- 1Faculty of Materials Science and Engineering, Kunming University of Science and Technology, Kunming, China
- 2State Key Laboratory of Infrared Physics, Shanghai Institute of Technical Physics, Chinese Academy of Sciences, Shanghai, China
Sr3YCo4O10.5+δ (314-SYCO), with an unusual ordered structure and a high Curie temperature (Tc ≈ 335 K), is attracting increasing attention. Herein, to improve the electrical performance of 314-SYCO, Cu-doped Sr3YCo4−xCuxO10.5+δ (x = 0–0.8) ceramics were prepared using a solid-state reaction method. Systematic research was conducted on both the ordered phase transformation and the effects of Cu doping on the microstructure, electrical transport characteristics, and magnetic properties. For x = 0–0.4, the (103) and (215) planes were observed and combined with Rietveld refinement results for the X-ray diffraction data, confirming the formation of ordered tetragonal Sr3YCo4−xCuxO10.5+δ. This phase was formed with a mass gain of ∼0.8% and heat released at ∼1,042°C. With increasing Cu content, the concentration of hole carriers also increased, leading to a substantial reduction in electrical resistivity. The electrical resistivity decreased by 92–99% at 300 K. The polycrystalline materials have semiconducting behaviour with a three-dimensional Mott variable-range hopping mechanism. For the magnetic properties, a Hopkinson peak was observed at 319 K, and the Tc was approximately 321 K for x = 0. The magnetisation and Tc decreased with increasing Cu content, and a G-type antiferromagnetic-to-ferromagnetic phase transition occurred due to the spin state change for some Co3+ ions from high/intermediate spin to low/intermediate spin. These results lay the groundwork for refinement of the sintering procedure and doping parameters to enhance the performance of 314-SYCO in the context of current applications such as microwave absorbers and solid oxide fuel cell cathodes.
1 Introduction
The oxygen-deficient perovskite Sr3YCo4O10.5+δ (314-SYCO) with an ordered tetragonal structure has potential applications in microwave absorbing materials, solid oxide fuel cells, and other fields owing to its room-temperature ferromagnetism (Golosova et al., 2009; Marik et al., 2018; Istomin et al., 2003), orbital and charge ordering (Khalyavin et al., 2011; Sheptyakov et al., 2009; Kishida et al., 2016), high electronic conductivity, and excellent activity for the oxygen-reduction reaction (Li et al., 2011; Lalan et al., 2019). The A-site ordered (AO) and oxygen vacancy ordered (OO) tetragonal superstructure of 314-SYCO is composed of alternating octahedral CoO6 layers and tetrahedral CoO4.25+δ layers stacked along the c-axis. The A-site cations are arranged as –Sr–Y–Y–Sr– units along the c-axis, and the Sr2+: Y3+ ratio is 3:1 in the ab-plane. The oxygen vacancies of the CoO4.25+δ layer are arranged in a zigzag pattern in the bc-plane (Khalyavin et al., 2011). For δ = −0.26–0.3, 314-SYCO usually has an AO/OO tetragonal superstructure, as illustrated in Figure 1A (Istomin et al., 2003; Fukushima et al., 2009). Moreover, the basic magnetic structure of 314-SYCO is a G-type antiferromagnet, as illustrated in Figure 1B (Sheptyakov et al., 2009). The magnetic properties are mainly derived from oxygen ordering at oxygen-vacancy sites (Kobayashi et al., 2005; Fukushima et al., 2008; Fukushima et al., 2009).
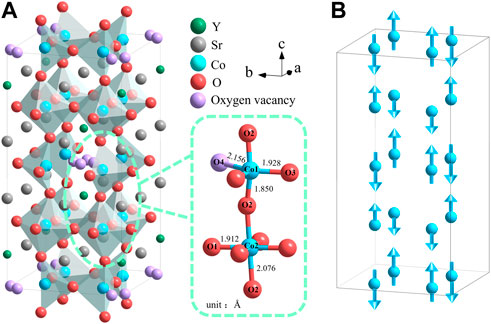
FIGURE 1. (A) Crystal structure of 314-SYCO shows the connection between the Co1O4+1 polyhedra and Co2O6 octahedra from neighbouring layers. (B) G-type antiferromagnetic ordering of Co cations along the c-axis.
The 314-SYCO material has a complex and interesting phase-transition process. Hu et al. (Hu et al., 2017) observed an exothermic peak at 963°C in differential scanning calorimetry (DSC) curves, corresponding to the crystallisation of 314-SYCO. In addition, a weight loss at 400°C in the thermogravimetric (TG) curves of 314-SYCO samples in oxygen, air, and helium atmospheres were observed due to the removal of O4 (the Co1–O4 distance is the longest, as illustrated in Figure 1). At approximately 1,000°C in a helium atmosphere, the oxygen content in polycrystals that had lost most of their O4 was close to 10 (Sr3YCo4O10), resulting in a brownmillerite-type structure (Istomin et al., 2003). However, there are limited reports on the phase transition of 314-SYCO during its synthesis.
The phase structure, ordering, and physical properties of 314-SYCO could be modulated by Co-site doping. With increased Fe doping, Sr0.75Y0.25Co1−xFexO2.625+δ changes from an ordered tetrahedral structure to a disordered cubic structure, while Sr0.75Y0.25Co1−xGaxO2.625+δ has a tetragonal superstructure with decreased magnetic order at x = 0.25 (Lindberg et al., 2006). In the CoO6 layer and/or antiferromagnetic CoO4.25 layer, Ga3+ ions both replace the high-spin-state Co3+ ions and increase the saturation magnetisation of Co ions. Further, it was shown that both the thermal expansion coefficient and conductivity of Sr3YCo4−xFexO10.5+y decrease at 1173 K (Istomin et al., 2008). According to previous studies, intermediate-spin state Co3+ ions get preferentially replaced by Al3+ ions in the ferrimagnetic CoO6 layer, which lowers the Sr3.1Y0.9Co4O10.5 saturation magnetisation (Tsuruta et al., 2020). The addition of Al3+ disrupts the oxygen-ordering superstructure, which further inhibits the ferromagnetism of 314-SYCO at room temperature (Rajan and Subodh, 2020). As Cu has a similar ionic radius to Co, Cu doping at the Co site can directly increase carrier concentration. Therefore, the electrical properties of 314-SYCO can be significantly improved by substituting Co with Cu. In addition, CuO, as a sintering agent, increases the content of the liquid phase during sintering, thus improving the sintering quality. Our research group first proposed that Cu doping can reduce the resistivity of 314-SYCO (Du et al., 2014). However, a thorough investigation of the effects of Cu doping on the electromagnetic characteristics of polycrystalline 314-SYCO has not yet been conducted. The oxygen-deficient perovskite 314-SYCO has been proven to be useful as a microwave absorber or as a cathode for solid oxide fuel cells due to its high electronic conductivity and excellent activity for oxygen reduction reactions (Li et al., 2011; Lalan et al., 2019). Moreover, low electrical resistivity is an important physical parameter for microwave absorbers or cathodes for solid oxide fuel cells.
Here, the ordered phase transformation of 314-SYCO is reported for the first time. The microstructure, electrical transport, and magnetic properties of Sr3YCo4−xCuxO10.5+δ (x = 0, 0.2, 0.4) were investigated in detail. For x = 0–0.4, the ordered tetragonal Sr3YCo4−xCuxO10.5+δ phase was formed. The key step in the formation of the ordered tetragonal phase is an exothermic reaction at ∼1,042°C and an oxygen mass gain of ∼0.8%. With increasing Cu content, the electrical resistivity decreases greatly, and the polycrystalline material shows the three-dimensional Mott variable-range hopping mechanism typical of a semiconductor. Additionally, a G-type antiferromagnetic-to-ferromagnetic phase transition occurs due to a reduction in the spin state of some Co3+ ions.
2 Material and methods
2.1 Materials and synthesis
Using a solid-state reaction technique, Sr3YCo4−xCuxO10.5+δ (x = 0, 0.2, 0.4, 0.6, and 0.8) was made polycrystalline. The raw materials were SrCO3 (99.95%), Y2O3 (99.9%), Co3O4 (99.9%), and CuO (99%). All starting materials were purchased from Shanghai Aladdin Biochemical Technology Co., Ltd. (Shanghai, China). Stoichiometric amounts of the starting reagents were weighed (Sr: Y: Co: Cu = 3:1: 4−x: x) and homogeneously mixed in an agate mortar for at least 120 min. Following this, the powdered mixture was pressed into disc-shaped tablets (4 MPa/5 min +5 MPa/5 min) with a diameter of 20 mm and height of 3.0–3.5 mm, which were sintered in air at 1,100°C for 24 h.
2.2 Characterization of samples
The phase transitions during the sintering of the Sr3YCo4−xCuxO10.5+δ raw ingredients were investigated using TG-DSC (STA449F3, Netzsch, Selb, Germany) from 25 to 1,100°C at a heating rate of ∼10°C/min; the ingredient quantities were 7.5–9.5 mg. The phase structure of the sintered samples was identified using X-ray diffraction (XRD; Ultima IV, Rigaku Corporation, Tokyo, Japan; CuKα radiation with wavelength λ = 1.5406 Å, step size of 0.02°) through θ–2θ scans (40 kV, 40 mA, scan range of 10–100°, scan rate of 4°/min) and slow scans (40 kV, 40 mA, scan ranges of 20–21°, 38.5–39.5°, or 46.5–48°, scan rate of 0.4°/min). High-resolution transmission electron microscopy (HRTEM; Tecnai G2 F30 S-TWIN, FEI Company, Oregon, United States) and fast Fourier transform (FFT) analyses were used to establish the crystal structure. To examine the morphologies and Cu ion distributions, scanning electron microscopy coupled with energy-dispersive spectrometry (SEM-EDS; XL30ESEM, Philips, Amsterdam, Netherlands) was used. The sample density was measured using Archimedes’ method. X-ray photoemission spectroscopy (XPS) was conducted on an electron spectrometer (PHI5000 VersaProbe III, ULVAC-PHI, Inc., Kanagawa, Japan) with a monochromatic Al Kα irradiation source. Using a four-probe method, resistivity-temperature (ρ-T) curves were produced for the temperature range of 75–300 K. Seebeck coefficient-temperature (S-T) curves were recorded using a Seebeck measurement device (LSR-3/1,000, Linseis Messgeräte GmbH, Selb, Germany) in the temperature range of 300–1100 K. Using a superconducting quantum interference device (MPMS-XL, Quantum Design, Inc., California, United States) with a magnetic field of ∼1 T and a temperature range of 4–380 K, magnetisation-temperature (M-T) curves were measured.
3 Results and discussion
Figure 2 shows the XRD patterns of the polycrystalline Sr3YCo4−xCuxO10.5+δ (x = 0–0.8) sintered at 1,100°C for 24 h. For x = 0–0.4, all diffraction peaks were indexed to the tetragonal superstructure (PDF#54–0,234), i.e., I4/mmm. As the Cu doping content increased, the diffraction peaks of the samples shifted toward a lower angle, indicating an increase in the lattice constant due to the partial replacement of Co3+/4+ (0.61/0.53 Å) by Cu2+ (0.73 Å) (Hsieh and Fung, 2008; Zhao et al., 2010). Figures 2A,B show the presence of (008)(400), (228)(424), and (408)(440) split peaks. Both sets of spectra indicate a = b ≠ 1/2c, which is characteristic of a tetragonal structure (Hu et al., 2017). The peaks of (103) and (215) in Figures 2C,D imply that Sr3YCo4−xCuxO10.5+δ polycrystals had an ordered lattice, consistent with the ordered tetragonal phase extinction law (h, k, l ≠ 2n) (Kobayashi et al., 2005). Figures 2E–G show that the experimentally measured XRD diffraction peaks exhibit a high degree of overlap with the feasibility of Rietveld refinement, indicating that the samples from x = 0 to 0.4 are pure ordered tetragonal phase. The final reliability factors for the recorded patterns are Rwp = 3.346% and χ2 = 1.69 for x = 0, Rwp = 3.503% and χ2 = 1.77 for x = 0.2, and Rwp = 6.719% and χ2 = 3.30 for x = 0.4.
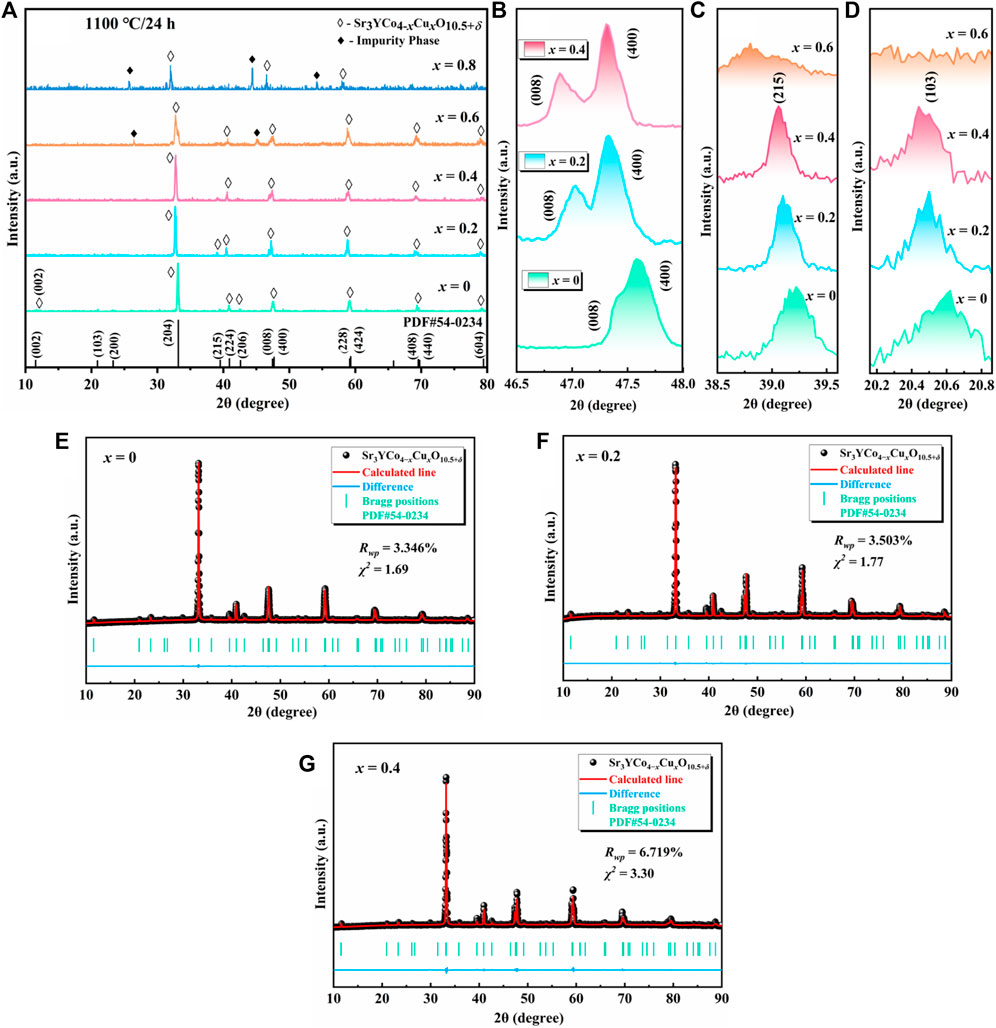
FIGURE 2. XRD patterns of Sr3YCo4−xCuxO10.5+δ (x = 0–0.8) polycrystals sintered at 1,100°C for 24 h (A) x = 0–0.8; slow scans (0.4°/min) of (B) (008)(400) split peak, (C) (103) peak, and (D) (215) peak. (E–G) XRD refinement results of Sr3YCo4−xCuxO10.5+δ (x = 0–0.4).
The average grain size in the direction of the vertical grain plane (204), D(204), was calculated using Scherrer equation

TABLE 1. Crystal and physical properties of Sr3YCo4−xCuxO10.5+δ polycrystals sintered at 1,100°C for 24 h.
Figure 3A displays the HRTEM pictures together with their accompanying FFT diffraction spots, and Figure 3B illustrates how the crystal structure of the Sr3YCo4−xCuxO10.5+δ (x = 0.2) samples correspond to the diffraction spots. The d-spacings of 0.539 and 0.424 nm are indexed to the
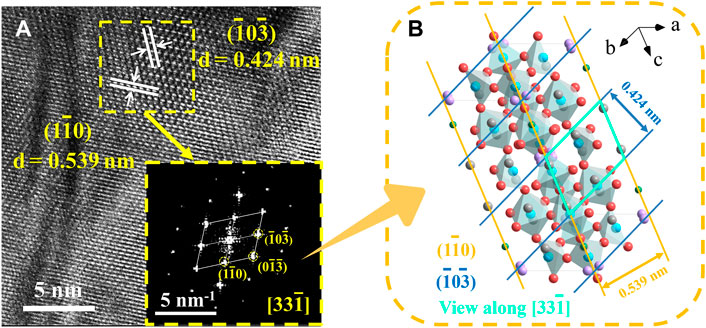
FIGURE 3. (A) HRTEM image of Sr3YCo4−xCuxO10.5+δ polycrystals: x = 0.2 (ordered tetrahedral). The inset shows the corresponding FFT pattern of Sr3YCo4−xCuxO10.5+δ. (B) Relationship between the FFT diffraction spots and the corresponding crystal structure.
TG-DSC curves were measured to simulate the sintering of the raw ingredients to produce Sr3YCo4−xCuxO10.5+δ (x = 0–0.8). Figures 4A–E show three stages in all TG curves, i.e., approximately 25–680°C for stage I, 680–969°C for stage II, and 969–1,100°C for stage III. The curves were similar for all samples in stages I and II.
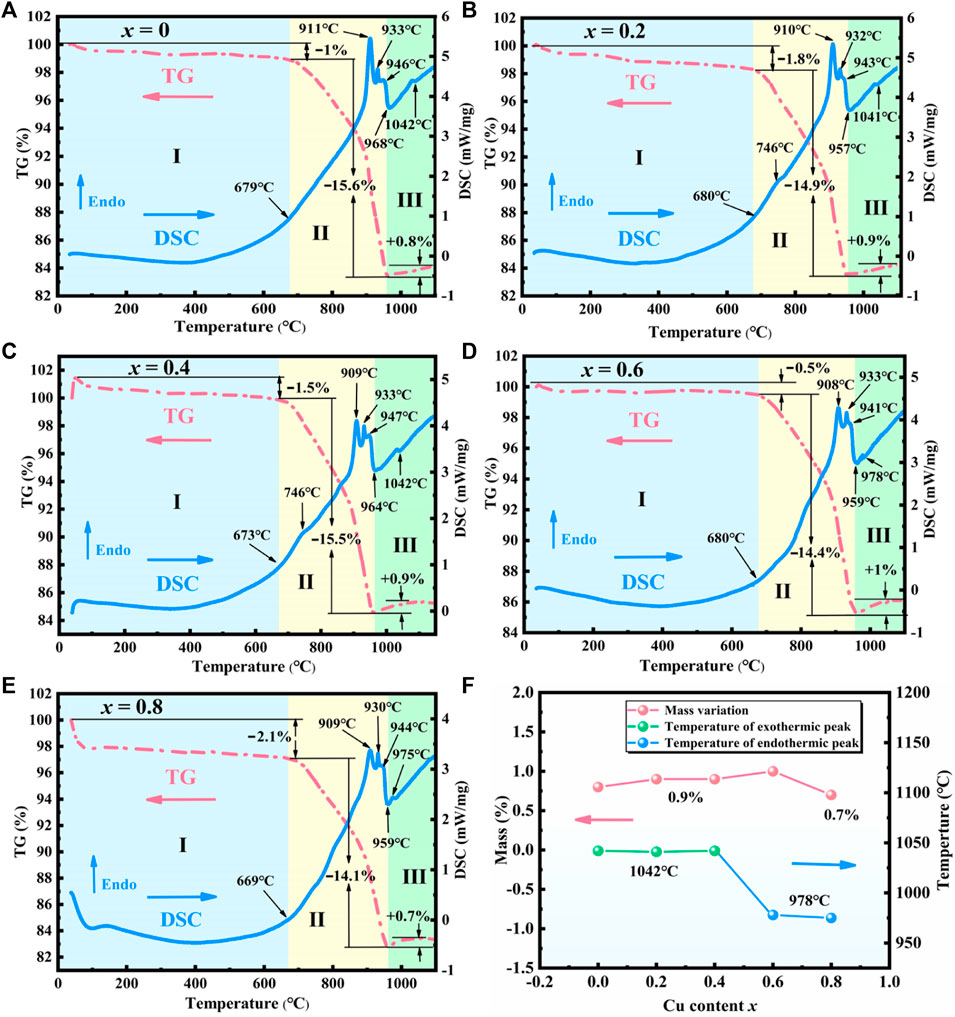
FIGURE 4. TG-DSC curves of the sintering ingredients of Sr3YCo4−xCuxO10.5+δ for x values of (A) 0, (B) 0.2, (C) 0.4, (D) 0.6, (E) 0.8. (F) Mass variation and thermal effects for x = 0–0.8 in stage Ⅲ.
In stage Ⅰ, the mass loss of all samples was in the range of 0.5–2.5% due to the dehydration of the raw materials. The mass loss was in the range of 12–15.6% in stage II. The endothermic peak at ∼910°C corresponds to the phase transition from orthorhombic to trigonal SrCO3 and the decomposition of Co3O4 into CoO and O2 (Delorme et al., 2015). Trigonal SrCO3 is broken into SrO and CO2, which produce the endothermic peak at ∼933°C (Ptáček et al., 2015).
As the temperature increased above 946°C, all TG curves showed further weight loss, indicating that SrCO3 continued to decompose. In the DSC curves, the exothermic peak at ∼968°C corresponds to the crystallisation of Sr3YCo4–xCuxO10.5, because one of the four adjacent oxygen vacancies (Figure 1A) can be occupied easily (O10→O10.5) (Istomin et al., 2003; Rupasov et al., 2009), as indicated by Eq. 1.
For x = 0.2–0.8, the weak endothermic peak observed at 746–811°C may be related to a trace eutectic mixture formed by CuO and SrCO3–Y2O3–Co3O4 (Kingery and Narasimhan, 1959).
In stage Ⅲ, the mass variation and thermal behaviour are significantly dependent on x. As shown in Figure 4F, the mass gain is 0.8–0.9% for x = 0–0.4, while the DSC curves show a weak exothermic peak at approximately 1,042°C, corresponding to the uptake of oxygen (δ) (Eq. 2).
For x = 0.6–0.8, the weak endothermic peak at 978°C may correspond to an impurity formed by heating. Despite a mass gain of 0.7–1%, the exothermic peaks related to the ordered phase were not observed at ∼1,042°C. This observation is consistent with the XRD patterns in Figure 2, where the (103) and (215) peaks were not observed for x = 0.6. The lack of the ordered phase may be caused by excessive Cu doping, implying that the solid solubility of Cu is x = 0.4–0.6.
Figure 5A shows photographs of polycrystalline Sr3YCo4−xCuxO10.5+δ sintered at 1,100°C for 24 h. For x = 0–0.4, the surfaces are smooth and flat, and the volume drastically reduced with increasing x (i.e., enhanced sintering). For x = 0.6–0.8, the corrosion of the samples with the crucible became increasingly severe as the dissolution exceeded the solid limit, and low-melting-point heterogeneous phases were produced. Cross-sectional SEM images of polycrystalline Sr3YCo4−xCuxO10.5+δ (x = 0–0.4) (Figures 5B–D) indicate the porous structures of these samples. With increasing x, an increased density is observed as a result of sintering, i.e., the pore volume reduced to form close grain connections (Figure 5E). These trends also align with the findings in Table 1. Figure 5F shows a surface SEM image of Sr3YCo4−xCuxO10.5+δ (x = 0.6) polycrystalline material. The grain clusters are highlighted in blue and the lighter lines are the grain boundaries. According to EDS analysis, spot 2 (Figure 5H) has significantly higher Cu content than spot 1 (Figure 5G). Therefore, the grain clusters are thought to be a Cu-rich phase.
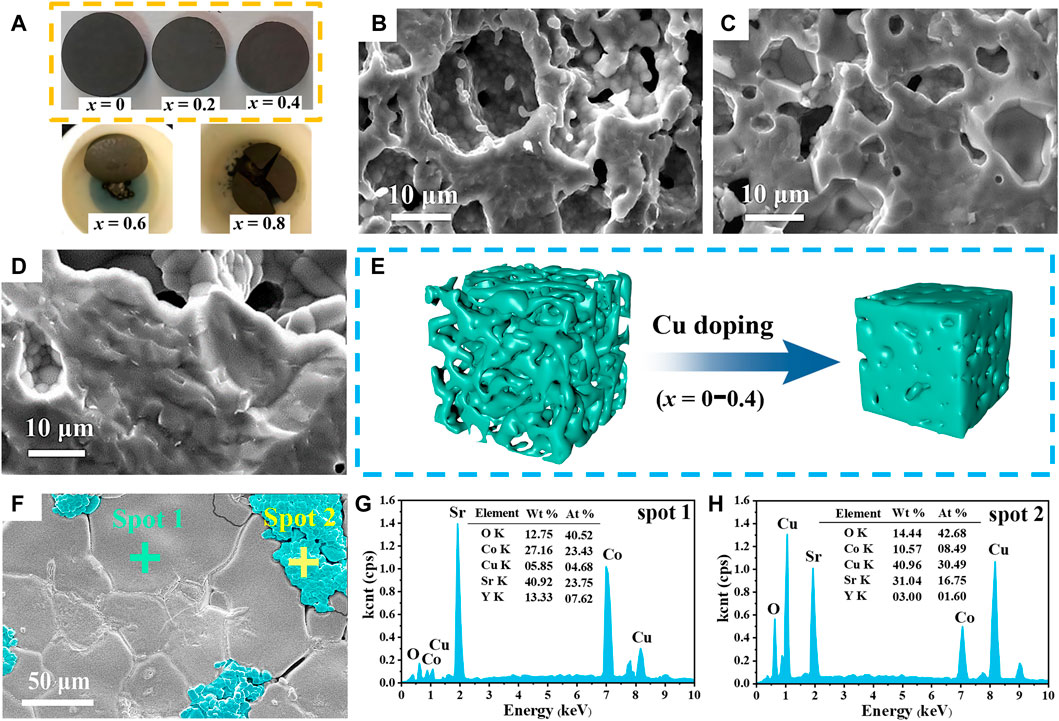
FIGURE 5. (A) Photographs of Sr3YCo4−xCuxO10.5+δ samples sintered at 1,100°C for 24 h for x values of 0, 0.2, and 0.4, 0.6, and 0.8. Cross-sectional SEM images of Sr3YCo4−xCuxO10.5+δ for x values of: (B) 0, (C) 0.2, and (D) 0.4. (E) Schematic of the morphological changes (x = 0–0.4). (F) Surface SEM image of the Sr3YCo4−xCuxO10.5+δ polycrystal (x = 0.6). Sample x = 0.6 EDS spectra at (G) spot 1 and (H) spot 2 in (F).
The XPS survey spectra of Sr3YCo4−xCuxO10.5+δ samples (x = 0, 0.2, and 0.4) are shown in Figure 6A, confirming the presence of elements in these samples; no other impurity elements were detected, demonstrating the high purity of the experimentally-produced polycrystals. The XPS Co elemental spectra for the samples (x = 0, 0.2, and 0.4) are presented in Figures 6B–D. In contrast with the Co3+ ion, Co4+ contains a more positive electrical charge and lower electron cloud density, thus the binding energy of 2p electrons should also be higher (LU et al., 2013). As a result, Co3+ ions have a lower binding energy than Co4+ ions. Therefore, the intensity peaks appearing at 779.77/794.74 eV for x = 0, 780.5/795.02 eV for x = 0.2 and 779.68/794.65 eV for x = 0.4 can be assigned to Co3+, while the peaks of 782.18/797.15 eV for x = 0, 782.36/797.33 eV for x = 0.2 and 781.81/796.78 eV for x = 0.4 can be assigned to Co4+ ion. For each composition, the Co3+/Co4+ ratio is determined by fitting the area under the curve. The Co3+/Co4+ ratio for each composition is presented in Table 2. It was observed that the Co3+ content decreased significantly with the increase in the Cu doping amount. The XPS results confirm that the Cu2+ ions predominantly occupy Co3+ sites. In Figure 6E, the Cu2+ signal (Cu2p3/2 and Cu2p1/2 are 934.07 and 953.87 eV for x = 0.2, 933.49 and 953.29 eV for x = 0.4, respectively) and its satellite peak (936.0–946.0 eV) in the Cu 2p spectral range are obvious (Wu et al., 2018; Li et al., 2022). The results show that in Sr3YCo4−xCuxO10.5+δ, Cu ions exist in the oxidation state of +2 valence. Compared to the element Co, Cu 2p is relatively weak. This phenomenon is consistent with its low element content.
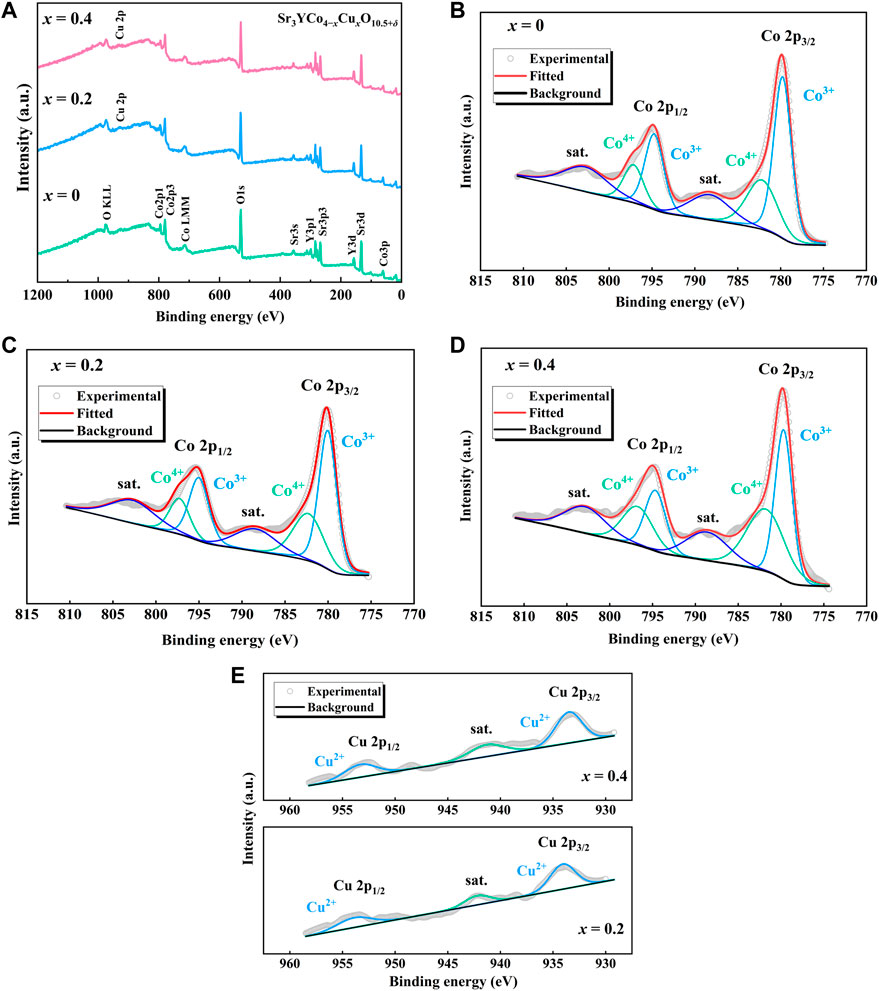
FIGURE 6. (A) Survey spectra of Sr3YCo4−xCuxO10.5+δ samples (x = 0, 0.2, and 0.4). (B–D) Co 2p XPS spectra of Sr3YCo4−xCuxO10.5+δ samples (x = 0, 0.2, and 0.4). (D) Cu 2p XPS spectra of Sr3YCo4−xCuxO10.5+δ samples (x = 0.2 and 0.4).
As shown in Figure 7A, the resistivity-temperature (ρ-T) curves of polycrystalline Sr3YCo4−xCuxO10.5+δ (x = 0–0.6) showed electrical transport characteristics typical of a semiconductor. The resistivity decreased significantly from x = 0 to 0.4 and stabilised from x = 0.4 to 0.6. Figure 7B shows that the room temperature resistivity at 300 K for x = 0.4 and x = 0.6 is 0.0277203 Ω cm and 0.0040672 Ω cm, respectively. Compared with the room temperature resistivity at x = 0 of 0.3403219 Ω cm, the resistivity decreased by 92–99% with Cu doping.
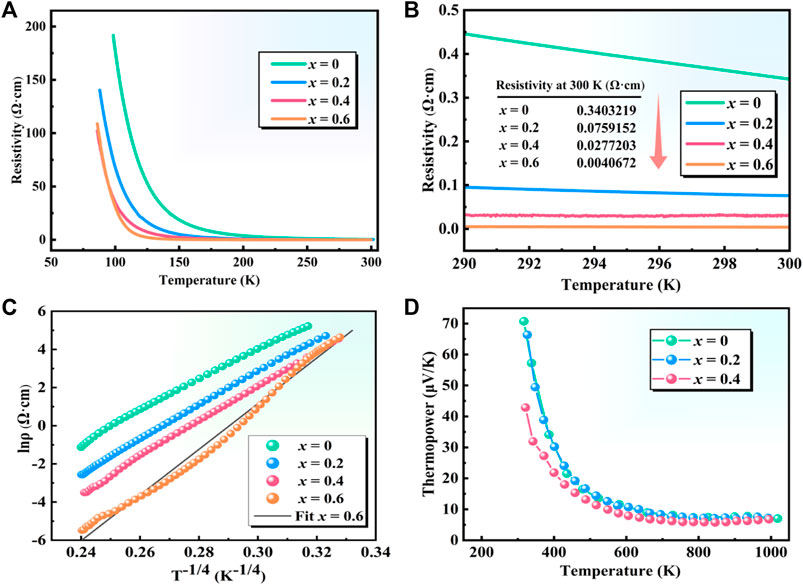
FIGURE 7. ρ-T curves of polycrystalline Sr3YCo4−xCuxO10.5+δ (x = 0–0.6): (A) 50–300 K, (B) 290–300 K. This is a Kelvin temperature unit. (C) lnρ-T−1/4 and (D) S-T curves of polycrystalline Sr3YCo4−xCuxO10.5+δ (x = 0–0.4).
The lnρ-T−1/(n+1) curve was fitted using the Mott variable-range hopping model expressed in Eq. 3 (Mott, 1969):
Here, ρ0 is the initial electrical resistivity (constant), Tm is the characteristic temperature of the variable-range hopping mechanism, and n = 2 or n = 3 is the dimension of the system. Figure 7C shows that when n = 3, lnρ-T−1/4 is almost linear for x = 0–0.04, indicating that a small number of Co4+ ions provide hole carriers (Terasaki et al., 2010), and hopping conduction occurs between Co4+ ions via a three-dimensional variable-range hopping mechanism. A deviation was found between the experimental and fitted curves for the x = 0.6 sample, which may be caused by changes in the resistivity induced by impurity phases produced beyond the limit of solid solubility of Cu in the perovskite structure. When Co3+/4+ was replaced with Cu2+, the concentration of hole carriers increased. In addition, the mobility of the hole carriers increased as the grain size and density of Sr3YCo4−xCuxO10.5+δ increased due to sintering.
The S-T curves of Sr3YCo4−xCuxO10.5+δ (x = 0–0.4) in Figure 7D indicate that the thermopower decreased with increasing temperature. The curves for x = 0 and 0.2 are very similar, and the thermopower of the x = 0.4 material was significantly lower due to the higher carrier concentration. In contrast, increasing the spin entropy of the Co3+ ions increases the thermopower (Yoshida et al., 2009).
The M-T and dM/dT-T curves of polycrystalline Sr3YCo4−xCuxO10.5+δ (x = 0–0.4) are shown in Figures 8A,B. For x = 0, the Hopkinson peak is observed at 319 K in the zero-field-cooling (ZFC) curve. Magnetic moments in the magnetic domains of materials are randomly distributed and frozen at low temperatures, and the net magnetic moments tend to be zero in G-type antiferromagnetic phases (Sheptyakov et al., 2009; Troyanchuk et al., 2009; Troyanchuk et al., 2019). As the temperature increases, the magnetic moments rotate to align along the direction of the external magnetic field, while the magnetic domain wall moves and the magnetic domain grows. When all magnetic moments are aligned in the same direction, the magnetic domain walls disappear, and the magnetisation reaches its maximum at 319 K. At Tc, thermal agitation causes magnetic moments to misalign, resulting in a ferromagnetic-paramagnetic second-order transition and a decrease in magnetisation. There is no resistance between the magnetic domain walls when the field-cooling (FC) curve is recorded, and the magnetic moments are aligned in the same direction under an external magnetic field. Therefore, the maximum magnetisation obtained for the FC curve was higher than that obtained for the ZFC curve. The FC curves had the highest peak at 267 K. As the temperature continues to decrease, a ferromagnetic-antiferromagnetic transition occurs, and a G-type antiferromagnetic phase is generated (Lindberg et al., 2006). The separation of the ZFC and FC curves at low temperature indicates that spin-glass state-like components may be present (Motohashi et al., 2005; Sutjahja et al., 2015; Guo et al., 2018; Srivastava et al., 2020).
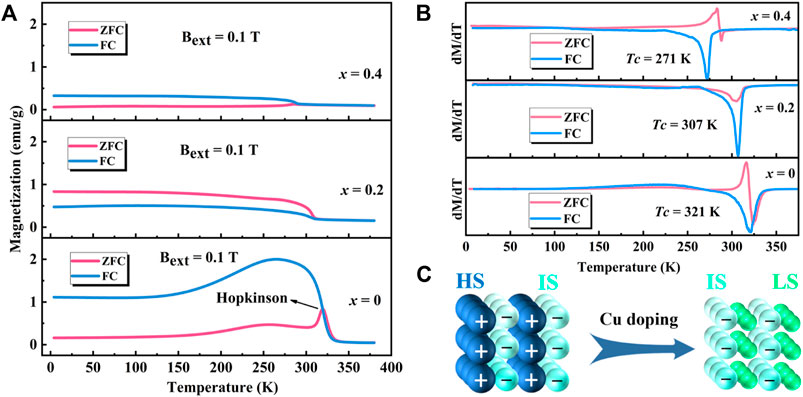
FIGURE 8. Magnetic properties of Sr3YCo4−xCuxO10.5+δ (x = 0–0.4): (A) M-T, (B) dM/dT-T curves, and (C) spin states of Co3+ ions in Sr3YCo4–xCuxO10.5+δ.
For x = 0.2, the maximum magnetisation of the ZFC curve was found at 4 K, attributed to the chemical compressive stress induced by lattice distortion due to Cu doping. It allows most Co3+ ions to transit from a high/intermediate spin (HS/IS, t2g4eg2/t2g5eg1, S = 2/1) to an intermediate/low spin (IS/LS, t2g5eg1/t2g6eg0, S = 1/0), resulting in a decrease in magnetisation (Tsuruta et al., 2020). The Co3+ ions in the LS state are nonmagnetic. At this point, the spin magnetic moments of the Co3+ ions in most regions are aligned in the same direction, as shown in Figure 8C, which is similar to the ferromagnetic structure. The Tc from the ZFC curves decreased from 320 K (x = 0) to 307 K (x = 0.2) and 288 K (x = 0.4), which is related to the decrease in the spin state of Co3+ ions, leading to a decrease in magnetisation and weakening of the magnetic interaction. Moreover, in conjunction with the XPS results, the doping of Cu ions instead of Co ions led to a reduction in Co3+ ion content, which has a direct effect on the reduction of the magnetisation intensity of the sample.
4 Conclusion
A conventional solid-state reaction method was used to synthesise polycrystalline Sr3YCo4−xCuxO10.5+δ (x = 0–0.8). An ordered tetragonal phase and ordering phase transformations due to oxygen uptake above 1,000°C were observed for x = 0–0.4 for the first time. An ordered tetragonal phase and ordering phase transformation due to oxygen uptake above 1,000°C were observed for x = 0–0.4 for the first time. A study of the phase-transformations process during heating provides a research reference for optimising the conditions of the material synthesis process, such as sintering temperature and holding time. Cu doping was observed to significantly reduce the electrical resistivity, and the three-dimensional Mott variable-range hopping conduction mechanism of polycrystalline Sr3YCo4−xCuxO10.5+δ was explored. We also found that Cu doping reduced both the spin state and content of Co3+ ions, thereby inhibiting the room temperature ferromagnetism of 314-SYCO and providing a reference for doping to modulate the properties of oxygen-deficient perovskites.
Data availability statement
The original contributions presented in the study are included in the article/supplementary material, further inquiries can be directed to the corresponding author.
Author contributions
Investigation, Data curation, Writing-original draft, Writing—review and editing, LR; Discussion, XZ, XD, and JW; Conceptualization, Investigation, Writing—review and editing, Supervision, Resources, LY.
Funding
This work was supported by the National Natural Science Foundation of China (grant numbers 51462017 and 51962017).
Conflict of interest
The authors declare that the research was conducted in the absence of any commercial or financial relationships that could be construed as a potential conflict of interest.
Publisher’s note
All claims expressed in this article are solely those of the authors and do not necessarily represent those of their affiliated organizations, or those of the publisher, the editors and the reviewers. Any product that may be evaluated in this article, or claim that may be made by its manufacturer, is not guaranteed or endorsed by the publisher.
References
Delorme, F., Diaz-Chao, P., Guilmeau, E., and Giovannelli, F. (2015). Thermoelectric properties of Ca3Co4O9–Co3O4 composites. Ceram. Int. 41 (8), 10038–10043. doi:10.1016/j.ceramint.2015.04.091
Du, X. L., Yu, L., Zhang, B., Song, S. J., and Li, G. F. (2014). Effects of Cu doping on the microstructure and electrical properties of Sr3YCo4O10.5+δ polycrystalline. Adv. Mat. Res. 934, 80–85. doi:10.4028/www.scientific.net/AMR.934.80
Fukushima, S., Sato, T., Akahoshi, D., and Kuwahara, H. (2009). Order-disorder effect of A-site and oxygen-vacancy on magnetic and transport properties of Y1/4Sr3/4CoO3−δ. J. Phys. Soc. Jpn. 78, 064706–6. doi:10.1143/JPSJ.78.064706
Fukushima, S., Sato, T., Akahoshi, D., and Kuwahara, H. (2008). Comparative study of ordered and disordered Y1−xSrxCoO3−δ. J. Appl. Phys. 103, 07F705–4. doi:10.1063/1.2830615
Golosova, N. O., Kozlenko, D. P., Dubrovinsky, L. S., Drozhzhin, O. A., Istomin, S. Y., and Savenko, B. N. (2009). Spin state and magnetic transformations in Sr0.7Y0.3CoO2.62 at high pressures. Phys. Rev. B 79, 104431–104437. doi:10.1103/PhysRevB.79.104431
Guo, X., Tong, P., Lin, J., Yang, C., Zhang, K., and Lin, S. (2018). Effects of Cr substitution on negative thermal expansion and magnetic properties of antiperovskite Ga1−xCrxN0.83Mn3 compounds. Front. Chem. 6, 75. doi:10.3389/fchem.2018.00075
Hsieh, C. Y., and Fung, K. Z. (2008). Effect of divalent dopants on defect structure and electrical properties of Bi2WO6. J. Phys. Chem. Solids 69, 302–306. doi:10.1016/j.jpcs.2007.07.106
Hu, J. L., Yu, L., Du, X. L., and Song, S. J. (2017). Preparation and properties of room-temperature ferromagnet Sr3YCo4O10.5+δ polycrystals. J. Synth. Cryst. 46, 238–242. (Chinese). doi:10.3969/j.issn.1000-985X.2017.02.008
Istomin, S. Y., Drozhzhin, O. A., Napolsky, P. S., Putilin, S. N., Gippius, A. A., and Antipov, E. V. (2008). Thermal expansion behavior and high-temperature transport properties of Sr3YCo4−xFexO10.5+y, x=0.0, 1.0, 2.0 and 3.0. Solid State Ionics 179, 1054–1057. doi:10.1016/j.ssi.2008.01.017
Istomin, S. Y., Grins, J., Svensson, G., Drozhzhin, O. A., Kozhevnikov, V. L., Antipov, E. V., et al. (2003). Crystal structure of the novel complex cobalt oxide Sr0.7Y0.3CoO2.62. Chem. Mat. 15, 4012–4020. doi:10.1021/cm034263e
Khalyavin, D. D., Chapon, L. C., Suard, E., Parker, J. E., Thompson, S. P., and Yaremchenko, A. A. (2011). Complex room-temperature ferrimagnetism induced by zigzag stripes of oxygen vacancies in Sr3YCo4O10+δ. Phys. Rev. B 83, 140403–140411. doi:10.1103/PhysRevB.83.140403
Kingery, W. D., and Narasimhan, M. D. (1959). Densification during sintering in the presence of a liquid phase. II. Experimental. J. Appl. Phys. 30, 307–310. doi:10.1063/1.1735156
Kishida, T., Kapetanakis, M. D., Yan, J. Q., Sales, B. C., Pantelides, S. T., and Pennycook, S. J. (2016). The origin of magnetic ordering in Sr3YCo4O10+x. Microsc. Microanal. 22, 1394–1395. doi:10.1017/S1431927616007819
Kobayashi, W., Ishiwata, S., Terasaki, I., Takano, M., Grigoraviciute, I., and Yamauchi, H. (2005). Room-temperature ferromagnetism in Sr1−xYxCoO3−δ (0.2≤x≤0.25). Phys. Rev. B 72, 1–5. doi:10.1103/PhysRevB.72.104408
Lalan, V., Puthiyedath Narayanan, A., Surendran, K. P., and Ganesanpotti, S. (2019). Room-temperature ferromagnetic Sr3YCo4O10+δ and carbon black-reinforced polyvinylidenefluoride composites toward high-performance electromagnetic interference shielding. ACS Omega 4, 8196–8206. doi:10.1021/acsomega.9b00454
Li, W., Liang, K., Wang, J., Wen, J., Shi, J., and Zhang, Z. (2022). Effects of Cu doping on electrochemical NOx removal by La0.8Sr0.2MnO3 perovskites. Environ. Res. 210, 112955. doi:10.1016/j.envres.2022.112955
Li, Y., Kim, Y. N., Cheng, J., Alonso, J. A., Hu, Z., and Chin, Y. Y. (2011). Oxygen-deficient perovskite Sr0.7Y0.3CoO2. 65−δ as a cathode for intermediate-temperature solid oxide fuel cells. Chem. Mat. 23, 5037–5044. doi:10.1021/cm202542q
Lindberg, F., Drozhzhin, O. A., Istomin, S. Y., Svensson, G., Kaynak, F. B., and Svedlindh, P. (2006). Synthesis and characterization of Sr0.75Y0.25Co1−xMxO2.625+δ (M=Ga, 0.125≤x≤0.500 and M=Fe, 0.125≤x≤0.875). J. Solid State Chem. 179, 1434–1444. doi:10.1016/j.jssc.2006.01.057
Lu, Y., Chen, L., Lu, C., Ni, Y., and Xu, Z. (2013). Effects of oxygen defects on structure and properties of Sm0.5Sr0.5CoO3-δ annealed in different atmospheres. J. Rare Earths 31, 1183–1190. doi:10.1016/s1002-0721(12)60424-4
Marik, S., Mohanty, P., Singh, D., and Singh, R. P. (2018). Moderate magnetic field induced large exchange bias effect in ferrimagnetic 314—Sr3YCo4O10.5 material. J. Phys. D. Appl. Phys. 51 (6), 065006. doi:10.1088/1361-6463/aaa452
Motohashi, T., Caignaert, V., Pralong, V., Hervieu, M., Maignan, A., and Raveau, B. (2005). Competition between ferromagnetism and spin glass: The key for large magnetoresistance in oxygen-deficient perovskitesSrCo1−xMxO3−δ(M=Nb, Ru). Phys. Rev. B 71, 214424. doi:10.1103/physrevb.71.214424
Mott, N. F. (1969). Conduction in non-crystalline materials: III. Localized states in a pseudogap and near extremities of conduction and valence bands. Philos. Mag. 19, 835–852. doi:10.1080/14786436908216338
Ptáček, P., Bartoníčková, E., Švec, J., Opravil, T., Šoukal, F., and Frajkorová, F. (2015). The kinetics and mechanism of thermal decomposition of SrCO3 polymorphs. Ceram. Int. 41 (1), 115–126. doi:10.1016/j.ceramint.2014.08.043
Rajan, A., and Subodh, G. (2020). Crystal structure, microstructure, and broadband electromagnetic response of Al3+-substituted Sr3YCo4O10+δ double perovskites. Ceram. Int. 46 (16), 25683–25690. doi:10.1016/j.ceramint.2020.07.044
Rupasov, D., Chroneos, A., Parfitt, D., Kilner, A., Grimes, W., and Istomin, S.-Y. (2009). Oxygen diffusion in Sr0.75Y0.25CoO2.625: A molecular dynamics study. Phys. Rev. B 79, 172102–172109. doi:10.1103/PhysRevB.79.172102
Sheptyakov, D. V., Pomjakushin, V. Y., Drozhzhin, O. A., Istomin, S. Y., Antipov, E. V., and Bobrikov, I. A. (2009). Correlation of chemical coordination and magnetic ordering in Sr3YCo4O10.5+δ (δ=0.02 and 0.26). Phys. Rev. B 80, 024409. doi:10.1103/PhysRevB.80.024409
Srivastava, A., Singh, A. K., Srivastava, O. N., Tewari, H. S., Masood, K. B., and Singh, J. (2020). Magnetic and dielectric properties of La and Ni Co-substituted BiFeO3 nanoceramics. Front. Phys. 8. doi:10.3389/fphy.2020.00282
Sutjahja, I. M., Berthalita, F., Mustaqima, M., Nugroho, A. A., and Tjia, M. O. (2015). Effects of partial Co replacement by Fe in Sr0.775Y0.225CoO3-δ on its magnetic property, oxygen deficiency and crystal structure. Mater. Pol. 33, 579–587. doi:10.1515/msp-2015-0078
Terasaki, I., Iwakawa, M., Nakano, T., Tsukuda, A., and Kobayashi, W. (2010). Novel thermoelectric properties of complex transition-metal oxides. Dalton Trans. 39, 1005–1011. doi:10.1039/b914661j
Troyanchuk, I. O., Bushinsky, M. V., Tereshko, N. V., Lanovsky, R. A., Sikolenko, V. V., and Ritter, С. (2019). Ferromagnet-antiferromagnet transition in layered perovskites of Sr3YCo4O10.5 type. Mat. Res. Express 6, 026105. doi:10.1088/2053-1591/aaef21
Troyanchuk, I. O., Karpinsky, D. V., Dobryanskiĭ, V. M., Chobot, A. N., Chobot, G. M., and Sazonov, A. P. (2009). Magnetic transformations in the Sr0.78Y0.22Co1−xFexO3−γ system with a perovskite structure. J. Exp. Theor. Phys. 108, 428–434. doi:10.1134/S1063776109030078
Tsuruta, A., Kawasaki, S., Mikami, M., Kinemuchi, Y., Masuda, Y., and Fujita, A. (2020). Co-substitution effect in room-temperature ferromagnetic oxide Sr3.1Y0.9Co4O10.5. Mater. (Basel) 13, 2301. doi:10.3390/ma13102301
Vinila, V. S., and Isac, J. (2022). Synthesis and structural studies of superconducting perovskite GdBa2Ca3Cu4O10.5+δ nanosystems. Des. Fabr. Charact. Multifunct. Nanomater., 319–341. doi:10.1016/b978-0-12-820558-7.00022-4
Wu, Y., Li, L., Chu, B., Yi, Y., Qin, Z., and Fan, M. (2018). Catalytic reduction of NO by CO over B-site partially substituted LaM0.25Co0.75O3 (M = Cu, Mn, Fe) perovskite oxide catalysts: The correlation between physicochemical properties and catalytic performance. Appl. Catal. A General 568, 43–53. doi:10.1016/j.apcata.2018.09.022
Yoshida, S., Kobayashi, W., Nakano, T., Terasaki, I., Matsubayashi, K., and Uwatoko, Y. (2009). Chemical and physical pressure effects on the magnetic and transport properties of the A-site ordered perovskite Sr3YCo4O10.5. J. Phys. Soc. Jpn. 78, 094711–094715. doi:10.1143/JPSJ.78.094711
Keywords: ordered phase transformation, electrical transport, room-temperature ferromagnetism, ceramics, Cu content
Citation: Ren L, Zhang X, Du X, Wang J and Yu L (2022) Ordered phase transformation and Cu doping effects in room-temperature ferromagnetic Sr3YCo4O10.5+δ. Front. Chem. 10:1073946. doi: 10.3389/fchem.2022.1073946
Received: 19 October 2022; Accepted: 21 November 2022;
Published: 08 December 2022.
Edited by:
Muhammad Asif, Wuhan Institute of Technology, ChinaReviewed by:
Tieqiao Chen, Hainan University, ChinaMazhar Ul-Islam, Dhofar University, Oman
Mudasir Nazar, Jiangsu University, China
Copyright © 2022 Ren, Zhang, Du, Wang and Yu. This is an open-access article distributed under the terms of the Creative Commons Attribution License (CC BY). The use, distribution or reproduction in other forums is permitted, provided the original author(s) and the copyright owner(s) are credited and that the original publication in this journal is cited, in accordance with accepted academic practice. No use, distribution or reproduction is permitted which does not comply with these terms.
*Correspondence: Lan Yu, eXVsYW4wMDBAaG90bWFpbC5jb20=