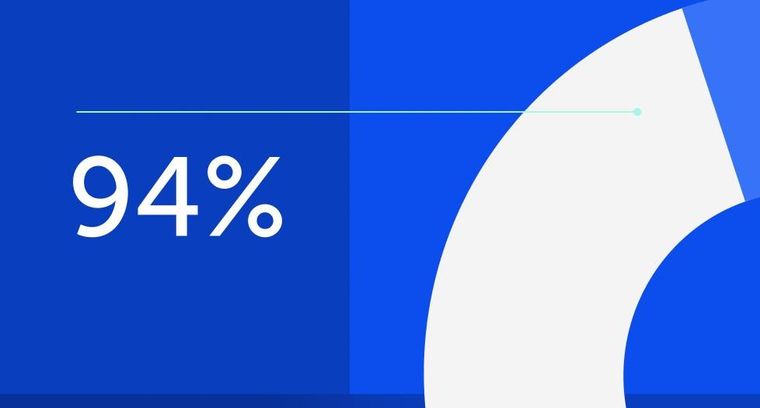
94% of researchers rate our articles as excellent or good
Learn more about the work of our research integrity team to safeguard the quality of each article we publish.
Find out more
ORIGINAL RESEARCH article
Front. Chem., 19 December 2022
Sec. Catalytic Reactions and Chemistry
Volume 10 - 2022 | https://doi.org/10.3389/fchem.2022.1079214
Oxidative steam reforming of ethanol (OSRE) to produce hydrogen has been investigated over a series of supported PtRu catalysts, with different supports. Bimetallic PtRu-based catalysts were prepared by the impregnation method using H2PtCl6 and RuCl3 as precursors. Six supports (reducible oxides of ZrO2, CeO2, and Co3O4, and irreducible oxides of ZnO, Al2O3, and NiO) were chosen to fabricate bimetallic catalysts. The catalytic performance of the OSRE reaction in the series of PtRu-based samples was evaluated using a fixed-bed flow reactor under atmospheric pressure. In front reaction, the catalyst was pre-activated by reduction under 200°C for 3 h. The gas hourly space velocity was adjusted at 66,000 h−1, and the optimal molar ratios of the H2O/EtOH and O2/EtOH feeds were 4.9 and 0.44, respectively. The results indicated that the PtRu supported on the ZrO2 and CeO2 exhibited superior catalytic performance in the OSRE reaction under a low temperature (a TR of approximately 320°C) for producing the main products of H2 and CO2 with lower CO and CH4 by-products. Also, it was quite stable during a long time evaluation; the maximum YH2 maintained at 4.5–4.2, and the CO distribution approached 3.3–3.5 mol% around 84 h test at 340°C over the PtRu/ZrO2 catalyst.
Hydrogen is a versatile element that is often used as a raw material for gasoline (the processes of hydrotreating and hydrocracking), chemistry (the synthesis of NH3 and CH3OH), foodstuff processing (hydrogenation of fats and oils), steel, and the manufacturing of electronics (Ramachandran and Menon, 1998; Armor, 1999). However, current industry does not produce hydrogen as an energy carrier or as a fuel for generators. In order to support sustainable global economic growth as well as reduce air pollution and the greenhouse effect, it is urgent to adopt hydrogen as an energy carrier; however, several technical hurdles must be conquered to manufacture and transfer the amount of hydrogen needed to reach a hydrogen-based economy.
Hydrogen can be manufactured from oxygenates, such as various ethanol reforming processes (Goldemberg, 2007; Ni et al., 2007; de la Piscina and Homs, 2008; Rabenstein and Hacker, 2008; Zanchet et al., 2015; Li et al., 2016) including the partial oxidation of ethanol (POE), steam reforming of ethanol (SRE), and oxidative steam reforming of ethanol (OSRE). The OSRE reaction is the incorporation of partial oxidation and steam reforming processes. Moreover, the OSRE reaction can lessen the energy of the SRE process and lower the rate of coke formation. Thermodynamic analysis of the production of hydrogen from ethanol by catalytic processes has found that the OSRE process possesses many advantages in terms of heat management and reforming efficiency (Rabenstein and Hacker, 2008).
Besides choosing a single active component (a noble or non-noble metal) as the reforming catalyst, multi-component catalysts have been reported in various catalytic reactions (Bi et al., 2007a; Alayoglu et al., 2008; Pereira et al., 2008; Koh et al., 2009; Pereira et al., 2010). Pereira et al. (Pereira et al., 2010) stated that the K-promoter enhances the catalytic behavior of CoRh/CeZr under the OSRE process to produce hydrogen, in which the addition of Rh facilitates the reducibility of Co. and enhances the catalytic activity. The same group also reported the effect of adding Ru and Rh to the Co./SiO2 catalysts and found a synergistic effect between Co and Ru (or Rh) in the OSRE reaction (Pereira et al., 2008). In particular, bimetallic PtRu catalysts have been widely used as CO tolerant for polymer electrolyte fuel cells (PEFCs) (Alayoglu et al., 2008). An investigation on the catalytic ability of PtRu-based catalysts for hydrogen production in reforming reactions confirmed that PtRu/ZrO2 is an excellent catalyst for low temperature OSRE reaction (Bi et al., 2007a). A nano-sized PtRu/Al2O3 originated from the organometallic clusters, which exhibited a high capability to manufacture hydrogen under the SRE reaction (Koh et al., 2009).
The performance of OSRE not only depends on the active metals; the other key factor is the nature of the support. To enhance the catalytic behavior of supported metal catalysts, support has influenced the catalytic performance (Llorca et al., 2002; Furtado et al., 2009; Youn et al., 2010; Wang and Spivey, 2015; Zhang et al., 2015; Tóth et al., 2016; Hsia et al., 2019). The effect of the support’s (ZnO, MgO, ZrO2, TiO2, and Al2O3) acidity on the OSRE activity of supported nickel catalysts was explored by Youn et al. (Youn et al., 2010), in which the intermediate acidity of Ni/Ti0.2Zr0.8O2 was identified as the optimal catalyst. The evaluation of the support materials (Al2O3, Nb2O5, and Ce0.6Zr0.4O2) of a bimetallic NiCu-based catalyst on the SRE reaction showed that the NiCu/Ce0.6Zr0.4O2 could achieve the best performance for hydrogen production (Furtado et al., 2009).
In our previous work, a PtRu/ZrO2 catalyst modified with Na in an OSRE indicates active at 300°C and begets little CO at 340°C (Wang et al., 2011). Here in, the OSRE reaction was further studied over series PtRu-based catalysts. Six supports (reducible oxides of ZrO2, CeO2, and Co3O4, and irreducible oxides of ZnO, Al2O3, and NiO) were picked to fabricate supported bimetallic catalysts. The goal of this effort was to find an excellent and durable catalyst that could be applied to the on-board reforming of ethanol at low temperatures.
Six supports (ZrO2, CeO2, Co3O4, ZnO, Al2O3, and NiO) were chosen to prepare the supported bimetallic catalysts. The Al2O3 was purchased from Merck (γ-form, 142 m2⋅g−1), while the others were self-synthesized. The ZrO2 (130 m2⋅g−1) was prepared using the sol-gel method (Bi et al., 2007a), the CeO2 (106 m2⋅g−1) was prepared using a simple reduction-oxidation method (Siang et al., 2010), the Co3O4 (102 m2⋅g−1) and NiO (104 m2⋅g−1) were prepared by the precipitation-oxidation method (Wang et al., 2005a; Lai et al., 2006), and the ZnO (89 m2⋅g−1) was obtained through the thermal decomposition of Zn(OH)2·2ZnCO3·xH2O (Stream) in the air (Chiou et al., 2012), respectively. The supported PtRu catalysts were prepared by the impregnation method using an aqueous solution of H2PtCl6 (Merck) and RuCl3 (Strem) as precursors (the loading of each component was 1.5 wt%). The as-prepared sample went through drying at 110°C and calcination at 400°C for 4 h in the air, after which the fabricated samples were smashed to 60–80 mesh and kept as fresh catalysts (both metal content and surface area are listed in Table 1).
Both the Pt and the Ru contents in the fresh catalysts were ascertained by the measurement of ICP-MS (Perkin-Elmer). The surface area (SBET) was measured with the physisorption using a Micromeritics ASAP 2010 instrument. First, the catalysts were pre-outgassed in a vacuum for 3 h at 110°C, then, the SBET was measured by the N2 adsorption at 77 K. The phases and crystalline structures were surveyed with X-ray diffraction (XRD) using a MAC Science MXP18 diffractometer with Cu Kα1 radiation (λ = 1.5405 Ǻ) at 30 mA and 40 kV. The particle size was observed using transmission electron microscopy (TEM) images from a JEM-2010 transmission electron microscope (JEOL) with a 200 kV acceleration voltage. During the TPR experiment, a 50 mg sample located in the reactor and was heated at a ramping rate of 7°C⋅min−1 from RT to 500°C under a reducing gas (10% H2/N2) with a flow rate of 10 ml⋅min−1. The consumption of H2 was detected continuously using a thermal conductivity detector (TCD).
A fixed-bed flow reactor was chosen to evaluate the performance of the PtRu-based catalysts towards the OSRE reaction under atmospheric pressure. A 100-mg sample was placed in a quartz tubular reactor (4 mm i. d.) and guarded with glass-wool plugs. The reactor was twined with heating tape and the temperature was controlled using a thermocouple (1.2 mm i. d.) placed in the center of the reactor bed. Prior to the reaction, the catalyst was pre-activated by reduction at 200°C for 3 h. The catalytic activity of the series of supported PtRu catalysts towards OSRE was executed by tuning the molar ratio of the H2O/EtOH (0.8–13) and O2/EtOH (0.32–0.61), which was executed between 280°C and 600°C under atmospheric pressure. The outlet gas was detected by both gas chromatography (GC), one with an MS-5A column (for the separation of H2, O2, CH4, and CO), and the other with a Porapak Q column (for the separation of C2H5OH, H2O, CH3CHO, CH3COOC2H5, and CO2). Based on the ethanol conversion (XEtOH), selectivity of carbon monoxide (SCO) and yield of hydrogen (YH2) to evaluate the catalytic performance as follows:
Figure 1A indicates the XRD patterns of the six bimetallic PtRu catalysts. Except for the PtRu/Al2O3 and PtRu/ZnO catalysts, which exhibited a very faint RuO2 phase, no significant diffraction peak of PtRu (111) was observed in all catalysts. This result demonstrated that the nanoparticles of the bimetallic PtRu were smaller than 5 nm. The crystal phase of metal oxides is conspicuous, i.e. the unique diffraction peaks of PtRu/NiO at 37.6o, 43.6o, 63.2o, and 75.7o, corresponding to the (111), (200), (220) and (311) planes, respectively, were attributed to the cubic NiO (JCPDS 04-0835); the clear diffraction peaks of PtRu/Co3O4 at 31.5o, 37.1o, 38.8o, 45.1o, 55.9o, 59.6o and 65.5o, corresponding to the (220), (311), (222), (400), (422), (511) and (440) planes, respectively, were ascribed to the spinel Co3O4 (JCPDS 43-1003); the noticeable diffraction peaks of PtRu/ZrO2 at 30.4o, 35.4o, 50.4o and 60.4o, corresponding to the (111), (200), (220) and (311) planes, respectively, were associated with the tetragonal ZrO2 (JCPDS 79-1769); the distinct diffraction peaks of PtRu/ZnO at 31.9o, 34.6o, 36.4o, 47.8o, 56.8o, 63.1o, 68.2o and 69.3o, corresponding to the (100), (002), (101), (102), (110), (103), (112) and (201) planes respectively, were well indexed to the hexagonal ZnO (JCPDS 79-2205); the bright diffraction peaks of PtRu/CeO2 at 28.5o, 33.1o, 47.5o and 56.4o corresponding to the (111), (200), (220) and (311) planes, respectively, were confirmed the cubic CeO2 (JCPDS 34-0394); the bright diffraction peaks of PtRu/Al2O3 at 37.3o, 45.9o and 66.9o, corresponding to the (311), (400) and (440) planes, respectively, were assigned to the γ-Al2O3 (JCPDS 10-0425). Figures 1B, C present the TEM images of the PtRu/CeO2 and PtRu/ZrO2, in which the average particle sizes of the PtRu were around 3.3 and 1.7 nm for both catalysts, respectively. The reducibility of the bimetallic PtRu catalysts was evaluated using temperature programmed reduction experiments (the TPR profile is listed in Figure 2). According to the literature (Trovarelli, 1996; Lin et al., 2003; Wang et al., 2005a; Wang et al., 2005b; Lai et al., 2006; Siang et al., 2010), the reduction of NiO occurs around 300°C (Wang et al., 2005b; Lai et al., 2006), the reduction of Co3O4 occurs around 200–400°C (Lin et al., 2003; Wang et al., 2005a), and the reduction of the surface capping oxygen ions of CeO2 occurs above 400°C (Trovarelli, 1996; Siang et al., 2010). The results showed that in addition to the reduction of the active species (the bimetallic oxide reduced at below 200°C for all catalysts and the RuO2 reduced at around 200°C–300°C for the PtRu/Al2O3 and PtRu/ZnO catalysts) (Bi et al., 2007a), the NiO, Co3O4, and the surface oxygen of the CeO2 supports could also be reduced at temperatures below 500°C.
FIGURE 1. XRD patterns and TEM images of series PtRu-based catalysts: (A) XRD profile (B) TEM image of PtRu/CeO2 catalyst (C) TEM image of PtRu/ZrO2 catalyst.
For the purpose of verification, the optimal feeds on the molar ratio of H2O/EtOH and O2/EtOH, the PtRu/ZrO2 sample has been chosen to tune both H2O/EtOH and O2/EtOH molar ratio, separately. First, a regular H2O/EtOH molar ratio of 4.9 was transmitted to the reactor to adjust the O2/EtOH molar ratio (0.32, 0.44, and 0.61, respectively). All situations revealed a complete conversion at and above 300°C. The distribution of products and the hydrogen yield are displayed in Table 2, which shows that the diminishing of the O2/EtOH ratio inclines to grow in number the amount of by-products (CO and CH4) and lessened the hydrogen yield from 280°C to 320°C. However, the raising of the O2/EtOH molar ratio inclines to facilitate the oxidation of hydrogen and reduced the hydrogen yield. Based on the tuning of O2/EtOH, 0.44 was the most appropriate molar ratio. Under this circumstance, both the YH2 improved and the CO reduced with the raising of the reaction temperature (TR). Second, a definite O2/EtOH molar ratio of 0.44 was delivered to the reactor to regulate the H2O/EtOH molar ratio (0.8, 2.2, 4.9, and 13, respectively). The product distribution and hydrogen yield are exhibited in Table 3, which reveals that the diminishing of the H2O/EtOH ratio inclines to boost the POE reaction, while the raising of the H2O/EtOH ratio stimulates produce more CO side-product. According to the regulating of the H2O/EtOH, 4.9 was the most appropriate molar ratio for the OSRE reaction, as it enhanced the hydrogen yield and reduced the CO distribution manifestly from 280°C to 320°C.
TABLE 2. Effect of O2/EtOH for OSRE reaction with various temperatures over the PtRu/ZrO2 catalyst under a molar ratio of H2O/EtOH = 4.9.
TABLE 3. Effect of H2O/EtOH for OSRE reaction with various temperatures over the PtRu/ZrO2 catalyst under a molar ratio of O2/EtOH = 0.44.
The literature (Mavrikakis and Barteau, 1998; Cavallaro et al., 2003; Fierro et al., 2005; Diagne et al., 2002) has reported that maintaining the OSRE process at a low temperature can preclude methanation (at an adequate temperature of around 500°C) and initiate large amounts of CO (at an adequate temperature of around 700°C). Based on the above consequents, the optimized feeds of the O2/EtOH and H2O/EtOH on the OSRE reaction were 0.44 and 4.9, respectively. Keeping this reforming condition, six bimetallic PtRu-based catalysts have been evaluated. Table 4 summarizes the conversion and distribution of products, and Figures 3, 4 gather up the YH2 and SCO over the series of PtRu-based catalysts for the OSRE reaction under various temperatures. The curves in Figures 3, 4 were drawn based on the fitting algorithm of the B-spline curve brought into the experimental data. In order to discuss the uncertainty analysis for numerical data, each sample was evaluated three times separately and the results indicated that the error was within ±1%–5%. Apparently, a synergistic effect emerged between the metal oxide supports and PtRu active species. The ethanol could be converted completely at lower temperatures for the reducible oxides (CeO2 and ZrO2 ≈ 280°C, Co3O4 > 300°C) supported catalysts with only C1 species (CH4, CO, and CO2), while the ethanol could be converted completely at higher temperatures for the irreducible oxides (Al2O3 > 350°C, NiO >400°C, and ZnO >450°C) supported catalysts with C1 species and a trace amount of undesirable C2H4 and CH3CHO species. Although the catalytic activity of the PtRu/Co3O4 catalyst was bright and the SCO was lower, the YH2 was also lower than 3, and the long-time reaction impel a deactivation by the progressive deposited carbon. The YH2 was high for the PtRu/NiO catalyst, while the TR approached a high temperature that initiated the decomposition of ethanol and increased the SCO as the TR moved above 500°C. Both the PtRu/Al2O3 and the PtRu/ZnO catalysts possessed a lower YH2 and higher SCO. Bi et al. (Bi et al., 2007b) suggested that the reducible oxides could offer the lattice oxygen (OL) to assist the oxidation of adsorbed CO (COad), and the irreducible oxides lacked the lattice oxygen which made the oxygen molecules had to be adsorbed on the metal surface (Oad). Since the OL is more effective than Oad for oxidation of COad, therefore, a high SCO produced easily from the desorption of COad over the irreducible oxides, and the Oad could integrate the adjacent adsorbed hydrogen to lower the YH2. Additionally, both the CeO2 and the ZrO2 can improve the dispersion of the active phase (Srinivas et al., 2003; Biswas and Kunzru, 2008), and possess large amount of surface oxygen vacancies that can restore/release oxygen (Hori et al., 1998; Silva et al., 2005) to prevent deactivation by the deposited coke and were active in the water gas shift (WGS) reaction (Gutierrez et al., 2011) to elevate the YH2 and lower the SCO. Both the Co3O4 and the NiO have been reported to exhibit the performance C−C bond cleavage on reforming of ethanol and a high YH2, however, both deactivated easily via the sintering and/or carbon deposition over the catalyst surface (Abdelkader et al., 2013; Sekine et al., 2009; da Silva et al., 2011). The Al2O3 has low mobile oxygen vacancies (Duprez, 1997), there is a limited number of oxygen intermediates available for CO oxidation, and the acidic support can stimulate the dehydration of ethanol to ethylene (Fatsikostas and Verykios, 2004), which pursued a high SCO and low YH2 production. Based on the demonstration and comparison of these reported literatures, in this study, both the PtRu/ZrO2 and the PtRu/CeO2 presented well-dispersed and excellent OSRE catalysts to produce hydrogen under low temperatures. The maximum YH2 approached 4.5 and the SCO was 3.3 mol% at 340°C for the PtRu/ZrO2 catalyst, while the YH2 was 4.0 and the CO distribution was 2.8 mol% for the PtRu/CeO2 catalyst, respectively.
TABLE 4. Catalytic performance in the OSRE reaction over series PtRu-based catalysts under O2/EtOH = 0.44 and H2O/EtOH = 4.9.
Further, in order to ascertain the features and derivations of the OSRE pathways over PtRu-based catalysts, the ethanol conversion along with the distribution of H2, CO2, CH4, and CO products in the outlet stream as a function of the TR was plotted between 260 and 380°C for both the PtRu/CeO2 and the PtRu/ZrO2 catalysts, as shown in Figures 5, 6. Summarizing with Table 4, also, comparing with other studies (Rabenstein and Hacker, 2008; Bi et al., 2007a; Mavrikakis and Barteau, 1998; Hung et al., 2012), the simplified reaction network in the OSRE process over the PtRu-based catalysts was outlined in Scheme 1. The involved intermediate steps included a variety of reactions which depended on the dependency relationships of different functionalities of the catalyst. Only minor ethylene produced over the PtRu/Al2O3 catalyst via the dehydration of adsorbed ethanol. All catalysts showed that the ethanol could be converted completely over the whole temperature range, and the consumption of oxygen was complete. The products of the H2 and CO2 increased with the TR. Thus, the performance at relatively low temperatures (<300°C) was mainly due to the partial oxidation of ethanol by consuming the O2 according to Eq. 4:
FIGURE 5. Catalytic performance in the OSRE reaction over the PtRu/CeO2 catalyst under O2/EtOH = 0.44, H2O/EtOH = 4.9.
FIGURE 6. Catalytic performance in the OSRE reaction over the PtRu/ZrO2 catalyst under O2/EtOH = 0.44, H2O/EtOH = 4.9.
The key step of ethanol reforming is the cracking of the C−C bond. The C−C bond can be cracked easily through the use of ruthenium and platinum (Davda et al., 2005; Zhao et al., 2019; Rajabi et al., 2021; Zare et al., 2021). The initial partial ethanol oxidation provided the potential for the dehydrogenation of the ethanol (Eqs 5, 6) and its decarbonylation (Eq. 8) over the PtRu/ZrO2 and PtRu/CeO2 catalysts are quicker than PtRu/ZnO and PtRu/NiO (where * is the active site), where the later produced a minor CH3CHO via desorption of adsorbed acetaldehyde.
The amount of CH4 was lower on both PtRu/ZrO2 and PtRu/CeO2 catalysts, indicating that the sequent steam reforming of the methyl (Eq. 9) after the splitting of the acetyl group (CH3CO) was preferential over the formation of methane (Eq. 10).
The SCO over PtRu/CeO2 was lower than PtRu/ZrO2 catalyst, while the CO distribution over PtRu/ZrO2 declined abruptly and accompanied the increase of CO2 and H2 as the TR upon 300°C. Also, the amount of CH4 grew and accompanied the decrease of H2 as the TR moved above 360°C. In accordance with these observations, the PtRu/CeO2 catalyst could preferentially oxidize the CO (Eq. 11) in the hydrogen-rich gases at a lower TR. The WGS (Eq. 12) reaction was favorable compared to the PtRu/ZrO2 catalyst at temperatures above 300°C for lowering the amount of CO, and the increase of CH4 coupled with the decrease of H2 upon 360°C was attributed to the methanation of CO or CO2 (Eq. 13).
Stability is a judgmental feature of any heterogeneous catalyst that decides its efficiency in a catalytic reaction. In order to highlight the discrepancy in the YH2 and SCO over the three reducible oxide-supported PtRu catalysts for the OSRE reaction, the evaluation of the catalyst stability was carried out as a function of the time-on-flux at 340°C, as shown in Figure 7. Except for the PtRu/Co3O4 catalyst, in which the activity decayed quickly with the reaction time, the conversion of the EtOH remained complete within the test over PtRu/ZrO2 and PtRu/CeO2 catalysts. Under the OSRE reaction, the deactivation of the Co-based catalyst could be attributed to the deposited coke, sintering of the active phase, and the encapsulation of metal sites into the support (da Silva et al., 2010; Espitia-Sibaja et al., 2017). Figure 8 displays the TEM image of the fresh and spent PtRu/Co3O4 catalyst. Apparently, the well-dispersed PtRu nanoparticles were somewhat agglomerated and capsulated by the amorphous carbon through the OSRE reaction over the PtRu/Co3O4 catalyst. To affirm the amorphous carbon, further analysis with thermal analysis, and the TG/DTG profile of spent PtRu/Co3O4 catalyst was listed in Figure 9. Apparently, the deposited carbon (∼6.5%wt loss) could be oxidized around 427°C. Both the PtRu/ZrO2 and PtRu/CeO2 catalysts showed preferential durability and exceeded 80 h of stability, whereas the PtRu/Co3O4 catalyst was deactivated within 20 h by the deposited carbon. The maximum YH2 was maintained at 4.5–4.2 and the CO distribution approached 3.3–3.5 mol% on the PtRu/ZrO2 catalyst. The maximum YH2 stayed at 4.0–3.6 and the CO distribution approached 2.8–3.2 mol% over the PtRu/CeO2 catalyst, respectively, around 84 h test at 340. Many reactions are associated with the OSRE, the aims to pursue a high YH2 while generating minimum CO. Greluk et al. (Greluk et al., 2016) studied the OSRE (H2O:EtOH:O2 = 9:1:0.7) using PtKCo/CeO2 catalyst, the temperature of 420°C was sufficient to achieve complete conversion but only maintained 5 h. Coke deposition that led to the removal of active sites from the catalyst surface was considered to be the major deactivation mechanism. The H2 distribution decreased from 68% to 60%, and the CO distribution increased from 2% to 2.5% with the increase of process time. Casanovas et al. (Casanovas et al., 2006) investigated the OSRE (H2O:EtOH:O2 = 13:1:0.5) using Pd/ZnO catalyst at low temperatures (300°C − 450°C), the ethanol could be converted completely. The H2 selectivity increased from 37% to 61%, and the CO selectivity decreased from 4.9% to 0.1% with increasing temperature. Palma et al. (Palma et al., 2017) surveyed the OSRE on mesoporous silica supported PteNi/CeO2 catalysts, which displayed stable behavior for 135 h under 500°C. Therefore, the fabricated Pt-Ru/ZrO2 showed potential as a candidate OSRE catalyst under low temperatures in this study.
FIGURE 7. Durability test for the OSRE reaction over PtRu/ZrO2, PtRu/CeO2 and PtRu/Co3O4 catalysts: (A) SCO (B) YH2.
This investigation indicated that the feed molar ratio and kinds of support have influence on the catalytic performance of PtRu-based catalysts. The optimal molar ratios of the H2O/EtOH and O2/EtOH feeds were 4.9 and 0.44, respectively for the OSRE reaction. In addition to the synergistic effect emerged between the metal oxide supports and PtRu active species, the reducible oxide supports also could provide the lattice oxygen to promote the oxidation of CO and the water gas shift reaction. Both the PtRu/ZrO2 and the PtRu/CeO2 catalysts appeared to be excellent OSRE catalysts to produce hydrogen under low temperatures with lesser distributions toward unwanted CO and CH4 with complete ethanol conversion. Evidently, the PtRu supported on ZrO2 and CeO2 exhibited superior catalytic performance in the OSRE reaction. It was quite stable during a long time evaluation of more than 80 h; the maximum YH2 was maintained at 4.5–4.2 and the CO distribution approached 3.3–3.5 mol% around 84 h test at 340°C on the PtRu/ZrO2 catalyst.
The original contributions presented in the study are included in the article/supplementary material, further inquiries can be directed to the corresponding authors.
C-HL: investigation, methodology, software. S-WY: investigation, methodology. C-WT: data curation, conceptualization, writing—original draft preparation. C-BW: resources, supervision, project administration, writing—review and editing.
We are pleased to acknowledge the financial support for this study from the Ministry of Science and Technology, Taiwan under contract number of MOST 108-2113-M-606-001-.
The authors declare that the research was conducted in the absence of any commercial or financial relationships that could be construed as a potential conflict of interest.
All claims expressed in this article are solely those of the authors and do not necessarily represent those of their affiliated organizations, or those of the publisher, the editors and the reviewers. Any product that may be evaluated in this article, or claim that may be made by its manufacturer, is not guaranteed or endorsed by the publisher.
Abdelkader, A., Daly, H., Saih, Y., Morgan, K., Mohamed, M. A., Halawy, S. A., et al. (2013). Steam reforming of ethanol over Co3O4-Fe2O3 mixed oxides. Int. J. Hydrogen Energy 38, 8263–8275. doi:10.1016/j.ijhydene.2013.04.009
Alayoglu, S., Nilekar, A. U., Mavrikakis, M., and Eichhorn, B. (2008). Ru–Pt core–shell nanoparticles for preferential oxidation of carbon monoxide in hydrogen. Nat. Mat. 7, 333–338. doi:10.1038/nmat2156
Armor, J. (1999). The multiple roles for catalysis in the production of H2. Appl. Catal. A General 176, 159–176. doi:10.1016/s0926-860x(98)00244-0
Bi, J. L., Hong, Y. Y., Lee, C. C., Yeh, C. T., and Wang, C. B. (2007). Novel zirconia-supported catalysts for low-temperature oxidative steam reforming of ethanol. Catal. Today 129, 322–329. doi:10.1016/j.cattod.2006.11.027
Bi, J. L., Hsu, S. N., Yeh, C. T., and Wang, C. B. (2007). Low-temperature mild partial oxidation of ethanol over supported platinum catalysts. Catal. Today 129, 330–335. doi:10.1016/j.cattod.2006.10.011
Biswas, P., and Kunzru, D. (2008). Oxidative steam reforming of ethanol over Ni/CeO2-ZrO2 catalyst. Chem. Eng. J. 136, 41–49. doi:10.1016/j.cej.2007.03.057
Casanovas, A., Llorca, J., Homs, N., Fierro, J. L. G., and de la Piscina, P. R. (2006). Ethanol reforming processes over ZnO-supported palladium catalysts: Effect of alloy formation. J. Mol. Catal. A Chem. 250, 44–49. doi:10.1016/j.molcata.2006.01.033
Cavallaro, S., Chiodo, V., Freni, S., Mondello, N., and Frusteri, F. (2003). Performance of Rh/Al2O3 catalyst in the steam reforming of ethanol: H2 production for MCFC. Appl. Catal. A General 249, 119–128. doi:10.1016/s0926-860x(03)00189-3
Chiou, J. Y. Z., Wang, W. Y., Wang, C. B., and Yeh, C. T. (2012). Surface area effect of zinc oxide for steam reforming of ethanol over supported-platinum catalystsffect of zinc oxide for steam reforming of ethanol over supported-platinum catalysts. e-J. Surf. Sci. Nanotechnol. 10, 431–436. doi:10.1380/ejssnt.2012.431
da Silva, A. M., da Costa, L. O. O., Souza, K. R., Mattos, L. V., and Noronha, F. B. (2010). The effect of space time on Co/CeO2 catalyst deactivation during oxidative steam reforming of ethanol. Catal. Commun. 11, 736–740. doi:10.1016/j.catcom.2010.02.005
da Silva, A. M., Mattos, L. V., den Breejen, J. P., Bitter, J. H., de Jong, K. P., and Noronha, F. B. (2011). Oxidative steam reforming of ethanol over carbon nanofiber supported Co catalysts. Catal. Today 164, 262–267. doi:10.1016/j.cattod.2010.11.013
Davda, R. R., Shabaker, J. W., Huber, G. W., Cortright, R. D., and Dumesic, J. A. (2005). A review of catalytic issues and process conditions for renewable hydrogen and alkanes by aqueous-phase reforming of oxygenated hydrocarbons over supported metal catalysts. Appl. Catal. B Environ. 56, 171–186. doi:10.1016/j.apcatb.2004.04.027
de la Piscina, P. R., and Homs, N. (2008). Use of biofuels to produce hydrogen (reformation processes). Chem. Soc. Rev. 37, 2459–2467. doi:10.1039/b712181b
Diagne, C., Idriss, H., and Kiennemann, A. (2002). Hydrogen production by ethanol reforming over Rh/CeO2–ZrO2 catalysts. Catal. Commun. 3, 565–571. doi:10.1016/s1566-7367(02)00226-1
Duprez, D. (1997). Study of surface mobility by isotopic exchange: Recent developments and perspectives. Stud. Surf. Sci. Catal. 112, 13–28.
Espitia-Sibaja, M., Muñoz, M., Moreno, S., and Molina, R. (2017). Effects of the cobalt content of catalysts prepared from hydrotalcites synthesized by ultrasound-assisted coprecipitation on hydrogen production by oxidative steam reforming of ethanol (OSRE). Fuel 194, 7–16. doi:10.1016/j.fuel.2016.12.086
Fatsikostas, A. N., and Verykios, X. E. (2004). Reaction network of steam reforming of ethanol over Ni-based catalysts. J. Catal. 225, 439–452. doi:10.1016/j.jcat.2004.04.034
Fierro, V., Akdim, O., Provendier, H., and Mirodatos, C. (2005). Ethanol oxidative steam reforming over Ni-based catalysts. J. Power Sources 145, 659–666. doi:10.1016/j.jpowsour.2005.02.041
Furtado, A. C., Alonso, C. G., Cantão, M. P., and Fernandes-Machado, N. R. C. (2009). Bimetallic catalysts performance during ethanol steam reforming: Influence of support materialsfluence of support materials. Int. J. Hydrogen Energy 34, 7189–7196. doi:10.1016/j.ijhydene.2009.06.060
Goldemberg, J. (2007). Ethanol for a sustainable energy future. Science 315, 808–810. doi:10.1126/science.1137013
Greluk, M., Słowik, G., Rotko, M., and Machocki, A. (2016). Steam reforming and oxidative steam reforming of ethanol over PtKCo/CeO2 catalyst. Fuel 183, 518–530. doi:10.1016/j.fuel.2016.06.068
Gutierrez, A., Karinen, R., Airaksinen, S., Kaila, R., and Krause, A. O. I. (2011). Autothermal reforming of ethanol on noble metal catalysts. Int. J. Hydrogen Energy 36, 8967–8977. doi:10.1016/j.ijhydene.2011.04.129
Hori, C. E., Permana, H., Simon Ng, K. Y., Brenner, A., More, K., Rahmoeller, K. M., et al. (1998). Thermal stability of oxygen storage properties in a mixed CeO2-ZrO2 system. Appl. Catal. B Environ. 16, 105–117. doi:10.1016/s0926-3373(97)00060-x
Hsia, Y. Y., Huang, Y. C., Zheng, H. S., Lai, Y. L., Hsu, Y. J., Luo, M. F., et al. (2019). Effects of O2 and H2O in the oxidative steam-reforming reaction of ethanol on Rh catalysts. J. Phys. Chem. C 123, 11649–11661. doi:10.1021/acs.jpcc.9b00747
Hung, C. C., Chen, S. L., Liao, Y. K., Chen, C. H., and Wang, J. H. (2012). Oxidative steam reforming of ethanol for hydrogen production on M/Al2O3. Int. J. Hydrogen Energy 37, 4955–4966. doi:10.1016/j.ijhydene.2011.12.060
Koh, A. C. K., Chen, L., Leong, K. W., Ang, T. P., Johnson, B. F. G., Khimyak, T., et al. (2009). Ethanol steam reforming over supported ruthenium and ruthenium–platinum catalysts: Comparison of organometallic clusters and inorganic salts as catalyst precursors. Int. J. Hydrogen Energy 34, 5691–5703. doi:10.1016/j.ijhydene.2009.05.044
Lai, T. L., Lee, C. C., Wu, K. S., Shu, Y. Y., and Wang, C. B. (2006). Microwave-enhanced catalytic degradation of phenol over nickel oxide. Appl. Catal. B Environ. 68, 147–153. doi:10.1016/j.apcatb.2006.07.023
Li, D., Li, X. Y., and Gong, J. L. (2016). Catalytic reforming of oxygenates: State of the art and future prospects. Chem. Rev. 116, 11529–11653. doi:10.1021/acs.chemrev.6b00099
Lin, H. K., Chiu, H. C., Tsai, H. C., Chien, S. H., and Wang, C. B. (2003). Synthesis, characterization and catalytic oxidation of carbon monoxide over cobalt oxide. Catal. Lett. 88, 169–174.
Llorca, J., Homs, N., Sales, J., and de la Piscina, P. R. (2002). Efficient production of hydrogen over supported cobalt catalysts from ethanol steam reformingficient production of hydrogen over supported cobalt catalysts from ethanol steam reforming. J. Catal. 209, 306–317. doi:10.1006/jcat.2002.3643
Mavrikakis, M., and Barteau, M. A. (1998). Oxygenate reaction pathways on transition metal surfaces. J. Mol. Catal. A Chem. 131, 135–147. doi:10.1016/s1381-1169(97)00261-6
Ni, M., Leung, D. Y. C., and Leung, M. K. H. (2007). A review on reforming bio-ethanol for hydrogen production. Int. J. Hydrogen Energy 32, 3238–3247. doi:10.1016/j.ijhydene.2007.04.038
Palma, V., Ruocco, C., Meloni, E., and Ricca, A. (2017). Oxidative steam reforming of ethanol on mesoporous silica supported Pt-Ni/CeO2 catalysts. Int. J. Hydrogen Energy 42, 1598–1608. doi:10.1016/j.ijhydene.2016.05.071
Pereira, E. B., de la Piscina, P. R., Martí, S., and Homs, N. (2010). H2 production by oxidative steam reforming of ethanol over K promoted Co-Rh/CeO2-ZrO2 catalysts. Energy Environ. Sci. 3, 487–493. doi:10.1039/b924624j
Pereira, E. B., Homs, N., Martí, S., Fierro, J. L. G., and de la Piscina, P. R. (2008). Oxidative steam-reforming of ethanol over Co/SiO2, Co–Rh/SiO2 and Co–Ru/SiO2 catalysts: Catalytic behavior and deactivation/regeneration processes. J. Catal. 257, 206–214. doi:10.1016/j.jcat.2008.05.001
Rabenstein, G., and Hacker, V. (2008). Hydrogen for fuel cells from ethanol by steam-reforming, partial-oxidation and combined auto-thermal reforming: A thermodynamic analysis. J. Power Sources 185, 1293–1304. doi:10.1016/j.jpowsour.2008.08.010
Rajabi, Z., Jones, L., Martinelli, M., Qian, D., Cronauer, D. C., Kropf, A. J., et al. (2021). Influence of Cs promoter on ethanol steam-reforming selectivity of Pt/m-ZrO2 catalysts at low temperature. Catalysts 11, 1104. doi:10.3390/catal11091104
Ramachandran, R., and Menon, R. K. (1998). An overview of industrial uses of hydrogen. Int. J. Hydrogen Energy 23, 593–598. doi:10.1016/s0360-3199(97)00112-2
Sekine, Y., Kazama, A., Izutsu, Y., Matsukata, M., and Kikuchi, E. (2009). Steam reforming of ethanol over cobalt catalyst modified with small amount of iron. Catal. Lett. 132, 329–334. doi:10.1007/s10562-009-0133-6
Siang, J. Y., Lee, C. C., Wang, C. H., Wang, W. T., Deng, C. Y., Yeh, C. T., et al. (2010). Hydrogen production from steam reforming of ethanol using a ceria-supported iridium catalyst: Effect of different ceria supports. Int. J. Hydrogen Energy 35, 3456–3462. doi:10.1016/j.ijhydene.2010.01.067
Silva, P. P., Silva, F. A., Portela, L. S., Mattos, L. V., Noronha, F. B., and Hori, C. E. (2005). Effect of Ce/Zr ratio on the performance of Pt/CeZrO2/Al2O3 catalysts for methane partial oxidation. Catal. Today 107, 734–740. doi:10.1016/j.cattod.2005.07.004
Srinivas, D., Satyanarayana, C. V. V., Potdar, H. S., and Ratnasamy, P. (2003). Structural studies on NiO-CeO2-ZrO2 catalysts for steam reforming of ethanol. Appl. Catal. A General 246, 323–334. doi:10.1016/s0926-860x(03)00085-1
Tóth, M., Varga, E., Oszkó, A., Baán, K., Kiss, J., and Erdőhelyi, A. (2016). Partial oxidation of ethanol on supported Rh catalysts: Effect of the oxide support. J. Mol. Catal. A Chem. 411, 377–387. doi:10.1016/j.molcata.2015.11.010
Trovarelli, A. (1996). Catalytic properties of ceria and CeO2-containing materials. Catal. Rev. 38, 439–520. doi:10.1080/01614949608006464
Wang, C. B., Gau, G. Y., Gau, S. J., Tang, C. W., and Bi, J. L. (2005). Preparation and characterization of nanosized nickel oxide. Catal. Lett. 101, 241–247. doi:10.1007/s10562-005-4899-x
Wang, C. B., Tang, C. W., Gau, S. J., and Chien, S. H. (2005). Effect of the surface area of cobaltic oxide on carbon monoxide oxidation. Catal. Lett. 101, 59–63. doi:10.1007/s10562-004-3750-0
Wang, C. H., Ho, K. F., Chiou, J. Y. Z., Lee, C. L., Yang, S. Y., Yeh, C. T., et al. (2011). Oxidative steam reforming of ethanol over PtRu/ZrO2 catalysts modified with sodium and magnesium. Catal. Commun. 12, 854–858. doi:10.1016/j.catcom.2011.02.002
Wang, Z., and Spivey, J. J. (2015). Effect of ZrO2, Al2O3 and La2O3 on cobalt-copper catalysts for higher alcohols synthesis. Appl. Catal. A General 507, 75–81. doi:10.1016/j.apcata.2015.09.032
Youn, M. H., Seo, J. G., Lee, H., Bang, Y., Chung, J. S., and Song, I. K. (2010). Hydrogen production by auto-thermal reforming of ethanol over nickel catalysts supported on metal oxides: Effect of support acidity. Appl. Catal. B Environ. 98, 57–64. doi:10.1016/j.apcatb.2010.05.002
Zanchet, D., Santos, J. B. O., Damyanova, S., Gallo, J. M. R., and Bueno, J. M. C. (2015). Toward understanding metal-catalyzed ethanol reforming. ACS Catal. 5, 3841–3863. doi:10.1021/cs5020755
Zare, M., Saleheen, M., Zare, M., Saleheen, M., Mamun, O., Heyden, A., et al. (2021). Aqueous-phase effects on ethanol decomposition over Ru-based catalysts. Catal. Sci. Technol. 11, 6695–6707. doi:10.1039/d1cy01057c
Zhang, R. J., Xia, G. F., Li, M. F., Wu, Y., Nie, H., and Li, D. D. (2015). Effect of support on the performance of Ni-based catalyst in methane dry reforming. J. Fuel Chem. Technol. 43, 1359–1365. doi:10.1016/s1872-5813(15)30040-2
Keywords: oxidative steam reforming of ethanol, H2 production, ZrO2, CeO2, PtRu-based based catalysts, support effect
Citation: Tang C-W, Liu C-H, Yu S-W and Wang C-B (2022) Effect of support on the performance of PtRu-based catalysts in oxidative steam reforming of ethanol to produce hydrogen. Front. Chem. 10:1079214. doi: 10.3389/fchem.2022.1079214
Received: 25 October 2022; Accepted: 05 December 2022;
Published: 19 December 2022.
Edited by:
Weijie Zhang, University of Virginia, United StatesReviewed by:
Fanji Kong, University of Virginia, United StatesCopyright © 2022 Tang, Liu, Yu and Wang. This is an open-access article distributed under the terms of the Creative Commons Attribution License (CC BY). The use, distribution or reproduction in other forums is permitted, provided the original author(s) and the copyright owner(s) are credited and that the original publication in this journal is cited, in accordance with accepted academic practice. No use, distribution or reproduction is permitted which does not comply with these terms.
*Correspondence: Chih-Wei Tang, Y3d0YW5nQGFhcm9jLmVkdS50dw==; Chen-Bin Wang, Y2hlbmJpbndhbmdAZ21haWwuY29t
Disclaimer: All claims expressed in this article are solely those of the authors and do not necessarily represent those of their affiliated organizations, or those of the publisher, the editors and the reviewers. Any product that may be evaluated in this article or claim that may be made by its manufacturer is not guaranteed or endorsed by the publisher.
Research integrity at Frontiers
Learn more about the work of our research integrity team to safeguard the quality of each article we publish.