- Department of Oncology, Tongji Hospital, Tongji Medical College, Huazhong University of Science and Technology, Wuhan, China
Nanozymes are nanomaterials with mimicked enzymatic activity, whose catalytic activity can be designed by changing their physical parameters and chemical composition. With the development of biomedical and material science, artificially created nanozymes have high biocompatibility and can catalyze specific biochemical reactions under biological conditions, thus playing a vital role in regulating physiological activities. Under pathological conditions, natural enzymes are limited in their catalytic capacity by the varying reaction conditions. In contrast, compared to natural enzymes, nanozymes have advantages such as high stability, simplicity of modification, targeting ability, and versatility. As a result, the novel role of nanozymes in medicine, especially in tumor therapy, is gaining increasing attention. In this review, function and application of various nanozymes in the treatment of cancer are summarized. Future exploration paths of nanozymes in cancer therapies based on new insights arising from recent research are outlined.
Introduction
Cancer is the main cause of death and has a significant negative impact on both the economy and quality of life (Sung et al., 2021). Although conventional cancer treatment strategies such as radiotherapy, chemotherapy, and immunotherapy have been developed and validated for various types of cancers, the clinical efficacy of these therapies is still restricted. Drug tolerance, toxic side effects, radiation resistance and immune evasion remain formidable obstacles in cancer therapy. As the incidence and mortality of malignancies continue to rise, novel therapeutic agents have long been sought by scientists (Bray et al., 2021).
Enzymes are proteins or nucleic acids synthesized in an organism as a biological catalyst. They can be involved directly or indirectly in a variety of life processes in organisms, including cell metabolism, proliferation, differentiation, and aging. Enzymes have also been shown to contribute to the emergence of diseases. Tyrosinase, for instance, is required for the body to synthesize melanin and its absence can cause albinism (Song et al., 2018; Michaud et al., 2022). Numerous lung illnesses, including asthma and chronic obstructive pulmonary disease, have been linked to nitric oxide synthases (Scott et al., 2021). It has been demonstrated that matrix metalloproteinases, which are overexpressed in tumor cells, facilitate cancer metastasis (Shi et al., 2015; Chen et al., 2020). However, protease and ribonuclease easily and quickly break down natural enzymes, making it challenging to store and transport them. On the other hand, the use of enzymes in therapeutic settings is constrained by the strict conditions that natural enzyme catalysis requires, such as a particular pH and temperature.
With the rapid development of nanotechnology, nanomaterials which can be manipulated on atomic and molecular scale are applied in medicine (Chai et al., 2022; Wang et al., 2022c; Zhang et al., 2022d; Farheen et al., 2022; Yang et al., 2022). Novel nanomaterials have demonstrated outstanding potentials in clinical applications with diverse functions (Filik et al., 2021; Li et al., 2022b; Kim et al., 2022). Nanozymes are artificial nanomaterials that exhibit intrinsic catalytic properties similar to that of natural enzymes and are attracting a massive attention (Jiang et al., 2019; Liang and Yan, 2019). To date, substantial nanomaterials have been discovered to possess enzyme-like activities, including carbon-based, metal-based, metal oxide-based and metal chalcogenide nanozymes (Robert and Meunier, 2022). By modifying structural composition and surface properties, nanozymes with different enzyme-like activities can be obtained, catalyzing the conversion of substrates into products and speeding up biological reactions under appropriate physiological circumstances. In contrast to natural enzymes, nanozymes possess the advantages of high stability, simplicity of modification, targeting ability, and low cost (Wang et al., 2019a). As a result, the application trend of nanozymes in tumor treatment has become a hotspot. However, there are few reviews on nanozymes for antitumor therapies.
In this review, the recent research achievements and progress of nanozymes in cancer treatment are highlighted (Scheme 1). Firstly, we summarize the mechanisms underlying the most common antitumor effect of nanozymes. Then the promising applications and defects of nanozyme-based synergistic antitumor strategies are discussed.
Antitumor mechanisms of nanozymes
In recent decades, Nanozymes in oncology are compelling hotspots. With the advancement of material science, the design and synthesis of nanozymes is becoming more and more sophisticated (Jiang et al., 2019). Due to their excellent performance, nanozymes have developed into powerful tools for the treatment of tumors. Nanozymes that are more widely used in tumor therapy are peroxidase (POD), oxidase (OXD), catalase (CAT), and superoxide dismutase (SOD) (Figure 1). In this section, we discuss the anticancer mechanisms of nanozymes based on the recent reports (Figure 2).
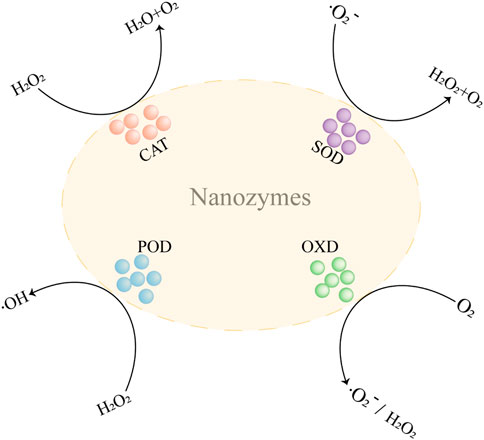
FIGURE 1. The catalysis reaction mediated by nanozymes. CAT, catalase; POD, peroxidase; OXD, oxidase; SOD, superoxide dismutase; H2O2, hydrogen peroxide; O2, oxygen; •OH, hydroxyl radicals; •O2−, superoxide radicals.
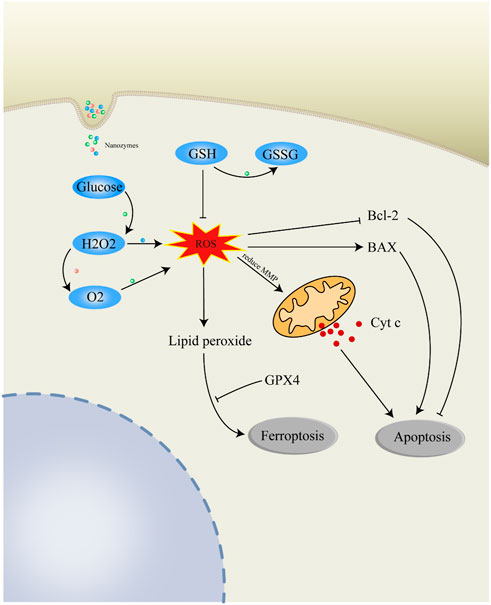
FIGURE 2. Anticancer mechanisms of nanozymes. Catalase (CAT)-like nanozymes can catalyze the decomposition of H2O2 and product O2 for the alleviation of hypoxia in TME. Peroxidase (POD)-like nanozymes can promote the degradation of H2O2 to produce highly toxic ROS. Oxidase (OXD)-like nanozymes can metabolize O2 to produce ROS. Nanozymes with glucose oxidase activity can promote the oxidation of glucose and generate H2O2 for further reaction. GSH can deplete intracellular ROS and be consumed by nanozymes to generate GSSG. Excessive intracellular ROS regulate mitochondria-related Bcl-2/BAX/Cyt c apoptosis pathway. Highly active ROS can form lipid peroxide which can induce ferroptosis of cells. ROS, reactive oxygen species; H2O2, hydrogen peroxide; O2, oxygen; GSH, glutathione; GSSG, glutathione disulfide; Cyt c, cytochrome c; Bcl-2, apoptosis regulator Bcl-2; BAX, apoptosis regulator BAX; GPX4, phospholipid hydroperoxide glutathione peroxidase.
Apoptosis induction
Apoptosis as a programmed cell death maintains the balance between cell death and cell proliferation, regulating the process of carcinogenesis. One of the characteristics of cancer is evasion of apoptosis, which makes cancer cells live with the ability to keep proliferating and escape cell death (Hanahan and Weinberg, 2011). Due to this characteristic of cancer, apoptosis induction becomes an effective approach for cancer therapy, which can kill malignant cells with no activation of an inflammatory response and little side effects to healthy cells (Chaudhry et al., 2022).
Nanozymes with multiple enzymatic activities can effectively produce reactive oxygen species in the tumor microenvironment through cascade catalytic reactions, thereby promoting the apoptosis of cancer cells (Zhao et al., 2021b; Yao et al., 2022b; Wang et al., 2022f). Ma et al. (2022) designed nanozymes with three nanoceria structures including nanoceria-cube, nanoceria-poly and nanoceria-rod. Among them, nanoceria-rod with highest concentration of surface oxygen vacancies has the highest POD-like and OXD-like enzyme activity. Due to the suitable isoelectric point, nanoceria-rod nanozyme can selectively enter the lysosomes and phagosomes of tumor cells in acidic environments. Then a large amount of ROS is produced and mitochondrial membrane potential is reduced, resulting in malignant cell apoptosis with no toxicity to normal cells.
Typically, the mechanism of nanozymes to induce apoptosis is mainly via mitochondrial apoptotic pathway. For example, PP-MnO(x) NPs, an OXD-like nanozyme, can catalyze O2 to produce abundant ROS and induce the decrease of mitochondrial membrane potential. Meanwhile, PP-MnO(x) NPs activate BAX and Caspase-3, as well as decrease the expression of Bcl-2 (Xi et al., 2021). Moreover, Chu et al. (2020) Synthesized M@AAO@HFe–TA, a nanozyme system with amino acid oxidase (AAO) and POD activity. M@AAO@HFe–TA system induced apoptotic in tumor due to amino acid starvation and cascade Fenton reaction effect. The expression of Bcl-2 was suppressed and the level of BAX was increased, releasing the cytochrome C (cyt C) to the further active caspase 3. These suggest that nanozymes induce ROS generation and oxidative stress to initiate mitochondrial-related apoptosis.
Ferroptosis induction
Ferroptosis is a type of iron-dependent non-apoptotic cell death related to abnormal level of intracellular ferrous iron. Recently, ferroptosis was found to be widely present in many cancers with characteristics of mitochondrial damage and plasma membrane disrepair (Chen et al., 2021b; Tang et al., 2021). The induction of ferroptosis can potentiate the efficacy of chemotherapy, radiotherapy and immunotherapy in drug-resistant cancer (Friedmann Angeli et al., 2019; Ye et al., 2020; Fan et al., 2021), suggesting that targeting ferroptosis strategy is a viable method for cancer treatment (Mou et al., 2019).
Ferroptosis is mainly provoked by ROS accretion and unrestricted lipid peroxidation, which is induced by excessive endogenous Fenton reaction and the exhaustion of GSH (Wei et al., 2020; Yang et al., 2021). Thus, nanozymes with peroxidase-like and glutathione peroxidase-like enzyme activity are designed as ferroptosis inducer for killing cancer cells (Meng et al., 2021). Zhang et al. (2022b) fabricated gemcitabine (Gem)-loaded carbonaceous nanoparticles (MFC-Gem) as peroxidase-like and glutathione oxidase-like nanozymes. The dual-activity MFC-Gem nanozyme effectively catalyzes the Fenton reaction to generate huge ROS and deplete GSH for oxidative damage. Meanwhile, glutathione peroxidase 4 (GPX4), a lipid repair enzyme which can use GSH to detoxify lipid peroxidation, loss activities in MFC and MFC-Gem treated mice, making cancer cells more susceptible to ferroptosis. Similarly, boron and nitrogen cooped graphdiyne (BN-GDY) as a metal-free nanozyme possesses capability to produce •OH in the presence of H2O2 and boost GSH depletion, setting the onset of ferroptosis. Interestingly, unlike the most reported enzymes, the catalytic reaction of BN-GDY undergoes a symbolic sequence Bi−Bi mechanism instead of a ping-pong Bi−Bi mechanism (Zhang et al., 2022a). Moreover, Fenton reaction-independent ferroptosis catalyzed by photothermal nanozyme is a novel insight for ferroptosis-related therapy, due to its satisfactory therapeutic effects in tumor therapy (Xing et al., 2021).
Tumor microenvironment regulation
Due to uncontrolled cell proliferation, aberrant cancer cell metabolism, and abnormal blood vessel development, tumor microenvironment (TME) is generally characterized by hypoxia (Semenza, 2000). The hypoxic TME make tumor cells exhibit increased drug efflux and lessen ROS-induced DNA damage, resulting in strong drug tolerance and radiation resistance (Brizel et al., 1999; Brown and Wilson, 2004; Gacche and Assaraf, 2018; Li et al., 2022a; Li et al., 2022c; Wang et al., 2022e). Nevertheless, the intrinsic hypoxia in TME suppresses the infiltration of anti-tumor immune cells and even induces the polarization of macrophages and T cells to pro-tumor subtype, such as M2 macrophages and regulatory T cells (Zhang et al., 2022c). Therefore, hypoxic TME hinders the efficacy of immunotherapy and augmenting O2 supply becomes urgent to improve cancer treatment (Kopecka et al., 2021). Nanozymes with CAT-mimic activity can transfer H2O2 to O2 and supply enough O2 to reverse hypoxia tumor microenvironment. Combined with GOx-like nanozymes which consume glucose and produced H2O2, self-supply O2 system is proposed by scientists (Yu et al., 2022).
Yu et al. (2022) modified carbon nitride (C3N4) with polydopamine before coating it with MIL-100, Gox, and hyaluronic acid to create a PCMGH nanozyme system. C3N4, as a water-splitting material, catalyzes H2O to generate O2, whereas MIL-100 acts like POD to raise the quantity of ROS. Upon 808 nm irradiation, polydopamine acts as a photothermal agent which convert light energy into hyperthermia to achieve photothermal therapy. The self-supplying O2 and photothermal effect work synergically to improve the performance of chemotherapy and phototherapy.
The mesoporous silica nanorod was served as nanoplatform to integrate MnO2 and Au nanoparticles, forming a new biomimetic nanozyme with dual enzyme activities. By decomposing H2O2, MnO2 can catalyze the production of O2, displaying enhanced CAT-like activity. Meanwhile, the Au nanoparticles which exhibit GOx-like activity effectively accelerate the oxidation of glucose and provide enough H2O2 for more O2 production. The alleviation of hypoxic environment surrounding tumor sensitizing tumor cells to radiation therapy and photothermal therapy (Yang et al., 2020).
In addition to directly enhance the efficacy of antitumor treatment such as chemotherapy, phototherapy and radiotherapy, increasing O2 level in vivo can change the expression of genes linked with tumor metabolism, proliferation and metastasis via regulating hypoxia-inducible factor 1 (HIF-1) pathway. Au-Pt nanozymes are reported to relieve the hypoxic TME and suppress the expression of HIF-1α, thus reducing lung metastasis (Shen et al., 2022).
Nanozyme-based enhanced cancer therapies
Though traditional treatments for cancer such as chemotherapy, radiotherapy and Immunotherapy have been widely practiced. Low response rate, drug resistance and toxic side effects still are obstacles that have not yet been overcome. In recent years, nanozymes are employed in multiple cancer treatments due to their various mimic enzyme activities. In this section, we focused on the synergy of nanozymes and conventional cancer therapy (Table 1).
Nanozymes in phototherapy
Phototherapies including photothermal therapy (PTT) and photodynamic therapy (PDT) are considered as novel strategies employed widely in tumor treatment, due to their advantages of highly selective, negligible side effects, and minimally invasive (Vieyra-Garcia and Wolf, 2021). PDT provides oxidative damage on the tumor primarily through singlet oxygen, which is converted from triplet molecular oxygen by the photosensitizer as part of the photoconversion reaction (Zheng et al., 2022). While PTT initiates tumor cell death predominantly through hyperthermia via plasmonic dissipation, which is converted by the energy of photons absorbed on the photothermal agent under near infrared (NIR) laser irradiation (Figure 3). However, strict conditions such as oxygen leakage, acidic condition and overexpression of GSH in the tumor microenvironment (TME) constrain the photothermal conversion efficacy and ROS production of Phototherapies (Song et al., 2022). Photosensitive nanozymes which can remodel the restricted environment in tumor are intensively studied by scientists to achieve better phototherapeutic efficiency (Wang et al., 2022a; Tang et al., 2022).
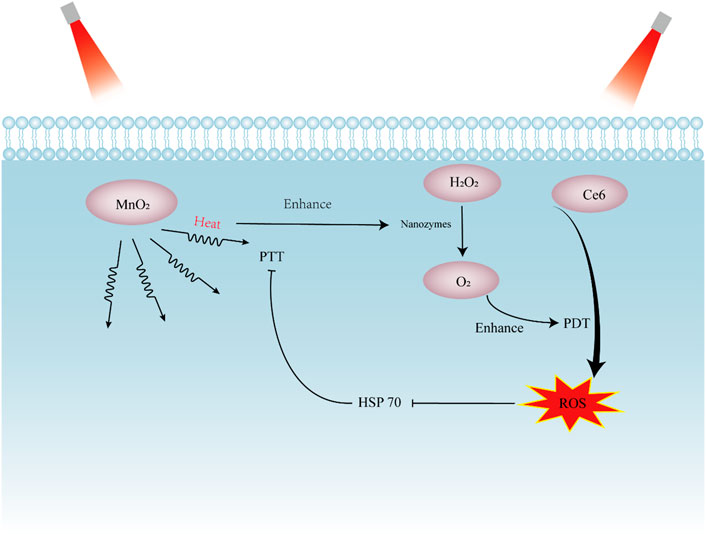
FIGURE 3. Schematic presentation showing the synergistic effect of nanozymes in photothermal therapy (PTT) and photodynamic therapy (PDT). The photosensitizer Ce6 absorb light and generate cytotoxic ROS. MnO2 act as photothermal agents to converts absorbed light into heat and kill tumor cells via hyperthermia. PDT decreases the expression of HSP70 to increase sensitivity of tumors to PTT. The heat produced by PTT can enhance the activity of nanozymes, augmenting PDT effect. Ce6, chlorin e6; MnO2, manganese dioxide; HSP 70, heat shock protein 70; ROS, reactive oxygen species.
In fact, the ROS generation in PDT often ceases with oxygen depletion. To solve this dilemma, an intelligent nanoplatform Ce6/Ftn@MnO2 with the ability of O2 self-supply was constructed, which can reverse unfavorable hypoxia conditions and decrease the expression of hypoxia-inducible factor (HIF)-1α. With adequate O2, Ce6/Ftn@MnO2 produced a significantly higher cytotoxic 1O2 amount. The enhanced photodynamic effect of Ce6/Ftn@MnO2 caused reduction of mitochondrial membrane potential and disruption of lysosome integrity, resulting in tumor cell death (Zhu et al., 2022b). To achieve profound photothermal therapeutic efficacy and minimized side effects, a “dual lock-and-key” type tumor-specific nanozyme (ABTS@PAH-CNts) was designed with higher selectivity. Activated under both H2O2 and acidic pH, ABTS@PAH-CNts present remarkable photothermal effect, with negligible off-target hyperthermic damage to normal tissues (Chen et al., 2021c).
It is worth noting that PTT can be used to integrate with PDT to get better synergistic antitumor effect. For instance, Ni0.5Fe0.5S2 efficiently converts near-infrared light energy into heat for realizing the photothermal therapy. Moreover, the peroxidase-like property of Ni0.5Fe0.5S2 triggers formation of cytotoxic •OH and 1O2 to further enhance the efficiency of PDT (Zeng et al., 2022a). Similarly, Song et al. (2022) generated a PTT-PDT dual mode nanoplatform of RGD-BPNS@SMFN, which had self-synergetic behavior and enhanced photonic response than single PDT and PTT. The key self-synergetic mechanism is the PTT promoted temperature-dependent CAT-like activity, which alleviates the hypoxia microenvironment and further encourages the PDT behavior. Indeed, PTT induced hyperthermic temperature can accelerate enzyme-catalyzed ROS generation, which enhances the further PDT therapeutic performance (Zhou et al., 2022). On the other hand, ROS produced by PDT decreases the expression of heat shock protein 70 (HSP70), which was found to protect cells from the damage of heat, thus augmenting photothermal effect (Sun et al., 2021b). Taken together, the combination of PTT and PDT achieves strikingly therapeutic efficacy.
Nanozymes in immunotherapy
In recent decades, immunotherapy is a hot topic in cancer therapy which revolutionizes cancer treatment because of its striking tumor ablation effect. Multiple immunotherapeutic strategies, including cancer vaccines, immune checkpoint blockade (ICB), and adoptive cell transfer, have been developed by scientists (Rudd, 2019; He and Xu, 2020). However, the application of cancer immunotherapy is limited by low response rates and individual differences mainly due to immunosuppressive TME and heterogenicity. Nanozymes can act as a modulator to reverse immunosuppressive TME by degrading immunosuppressive molecules, inducing infiltration of anti-tumor immune cells and repolarizing pro-tumor cells (Wang et al., 2022b). Thus, nanozymes can be utilized as adjuvant therapeutics to boost immunotherapy efficacy.
The ChA CQDs nanozyme, formed by carbon quantum dots and chlorogenic acid, was reported with GSH oxidase-like activities and induction of cell ferroptosis. In addition to directly inducing cancer cell death through catalytic therapy, ChA CQDs activated the tumor immune microenvironment and converted the “cold tumor” to “hot tumor” by recruiting CD4+/CD8+ T cells, macrophages, and NK cells (Figure 4). The increase in immune cells provides an important basis for subsequent immunotherapy, changing the situation that immunotherapy is ineffective in several types of cancer (Yao et al., 2022a).
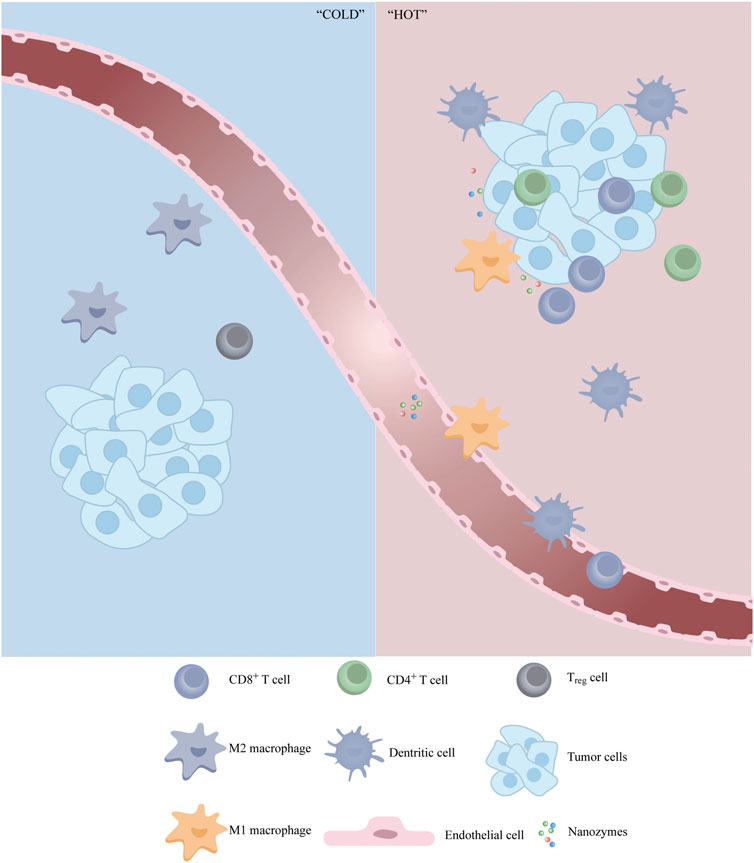
FIGURE 4. Schematic illustration of the synergistic effect of nanozymes in immunotherapy. Nanozymes can act as modulator to reverse immunosuppressive TME by inducing infiltration of anti-tumor immune cells and repolarizing pro-tumor cells.
A novel nanozyme (CuPP) with trienzyme-like activities has been proposed to efficiently modulate immunosuppressive TME by releasing O2 and •OH but consuming glutathione (GSH). With the reversion of hypoxic microenvironment, macrophages in tumor site were repolarized from pro-tumoral M2 to anti-tumoral M1 phenotype, accompanied by the upregulation of IL-12 and the downregulation of IL-10. Moreover, the ratio of CD4+ and CD8+ T cells was elevated by further combining with αPD-L1, exhibiting strong immune responses (Zeng et al., 2022b). Except for αPD-L1, the synergistic effect is also achieved by combining nanozymes with CTLA-4 blockade. CaO2/DOX@SiO2/DOX-MnO2 (CDSDM) nanozyme reactor was reported with self-oxygenation and alleviation of hypoxia-adenosinergic signaling. Through the positive immune modulation of CDSDM NRs, the infiltration of cytotoxic T cells was facilitated and the population of immunosuppressive regulatory T cells was decreased. On the other hand, the DOX in CDSDM NRs promoted the maturation of antigen-presenting dendritic cells. Thus, the reversion of the immunosuppressive TME is expected to favor CTLA-4-mediated immunotherapy (Wang et al., 2019b).
HA@Cu2−xS were synthesized by hyaluronic acid, CuCl2 and Na2S, which were further modified by PEG to form HA@Cu2−xS-PEG nanozymes (PHCNs). The PHCNs nanosystem effectively triggers apoptosis of tumor cells due to its dual photothermal and peroxidase-like catalytic properties. Tumor-specific antigen released from dead tumor cells facilitates the infiltration and activation of chimeric antigen receptor (CAR) T cells, presenting a synergistic effect with CAR T-cell therapy (Zhu et al., 2021).
Nanozymes in radiotherapy
Radiotherapy occupies an essential position in cancer treatment and has been widely applied for solid tumors in the clinic. However, radioresistance, mainly caused by hypoxia and antioxidant environment around tumor, constrains the efficacy of radiotherapy (Dai et al., 2022). On the one hand, tumor cells overexpress the DNA repair proteins, making them more endurable than normal cells (Roos et al., 2016). On the other hand, the toxicities of the radiation to normal tissues can cause gastrointestinal damage, lung fibrosis, and cognitive impairment in patients, preventing them from being given at high enough doses (Stone et al., 2003). Thus, how to improve the radiation sensitivity of tumors and protect normal tissues from radiation damage has drawn considerable attention (Gong et al., 2022a; Gong et al., 2022b). Therefore, highly selective and multifunctional radiosensitizers are urgently needed for radiotherapy enhancement. To address this issue, nanozymes that can target the tumor and improve hypoxia are promising strategies (Li et al., 2021; Zeng et al., 2021).
The tumor ablation effect of radiotherapy mainly relies on the radiation-induced DNA damage and toxicity of radiation-induced ROS. By coating pyrite (FeS2) with cancer cell-derived exosomes, a nanozyme system with homologous targeting abilities, GSH-OXD and POD-like properties was created. The dual enzyme activities of FeS2 reduce intracellular GSH levels and generate huge •OH to disrupt redox homeostasis and mitochondria integrity, thus significantly reducing radiotherapy resistance of cancer cells and amplifying the radiotherapeutic efficacy (Huang et al., 2021a).
GDY–CeO2 nanozyme are synthesized by 2D graphdiyne (GDY) anchoring to the Cerium oxide (CeO2) nanoparticles. The sustainable catalase activity of GDY–CeO2 can decompose H2O2 and elevate O2 concentration in TME, while high-Z element cerium in GDY–CeO2 act as a radiosensitizer to enhance the intracellular radiation energy deposition (Figure 5). Moreover, combining miR181a with the GDY–CeO2 further regulates the Serine/threonine-protein kinase Chk2 pathway and destroy the DNA repair system in cancer cells, strikingly reinforcing the effect of radiotherapy (Zhou et al., 2021a).
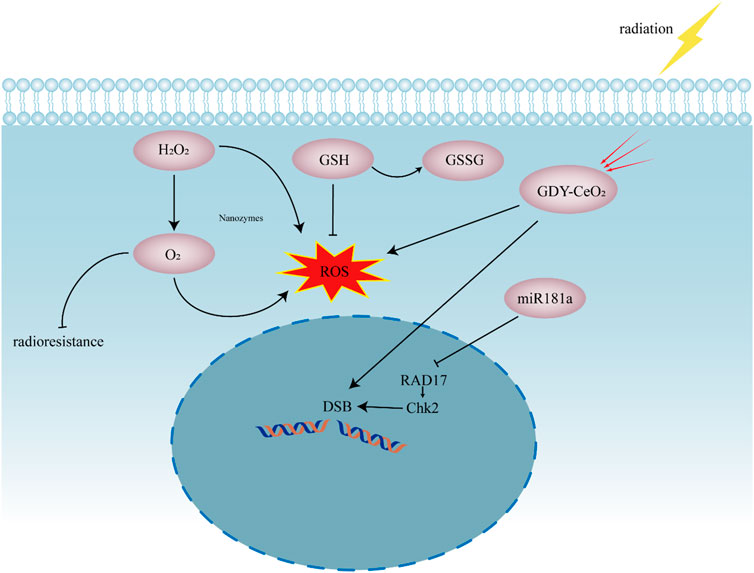
FIGURE 5. Schematic presentation showing the synergistic effect of nanozymes in radiotherapy. Nanozymes supply O2 to reduce radioresistance of tumor cells. The production of ROS enhances the effect of radiotherapy. GDY–CeO2 act as radiosensitizer to enhance the intracellular radiation energy deposition. miR181a can regulate RAD17/Chk2 pathway to suppress DNA repair. DSB, DNA double-strand break; GDY, 2D graphdiyne; CeO2, Cerium oxide, miR181a, miR181a-2-3p; RAD17, Cell cycle checkpoint protein RAD17; Chk2, Serine/threonine-protein kinase Chk2, ROS, reactive oxygen species; H2O2, hydrogen peroxide; O2, oxygen; GSH, glutathione; GSSG, glutathione disulfide.
A novel nanoprobes (Mn-doped Ag2Se QDs) are engineered by doping Mn (II) ions into Ag2Se quantum dot, using Mn (II) ions as CAT mimic to translate H2O2 into O2 and Ag2Se QDs as a radiosensitizer and NIR-II fluorescent materials to enhance radiation energy deposition and NIR-II imaging. By conjugating arginine-glycine-aspartate (RGD) tripeptides and polyethylene glycol (PEG) with Mn-doped Ag2Se QDs, Mn-doped Ag2Se-RGD-PEG nanoprobes were formed showing great biocompatibility and tumor specificity. The specific tumor-targeting and NIR-II-emitting abilities of the nanozymes enable precise NIR-II imaging-guided radiotherapy with negligible damage to normal tissues, which can be applied to boost the efficacy of RT (Wang et al., 2021).
Nanozymes in chemotherapy
Conventional tumor chemotherapy, which is most commonly applied as first-line therapy for cancer in the clinic, remains a formidable challenge due to the severe side effects and drug resistance (Alkhatib et al., 2022; Zhu et al., 2022a; Wang et al., 2022d; Kang et al., 2022). Through tremendous research by scientists, multifunctional nanozymes are proven to be a promising avenue to achieve higher tumor elimination efficacy in synergistic chemotherapy and catalytic therapy.
On the one hand, nanozymes have the advantage of their enzyme-mimic activities to catalase abundant ROS generation, which cause redox homeostasis disruption and damage to the cell membrane (Yuan et al., 2022). The direct effect of nanozymes can alleviate drug resistance and even kill cancer cells. On the other hand, nanozymes as nanoparticles can encapsulate chemotherapy drugs and release them in the tumor position (Ning et al., 2021). The specific targeting tumor property of nanozymes loaded drug system makes it possible to get better tumor elimination with a lower dose of chemotherapy drugs and less normal tissue injury (Han et al., 2022).
Zeolitic imidazolate framework (ZIF)-based nanoparticles co-loaded with glucose oxidase (GOX) and doxorubicin (DOX) were created to synergistically enhance chemotherapy. GOX activity of DOX/GOX-loaded ZIF (DGZ) effectively consumes glucose and generates adequate H2O2, restraining the mitochondrial energy metabolism and promoting ROS production. As degradation of DGZ, Zn2+ was released to break antioxidation homeostasis in cancer cells via inhibiting reductase systems such as glutathione reductase and thioredoxin reductase. In case of energy shortage and ROS accumulation, the therapeutic effects of DOX delivered by DGZ get greatly enhanced. More importantly, DGZ releases DOX drug only in the weak acid environment surrounding tumor rather than in normal tissue (Figure 6). This high degree of targeting makes it possible to use higher doses of chemotherapy drugs to get better therapeutic outcomes with fewer side effects (Huo et al., 2022).
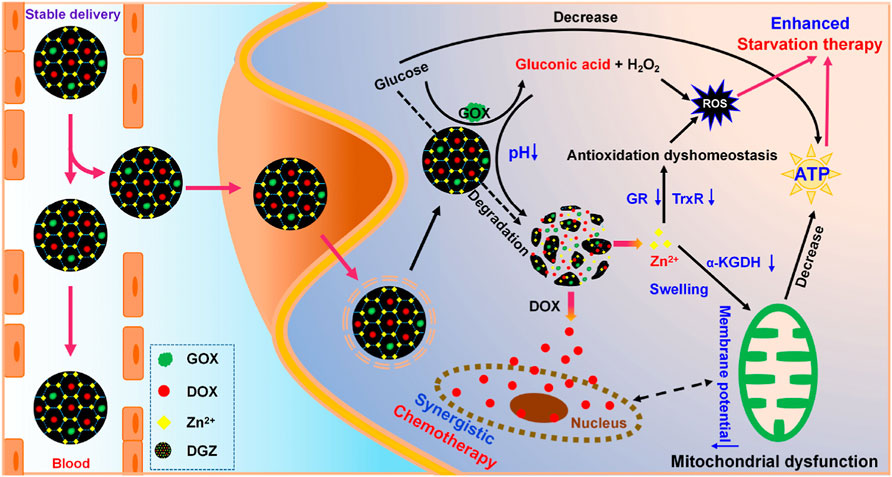
FIGURE 6. Schematic illustration of the synergistic effect of nanozymes in chemotherapy. Adapted with permission from (Huo et al., 2022). Copyright 2022 American Chemical Society.
The hypoxia-related drug resistance and the lack of potential uptake of drugs also hamper chemotherapy application in tumor treatment. Zheng et al. fabricated Fe3O4/Pt-FLU nanozymes by loading Pt nanozymes and 5-fluorouracil on the Fe3O4 nanospheres to achieve improvement in the treatment of breast cancer. The POD-liked and CAT-liked activities of Fe3O4/Pt-FLU alleviate the hypoxia environment in TME by increasing the level of ROS and O2, which leads to the reduction of drug resistance of cancerous tissue. Furthermore, Fe3O4/Pt-FLU specifically delivers 5-fluorouracil to the tumor site in a pH-sensitive drug release manner. The drug carrier role of Fe3O4/Pt-FLU significantly increases the stability of 5-fluorouracil and reduces the cytotoxicity in off-target tissues. The catalytic/chemotherapy double-modality therapy shows great therapeutic outcome for tumor elimination (Nie et al., 2022).
Nanozymes in sonodynamic therapy
Ultrasound (US)—triggered sonodynamic therapy (SDT) is mainly based on low-intensity US and sonosensitizers, which can induce the generation of cytotoxic ROS for tumor elimination (Chen et al., 2021a; Huang et al., 2021b). Compared with traditional therapy, SDT has the advantage of noninvasiveness, high tissue-penetrating capability, and safety, showing promising applications in new generation antitumor treatment (Pan et al., 2018; Son et al., 2020; Sun et al., 2021a). However, the ROS yield of sonosensitizers requires adequate O2 assistance which is strongly hampered by hypoxia of TME. Thus, synergistic therapy with nanozyme-based O2 supply and SDT may be a practical strategy (Gong and Dai, 2021).
AIMP, a multifunctional nanozyme, is formed by linking Angiopep-2 (Ang) with PLGA, in which IR780 and MnO2 are encapsulated. Through the function of Ang and IR780, AIMP can target cancer cells and remain inside the mitochondria. Upon low-intensity US irradiation, IR780 behave as sonosensitizer to produce ROS and cause damage to cancer cells. On the other hand, enzyme-like activity of MnO2 is responsible for O2 release and GSH depletion, thereby boosting the production of ROS and enhancing SDT effect (Liu et al., 2021b).
The Pt-CuS Janus loaded with tetra-(4-aminophenyl) porphyrin (TAPP) as a sonosensitizer is designed to facilitate SDT-based synergistic therapeutic modality. Upon exposure to US, TAPP are released from the hollow Pt-CuS Janus and interact with O2 to produce 1O2. Meanwhile, Pt possesses CAT-like activity for the degradation of H2O2 and production of O2, which provides an O2 source for SDT-induced ROS production (Figure 7). Importantly, the photothermal effect of Pt-CuS elevates the Pt catalytic activity for O2 generation and facilitates SDT efficacy (Liang et al., 2019).
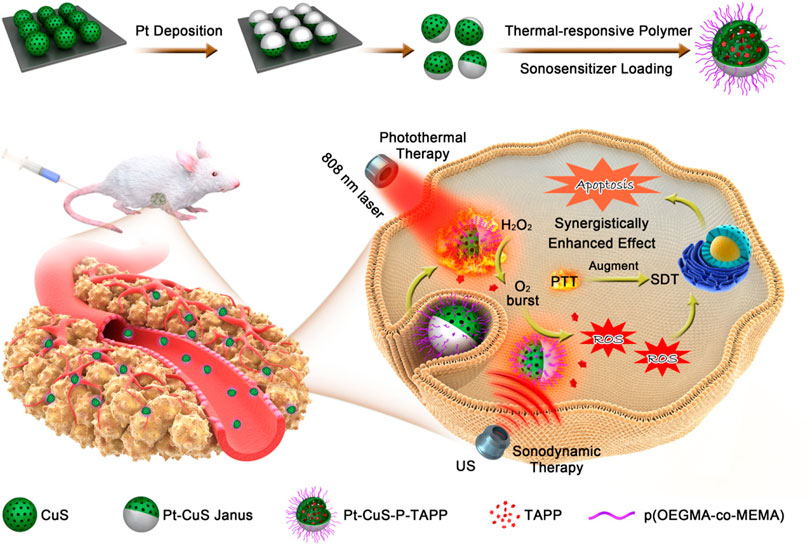
FIGURE 7. Schematic illustration of the synergistic effect of nanozymes in sonodynamic therapy. Adapted with permission from (Liang et al., 2019). Copyright 2019 American Chemical Society.
Nanozymes in chemodynamic therapy
Chemodynamic therapy (CDT) is an emerging therapeutic strategy that can destroy cancer cells through catalyzing the endogenous H2O2 into lethal hydroxyl radical by Fenton reaction or Fenton-like reaction (Zhou et al., 2021b; Jia et al., 2022). CDT takes advantage of high selectivity, low side effects and no requirement for exogenous stimulus. However, the limited concentration of endogenous H2O2 and excessive antioxidant GSH hinder the application of CDT. Supplying sufficient intratumoral H2O2 and depleting endogenous GSH are necessary to improve the efficiency of CDT. Recently nanozyme cascade platforms have been extensively studied and wildly applied to enhance the antitumor effect of chemodynamic therapy (Zhang et al., 2021; Zhang et al., 2022e).
The cascade reaction systems ACD is fabricated by loading copper ion-doped ZIF-8 with ZIF-8 doped with Au nanozymes and doxorubicin hydrochloride (Zhao et al., 2021a). The POD-like activity of Au nanozymes can catalyze H2O2 to ·OH for CDT. While Au can also serve as an oxidase to deplete the GSH. Moreover, the Cu2+ ions released from ACD could consume the GSH via redox reactions and the generated Cu+ can produce ·OH by Fenton-like reaction. Thus, the increased ROS and exhaustion of GSH can effectively amplify the antitumor effect of CDT. On the other hand, doxorubicin hydrochloride not only act as chemotherapeutic drug to kill cancer cells but also supply sufficient H2O2 to boost the effect of CDT (Figure 8). Herein, the application of nanozymes in CDT can achieve better treatment effects and are promising for tumor elimination.
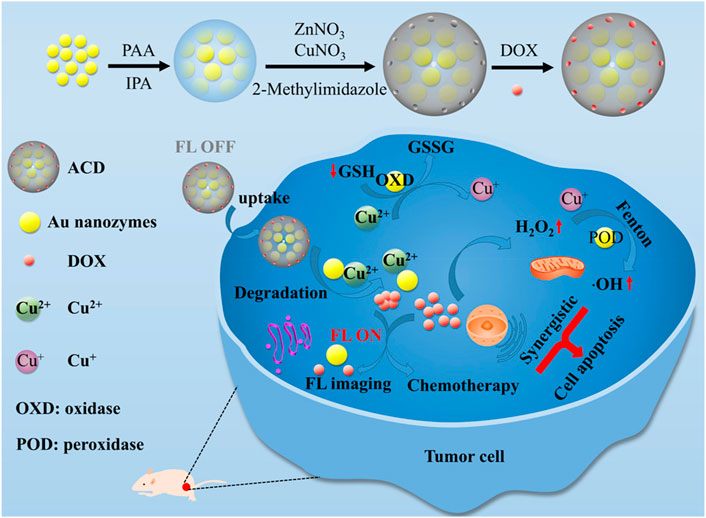
FIGURE 8. Schematic illustration of the synergistic effect of nanozymes in chemodynamic therapy. Adapted with permission from (Zhao et al., 2021a). Copyright 2021 American Chemical Society.
Apart from increasing ROS production and promoting GSH depletion, strengthening the tumor targeting ability of nanodrug is another way to augment the effect of CDT. Au-DNA-Fe, a microRNA-triggered nanozyme cascade platform is fabricated for enhanced tumor-specific chemodynamic therapy. Au nanozyme as glucose oxidase-like nanozyme catalyze Glucose into gluconic acid and H2O2, while the produced H2O2 is subsequently catalyzed by Fe3O4 nanoparticles to generate hydroxyl radicals for CDT. Interestingly, Au and Fe3O4 nanoparticles are modified by two different single-stranded DNA which are complementary to each part of miRNA-21. Due to the overexpression of miRNA-21 in cancer cells, the nanozyme Au-DNA-Fe can trigger the cascade reaction specifically in cancer cells with negligible side effects on normal tissues (Liu et al., 2021a).
Conclusion and perspectives
Nanozyme is a breakthrough in the treatment of cancer, and their extremely small size, good modifiability and applicability provide endless opportunities to design multi-program cancer therapy strategies. A lot of research has generated a large number of feasible solutions for nanozymes involved in cancer treatment, mainly via inducing oxidative stress by catalyzing ROS production and disrupting the function of tumor cells by altering the metabolism. The imbalance in redox homeostasis caused by excessive ROS induces apoptosis or ferroptosis, which both result in tumor death. In addition to the direct killing of tumor cells, modification of the tumor microenvironment by nanozymes effectively reduces the resistance of tumor cells to therapy, including drug resistance, radiation desensitization, or immune evasion. This creates an amazing synergy when used in conjunction with other classical therapies such as phototherapy, immunotherapy, radiotherapy, and chemotherapy.
Despite the promising future, many hurdles remain to be overcome in the application of nanozymes in cancer therapy. Oxidases, catalase and hydrolases already exist in numerous applications in the treatment of cancer, but the study of other enzymes such as transferases and lysates are new direction and relatively empty areas of research. Exploration of novel enzymatic nanoparticles can help us establish a multi-enzyme nanoparticle therapeutic system. Due to their diverse catalytic activities, different enzyme types can synergistically address the difficulties that exist in aberrant tumor environments and play a more effective role in tumors.
On the other hand, the catalytic activity of nanozymes needs to be improved. Although there is a small subset of nanozymes with greater catalytic activity than natural enzymes. However, achieving optimal catalytic efficiency in a complex tumor microenvironment remains a challenge. Most importantly, good biocompatibility is a pre-requisite for nanozymes to be used in the clinic. There is an urgent demand to reduce nanozymes inherent toxicity or design nontoxic nanozymes, for which ensuring that nanozymes remain effective at low doses is a viable method. Thus, it is the key to improving the targeting ability of nanozymes, as well as the ability to deliver them into the bloodstream in a sustained manner. The construction of a nanozymes-system with good biocompatibility and high target efficiency will be future development trend in new generation cancer therapy.
As nanotechnology continues to develop and cancer treatment improves, nanozymes with high catalytic efficiencies, excellent biocompatibility, specificity, and multiple functionalities in combination with traditional anticancer therapy are expected to emerge in the future.
Author contributions
YY performed a literature search, interpreted the data, and wrote the manuscript. RL, XY, and WZ supervised and contributed to the writing process. All authors contributed to the article and approved the submitted version.
Funding
This work was supported by the National Natural Science Foundation of China (Grant No. 82130092).
Conflict of interest
The authors declare that the research was conducted in the absence of any commercial or financial relationships that could be construed as a potential conflict of interest.
Publisher’s note
All claims expressed in this article are solely those of the authors and do not necessarily represent those of their affiliated organizations, or those of the publisher, the editors and the reviewers. Any product that may be evaluated in this article, or claim that may be made by its manufacturer, is not guaranteed or endorsed by the publisher.
References
Alkhatib, H., Rubinstein, A. M., Vasudevan, S., Flashner-Abramson, E., Stefansky, S., Chowdhury, S. R., et al. (2022). Computational quantification and characterization of independently evolving cellular subpopulations within tumors is critical to inhibit anti-cancer therapy resistance. Genome Med. 14, 120. doi:10.1186/s13073-022-01121-y
Bray, F., Laversanne, M., Weiderpass, E., and Soerjomataram, I. (2021). The ever-increasing importance of cancer as A leading cause of premature death worldwide. Cancer 127, 3029–3030. doi:10.1002/cncr.33587
Brizel, D. M., Dodge, R. K., Clough, R. W., and Dewhirst, M. W. (1999). Oxygenation of head and neck cancer: Changes during radiotherapy and impact on treatment outcome. Radiother. Oncol. 53, 113–117. doi:10.1016/s0167-8140(99)00102-4
Brown, J. M., and Wilson, W. R. (2004). Exploiting tumour hypoxia in cancer treatment. Nat. Rev. Cancer 4, 437–447. doi:10.1038/nrc1367
Chai, Z., Childress, A., and Busnaina, A. A. (2022). Directed assembly of nanomaterials for making nanoscale devices and structures: Mechanisms and applications. Acs Nano. doi:10.1021/acsnano.2c07910
Chaudhry, G. E., Md Akim, A., Sung, Y. Y., and Sifzizul, T. M. T. (2022). Cancer and apoptosis: The apoptotic activity of plant and marine natural products and their potential as targeted cancer therapeutics. Front. Pharmacol. 13, 842376. doi:10.3389/fphar.2022.842376
Chen, H., Liu, L., Ma, A., Yin, T., Chen, Z., Liang, R., et al. (2021a). Noninvasively immunogenic sonodynamic therapy with manganese protoporphyrin liposomes against triple-negative breast cancer. Biomaterials 269, 120639. doi:10.1016/j.biomaterials.2020.120639
Chen, H., Ye, Y., Yang, Y., Zhong, M., Gu, L., Han, Z., et al. (2020). Tipe-mediated up-regulation of mmp-9 promotes colorectal cancer invasion and metastasis through mkk-3/P38/nf-?b pro-oncogenic signaling pathway. Signal Transduct. Target. Ther. 5, 163. doi:10.1038/s41392-020-00276-7
Chen, X., Kang, R., Kroemer, G., and Tang, D. (2021b). Broadening horizons: The role of ferroptosis in cancer. Nat. Rev. Clin. Oncol. 18, 280–296. doi:10.1038/s41571-020-00462-0
Chen, Y., Yin, C., Zhang, Y., Liu, Y., Zan, J., Xie, C., et al. (2021c). Dual lock-and-key"-controlled ceria nanotubes-based nanozymes for tumor-specific photothermal therapy. Dyes And Pigments 191, 109350. doi:10.1016/j.dyepig.2021.109350
Chu, Q., Zhu, H., Liu, B., Cao, G., Fang, C., Wu, Y., et al. (2020). Delivery of amino acid oxidase via catalytic nanocapsules to enable effective tumor inhibition. J. Mat. Chem. B 8, 8546–8557. doi:10.1039/d0tb01425g
Dai, Q., Wang, L., Ren, E., Chen, H., Gao, X., Cheng, H., et al. (2022). Ruthenium-based metal–organic nanoradiosensitizers enhance radiotherapy by combining ROS generation and CO gas release. Angew. Chem. Int. Ed. Engl., e202211674. doi:10.1002/anie.202211674
Fan, F., Liu, P., Bao, R., Chen, J., Zhou, M., Mo, Z., et al. (2021). A dual pi3k/hdac inhibitor induces immunogenic ferroptosis to potentiate cancer immune checkpoint therapy. Cancer Res. 81, 6233–6245. doi:10.1158/0008-5472.can-21-1547
Farheen, J., Hosmane, N. S., Zhao, R., Zhao, Q., Iqbal, M. Z., and Kong, X. (2022). Nanomaterial-assisted crispr gene-engineering - a hallmark for triple-negative breast cancer therapeutics advancement. Mat. Today Bio 16, 100450. doi:10.1016/j.mtbio.2022.100450
Feng, N., Li, Q., Bai, Q., Xu, S., Shi, J., Liu, B., et al. (2022). Development of an Au-anchored Fe single-atom nanozyme for biocatalysis and enhanced tumor photothermal therapy. J. Colloid Interface Sci. 618, 68–77. doi:10.1016/j.jcis.2022.03.031
Filik, H., Avan, A. A., and Tokatlı, Z. F. (2021). A review on colorimetric sensing of tumor markers based on enzyme-mimicking nanomaterials. Curr. Med. Chem. 28, 6123–6145. doi:10.2174/0929867328666210412122604
Friedmann Angeli, J. P., Krysko, D. V., and Conrad, M. (2019). Ferroptosis at the crossroads of cancer-acquired drug resistance and immune evasion. Nat. Rev. Cancer 19, 405–414. doi:10.1038/s41568-019-0149-1
Gacche, R. N., and Assaraf, Y. G. (2018). Redundant angiogenic signaling and tumor drug resistance. Drug resist. updat. 36, 47–76. doi:10.1016/j.drup.2018.01.002
Gong, L., Zhang, Y., Zhao, J., Zhang, Y., Tu, K., Jiao, L., et al. (2022a). All-in-one biomimetic nanoplatform based on hollow polydopamine nanoparticles for synergistically enhanced radiotherapy of colon cancer. Small 18, E2205198. doi:10.1002/smll.202205198
Gong, X., Li, J., Xu, X., Wu, Y., Lei, Y., Liu, H., et al. (2022b). Microvesicle-inspired oxygen-delivering nanosystem potentiates radiotherapy-mediated modulation of tumor stroma and antitumor immunity. Biomaterials 290, 121855. doi:10.1016/j.biomaterials.2022.121855
Gong, Z., and Dai, Z. (2021). Design and challenges of sonodynamic therapy system for cancer theranostics: From equipment to sensitizers. Adv. Sci. (Weinh). 8, 2002178. doi:10.1002/advs.202002178
Han, Z. Y., Chen, Q. W., Fu, Z. J., Cheng, S. X., and Zhang, X. Z. (2022). Probiotic spore-based oral drug delivery system for enhancing pancreatic cancer chemotherapy by gut-pancreas-axis-guided delivery. Nano Lett. 22, 8608–8617. doi:10.1021/acs.nanolett.2c03131
Hanahan, D., and Weinberg, R. A. (2011). Hallmarks of cancer: The next generation. Cell 144, 646–674. doi:10.1016/j.cell.2011.02.013
He, X., and Xu, C. (2020). Immune checkpoint signaling and cancer immunotherapy. Cell Res. 30, 660–669. doi:10.1038/s41422-020-0343-4
Huang, C., Liu, Z., Chen, M., Du, L., Liu, C., Wang, S., et al. (2021a). Tumor-derived biomimetic nanozyme with immune evasion ability for synergistically enhanced low dose radiotherapy. J. Nanobiotechnology 19, 457. doi:10.1186/s12951-021-01182-y
Huang, J., Xiao, Z., An, Y., Han, S., Wu, W., Wang, Y., et al. (2021b). Nanodrug with dual-sensitivity to tumor microenvironment for immuno-sonodynamic anti-cancer therapy. Biomaterials 269, 120636. doi:10.1016/j.biomaterials.2020.120636
Huo, T., Chen, L., Nie, H., Li, W., Lin, C., Akhtar, M., et al. (2022). Mitochondrial dysfunction and antioxidation dyshomeostasis-enhanced tumor starvation synergistic chemotherapy achieved using A metal-organic framework-based nano-enzyme reactor. ACS Appl. Mat. Interfaces 14, 3675–3684. doi:10.1021/acsami.1c18654
Jia, C., Guo, Y., and Wu, F. G. (2022). Chemodynamic therapy via Fenton and fenton-like nanomaterials: Strategies and recent advances. Small 18, E2103868. doi:10.1002/smll.202103868
Jiang, D., Ni, D., Rosenkrans, Z. T., Huang, P., Yan, X., and Cai, W. (2019). Nanozyme: New horizons for responsive biomedical applications. Chem. Soc. Rev. 48, 3683–3704. doi:10.1039/c8cs00718g
Kang, X., Wang, J., Huang, C. H., Wibowo, F. S., Amin, R., Chen, P., et al. (2022). Diethyldithiocarbamate copper nanoparticle overcomes resistance in cancer therapy without inhibiting P-glycoprotein. Nanomedicine Nanotechnol. Biol. Med. 47, 102620. doi:10.1016/j.nano.2022.102620
Kim, S., Palani, S., Civitci, F., Nan, X., and Ibsen, S. (2022). A versatile synthetic pathway for producing mesostructured plasmonic nanostructures. Small 21, E2203940. doi:10.1002/smll.202203940
Kopecka, J., Salaroglio, I. C., Perez-Ruiz, E., Sarmento-Ribeiro, A. B., Saponara, S., De Las Rivas, J., et al. (2021). Hypoxia as A driver of resistance to immunotherapy. Drug resist. updat. 59, 100787. doi:10.1016/j.drup.2021.100787
Li, H., Sun, X., Li, J., Liu, W., Pan, G., Mao, A., et al. (2022a). Hypoxia induces docetaxel resistance in triple-negative breast cancer via the hif-1α/mir-494/survivin signaling pathway. Neoplasia 32, 100821. doi:10.1016/j.neo.2022.100821
Li, S., Sun, W., Luo, Y., Gao, Y., Jiang, X., Yuan, C., et al. (2021). Hollow ptco alloy nanospheres as A high-Z and oxygen generating nanozyme for radiotherapy enhancement in non-small cell lung cancer. J. Mat. Chem. B 9, 4643–4653. doi:10.1039/d1tb00486g
Li, Y., Deng, G., Hu, X., Li, C., Wang, X., Zhu, Q., et al. (2022b). Recent advances in mesoporous silica nanoparticle-based targeted drug-delivery systems for cancer therapy. Nanomedicine (Lond) 17 (18), 1253–1279. doi:10.2217/nnm-2022-0023
Li, Z., Cheng, G., Zhang, Q., Wu, W., Zhang, Y., Wu, B., et al. (2022c). Px478-Loaded silk fibroin nanoparticles reverse multidrug resistance by inhibiting the hypoxia-inducible factor. Int. J. Biol. Macromol. 222, 2309–2317. doi:10.1016/j.ijbiomac.2022.10.018
Liang, M., and Yan, X. (2019). Nanozymes: From new concepts, mechanisms, and standards to applications. Acc. Chem. Res. 52, 2190–2200. doi:10.1021/acs.accounts.9b00140
Liang, S., Deng, X., Chang, Y., Sun, C., Shao, S., Xie, Z., et al. (2019). Intelligent hollow Pt-cus Janus architecture for synergistic catalysis-enhanced sonodynamic and photothermal cancer therapy. Nano Lett. 19, 4134–4145. doi:10.1021/acs.nanolett.9b01595
Liu, H., You, Y., Sang, Y., Pu, F., Ren, J., and Qu, X. (2021a). Microrna-triggered nanozymes cascade reaction for tumor-specific chemodynamic therapy. Chem. A Eur. J. 27, 18201–18207. doi:10.1002/chem.202103547
Liu, S., Zhang, W., Chen, Q., Hou, J., Wang, J., Zhong, Y., et al. (2021b). Multifunctional nanozyme for multimodal imaging-guided enhanced sonodynamic therapy by regulating the tumor microenvironment. Nanoscale 13, 14049–14066. doi:10.1039/d1nr01449h
Ma, B., Han, J., Zhang, K., Jiang, Q., Sui, Z., Zhang, Z., et al. (2022). Targeted killing of tumor cells based on isoelectric point suitable nanoceria-rod with high oxygen vacancies. J. Mat. Chem. B 10, 1410–1417. doi:10.1039/d1tb02787e
Meng, X., Li, D., Chen, L., He, H., Wang, Q., Hong, C., et al. (2021). High-performance self-cascade pyrite nanozymes for apoptosis-ferroptosis synergistic tumor therapy. Acs Nano 15, 5735–5751. doi:10.1021/acsnano.1c01248
Michaud, V., Lasseaux, E., Green, D. J., Gerrard, D. T., Plaisant, C., Fitzgerald, T., et al. (2022). The contribution of common regulatory and protein-coding tyr variants to the genetic architecture of albinism. Nat. Commun. 13, 3939. doi:10.1038/s41467-022-31392-3
Mou, Y., Wang, J., Wu, J., He, D., Zhang, C., Duan, C., et al. (2019). Ferroptosis, A new form of cell death: Opportunities and challenges in cancer. J. Hematol. Oncol. 12, 34. doi:10.1186/s13045-019-0720-y
Nie, Z., Vahdani, Y., Cho, W. C., Bloukh, S. H., Edis, Z., Haghighat, S., et al. (2022). 5-Fluorouracil-Containing inorganic iron oxide/platinum nanozymes with dual drug delivery and enzyme-like activity for the treatment of breast cancer. Arabian J. Chem. 15, 103966. doi:10.1016/j.arabjc.2022.103966
Ning, S., Zheng, Y., Qiao, K., Li, G., Bai, Q., and Xu, S. (2021). Laser-triggered combination therapy by iron sulfide-Doxorubicin@Functionalized nanozymes for breast cancer therapy. J. Nanobiotechnology 19, 344. doi:10.1186/s12951-021-01023-y
Pan, X., Wang, H., Wang, S., Sun, X., Wang, L., Wang, W., et al. (2018). Sonodynamic therapy (sdt): A novel strategy for cancer nanotheranostics. Sci. China Life Sci. 61, 415–426. doi:10.1007/s11427-017-9262-x
Robert, A., and Meunier, B. (2022). How to define A nanozyme. Acs Nano 16, 6956–6959. doi:10.1021/acsnano.2c02966
Roos, W. P., Thomas, A. D., and Kaina, B. (2016). Dna damage and the balance between survival and death in cancer biology. Nat. Rev. Cancer 16, 20–33. doi:10.1038/nrc.2015.2
Rudd, C. E. (2019). Advances in T-cell Co-receptor biology and cancer immunotherapy. Semin. Immunol. 42, 101281. doi:10.1016/j.smim.2019.101281
Scott, J. A., Maarsingh, H., Holguin, F., and Grasemann, H. (2021). Arginine therapy for lung diseases. Front. Pharmacol. 12, 627503. doi:10.3389/fphar.2021.627503
Semenza, G. L. (2000). Hypoxia, clonal selection, and the role of hif-1 in tumor progression. Crit. Rev. Biochem. Mol. Biol. 35, 71–103. doi:10.1080/10409230091169186
Shen, W., Han, G., Yu, L., Yang, S., Li, X., Zhang, W., et al. (2022). Combined prussian blue nanozyme carriers improve photodynamic therapy and effective interruption of tumor metastasis. Int. J. Nanomedicine 17, 1397–1408. doi:10.2147/ijn.s359156
Shi, M., Cao, M., Song, J., Liu, Q., Li, H., Meng, F., et al. (2015). Pinx1 inhibits the invasion and metastasis of human breast cancer via suppressing nf-?b/mmp-9 signaling pathway. Mol. Cancer 14, 66. doi:10.1186/s12943-015-0332-2
Son, S., Kim, J. H., Wang, X., Zhang, C., Yoon, S. A., Shin, J., et al. (2020). Multifunctional sonosensitizers in sonodynamic cancer therapy. Chem. Soc. Rev. 49, 3244–3261. doi:10.1039/c9cs00648f
Song, L., Chen, B., Qin, Z., Liu, X., Guo, Z., Lou, H., et al. (2022). Temperature-dependent cat-like rgd-Bpns@Smfn nanoplatform for ptt-pdt self-synergetic tumor phototherapy. Adv. Healthc. Mat. 11, E2102298. doi:10.1002/adhm.202102298
Song, Y., Zhang, Y., Chen, M., Deng, J., Sui, T., Lai, L., et al. (2018). Functional validation of the albinism-associated tyrosinase T373k snp by crispr/cas9-mediated homology-directed repair (hdr) in rabbits. Ebiomedicine 36, 517–525. doi:10.1016/j.ebiom.2018.09.041
Stone, H. B., Coleman, C. N., Anscher, M. S., and Mcbride, W. H. (2003). Effects of radiation on normal tissue: Consequences and mechanisms. Lancet Oncol. 4, 529–536. doi:10.1016/s1470-2045(03)01191-4
Sun, L., Wang, X., Gong, F., Yin, K., Zhu, W., Yang, N., et al. (2021a). Silicon nanowires decorated with platinum nanoparticles were applied for photothermal-enhanced sonodynamic therapy. Theranostics 11, 9234–9242. doi:10.7150/thno.58755
Sun, W., Yu, H., Wang, D., Li, Y., Tian, B., Zhu, S., et al. (2021b). Mno(2) nanoflowers as A multifunctional nano-platform for enhanced photothermal/photodynamic therapy and mr imaging. Biomater. Sci. 9, 3662–3674. doi:10.1039/d1bm00033k
Sung, H., Ferlay, J., Siegel, R. L., Laversanne, M., Soerjomataram, I., Jemal, A., et al. (2021). Global cancer statistics 2020: Globocan estimates of incidence and mortality worldwide for 36 cancers in 185 countries. Ca. A Cancer J. Clin. 71, 209–249. doi:10.3322/caac.21660
Tang, D., Chen, X., Kang, R., and Kroemer, G. (2021). Ferroptosis: Molecular mechanisms and health implications. Cell Res. 31, 107–125. doi:10.1038/s41422-020-00441-1
Tang, M., Zhang, Z., Ding, C., Li, J., Shi, Y., Sun, T., et al. (2022). Two birds with one stone: Innovative ceria-loaded Gold@Platinum nanospheres for photothermal-catalytic therapy of tumors. J. Colloid Interface Sci. 627, 299–307. doi:10.1016/j.jcis.2022.07.065
Vieyra-Garcia, P. A., and Wolf, P. (2021). A deep dive into uv-based phototherapy: Mechanisms of action and emerging molecular targets in inflammation and cancer. Pharmacol. Ther. 222, 107784. doi:10.1016/j.pharmthera.2020.107784
Wang, H., Qu, R., Chen, Q., Zhang, T., Chen, X., Wu, B., et al. (2022a). Pegylated prussian blue nanoparticles for modulating polyethyleneimine cytotoxicity and attenuating tumor hypoxia for dual-enhanced photodynamic therapy. J. Mat. Chem. B 10, 5410–5421. doi:10.1039/d2tb00571a
Wang, H., Wan, K., and Shi, X. (2019a). Recent advances in nanozyme research. Adv. Mat. 31, E1805368. doi:10.1002/adma.201805368
Wang, J., Fang, L., Li, P., Ma, L., Na, W., Cheng, C., et al. (2019b). Inorganic nanozyme with combined self-oxygenation/degradable capabilities for sensitized cancer immunochemotherapy. Nanomicro. Lett. 11, 74. doi:10.1007/s40820-019-0305-x
Wang, M., Li, H., Huang, B., Chen, S., Cui, R., Sun, Z. J., et al. (2021). An ultra-stable, oxygen-supply nanoprobe emitting in near-infrared-ii window to guide and enhance radiotherapy by promoting anti-tumor immunity. Adv. Healthc. Mat. 10, E2100090. doi:10.1002/adhm.202100090
Wang, S., Wang, Z., Li, Z., Zhang, X., Zhang, H., Zhang, T., et al. (2022b). Amelioration of systemic antitumor immune responses in cocktail therapy by immunomodulatory nanozymes. Sci. Adv. 8, eabn3883. doi:10.1126/sciadv.abn3883
Wang, T., Gao, Z., Zhang, Y., Hong, Y., Tang, Y., Shan, K., et al. (2022c). A supramolecular self-assembled nanomaterial for synergistic therapy of immunosuppressive tumor. J. Control. Release 351, 272–283. doi:10.1016/j.jconrel.2022.09.018
Wang, Y. M., Zhao, Q. W., Sun, Z. Y., Lin, H. P., Xu, X., Cao, M., et al. (2022d). Circular rna Hsa_Circ_0003823 promotes the tumor progression, metastasis and apatinib resistance of esophageal squamous cell carcinoma by mir-607/crisp3 Axis. Int. J. Biol. Sci. 18, 5787–5808. doi:10.7150/ijbs.76096
Wang, Z., Mu, X., Yang, Q., Luo, J., and Zhao, Y. (2022e). Hypoxia-responsive nanocarriers for chemotherapy sensitization via dual-mode inhibition of hypoxia-inducible factor-1 alpha. J. Colloid Interface Sci. 628, 106–115. doi:10.1016/j.jcis.2022.08.060
Wang, Z., Xu, Z., Xu, X., Xi, J., Han, J., Fan, L., et al. (2022f). Construction of core-in-shell Au@N-hcns nanozymes for tumor therapy. Colloids And Surfaces B-Biointerfaces 217, 112671. doi:10.1016/j.colsurfb.2022.112671
Wei, S., Qiu, T., Yao, X., Wang, N., Jiang, L., Jia, X., et al. (2020). Arsenic induces pancreatic dysfunction and ferroptosis via mitochondrial ros-autophagy-lysosomal pathway. J. Hazard. Mat. 384, 121390. doi:10.1016/j.jhazmat.2019.121390
Xi, J., Huang, Y., Chen, J., Zhang, J., Gao, L., Fan, L., et al. (2021). Artesunate-loaded poly (lactic-Co-glycolic acid)/polydopamine-manganese oxides nanoparticles as an oxidase mimic for tumor chemo-catalytic therapy. Int. J. Biol. Macromol. 181, 72–81. doi:10.1016/j.ijbiomac.2021.03.124
Xing, L., Liu, X. Y., Zhou, T. J., Wan, X., Wang, Y., and Jiang, H. L. (2021). Photothermal nanozyme-ignited Fenton reaction-independent ferroptosis for breast cancer therapy. J. Control. Release 339, 14–26. doi:10.1016/j.jconrel.2021.09.019
Yang, J., Ma, S., Xu, R., Wei, Y., Zhang, J., Zuo, T., et al. (2021). Smart biomimetic metal organic frameworks based on ros-ferroptosis-glycolysis regulation for enhanced tumor chemo-immunotherapy. J. Control. Release 334, 21–33. doi:10.1016/j.jconrel.2021.04.013
Yang, L., Ren, C., Xu, M., Song, Y., Lu, Q., Wang, Y., et al. (2020). Rod-shape inorganic biomimetic mutual-reinforcing Mno2-Au nanozymes for catalysis-enhanced hypoxic tumor therapy. Nano Res. 13, 2246–2258. doi:10.1007/s12274-020-2844-3
Yang, Y., Huang, Q., Xiao, Z., Liu, M., Zhu, Y., Chen, Q., et al. (2022). Nanomaterial-based biosensor developing as A route toward in vitro diagnosis of early ovarian cancer. Mat. Today Bio 13, 100218. doi:10.1016/j.mtbio.2022.100218
Yao, L., Zhao, M. M., Luo, Q. W., Zhang, Y. C., Liu, T. T., Yang, Z., et al. (2022a). Carbon quantum dots-based nanozyme from coffee induces cancer cell ferroptosis to activate antitumor immunity. Acs Nano 16, 9228–9239. doi:10.1021/acsnano.2c01619
Yao, Y., Wang, Z., Cao, Q., Li, H., Ge, S., Liu, J., et al. (2022b). Degradable tumor-responsive iron-doped phosphate-based glass nanozyme for H(2)O(2) self-supplying cancer therapy. ACS Appl. Mat. Interfaces 14, 17153–17163. doi:10.1021/acsami.2c02669
Ye, L. F., Chaudhary, K. R., Zandkarimi, F., Harken, A. D., Kinslow, C. J., Upadhyayula, P. S., et al. (2020). Radiation-induced lipid peroxidation triggers ferroptosis and synergizes with ferroptosis inducers. ACS Chem. Biol. 15, 469–484. doi:10.1021/acschembio.9b00939
Yu, H., Cheng, Y., Wen, C., Sun, Y. Q., and Yin, X. B. (2022). Triple cascade nanocatalyst with laser-activatable O(2) supply and photothermal enhancement for effective catalytic therapy against hypoxic tumor. Biomaterials 280, 121308. doi:10.1016/j.biomaterials.2021.121308
Yuan, Z., Liu, X., Ling, J., Huang, G., Huang, J., Zhu, X., et al. (2022). In situ-transition nanozyme triggered by tumor microenvironment boosts synergistic cancer radio-/chemotherapy through disrupting redox homeostasis. Biomaterials 287, 121620. doi:10.1016/j.biomaterials.2022.121620
Zeng, J., Wang, Y., Wu, J., Wang, H., Lu, J., Li, S., et al. (2022a). Nanozymes of Ni0.5fe0.5s2 mediated synergetic antitumor treatment. Chemnanomat 8. doi:10.1002/cnma.202200090
Zeng, W., Liu, C., Wang, S., Wang, Z., and Huang, Q. (2021). Snfe(2)O(4) nanozyme based tme improvement system for anti-cancer combination thermoradiotherapy. Front. Oncol. 11, 768829. doi:10.3389/fonc.2021.768829
Zeng, W., Yu, M., Chen, T., Liu, Y., Yi, Y., Huang, C., et al. (2022b). Polypyrrole nanoenzymes as tumor microenvironment modulators to reprogram macrophage and potentiate immunotherapy. Adv. Sci. (Weinh). 9, E2201703. doi:10.1002/advs.202201703
Zhang, C., Chen, L., Bai, Q., Wang, L., Li, S., Sui, N., et al. (2022a). Nonmetal graphdiyne nanozyme-based ferroptosis-apoptosis strategy for colon cancer therapy. ACS Appl. Mat. Interfaces 14, 27720–27732. doi:10.1021/acsami.2c06721
Zhang, G., Li, N., Qi, Y., Zhao, Q., Zhan, J., and Yu, D. (2022b). Synergistic ferroptosis-gemcitabine chemotherapy of the gemcitabine loaded carbonaceous nanozymes to enhance the treatment and magnetic resonance imaging monitoring of pancreatic cancer. Acta Biomater. 142, 284–297. doi:10.1016/j.actbio.2022.02.006
Zhang, G., Tao, X., Ji, B., and Gong, J. (2022c). Hypoxia-driven M2-polarized macrophages facilitate cancer aggressiveness and temozolomide resistance in glioblastoma. Oxid. Med. Cell. Longev. 2022, 1–20. doi:10.1155/2022/1614336
Zhang, H., Zhao, L., Jiang, J., Zheng, J., Yang, L., Li, Y., et al. (2022d). Multiplexed nanomaterial-assisted laser desorption/ionization for pan-cancer diagnosis and classification. Nat. Commun. 13, 617. doi:10.1038/s41467-021-26642-9
Zhang, L., Li, C. X., Wan, S. S., and Zhang, X. Z. (2022e). Nanocatalyst-mediated chemodynamic tumor therapy. Adv. Healthc. Mat. 11, E2101971. doi:10.1002/adhm.202101971
Zhang, R., Liu, C., Zhao, R., Du, Y., Yang, D., Ding, H., et al. (2022f). Engineering oxygen vacancy of moo(X) nanoenzyme by Mn doping for dual-route cascaded catalysis mediated high tumor eradication. J. Colloid Interface Sci. 623, 155–167. doi:10.1016/j.jcis.2022.05.037
Zhang, W., Liu, J., Li, X., Zheng, Y., Chen, L., Wang, D., et al. (2021). Precise chemodynamic therapy of cancer by trifunctional bacterium-based nanozymes. Acs Nano 15, 19321–19333. doi:10.1021/acsnano.1c05605
Zhao, D. H., Li, C. Q., Hou, X. L., Xie, X. T., Zhang, B., Wu, G. Y., et al. (2021a). Tumor microenvironment-activated theranostics nanozymes for fluorescence imaging and enhanced chemo-chemodynamic therapy of tumors. ACS Appl. Mat. Interfaces 13, 55780–55789. doi:10.1021/acsami.1c12611
Zhao, X., Wu, J., Zhang, K., Guo, D., Hong, L., Chen, X., et al. (2021b). The synthesis of A nanodrug using metal-based nanozymes conjugated with ginsenoside Rg3 for pancreatic cancer therapy. Nanoscale Adv. 4, 190–199. doi:10.1039/d1na00697e
Zheng, N., Fu, Y., Liu, X., Zhang, Z., Wang, J., Mei, Q., et al. (2022). Tumor microenvironment responsive self-cascade catalysis for synergistic chemo/chemodynamic therapy by multifunctional biomimetic nanozymes. J. Mat. Chem. B 10, 637–645. doi:10.1039/d1tb01891d
Zhou, X., You, M., Wang, F., Wang, Z., Gao, X., Jing, C., et al. (2021a). Multifunctional graphdiyne-cerium oxide nanozymes facilitate microrna delivery and attenuate tumor hypoxia for highly efficient radiotherapy of esophageal cancer. Adv. Mat. 33, E2100556. doi:10.1002/adma.202100556
Zhou, Y., Fan, S., Feng, L., Huang, X., and Chen, X. (2021b). Manipulating intratumoral Fenton Chemistry for enhanced chemodynamic and chemodynamic-synergized multimodal therapy. Adv. Mat. 33, E2104223. doi:10.1002/adma.202104223
Zhou, Z., Wang, Y., Peng, F., Meng, F., Zha, J., Ma, L., et al. (2022). Intercalation-activated layered Moo3 nanobelts as biodegradable nanozymes for tumor-specific photo-enhanced catalytic therapy. Angew. Chemie-International Ed. 61, e202115939. doi:10.1002/anie.202115939
Zhu, E. Y., Riordan, J. D., Vanneste, M., Henry, M. D., Stipp, C. S., and Dupuy, A. J. (2022a). Src-Rac1 signaling drives drug resistance to braf inhibition in de-differentiated cutaneous melanomas. NPJ Precis. Oncol. 6, 74. doi:10.1038/s41698-022-00310-7
Zhu, L., Liu, J., Zhou, G., Liu, T.-M., Dai, Y., Nie, G., et al. (2021). Remodeling of tumor microenvironment by tumor-targeting nanozymes enhances immune activation of car T cells for combination therapy. Small 17, 2102624. doi:10.1002/smll.202102624
Keywords: nanozymes, catalytic therapy, enzyme mimics, cancer therapy, reactive oxygen species
Citation: Yu Y, Zhao W, Yuan X and Li R (2022) Progress and prospects of nanozymes for enhanced antitumor therapy. Front. Chem. 10:1090795. doi: 10.3389/fchem.2022.1090795
Received: 06 November 2022; Accepted: 21 November 2022;
Published: 02 December 2022.
Edited by:
Xianwen Wang, Anhui Medical University, ChinaCopyright © 2022 Yu, Zhao, Yuan and Li. This is an open-access article distributed under the terms of the Creative Commons Attribution License (CC BY). The use, distribution or reproduction in other forums is permitted, provided the original author(s) and the copyright owner(s) are credited and that the original publication in this journal is cited, in accordance with accepted academic practice. No use, distribution or reproduction is permitted which does not comply with these terms.
*Correspondence: Xianglin Yuan, eXVhbnhpYW5nbGluQGh1c3QuZWR1LmNu; Rui Li, ZnJlc2gwNDE5QDE2My5jb20=