punjab New Campus, Lahore, Pakistan
- 3Institute of Biochemistry and Biotechnology, University of Veterinary and Animal Sciences Lahore, Lahore, Pakistan
- 4Department of Clinical Laboratory Sciences, College of Applied Medical Sciences, King Khalid University, Abha, Saudi Arabia
- 5Department of Public Health, College of Applied Medical Sciences, Khamis Mushait Campus, King Khalid University, Abha, Saudi Arabia
- 6Department of Exact Science and Technology, State University of Santa Cruz, Ilhéus, Brazil
- 7School of Medicine, Dalian University, Dalian, China
Hyaluronan is a biodegradable, biopolymer that represents a major part of the extracellular matrix and has the potential to be fabricated in a fibrous form conjugated with other polymers via electrospinning. Unique physicochemical features such as viscoelasticity, conductivity, and biological activity mainly affected by molecular weight attracted the attention of biomedical researchers to utilize hyaluronan for designing novel HA-based nano-devices. Particularly HA-based nanofibers get focused on a diverse range of applications in medical like tissue implants for regeneration of damaged tissue or organ repair, wound dressings, and drug delivery carriers to treat various disorders. Currently, electrospinning represents an effective available method for designing highly porous, 3D, HA-based nanofibers with features similar to that of the extra-cellular matrix making them a promising candidate for designing advanced regenerative medicines. This review highlights the structural and physicochemical features of HA, recently cited protocols in literature for HA production via microbial fermentation with particular focus on electrospun fabrication of HA-based nanofibers and parameters affecting its synthesis, current progress in medical applications of these electrospun HA-based nanofibers, their limitations and future perspective about the potential of these HA-based nanofibers in medical field.
1 Introduction
A unique, naturally occurring biopolymer “hyaluronan” (hyaluronic acid, HA) has received great attention in the biomaterial, bioengineering, and medical industry because of its exceptional physicochemical and biological features (Collins and Birkinshaw 2013). Biocompatibility, high moisture absorption capability, viscoelasticity, and excellent hygroscopic nature enable HA to be used as a joint structure stabilizer, shock absorber, lubricant, maintain water balance in the skin, and also serve as a flow resistance regulator (Necas et al., 2008; Liu et al., 2011; Ström et al., 2015). Key functions of HA include regulation of tissue hydration, cell adhesion, proliferation, and directed differentiation, and other responses such as wound healing, reducing inflammation, angiogenesis, and damaged tissue regeneration that makes HA an ideal candidate for bioengineering and designing novel devices for diverse applications in the medical field (Kang et al., 2011; Gayathri et al., 2018; Sihan et al., 2019). Thus, the medical and pharmaceutical industry particularly get focused on HA and constantly searching for new opportunities to optimize the production of economical, high-quality biocompatible, biodegradable HA for designing novel HA-based nano-devices for applications such as skin substitutes (Debels et al., 2015), novel wound dressings (Wang et al., 2022), drug carriers (Hosseini et al., 2021) cataract surgery, cancer treatment (Snetkov et al., 2019), tissue regeneration (Niu et al., 2021a) and hydrophilic membranes for postoperative tissue adhesion (Chen et al., 2021).
Advancement in regenerative medicines particularly relied upon designing novel 3D biocompatible nanostructures such as nanofibers (NFs) with an average diameter of <1000 nm. Currently, available synthetic tissue engineering constructs do not possess appropriate mechanical and structural features to provide an effective micro-environment to promote proliferation and cell adhesion; thus, biopolymers particularly the part of extracellular matrix (ECM) like hyaluronan (HA), collagen, fibronectin, etc. got attention and research for designing easy, cost-effective way for fabricating nanomaterials based on them is still in progress (Li et al., 2017; Tenchurin et al., 2021). Although researchers have developed several HA-based nanodevices of medical significance such as nano-hydrogels (Bhattacharya et al., 2019), liposomes (Choi et al., 2017), sponges (Niyama and Kuroyanagi, 2014), composites (Karimi et al., 2019), and nanoparticles (Fouda et al., 2016). This review particularly focuses on the promising medical applications of electrospun HA-based NFs. The unique features of NF include the 3D structure with high chemical stability, large aspect ratio, desired mechanical strength, loading efficiency, and controlled release capability enabling their widespread use in the biomedical field (Garg et al., 2015; Snetkov et al., 2019). For example, for tissue engineering, NFs are incorporated along-with seeded cells while in wound dressing high porosity of nanofibrous mat reported to improve the localized drug release which in turn may promotes the natural healing process (Jannesari et al., 2011; Ahadian et al., 2017).
Several techniques are used for nanofiber fabrication such as template synthesis (Zahmatkeshan et al., 2018), centrifugal spinning (Rim et al., 2013), drawing (Cheng et al., 2008), air jet spinning (Ashammakhi et al., 2009), phase separation (Taghavi and Larson, 2014), self-assembly (Asmatulu and Khan, 2019), melt spinning (Rim et al., 2013), electrospinning (aligned, random, core-shell NFs) (Samimi et al., 2018). Among these methods, electrospinning is an advance, relatively simple, easy way to generate micro and nanofibers of biopolymer solution. An electrospinning biopolymer such as HA solution is subjected to an electrostatic force which in turn generates porous, finished NFs with excellent surface adhesion capacity (Habibi et al., 2019). Processing conditions and biomaterial being used are key factors affecting NF’s morphology (Hajinasrollah et al., 2019). This review highlights the structural and physicochemical features of HA, natural sources, and recently reported protocols for industrial production of HA via microbial fermentation and HA-based NFs fabrication through electrospinning. Moreover, this review also reported the parameters affecting the fabrication process and morphology of NFs, medical applications of these electrospun NFs with particular focus on the recent progress in designing biocompatible HA-NFs-based tissue-engineering constructs and wound dressings to aid wound healing, limitations associated with toxic effects and future perspective regarding their wide-spread applications in biomedical industry.
2 Structural and physicochemical properties of HA
2.1 Structural features
HA is a non-sulphated, anionic and the simplest glycosaminoglycan (GAGs) comprised of N-acetylglucosamine and D-glucuronic acid repeating units up to 10,000 or more linked via β-1,3 and β-1,4-glycosidic bonds as shown in Figure 1 (Xu et al., 2012; Xing et al., 2020). HA, ability to retain large quantities of water is attributed to the abundance of the anionic hydroxyl group in the HA structure (Huang and Huang, 2018; Xing et al., 2020). This negatively charged gel-like polymeric structure not only permit HA to act as a lubricating agent for joints but also contributes to its shock-absorber ability around surrounding tissues (Xing et al., 2020). Presence of these polar and non-polar groups in the HA polymer permit it to chemically interact with other molecules such as chitosan, metachromatic dyes that results in the formation of polyelectrolyte complexes which in extend its applications in the biomedical field (Ma et al., 2012; Sridharan and Shankar, 2012).In solution, long-chain HA polymer exists in poly-dispersed form and possesses a 3D, viscous, random structure with high hydration volume that permits the free passage of micro-molecules through it but restricts the penetration of other macromolecules into the HA domain thus, contributes to the excluded volume effects of HA (Bastow et al., 2008; Xing et al., 2020).
2.2 Rheology
HA solution shows unique rheological behavior and retains its significance not only in medical, cosmetics, and bioengineering applications but also in biochemical and physiological processes and is primarily dependent on the HA structure and polyelectrolyte behavior of HA solution (Snetkov et al., 2020a). In general, the Hyaluronan solution displays viscoelastic, non-Newtonian behavior with shear-thinning (Fallacara et al., 2018; Kim et al., 2018). Various factors contribute to the shear-thinning behavior of HA solution such as increment in hydrophobic effects with enhanced shear rate and intramolecular hydrogen bond disruption. The hydrophobic effect is mainly generated by the implication of deformed HA molecular chains in flow direction which in turn reduce solution viscosity (Snetkov et al., 2020b). Falcon et al. (2006) have reported a reduction in untwining time of the 3D HA network by decreasing the molecular weight of hyaluronan. Generally, these rheological features of HA such as high viscoelasticity and high surface tension along with high hydrophilicity hinder the electrospinning of HA solution (Zhao et al., 2015). Furthermore, low vaporability and high conductivity of HA polymeric solution may cause circuit failure between needle and collector, thus limiting the efficacy of the electrospinning process (Snetkov et al., 2019).
2.3 Effect of molecular weight of HA on biological activity
Molecular mass and the HA synthesis and degradation conditions are the key factors affecting the biological activity of HA (Cyphert et al., 2015; Heldin et al., 2019). Generally, HMW (High molecular weight) and LMW (Low molecular weight) HA perform opposite biological functions (Girish and Kemparaju, 2007; Heldin et al., 2019). HMW hyaluronan (>106 g/mol) is mainly present in synovial joints as it is highly viscoelastic and thus, acts as a lubricating agent and offers protection to articulate cartilage (Tamer 2013). Moreover, it displays anti-angiogenic activity and also plays a useful role in wound healing, tissue repair, immunosuppression, and inflammation by regulating the recruitment of inflammatory cytokines, inflammatory cells, fibrinogen binding, and stem-cell migration (Girish and Kemparaju, 2007; Jiang et al., 2011). While LMW hyaluronan (2 × 104–2 × 106 g/mol) can induce tumor progression by enhancing ECM remodeling (Cyphert et al., 2015; Wu et al., 2015). LMW HA also has the potential to stimulate the secretion of growth factors, chemokines, and proinflammatory cytokines (Heldin et al., 2019). Moreover, different studies have also evaluated the effect of HA’s molecular weight on the wound healing process. It was identified that HMW HA prevents apoptosis, enhances cell quiescence, contributes to the inflammatory phase of healing, maintains tissue integrity, and stimulates in-vitro proliferation of fibroblast (Selders et al., 2017; Sasaki et al., 2011)
3 Natural sources of HA
HA is widely distributed in humans and other animals such as roosters comb. In the human body, HA is primarily present in ECM of connective tissues, the pericellular coating around the cells, skin layers (about 50%) including both dermis and epidermis, eye vitreous body (0.1 mg/ml), synovial joints that contain approximately 3–4 mg/ml (wet weight) of hyaluronan (Schiraldi et al., 2010) and in the umbilical cord (4 mg/ml) where along-with chondroitin sulphate it represents a major constituent of Wharton’s jelly (Robert et al., 2010). HA is also naturally synthesized by bacteria such as Streptococcus zooepidemicus, Streptococcus equi., Streptococcus equisemilis, Streptococcus uberis, Streptococcus pyogenes and Pasteurella multocida (Schiraldi et al., 2010; De-Oliveira et al., 2016). All of these natural producers are known pathogens that produce endotoxins. Thus, researchers are focusing on the development of endotoxin-free alternative expression systems for HA production such as Baccili or Escherichia coli (Sze et al., 2016). Moreover, HA has also been produced by Cryptococcus neoformans (De-Olivera et al., 2016), mollusks (Volpi and Maccari, 2003) and algae such as Chlorovirus infected Chlorella sp. (De-Olivera et al., 2016).
4 Industrial production of HA
HA has received extraordinary attention in the industrial sector primarily because of its unique physiochemical features such as biocompatibility, viscoelasticity, biodegradability, hygroscopicity, lubricity, non-immunogenicity, mucoadhesive features, and biological functions like anti-inflammatory, wound healing and immunosuppressive effects. Hence, there is growing interest in developing systems for optimized production of cost-effective high-quality, HMW HA (Fallacara et all., 2018). The first industrial protocol developed for HA production involved the extraction of HA from animal sources. Although improved extraction protocols have been developed with time, animal-derived HA still poses several technical issues such as harsh purification conditions, low concentration, high degradation rate due to endogenous hyaluronidases, high polymer intrinsic dispersity, and risk of contamination which in turn requires processing via costly purification techniques (Boeriu et al., 2013; De-Oliveira et al., 2016; Knopf-Marques et al., 2016). Thus, alternative methods were searched and currently, commercial production of HA mainly relied upon microbial fermentation. Initially, Streptococci strains A and C were employed for the industrial production of HA. Nowadays, various HA-based products such as Juvederm® by Allergan and Restylane® by Q-med AB are commercially produced via Streptococcus equi. HMW HA (3.5–3.9 × 106 Da) was produced at a rate of 6–7 g/L by optimizing the bacterial culture parameters at 37°C, pH 7, and in the presence of sucrose or lactose (Rangaswamy and Jain, 2008). Some recently cited protocols in the literature for HA production through microbial fermentation are enlisted in Table 1.
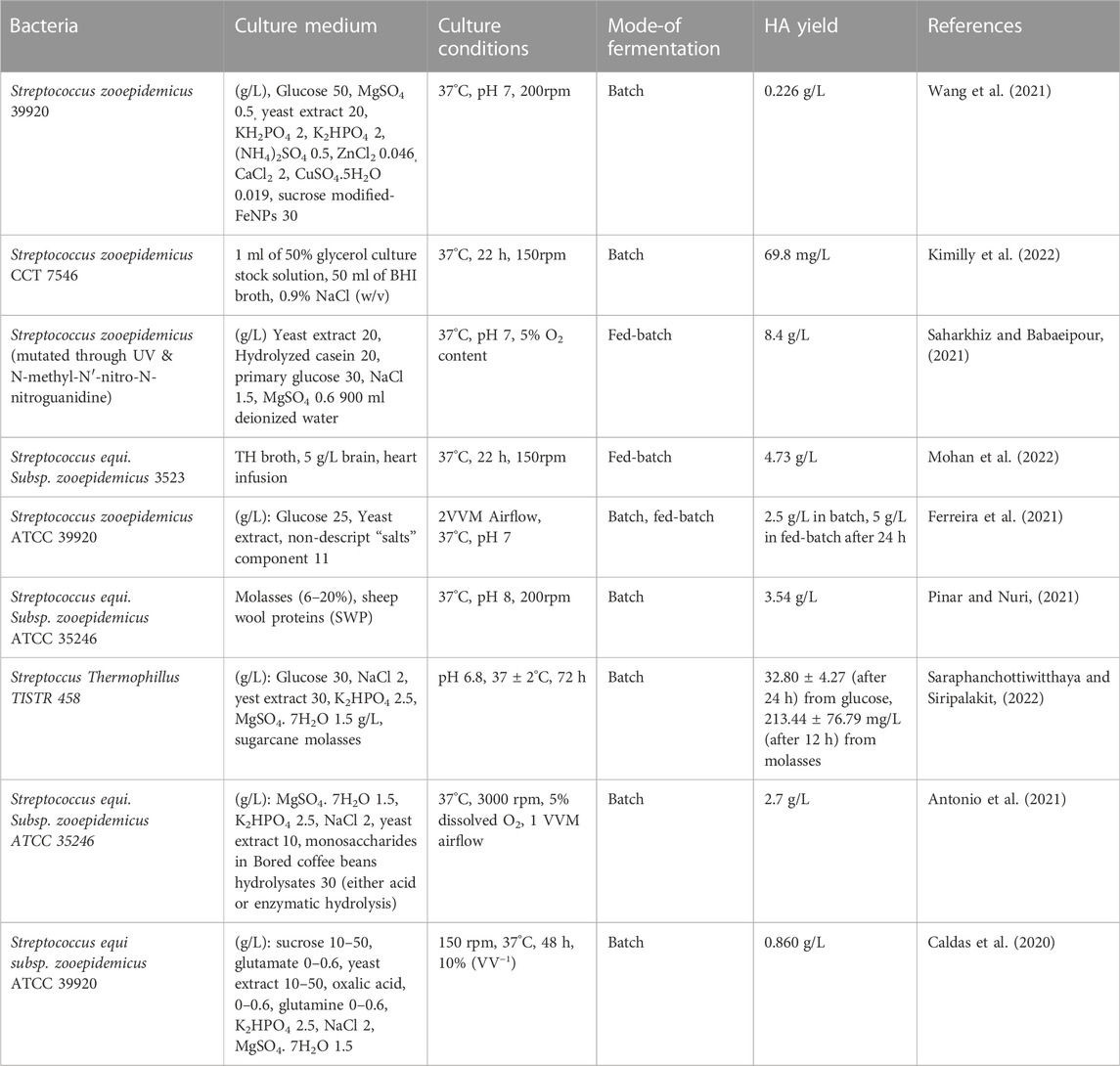
TABLE 1. Some recently cited protocols in literature for microbial production of HA via fermentation.
5 Electrospinning process
Electrospinning is a versatile, cross-sectional technique employed for the synthesis of polymers derived from micro and NFs that have found a diverse range of promising applications in the medical and pharmaceutical fields (Balusamy et al., 2017; Selders et al., 2017; Uyar and Kny, 2017). The electrospinning process involves the exposure of polymer solution to electrostatic forces and the production of a finished fibrous network thus, it is also termed hydrodynamic jetting (Jiang et al., 2015). A schematic representation of the electrospinning system is shown in Figure 2. The key component of electrospinning involves a delivery system for subjecting polymer solutions to electrostatic force. The syringe pump carries out this function and effectively delivers polymer solution to the metal needle spinneret. The second key constituent of electrospinning is a high voltage power supply (1–50 kV) applied between the spinneret and collector (Fahimirad and Ajalloueian, 2019; Memic et al., 2019; Snetkov et al., 2019).
A collector is the third central component that is a grounded, metal collecting electrode and is available in various forms such as disk, drum, plate, and mandrel. When high voltage is applied, a positively charged elongated polymer solution beam is ejected as a whip jet from the spinneret nozzle. During passage towards the collector electrode solvent evaporates and solidified network of polymer nanofiber is collected (Abrigo et al., 2014; Kalaf et al., 2017; Snetkov et al., 2019). Electrospinning of HA solution poses some challenges such as insolubility of HA in organic solvents which are usually preferred for electrospinning, probability of short-circuiting between collector electrode and spinneret needle because of high electrical conductivity of HA solution and low rate of evaporation. Advance electrospinning systems can detect any unusual circumstances and stop the process automatically (Selyanin et al., 2015).
5.1 HA-based NF fabrication via electrospinning
Several studies have reported the fabrication of HA-based NFs via electrospinning. For example, Snetkov et al. (2020a) have fabricated stable curcumin/usnic acid-loaded HA NFs via electrospinning of 1.9% (by wt.) HA solution containing curcumin/usnic acid and water-DMSO (di-methyl sulfoxide) solution at the volume ratio of 50:50. Scanning electron microscopy (SEM) images revealed the mean NFs diameter of 298 nm. Electric voltage was reported to be the most influencing factor during electrospinning and stable fiber was obtained at the applied voltage of 20–22 KV. However, some irregular morphologies i.e., beads, twisting, and drops that appeared at this voltage were reported to be greatly reduced at an applied voltage of 26–28 KV (Snetkov et al., 2020a). Authors reported that the possible occurrence of residual DMSO in electrospun NF can increase the antiseptic, analgesic, and anti-inflammatory activity of HA-NFs (Snetkov et al., 2020b). Similarly, Salim et al. (2021) have synthesized highly porous, 3D HA/PVA (Polyvinyl alcohol) mats through electrospinning. Electrospinning conditions were optimized, and stable fiber was obtained at a voltage supply of 30 KV and distance of 15 cm between the collector electrode and syringe-nozzle. Furthermore., the feeding rate of HA/PVA solution was reported to be a key factor that influences the topography of NF mat and uniform fiber was generated by optimizing the feeding rate at 0.3 ml/h. After collection, residual solvent from the NF mat was removed by heating in a dry oven for 2 h at 60°C. The biocompatibility of synthesized PVA/HA NFs was further improved by incorporating chitosan and hydroxyapatite (HAP) into the fibrous mat. Authors reported that the incorporation of chitosan greatly increased the swelling index, antimicrobial activity, protein adsorption, and hemocompatibility of PVA/HA NFs. While the incorporation of HAP significantly enhanced the thermal/mechanical stability of PVA/HA NF mats (Salim et al., 2021).
Moreover, PVA/Chi/HA NFs were also effectively fabricated via electrospinning (Hosseini et al., 2021). The polymeric solution was subjected to electrospinning at an applied voltage of 19 kV, 15 cm distance between collector and syringe needle, and a pump feeding rate of 0.8 mm/h. NF stability was further improved by exposing fibrous material to glutaraldehyde vapors (serve as crosslinking agent) for 3 h. SEM analysis showed that the mean diameter of NF before and after crosslinking was 261 ± 17 and 288 ± 20 nm. Authors reported that PVA/Chi/HA NFs exhibited significant potential for controlled release of hGA (human growth agent) with an initial burst release rate of 11% followed by gradient release of hGA up to 64% within 48 h (Hosseini et al., 2021). Niu et al. (2021a) have reported the fabrication of a porous, hierarchical, tubular HA/collagen nanofiber scaffold by electrospinning. 2% HA and 10% collagen solution were injected at the rate of 0.36 ml/L at a voltage supply of 11 KV. Electrospinning was carried out at 18–25°C, 40–45% relative humidity for 20 h and 60 h for HA and collagen solution respectively. Tensile strength and stability of HA/collagen NF scaffolds were further improved by exposing composite NF scaffolds to 2.5% glutaraldehyde vapors for 6 h that serve as crosslinking agents followed by sterilization under a UV lamp for 2 h. These double-layered HA/collagen NFs have tightly layered outer walls and loose inner layers with a mean diameter of 905 ± 113 nm. In-vitro evaluations revealed that the highly porous outer surface of tubular NF scaffold can promote the photomorphogenesis of vascular smooth muscles (SMs) via diffusion and infiltration, while the inner wall can promote the adhesion of vascular endothelial cells (ECs) (Niu et al., 2021b). Abou-Okeil et al. (2021) have reported the fabrication of novel electrospun HA/oxidized-K-carrageenan (OKC) nanofibers. Polymer solution (1% HA and OKC and 10% PVA solution) was injected into the electrospinning system at the rate of 0.5 ml/h, at 25°C ± 2°C, and voltage supply of 17.5 KV. The finished electrospun NF was collected at a distance of 10 cm from the syringe needle. Authors reported that obtained HA/OKC NF mats exhibited good antibacterial activity against both Gram -ve (E. coli) and Gram + ve (S. aureus) bacteria (Abou-Okeil et al., 2021).
6 Medical applications of HA-derived nanofibers
Currently, bioengineering and biomedical research get focused on designing biomolecules derived nanofibrous structures as the majority of human tissues (cartilage, bone, skin, etc.) exist in nanofibrous form with organized hierarchical structure (Khadka and Haynie, 2012; Selyanin et al., 2015). As HA is a central component of ECM, thus; HA-based electrospun NF has porosity, surface area, and diameter like ECM and can be a promising approach for advancement in tissue engineering, wound healing, and localized drug release. Here, recent progress in designing novel HA-based tissue implants and wound dressings is enlisted.
6.1 HA nanofibers as wound dressings:
Biomedical research is constantly searching for new opportunities to promote cost-effective wound healing therapy (Veith et al., 2019). During the inflammatory phase of the healing process, the limited number and brief life span of neutrophils make wounds highly susceptible to bacterial attack which in turn limits the efficacy of the healing process (Bjarnsholt 2013). Ideal wound dressings should maintain appropriate moisture levels, permit gaseous exchange, and absorb toxic metabolites and surplus exudates to promote cell growth and differentiation that ultimately aid in the natural healing process (Barbosa et al., 2016; Debone et al., 2019). The absence of bioactivity and biodegradability, inconvenient cleaning, hemostasis during replacement, and Potential source of allergenicity and immunogenicity make traditional wound dressings clinically less desirable (Tang et al., 2019; Ying et al., 2019). Hence, engineered biomaterials such as NF membranes have received attention as their flexible, large, porous, 3D networks identical to ECM promote biological functionalization and chemical modifications making them an ideal candidate for modern wound dressings (Chen et al., 2018). Moreover, biodegradable, biopolymers (e.g., fibroin, HA, chitosan) derived NFs as wound dressings permit incorporation and localized release of biomolecules that enhance cell adhesion, migration, and regeneration as shown in Figure 3; thus, greatly expanding their applications in wound care management (Miguel et al., 2019; Jain et al., 2020; Stojanov and Berlec, 2020).
Various studies have reported the excellent wound healing potential of HA-derived electrospun NFs. For example, Wang et al. (2022) have fabricated berberine (BBR) loaded electrospun cellulose acetate-hyaluronic acid (CA/HA) NFs. These CA/HA/BBR NFs exhibited a tensile strength of 2.99
Elibol et al. (2021) have evaluated the vocal cord wound healing potential of HA-collagen NFs in white rabbits. Results of seventh day H&E staining, Masson trichome staining, and Van Gieson staining analysis have shown that HA-collagen NFs can be employed to treat impaired viscoelasticity because of fibrosis after tissue injury and impaired voice quality in disorders that are characterized by thickening of the propria layer in the vocal cord (Elibol et al., 2021). Moreover, crosslinked HA NFs fabricated via periodate oxidation-Adipic acid dihydrazide (ADH) crosslinking approach exhibited high tensile strength up to 0.88 MPa, excellent biocompatibility, absorbance, and water resistance over 14 days making the OHA-ADH nanofibrous mats a promising wound dressing candidate (Xue et al., 2021). El-Assar et al. (2020) have fabricated AgNPs embedded NFs composed of HA and polygalactuoronic acid (PGA) via electrospinning. Nanoscale electrospinning of (Ag-PGA/HA)-PVA NF was confirmed via SEM which displayed an average diameter of 326 nm. In (Ag-PGA/HA)-PVA NF, AgNPs serve as anti-inflammatory and antioxidant agents that speed up the healing process by offering protection against augmented ROS generation. While HA constituents contribute to strain activities and high hydrophilicity. In-vivo administration of this (Ag-PGA/HA)-PVA NF has shown maximum collagen deposition and wound epithelialization after 14 days (El-Aassar et al., 2020).
6.2 HA nanofibrous scaffold mediated tissue engineering
Tissue engineering represents an advanced method of treating the complications that arise during surgical treatments and a promising technique for organ regeneration as thousands of patients worldwide suffer from organ failures annually (Dzobo et al., 2018; Rasouli et al., 2019; Lanza et al., 2020). Tissue engineering involves growing desired cells and tissues in-vitro and then implanting them into the host organism (Dzobo et al., 2018). Currently, nanofibrous tissue-engineered scaffolds have received attention as the most appropriate way to support in-vitro grown cells/tissues. Along with the biocompatible and biodegradable nature of biomolecules-derived NF scaffolds, other factors that should also be considered while designing tissue engineering scaffolds include porosity, size, and tensile strength of biological material (Sridhar et al., 2011; Khanna et al., 2021; Sharifianjazi et al., 2021). Nanofibrous scaffolds provide an innovative class of materials as their fibrous, porous structure, high surface energy, and area in contrast with bulk material and similarity with the natural ECM and biological tissues permit increased adhesion, differentiation of cells, and unhindered transport of waste and nutrients (Freed et al., 2006). Unique features of HA such as immune neutrality (Andorko and Jewell 2017; Jin et al., 2020), ability to modify the mechanical strength of ECM (Ibrahim et al., 2010), high hydrophilicity (Gebe et al., 2017), biodegradability (Fuenteslopez and Ye, 2020), and capability to bind with cell surface receptors (e.g., ICAM, CD44) (Galarza et al., 2019; Amorim et al., 2021) that promotes cell motility make HA and its derivatives highly promising candidates for tissue regeneration and surgical implants. The mode of action of HA and biopolymer-based NF scaffolds for promoting tissue regeneration is schematically described in Figure 4.
Various studies have reported the excellent tissue regeneration capacity of HA-derived NFs. For example, Niu et al. (2021c) have evaluated the effectiveness of HA functionalized collagen NFs for urethral regeneration by modifying the pro-healing phenotype expression of macrophages. HA-collagen nanofibrous mats with HA coating were fabricated via coaxial electrospinning. HA coating provides better mechanical softness and higher anisotropic wettability to NFs. ELISA and immunofluorescence assays have demonstrated that elongated macrophages growing over HA-collagen nanofibers could potentially decrease the release of inflammatory cytokines and upregulate the expression of the M2 phenotype marker. Moreover, in-vivo administration in male puppies revealed the enrichment of recruited anti-inflammatory M2 macrophages over the nanofiber surface, thus generating signals that promote urethral regeneration through the proliferation of endogenous urethral progenitor cells and angiogenesis (Niu et al., 2021a). Salim et al. (2021) have synthesized chitosan and hydroxyapatite (HAP) incorporated polyvinyl alcohol-hyaluronan (PVA/HA) NF mats and evaluate their potential for bone tissue regeneration. Authors reported that chitosan incorporation significantly increases the antimicrobial activity and swelling index of PVA/HA nanofibrous mats. While the addition of HAP improves thermal and mechanical stability. Moreover, all the tested NF mats with variable composition have shown high cell viability independent of concentration and incubation time of nanofibers. In-vitro analysis revealed a high rate of WI38 cell adherence and proliferation over HAP-loaded PVA/HA NFs making them a promising biomaterial for bone tissue regeneration (Table 2) (Salim et al., 2021).
Moreover, Martin et al. (2021), have fabricated nanofibrous HA scaffolds, which efficiently release Transforming Growth Factor-β3 (TGF-β3) and Stromal-Cell Derived Factor-1α (SDF-1α); thus, promoting cartilage tissue repair. In-vitro analysis has shown that dual factor release (TGF-β3 and SDF-1α) significantly enhances cell migration and improves matrix deposition by mesenchymal progenitor cells. While in-vivo evaluation in a large animal model to repair a large cartilage defect revealed that local release of SDF-1α impedes neo-cartilage tissue regeneration and thus, possesses lower cartilage healing potential in contrast with TGF-β3 that significantly increases cartilage tissue regeneration (Martin et al., 2021).
6.3 Electrospun HA nanofibers as drug delivery carrier
NFs have received great attention in the medical field as a carrier for localized drug release or other active substances. Usually, drugs or other active compounds are either blended with NFs or supplied in bound form by using one or more nanoparticles. Various studies have reported electrospun HA-based NFs as efficient localized drug release carriers. For example, Hosseini et al. (2021) have evaluated the efficacy of biocompatible electrospun PVA/Chi/HA NFs for sustained release of human growth hormone (hGH). Authors have reported that initial burst release of hGH (11%) occurs within the first 2 h followed by sustained release of hGH (64%) after 48 h (Hosseini et al., 2021). Initial burst release can be attributed to the hydrophilic nature of NFs and the presence of hGH molecules on NF’s surface. While later 64% hGH release from PVA/Chi/HA NF might be due to polymeric eruption (Hosseini et al., 2021). In another study, Seon-Lutz et al. (2018) have fabricated insoluble HA/PVA/HPβCD (hydroxypropyl-β-cyclodextrin) NFs via electrospinning in pure water. The drug release potential of these insoluble HA-based NFs was evaluated by using non-steroidal anti-inflammatory naproxen (NAP) as a model. NAP was impregnated into nanofibrous scaffolds either under super-critical CO2 or in an aqueous solution. The functional NFs revealed maximum drug release after 24 h while sustainable drug release potential of more than 48 h without damaging fibrous structure (Seon-Lutz et al., 2018).
7 Conclusion and future perspective
Currently, biomedical research is mainly focused on designing biocompatible, biodegradable, 3D, biopolymer-based nanostructures to overcome the challenges associated with synthetic nano-devices and to provide a microenvironment similar to that of natural ECM. These nano constructs show potential to treat medical dilemmas. Thus, biopolymers such as HA have attracted attention as it represents a key component of ECM and also possesses the ability to design fibrous structures at nanoscales for diverse medical applications. Various protocols have been developed for the economical, commercial production of HA. Although various studies have reported the fabrication of HA-based NFs still several technical issues such as circuit breaks and solution parameters such as high viscosity and conductivity of HA are required to be managed to improve the stability, tensile strength, and structural features like porosity which in regulate the biological activity of these NFs. Progress in designing optimized electrospun protocols and implantation strategies can diversify the range of medical applications of these biopolymer-based NFs.
Author contributions
H written the first draft, SB figure drawing, HS, MK, and SS performed literature review; IA, KM, and KL acquired funding, MI supervised the study, MI, MF reviewed and edited the manuscript
Funding
This work is supported by Scientific Research Deanship at King Khalid University, Abha, Saudi Arabia for their financial support through the Small Research Group Project under grant number (RGP.02-186-43) and Scientific Research Funding project of Liaoning provincial Education department of China [grant number L2019601] China.
Conflict of interest
The authors declare that the research was conducted in the absence of any commercial or financial relationships that could be construed as a potential conflict of interest.
Publisher’s note
All claims expressed in this article are solely those of the authors and do not necessarily represent those of their affiliated organizations, or those of the publisher, the editors and the reviewers. Any product that may be evaluated in this article, or claim that may be made by its manufacturer, is not guaranteed or endorsed by the publisher.
References
Abou-Okeil, A., Fahmy, H. M., Moustafa, M. G., Fouda, A. A., Aly, A. A., and Ibrahim, H. M. (2021). Hyaluronic acid/oxidized ?-carrageenan electrospun nanofibers synthesis and antibacterial properties. BioNanoScience 11, 687–695. doi:10.1007/s12668-021-00884-9
Abrigo, M., McArthur, S. L., and Kingshott, P. (2014). Electrospun nanofibers as dressings for chronic wound care: Advances, challenges, and future prospects. Macromol. Biosci. 14, 772–792. doi:10.1002/mabi.201300561
Ahadian, S., Obregón, R., Ramón-Azcón, J., Salazar, G., and Ramalingam, M. (2017). Clinical/preclinical aspects of nanofiber composites. Nanofiber Compos. Biomed. Appl., 507–528. doi:10.1016/B978-0-08-100173-8.00020-X
Amorim, S., Reis, C. A., Reis, R. L., and Pires, R. A. (2021). Extracellular matrix mimics using hyaluronan-based biomaterials. Trends Biotechnol. 39, 90–104. doi:10.1016/j.tibtech.2020.06.003
Andorko, J. I., and Jewell, C. M. (2017). Designing biomaterials with immunomodulatory properties for tissue engineering and regenerative medicine. Bio-eng Transl. Med. 2, 139–155. doi:10.1002/btm2.10063
Antonio, D., Roberto, J., Toriz, G., Arriola-Guevara, E., Guatemala-Morales, G., and Isela, R. (2021). Bored coffee beans for production of hyaluronic acid by Streptococcus zooepidemicus. Fermentation 7, 121. doi:10.3390/fermentation7030121
Ashammakhi, N., Wimpenny, I., Nikkola, L., and Yang, Y. (2009). Electrospinning: Methods and development of biodegradable nanofibres for drug release. J. Biomed. Nanotechnol. 5, 1–19. doi:10.1166/jbn.2009.1003
Balusamy, B., Senthamizhan, A., and Uyar, T. (2017). “Electrospun nanofibrous materials for wound healing applications,” in Electrospun mater (Elsevier), 147–177. Tissue Eng. Biomed. Appl. Res. Des. Commer. doi:10.1016/B978-0-08-101022-8.00012-0.
Barbosa, G. P., Debone, H. S., Severino, P., Souto, E. B., and Silva, C. F. (2016). Design and characterization of chitosan/zeolite composite films — Effect of zeolite type and zeolite dose on the film properties. Mat. Sci. Eng. C 60, 246–254. doi:10.1016/j.msec.2015.11.034
Bastow, E. R., Byers, S., Golub, S. B., Clarkin, C. E., Pitsillides, A. A., and Fosang, A. J. (2008). Hyaluronan synthesis and degradation in cartilage and bone. Cell Mol. Life Sci. 65, 395–413. doi:10.1007/s00018-007-7360-z
Bhattacharya, D., Ghosh, B., and Mukhopadhyay, M. (2019). Development of nanotechnology for advancement and application in wound healing: A review. IET Nanobiotechnol 13, 778–785. doi:10.1049/iet-nbt.2018.5312
Bjarnsholt, T. (2013). The role of bacterial biofilms in chronic infections. APMIS. Suppl. 136, 1–58. doi:10.1111/apm.12099
Boeriu, C. G., Springer, J., Kooy, F. K., van den Broek, L. A. M., and Eggink, G. (2013). Production methods for hyaluronan. Int. J. Carbohydr. Chem. 14, 1–14. doi:10.1155/2013/624967
Caldas, N. P., Biz, G., Baldo, C., and Antonia, M. P. C. (2020). Factorial design in fermentation medium development for hyaluronic acid production by Streptococcus zooepidemicus. Acta Sci. Technol., 42. doi:10.1080/10826068.2021.1955710
Chen, C.-H., Cheng, Y.-H., Chen, S.-H., Chuang, A. D.-C., and Chen, J.-P. (2021a). Functional hyaluronic acid-polylactic acid/silver nanoparticles core-sheath nanofiber membranes for prevention of post-operative tendon adhesion. Int. J. Mol. Sci. 22, 8781. doi:10.3390/ijms22168781
Chen, C.-H., Li, D.-L., Chuang, A. D.-C., Dash, B. S., and Chen, J.-P. (2021b). Tension stimulation of tenocytes in aligned hyaluronic acid/PlateletRich plasma-polycaprolactone core-sheath nanofiber membrane scaffold for tendon tissue engineering. Int. J. Mol. Sci. 22, 11215. doi:10.3390/ijms222011215
Chen, S., Carlson, M. A., Zhang, Y. S., Hu, Y., and Xie, J. (2018). Fabrication of injectable and superelastic nanofiber rectangle matrices ("peanuts") and their potential applications in hemostasis. Biomaterials 179, 46–59. doi:10.1016/j.biomaterials.2018.06.031
Cheng, F., Tao, Z., Liang, J., and Chen, J. (2008). Template-directed materials for rechargeable lithium-ion batteries. Chem. Mat. 20, 667–681. doi:10.1021/cm702091q
Choi, J. U., Lee, S. W., Pangeni, R., Byun, Y., Yoon, I.-S., and Park, J. W. (2017). Preparation and in vivo evaluation of cationic elastic liposomes comprising highly skin-permeable growth factors combined with hyaluronic acid for enhanced diabetic wound healing therapy. Acta Biomater. 57, 197–215. doi:10.1016/j.actbio.2017.04.034
Collins, M. N., and Birkinshaw, C. (2013). Hyaluronic acid based scaffolds for tissue engineering—a review. Carbohydr. Polym. 92, 1262–1279. doi:10.1016/j.carbpol.2012.10.028
Cyphert, J. M., Trempus, C. S., and Garantziotis, S. (2015). Size matters: Molecular weight specificity of hyaluronan effects in cell biology. Int. J. Cell Biol. 563818, 563818. doi:10.1155/2015/563818
De-Oliveira, J. D., Carvalho, L. S., Gomes, A. M., Queiroz, L. R., Magalhães, B. S., and Parachin, N. S. (2016). Genetic basis for hyper production of hyaluronic acid in natural and engineered microorganisms. Microb. Cell Fact. 15, 119. doi:10.1186/s12934-016-0517-4
Debels, H., Hamdi, M., Abberton, K., and Morrison, W. (2015). Dermal matrices and bioengineered skin substitutes: A critical review of current options. Plast. Reconstr. Surg. Glob. Open 3, e284. doi:10.1097/GOX.0000000000000219
Debone, H. S., Lopes, P. S., Severino, P., Yoshida, C. M. P., Souto, E. B., and Silva, C. F. (2019). Chitosan/Copaiba oleoresin films for would dressing application. Int. J. Pharm. 555, 146–152. doi:10.1016/j.ijpharm.2018.11.054
Dzobo, K., Thomford, N. E., Senthebane, D. A., Shipanga, H., Rowe, A., Dandara, C., et al. (2018). Advances in regenerative medicine and tissue engineering: Innovation and transformation of medicine. Stem Cells Int. 2018, 1–24. doi:10.1155/2018/2495848
El-Aassar, M. R., Ibrahim, O. M., Fouda, M. M. G., El-Beheri, N. G., and Agwa, M. M. (2020). Wound healing of nanofiber comprising polygalacturonic/hyaluronic acid embedded silver nanoparticles: In-vitro and in-vivo studies. Carbohydr. Polym. 238, 116175. doi:10.1016/j.carbpol.2020.116175
Elibol, E., Fuat, Y. Y., Unal, A., Ozcan, M., Yurtsever, N. K., Oguzhan, R. K., et al. (2021). Effects of hyaluronic acid-collagen nanofibers on early wound healing in vocal cord trauma. Eur. Archives Oto-Rhino-Laryngology 278, 1537–1544. doi:10.1007/s00405-021-06703-x
Elisabeth, M., Steel, Jean-Yves Azar., and Harini, G. (2019). Electrospun hyaluronic acid-carbon nanotube nanofibers for neural engineering. Materialia 9, 100581. doi:10.1016/j.mtla.2019.100581
Fahimirad, S., and Ajalloueian, F. (2019). Naturally-derived electrospun wound dressings for target delivery of bio-active agents. Int. J. Pharm. 566, 307–328. doi:10.1016/j.ijpharm.2019.05.053
Falcone, S. J., Palmeri, D. M., and Berg, R. A. (2006). Rheological and cohesive properties of hyaluronic acid. J. Biomed. Mat. Res. A 76, 721–728. doi:10.1002/jbm.a.30623
Fallacara, A., Baldini, E., Manfredini, S., and Vertuani, S. (2018). Hyaluronic acid in the third millennium. Polymers 10, 701. doi:10.3390/polym10070701
Ferreira, R. G., Azzoni, A. R., Santana, M. H. A., and Petrides, D. (2021). Techno-economic analysis of a hyaluronic acid production process utilizing streptococcal fermentation. Processes 9, 241. doi:10.3390/pr9020241
Fouda, M. M., Abdel-Mohsen, A. M., Ebaid, H., Hassan, I., Al-Tamimi, J., Abdel-Rahman, R. M., et al. (2016). Wound healing of different molecular weight of hyaluronan; in-vivo study. Int. J. Biol. Macromol. 89, 582–591. doi:10.1016/j.ijbiomac.2016.05.021
Freed, L. E., Guilak, F., Guo, X. E., Gray, M. L., Tranquillo, R., Holmes, J. W., et al. (2006). Advanced material strategies for tissue engineering scaffolds. Tissue Eng. 12, 3410–3418. doi:10.1002/adma.200900303
Fuenteslopez, C. V., and Ye, H. (2020). Electrospun fibres with hyaluronic acid-chitosan nanoparticles produced by a portable device. Nanomaterials 10, 2016. doi:10.3390/nano10102016
Galarza, S., Kim, H., Atay, N., Peyton, S. R., and Munson, J. M. (2019). 2D or 3D? How cell motility measurements are conserved across dimensions in vitro and translate in vivo. Bioeng. Transl. Med. 5, e10148. doi:10.1002/btm2.10148
Garg, T., Rath, G., and Goyal, A. K. (2015). Biomaterials-based nanofiber scaffold: Targeted and controlled carrier for cell and drug delivery. J. Drug Target 23, 202–221. doi:10.3109/1061186X.2014.992899
Gayathri, K., Vadim, A., and Maheswari, U. K. (2018). Nanoimmunotherapy – cloaked defenders to breach the cancer fortress. Nanotechnol. Rev. 7, 317–340. doi:10.1515/ntrev-2018-0013
Gebe, J. A., Yadava, K., Ruppert, S. M., Marshall, P., Hill, P., Falk, B. A., et al. (2017). Modified high-molecular weight hyaluronan promotes allergen-specific immune tolerance. Am. J. Respir. Cell Mol. Biol. 56, 109–120. doi:10.1165/rcmb.2016-0111OC
Girish, K. S., and Kemparaju, K. (2007). The magic glue hyaluronan and its eraser hyaluronidase: A biological overview. Life Sci. 80, 1921–1943. doi:10.1016/j.lfs.2007.02.037
Habibi, S., Saket, M., Nazockdast, H., and Hajinasrollah, K. (2019). Fabrication and characterization of exfoliated chitosan-gelatin-montmorillonite nanocomposite nanofibers. J. Text. Inst. 110, 1672–1677. doi:10.1080/00405000.2019.1613029
Hajinasrollah, K., Habibi, S., and Nazockdast, H. (2019). Fabrication of gelatin–chitosan–gum tragacanth with thermal annealing cross-linking strategy. J. Eng. Fibers Fabr. 14, 155892501988114–155892501988119. doi:10.1177/1558925019881142
Heldin, P., Lin, C. Y., Kolliopoulos, K., Chen, Y. H., and Skandalis, S. S. (2019). Regulation of hyaluronan biosynthesis and clinical impact of excessive hyaluronan production. Matrix Biol. 78-79, 100–117. doi:10.1016/j.matbio.2018.01.017
Hosseini, H., Khodabandeh, M. S., Amani, A., and Landi, F. S. (2021). Electrospinning of polyvinyl alcohol/chitosan/hyaluronic acid nanofiber containing growth hormone and its release investigations. Polym. Adv. Technol. 32, 574–581. doi:10.1002/pat.5111
Huang, G., and Huang, H. (2018). Application of hyaluronic acid as carriers in drug delivery. Drug Deliv. 25, 766–772. doi:10.1080/10717544.2018.1450910
Ibrahim, S., Kang, Q. K., and Ramamurthi, A. (2010). The impact of hyaluronic acid oligomer content on physical, mechanical, and biologic properties of divinyl sulfone-crosslinked hyaluronic acid hydrogels. J. Biomed. Mater Res. A 94A, 355–370. doi:10.1002/jbm.a.32704
Jain, R., Shetty, S., and Yadav, K. S. (2020). Unfolding the electrospinning potential of biopolymers for preparation of nanofibers. J. Drug Deliv. Sci. Technol. 57, 101604. doi:10.1016/j.jddst.2020.101604
Janmohammadi, M., Nourbakhsh, M. S., and Bonakdar, S. (2021). Electrospun skin tissue engineering scaffolds based on polycaprolactone/hyaluronic acid/L-ascorbic acid. Fibers Polym. 22, 19–29. doi:10.1007/s12221-021-0036-8
Jannesari, M., Varshosaz, J., Morshed, M., and Zamani, M. (2011). Composite poly(vinyl alcohol)/poly(vinyl acetate) electrospun nanofibrous mats as a novel wound dressing matrix for controlled release of drugs. Int. J. Nanomedicine 6, 993–1003. doi:10.2147/IJN.S17595
Jiang, D., Liang, J., and Noble, P. W. (2011). Hyaluronan as an immune regulator in human diseases. Physiol. Rev. 91, 221–264. doi:10.1152/physrev.00052.2009
Jiang, T., Carbone, E. J., Lo, K. W.-H., and Laurencin, C. T. (2015). Electrospinning of polymer nanofibers for tissue regeneration. Prog. Polym. Sci. 46, 1–24. doi:10.1016/j.progpolymsci.2014.12.001
Jin, Y. J., Koh, R. H., Kim, S. H., Kim, K. M., Park, G. K., and Hwang, N. S. (2020). Injectable anti-inflammatory hyaluronic acid hydrogel for osteoarthritic cartilage repair. Korean J. Couns. Psychother. 115, 111096. doi:10.1016/j.msec.2020.111096
Kalaf, E. A. G., Hixon, K. R., Kadakia, P. U., Dunn, A. J., and Sell, S. A. (2017). “Electrospun biomaterials for dermal regeneration,” in Electrospun mater (Elsevier), 179–232. Tissue Eng. Biomed. Appl. Res. Des. Commer. doi:10.1016/B978-0-08-101022-8.00005-3
Kang, J. H., Kim, Y. Y., Chang, J. Y., and Kho, H. S. (2011). Influences of hyaluronic acid on the anticandidal activities of lysozyme and the peroxidase system. Oral Dis. 17, 577–583. doi:10.1111/j.1601-0825.2011.01807.x
Kang, L., Jia, W., Li, M., Wang, Q., Wang, C., Liu, Y., et al. (2019). Hyaluronic acid oligosaccharide-modified collagen nanofibers as vascular tissue-engineered scaffold for promoting endothelial cell proliferation. Carbohydr. Polym. 223, 115106. doi:10.1016/j.carbpol.2019.115106
Karimi, D. N., Minaiyan, M., Talebi, A., Akbari, V., and Taheri, A. (2019). Nanocrystalline cellulose-hyaluronic acid composite enriched with GM-CSF loaded chitosan nanoparticles for enhanced wound healing. Biomed. Mat. 14, 035003. doi:10.1088/1748-605X/ab026c
Khadka, D. B., and Haynie, D. T. (2012). Protein- and peptide-based electrospun nanofibers in medical biomaterials. Nanomed. Nanotechnol. Biol. Med. 8, 1242–1262. doi:10.1016/j.nano.2012.02.013
Khanna, A., Zamani, M., and Huang, N. F. (2021). Extracellular matrix-based biomaterials for cardiovascular tissue engineering. J. Cardiovasc. Dev. Dis. 8, 137. doi:10.3390/jcdd8110137
Kim, J., Chang, J.-Y., Kim, Y.-Y., Kim, M.-J., and Kho, H.-S. (2018). Effects of molecular weight of hyaluronic acid on its viscosity and enzymatic activities of lysozyme and peroxidase. Arch. Oral Biol. 89, 55–64. doi:10.1016/j.archoralbio.2018.02.007
Kimilly, W. V., Rayane, W. D., Fernandes, C. de-A., Silvino, E. D., and Caninde, F. (2022). Physiochemical characterization and in-vitro antioxidant activity of hyaluronic acid produced by Streptococcus zooepidemicus CCT 7546. Prep. Biochem. Biotechnol. 52, 234–243. doi:10.1080/10826068.2021.1929320
Knopf-Marques, H., Pravda, M., Wolfova, L., Velebny, V., Schaaf, P., Vrana, N. E., et al. (2016). Hyaluronic acid and its derivatives in coating and delivery systems: Applications in tissue engineering, regenerative medicine and immunomodulation. Adv. Health C. Mat. 5, 2841–2855. doi:10.1002/adhm.201600316
Lanza, R., Langer, R., Vacanti, J. P., and Atala, A. (2020). Principles of tissue engineering. London United Kingdom: Academic Press.
Li, M., Zhang, X., Jia, W., Wang, Q., Liu, Y., Wang, X., et al. (2019). Improving in vitro biocompatibility on biomimetic mineralized collagen bone materials modified with hyaluronic acid oligosaccharide. Mater. Sci. Eng. C 104, 110008. doi:10.1016/j.msec.2019.110008
Li, Y., Xiao, Y., and Liu, C. (2017). The horizon of materiobiology: A perspective on material-guided cell behaviors and tissue engineering. Chem. Rev. 117, 4376–4421. doi:10.1021/acs.chemrev.6b00654
Liu, L., Liu, Y., Li, J., Du, G., and Chen, J. (2011). Microbial production of hyaluronic acid: Current state, challenges and perspectives. Microb. Cell Fact. 10, 99. doi:10.1186/1475-2859-10-99
Ma, G., Liu, Y., Fang, D., Chen, J., Peng, C., Fei, X., et al. (2012). Hyaluronic acid/chitosan polyelectrolyte complexes nanofibers prepared by electrospinning. Mat. Lett. 74, 78–80. doi:10.1016/j.matlet.2012.01.012
Mahmood, U., Masood, R., Ali, M. A., Ali, Z. R., Abid, S., Zahir, A., et al. (2022). Development of zinc, silver, and hyaluronic acid mediated wet spun alginate fibers for potential wound care applications. J. Industrial Text. 51, 1916S–1930S. doi:10.1177/15280837221090666
Martin, A. R., Patel, J. M., Locke, R. C., Eby, M. R., Saleh, K. S., Sennett, M. L., et al. (2021). Nanofibrous hyaluronic acid scaffolds delivering TGF-β3 and SDF-1α for articular cartilage repair in a large animal model. Acta Biomater. 126, 170–182. doi:10.1016/j.actbio.2021.03.013
Mays, E. A., Kallakuri, S. S., and Sundararaghavan, H. G. (2020). Heparin-hyaluronicacid nanofibers for growth factor sequestration in spinal cord repair. J. Biomed. Mater Res. A 108, 2023–2031. doi:10.1002/jbm.a.36962
Memic, T., Abudula, H. S., Mohammed, K., Navare, J. K., Colombani, T., and Bencherif, S. A. (2019). Latest progress in electrospun nanofibers for wound healing applications. ACS Appl. Bio Mat. 2, 952–969. doi:10.1021/acsabm.8b00637
Miguel, S. P., Sequeira, R. S., Moreira, A. F., Cabral, C. S. D., Mendonca, A. G., Ferreira, P., et al. (2019). An overview of electrospun membranes loaded with bioactive molecules for improving the wound healing process. Eur. J. Pharm. Biopharm. 139, 1–22. doi:10.1016/j.ejpb.2019.03.010
Mohan, N., Sai, S. P., Jayakumar, A., Rathinavelu, S., and Sivaprakasam, S. (2022). Real-time metabolic heat-based growth rate soft sensor for monitoring and control of high molecular weight hyaluronic acid production by Streptococcus zooepidemicus. Appl. Microbiol. Biotechnol. 106, 1079–1095. doi:10.1007/s00253-022-11760-1
Necas, J., Bartosicova, L., Brauner, P., and Kolar, J. (2008). Hyaluronic acid (hyaluronan): A review. Veterin Med. 53, 397–411. doi:10.17221/1930-VETMED
Niiyama, H., and Kuroyanagi, Y. J. (2014). Development of novel wound dressing composed of hyaluronic acid and collagen sponge containing epidermal growth factor and vitamin C derivative. Artif. Organs 17, 81–87. doi:10.1007/s10047-013-0737-x
Niu, Y., Galluzi, M., Deng, F., Zhao, Z., Fu, M., Su, L., et al. (2021c). A biomimetic hyaluronic acid-silk fibroin nanofiber scaffold promoting regeneration of transected urothelium. Bioeng. Transl. Med. 10268, e10268. doi:10.1002/btm2.10268
Niu, Y., Galluzzi, M., Fu, M., Hu, J., and Xia, H. (2021b). In vivo performance of electrospun tubular hyaluronic acid/collagen nanofibrous scaffolds for vascular reconstruction in the rabbit model. J. Nanobiotechnol 19, 349. doi:10.1186/s12951-021-01091-0
Niu, Y., Stadler, F. J., Yang, X., Deng, F., Liu, G., and Xia, H. (2021a). HA-coated collagen nanofibers for urethral regeneration via in situ polarization of M2 macrophages. J. Nanobiotechnol 19, 283. doi:10.1186/s12951-021-01000-5
Pinar, N. A., and Nuri, M. A. (2021). Evaluation of sheep wool protein hydrolysate and molasses as low-cost fermentation substrates for hyaluronic acid production by Streptococcus zooepidemicus ATCC 35246. Waste Biomass Valorization 12, 925–935. doi:10.1007/s12649-020-01062-w
Rangaswamy, V., and Jain, D. (2008). An efficient process for production and purification of hyaluronic acid from Streptococcus equi subsp. zooepidemicus. Zooepidemicus. Biotechnol. Lett. 30, 493–496. doi:10.1007/s10529-007-9562-8
Rasouli, R., Barhoum, A., Bechelany, M., and Dufresne, A. (2019). Nanofibers for biomedical and healthcare applications. Macromol. Biosci. 19, 1800256. doi:10.1002/mabi.201800256
Rim, N. G., Shin, C. S., and Shin, H. (2013). Current approaches to electrospun nanofibers for tissue engineering. Biomed. Mater 8, 014102. doi:10.1088/1748-6041/8/1/014102
Robert, L., Robert, A. M., and Renard, G. (2010). Biological effects of hyaluronan in connective tissues, eye, skin, venous wall. Role in aging. Pathol. Biol. 58, 187–198. doi:10.1016/j.patbio.2009.09.010
Saharkhiz, S., and Babaeipour, V. (2021). The dilution effect of media culture on mixing time, KIa O2, and hyaluronic acid production in S. zooepidemicus fed-batch culture. Biotechnol. Lett. 43, 2217–2222. doi:10.1007/s10529-021-03192-0
Salim, S. A., Loutfy, S. A., El-Fakharany, E. M., Taha, T. H., Hussein, Y., and Kamoun, E. A. (2021). Influence of chitosan and hydroxyapatite incorporation on properties of electrospun PVA/HA nanofibrous mats for bone tissue regeneration: Nanofibers optimization and in-vitro assessment. J. Drug Deliv. Sci. Technol. 62, 102417. doi:10.1016/j.jddst.2021.102417
Samimi, G. S., Habibi, S., and Nazockdast, H. (2018). Fabrication and characterization of chitosan/gelatin/thermoplastic polyurethane blend nanofibers. J. Text. Fibrous Mat. 1, 251522111876932–251522111876938. doi:10.1177/2515221118769324
Saraphanchotiwitthaya, A., and Siripalakit, P. (2022). Production of hyaluronic acid from molasses by Streptococcus thermophilus TISTR 458. Trends Sci. 19, 2192. doi:10.48048/tis.2022.2192
Sasaki, Y., Uzuki, M., Nnohmi, K., Kitagawa, H., Kamataki, A., Komagamine, M., et al. (2011). Quantitative measurement of serum hyaluronic acid molecular weight in rheumatoid arthritis patients and the role of hyaluronidase. Int. J. Rheum. Dis. 14, 313–319. doi:10.1111/j.1756-185X.2011.01683.x
Schiraldi, C., La Gatta, A., and De Rosa, M. (2010). Biotechnological production and application of hyaluronan. Biopolymers 20. doi:10.5772/10271
Selders, G. S., Fetz, A. E., Gehrmann, C. J., and Bowlin, G. L. (2017). “Electrospun systems for drug delivery,” in Electrospun mater (Elsevier), 115–146. Tissue Eng. Biomed. Appl. Res. Des. Commer.
Selyanin, M. A., Khabarov, V. N., and Boykov, P. Y. (2015). Hyaluronic acid: Production, properties, application in biology and medicine. Chichester, UK: John Wiley & Sons, 215. doi:10.1002/9781118695920
Seon-Lutz, M., Couffin, A. C., Vignoud, S., Schlatter, G., and Hebraud, A. (2018). Electrospinning in water and in situ crosslinking of hyaluronic acid/cyclodextrin nanofibers: Towards wound dressing with controlled drug release. Carbohydr. Polym. 207, 276–287. doi:10.1016/j.carbpol.2018.11.085
Sharifianjazi, F., Esmaeilkhanian, A., Moradi, M., Pakseresht, A., Asl, M. S., Karimi-Maleh, H., et al. (2021). Biocompatibility and mechanical properties of pigeon bone waste extracted natural nano-hydroxyapatite for bone tissue engineering. Mater. Sci. Eng. B 264, 114950. doi:10.1016/j.mseb.2020.114950
Sihan, G., Dongwei, F., Assem, U., Dejun, S., Mo, Z., Zheng, J., et al. (2019). Applications of polymer-based nanoparticles in vaccine field. Nanotechnol. Rev. 8, 143–155. doi:10.1515/ntrev-2019-0014
Snetkov, P., Morozkina, S., Olekhnovich, R., Hong, T. N. V., Tyanutova, M., and Uspenskaya, M. (2020b). Curcumin/usnic acid-loaded electrospun nanofibers based on hyaluronic acid. Materials 13, 3476. doi:10.3390/ma13163476
Snetkov, P., Morozkina, S., Uspenskaya, M., and Olekhnovich, R. (2019). Hyaluronan-based nanofibers: Fabrication, characterization and application. Polymers 11, 2036. doi:10.3390/polym11122036
Snetkov, P., Zakharova, K., Morozkina, S., Olikhnovich, R., and Uspenskaya, M. (2020a). Hyaluronic acid: The influence of molecular weight on structural, physical, physico-chemical, and degradable properties of biopolymer. Polymers 12, 1800. doi:10.3390/polym12081800
Sridhar, R., Venugopal, J. R., Sundarrajan, S., Ravichandran, R., Ramalingam, B., and Ramakrishna, S. (2011). Electrospun nanofibers for pharmaceutical and medical applications. J. Drug Deliv. Sci. Technol. 21, 451–468. doi:10.1016/S1773-2247(11)50075-9
Sridharan, G., and Shankar, A. A. (2012). Toluidine blue: A review of its chemistry and clinical utility. J. Oral. Maxillofac. Pathol. 16, 251–255. doi:10.4103/0973-029x.99081
Stojanov, S., and Berlec, A. (2020). Electrospun nanofibers as carriers of microorganisms, stem cells, proteins, and nucleic acids in therapeutic and other applications. Front. Bioeng. Biotechnol. 8, 130. doi:10.3389/fbioe.2020.00130
Ström, A., Larsson, A., and Okay, O. (2015). Preparation and physical properties of hyaluronic acid-based cryogels. J. Appl. Polym. Sci. 132, app.42194. doi:10.1002/app.42194
Sze, J. H., Brownlie, J. C., and Love, C. A. (2016). Biotechnological production of hyaluronic acid: A mini review. 3 Biotech. 367, 67. doi:10.1007/s13205-016-0379-9
Taghavi, S. M., and Larson, R. G. (2014). Erratum: Regularized thin-fiber model for nanofiber formation by centrifugal spinning [Phys. Rev. E89, 023011 (2014)]. Phys. Rev. E. 89, 059903. doi:10.1103/physreve.89.059903
Tamer, T. M. (2013). Hyaluronan and synovial joint: Function, distribution and healing. Interdiscip. Toxicol. 6, 111–125. doi:10.2478/intox-2013-0019
Tang, p., Han, L., Li, P., Jia, Z., Wang, K., Zhang, H., et al. (2019). Mussel-inspired electroactive and antioxidative scaffolds with incorporation of polydopamine-reduced graphene oxide for enhancing skin wound healing. ACS Appl. Mater Interfaces 11, 7703–7714. doi:10.1021/ACSAMI.8B18931
Tenchurin, T. K., Shepelev, A. D., Belousov, S. I., Puchkov, A. A., Yastremskii, E. V., and Chvalun, S. N. (2021). Production of nanofiber materials based on macromolecular hyaluronic acid by electrospinning. Nanobiotechnology Rep. 16, 89–95. doi:10.1134/S2635167621010092
Uyar, T., and Kny, E. (2017). Electrospun materials for tissue engineering and biomedical applications: Research, design and commercialization. 1st ed. Cambridge, UK: Woodhead Publishing.
Veith, A. P., Henderson, K., Spencer, A., Sligar, A. D., and Baker, A. B. (2019). Therapeutic strategies for enhancing angiogenesis in wound healing. Adv. Drug Deliv. Rev. 146, 97–125. doi:10.1016/j.addr.2018.09.010
Volpi, N., and Maccari, F. (2003). Purification and characterization of hyaluronic acid from the mollusc bivalve Mytilus galloprovincialis. Biochimie 85, 619–625. doi:10.1016/s0300-9084(03)00083-x
Wang, F., Wang, X., Hu, N., Qin, G., Ye, B., and He, J-S. (2022). Improved antimicrobial ability of dressings containing berberine loaded cellulose acetate/hyaluronic acid electrospun fibers for cutaneous wound healing. J. Biomed. Nanotechnol. 18, 77–86. doi:10.1166/jbn.2022.3225
Wang, J., He, W., Wang, T., Li, M., and Li, X. (2021). Sucrose-modified iron nanoparticles for highly efficient microbial production of hyaluronic acid Streptococcus zooepidemicus. Colloids Surfaces B Biointerfaces 205, 111854. doi:10.1016/j.colsurfb.2021.111854
Wu, M., Cao, M., He, Y., Liu, Y., Yang, C., Du, Y., et al. (2015). A novel role of low molecular weight hyaluronan in breast cancer metastasis. FASEB J. 29, 1290–1298. doi:10.1096/fj.14-259978
Xing, F., Zhou, C., Hui, D., Du, C., Wu, L., Wang, L., et al. (2020). Hyaluronic acid as a bioactive component for bone tissue regeneration: Fabrication, modification, properties, and biological functions. Nanotechnol. Rev. 9, 1059–1079. doi:10.1515/ntrev-2020-0084
Xu, X., Jha, A. K., Harrington, D. A., Farach-Carson, M. C., and Jia, X. (2012). Hyaluronic acid-based hydrogels: From a natural polysaccharide to complex networks. Soft Matter 8, 3280–3294. doi:10.1039/C2SM06463D
Xue, F., Zhang, H., Hu, J., and Liu, Y. (2021). Hyaluronic acid nanofibers crosslinked with a nontoxic reagent. Carbohydr. Polym. 259, 117757. j.carbpol. doi:10.1016/j.carbpol.2021.117757
Yang, Q., Xie, Z., Hu, J., and Liu, Y. (2021). Hyaluronic acid nanofiber mats loaded with antimicrobial peptide towards wound dressing applications. Mater. Sci. Eng. C 128, 112319. doi:10.1016/j.msec.2021.112319
Ying, H., Zhou, J., Wang, M., Su, D., Ma, Q., Lv, G., et al. (2019). In situ formed collagen-hyaluronic acid hydrogel as biomimetic dressing for promoting spontaneous wound healing. Mater Sci. Eng. C Mater Biol. Appl. 101, 487–498. doi:10.1016/j.msec.2019.03.093
Zahmatkeshan, M., Adel, M., Bahrami, S., Esmaeili, F., Mahdi, S. R., Saeedi, Y., et al. (2018). Polymer based nanofibers: preparation, fabrication, and applications, in Handbook of nanofibers. doi:10.1007/978-3-319-53655-2
Zhang, D., Huang, X., Wang, H., Wei, Y., Yang, Z., Huang, R., et al. (2021). Core–shell poly(l-lactic acid)-hyaluronic acid nanofibers for cell culture and pelvic ligament tissue engineering. J. Biomed. Nanotechnol. 17, 399–406. doi:10.1166/jbn.2021.3057
Keywords: hyaluronic acid, nanofibers, electrospinning, tissue engineering, wound healing
Citation: Humaira , Raza Bukhari SA, Shakir HA, Khan M, Saeed S, Ahmad I, Muzammil K, Franco M, Irfan M and Li K (2022) Hyaluronic acid-based nanofibers: Electrospun synthesis and their medical applications; recent developments and future perspective. Front. Chem. 10:1092123. doi: 10.3389/fchem.2022.1092123
Received: 07 November 2022; Accepted: 14 December 2022;
Published: 23 December 2022.
Edited by:
Muhammad Ikram, Pir Mehr Ali Shah Arid Agriculture University, PakistanReviewed by:
Azza Hosni Mohamed, University of Florida, United StatesAhmad A Omar, University of Florida, United States
Seema Satti, Pir Mehr Ali Shah Arid Agriculture University, Pakistan
Copyright © 2022 Humaira, Raza Bukhari, Shakir, Khan, Saeed, Ahmad, Muzammil, Franco, Irfan and Li. This is an open-access article distributed under the terms of the Creative Commons Attribution License (CC BY). The use, distribution or reproduction in other forums is permitted, provided the original author(s) and the copyright owner(s) are credited and that the original publication in this journal is cited, in accordance with accepted academic practice. No use, distribution or reproduction is permitted which does not comply with these terms.
*Correspondence: Muhammad Irfan, aXJmYW4uYXNocmFmQHVvcy5lZHUucGs=; Kun Li, bGlrdW5AZGx1LmVkdS5jbg==