- 1School of Mechanical Engineering, Chengdu University, Chengdu, China
- 2School of Materials Science and Engineering, Northwestern Polytechnical University, Xi’an, China
- 3Institute of Process Engineering, Chinese Academy of Sciences, Beijing, China
- 4Institute of Botany, Jiangsu Province and Chinese Academy of Sciences, Nanjing, China
- 5Jiangsu Key Laboratory for the Research and Utilization of Plant Resources, Jiangsu, China
The practical application of splitting water to generate hydrogen is to a large extent hindered by an oxygen evolution reaction (OER) process. Electrocatalysts with low-cost, high activity, and durability are essential for the low kinetic threshold of the OER. Despite the high active performances of noble metal compound electrocatalysts like IrO2 and RuO2, they are heavily restricted by the high cost and scarcity of noble metal elements. In this context, noble-metal-free electrocatalysts have acquired increasing significance in recent years. So far, a broad spectrum of noble-metal-free electrocatalysts has been developed for improved OER performance. In this review, three types of electrolysis and some evaluation criteria are introduced, followed by recent progress in designing and synthesizing noble-metal-free alkaline OER electrocatalysts, with the classification of metal oxides/(oxy)hydroxides, carbon-based materials, and metal/carbon hybrids. Finally, perspectives are also provided on the future development of the alkaline OER on active sites and stability of electrocatalysts.
1 Introduction
Splitting water to generate H2 and O2, through the hydrogen evolution reaction (HER) and oxygen evolution reaction (OER), is regarded as an ideal solution to the energy shortage and climate problems. (Doyle and Lyons, 2016; Liu et al., 2018; Lyu et al., 2019) Benefitting from its high-density energy and non-polluting nature, H2 is usually acknowledged to be the ultimate clean energy. (Hunter et al., 2016; Zuo et al., 2019; Park et al., 2022) However, the realization of large-scale production of H2 is heavily dependent on the development of low-cost alternative technologies. Steam reforming of natural gas can be used for large-scale production of H2 (Wu et al., 2020; Ďurovič et al., 2021), but this method is unsustainable and harmful to the environment. Alternatively, the water electrolysis produces hydrogen gas in a renewable and potentially cost-effective way, which utilizes electric power to split the oxygen and hydrogen bond in a water molecule. It is an especially attractive solution for H2 production compared to the use of steam reforming of natural gas. (Jamesh and Harb, 2021) According to the type of ions transporting through the electrolyte and operating temperature, three main types (Plevová et al., 2021) of the water electrolysis process are distinguished: high-temperature solid oxide steam electrolysis, proton exchange membrane (PEM) electrolysis, and anion exchange membrane (AEM) electrolysis (Carmo et al., 2013; Peng et al., 2016; Reier et al., 2017; Xu et al., 2018; Todoroki and Wadayama, 2019; Li et al., 2021).
1.1 High-temperature solid oxide steam electrolysis
Solid oxide steam electrolysis exhibits high performance in the absence of noble-metal catalysts like platinum. Nevertheless, this is at the cost of the high energy consumption and strict material requirements, thus becoming the least-used technology among the three types mentioned in this study. (Hauch et al., 2008)
1.2 PEM water electrolysis
PEM electrolyzers use proton exchange membranes to separate the gas products. (Carmo et al., 2013) It is a highly flexible, intensive, and compact technology with higher current density, lower Ohmic losses, and a larger partial load range in comparison to alkaline electrolyzers. Nevertheless, the high cost of PEM and the narrow choice of available catalysts hinder its widespread application. (Eftekhari, 2017a)
1.3 AEM water electrolysis
In contrast to the PEM process, the AEM process performs in an alkaline environment (Figure 1), which could circumvent the limitations of the PEM system and enable the use of cheap catalysts like metal oxides. (Xu et al., 2018) Consequently, AEM is by far the most prevailing technology because of its low cost and a variety of available catalysts, making it an attractive candidate for large-scale commercial applications, e.g., in hydrogen production. (Pletcher and Li, 2011)
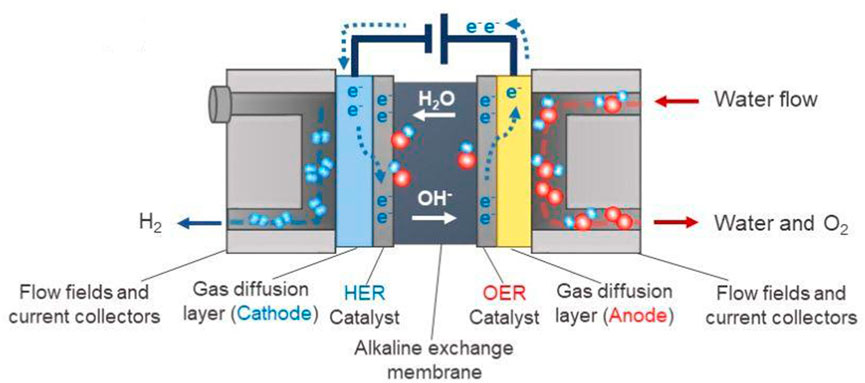
FIGURE 1. Schematic diagram of an AEM process system. (Xu et al., 2018) Copyright 2018, American Chemical Society.
AEM is an attractive option because of its well-matured technology in the hydrogen economy scheme, which however AEM technology needs further improvement to become competitive with the PEM process. This goal creates some important research fields of AEM and leads to the development of increasingly more active catalysts. (Ďurovič et al., 2021) Therefore, this review specifically focuses on the catalysts for an OER in the AEM process. In the following section, fundamental electrochemistry and evaluation criteria of an OER in the AEM process will be discussed.
1.4 Fundamental electrochemistry of an OER in alkaline environments
Under alkaline conditions, the overall water oxidation reaction is (Liang et al., 2021)
where (g) refers to the gas phase and (l) the liquid phase.
The OER process in an alkaline medium is considered to proceed as the following steps (Zhou et al., 2017):
where ∗ represents the catalyst active site and ∗OH, ∗O, and ∗OOH represent the species adsorbed on the active site.
1.5 Evaluation criteria of OER catalysts
It is essentially important to establish standards for evaluating the performance of OER electrocatalysts. Here, we summarized some well-established criteria including overpotential (η), onset potential, turnover frequency (TOF), Faradic efficiency, Tafel slope (b), stability, and activity. (Tahir et al., 2017; Lyu et al., 2019)
1.5.1 Overpotential
The overpotential critically determines the extra energy consumption and energy conversion efficiencies. Low overpotential is a noteworthy quality of OER catalysts for reducing these parameters. (Wei and Xu, 2018)
where
Here, j represents the corresponding current density, jo represents the exchange current density, and α represents exchange coefficients, and generally, α ≈ 1/2. (Naimi and Antar, 2018)
1.5.2 Tafel slope
Another significant parameter is the Tafel slope (b), which can be calculated from the equation (Park et al., 2022). A high Tafel slope means a rapid increase of overpotential with the current density, indicating inferior OER kinetics of the electrocatalysts.
1.5.3 Faradic efficiency
Faradic efficiency (FE) is the ratio of the amount of target gas detected in the experiment to the amount of gas calculated. (Sun et al., 2022) Faradic efficiency can be calculated as follows:
where F represents Faraday’s constant,
1.5.4 Turnover frequency
The TOF for the OER is the average number of moles of O2 evolved per active site and time unit. (Ďurovič et al., 2021) It is also an important parameter to estimate the OER catalysts. The calculation of TOF is demonstrated as follows:
Here, j is the current density, S represents the electrode area, and n represents the number of active sites.
Active sites normally originate from the surface self-reconstruction during the dynamic catalysis process. (Fabbri et al., 2017) However, it is difficult to determine the precise number of active sites in practice. The evaluation of the number of active sites by measuring the total active species often leads to overestimated results as inert species are not excluded. Despite the advances in the current understanding of reaction mechanisms, the exact mechanism involved in a catalysis process is still insubstantial. A combination of various in situ techniques and theoretical calculations may be helpful to better understand the reaction mechanism and the true active sites.
1.5.5 Electrolyte
Acidity and basicity of the electrolyte exert considerable influence on the performance of OER electrocatalysts. So far, as reported in literatures, alkaline electrolytes are the most favorable for most OER electrocatalysts, followed by neutral electrolytes, and the least favorable for acidic electrolytes. (Tahir et al., 2017; Cai et al., 2019; Zeradjanin et al., 2021; Kim et al., 2022) At present, most researchers focus on designing and synthesizing electrocatalysts which are stable in an alkaline electrolyte. Nevertheless, most of these catalysts cannot resist the high oxidative potential under acidic conditions. Therefore, it is of great importance to develop OER electrocatalysts that can perform over a wide pH range.
1.5.6 Stability
It is essential to evaluate the stability of electrocatalysts toward practical applications. An electrolyte plays an important role in the stability of catalysts as most catalysts are apt to be corrupted in an acidic medium but can perform well in a basic medium. The stability of catalysts is also affected by a working electrode in most cases, and typically catalysts fabricated on the working electrode directly show higher stability. It is questionable whether the reported stability in the literature meets the requirements of real devices. The recently developed techniques which employed ex situ and in situ XRD, TEM, SEM, and XPS for monitoring the structure evolution of catalysts might be the answer to the problem.
The OER is a key step in the AEM process, but it is kinetically sluggish due to its four-electron transfer process. (Xie et al., 2022) In order to maintain the momentum of future advances of water splitting, the development of high-performance OER electrocatalysts is necessary. RuO2 and IrO2 are at present the ideal OER catalysts because of their high catalytic activity both in an acidic and alkaline environment. Nevertheless, the scarcity of Ir and Ru elements inducing the large-scale production of RuO2 and IrO2 in an OER runs into bottlenecks. (Ďurovič et al., 2021) For the last few years, the search for more abundant and lower cost alternatives to the noble-metal-based catalysts has stimulated an influx of research into this field and leads to significant advances. (Doyle and Lyons, 2016; Eftekhari, 2017b; Stelmachowski et al., 2021) For instance, some noble-metal-free-based OER electrocatalysts like transition metal-based materials (Huynh et al., 2015; Gao et al., 2021; Wang et al., 2021; Wang et al., 2022) and carbon-based materials (Gao et al., 2019) are recently reported to perform well in both acidic and alkaline conditions. The encouraging results shows that some noble-metal-free-based electrocatalysts exhibit a remarkable catalytic activity in alkaline conditions and sometimes are superior to noble-metal-based electrocatalysts (Lu et al., 2017; Lu et al., 2019). For this reason, this review aims to briefly summarize the recent advances in noble-metal-free electrocatalysts for an alkaline OER process.
2 Noble-metal-free electrocatalysts for the OER in an alkaline electrolyte
2.1 Non-precious metal oxide/(oxy)hydroxide materials
Noble-metal-free metal oxides/(oxy)hydroxides attracted great interest due to their outstanding stability and abundance (Marschall and Wang, 2014). They can be prepared and/or obtained directly from metal X-ides (X-: sulf-, nitr-, carb-, chalcogen-, etc.) during an OER process. (Ding et al., 2019; Huang et al., 2019; Zuo et al., 2019; Xie et al., 2022) In alkaline electrolytes, iron oxides/hydroxides, cobalt oxides/hydroxides, and nickel oxides/hydroxides have been studied extensively among the earth-abundant metals, which thus will be emphasized in the following.
Iron oxides are promising electrocatalysts due to the low cost and abundance of iron and have been studied extensively. (Hunter et al. 2016) found that OER activity of Fe2O3 nanostructures depends on the number of Fe edge-site atoms. (Kauffman et al., 2019) Edge-site located Fe atoms were recognized as the main reaction centers, and the OER turnover frequencies produced by an edge-site located Fe is approximately 150 times greater than that of Fe atoms on the surface (Figure 2). Furthermore, by using DFT calculations, more advantageous edge site-based OERs were revealed, which have lower predicted overpotentials benefited from the modification of intermediate binding. Boettcher et al. reported a new OER catalytic model of iron (oxy) hydroxide in alkaline media. (Zou et al., 2015) The catalyst dissolution rate is low at overpotentials of about 350 mV. Mass loading influences the OER current density to a great extent, and the AuOx/Au substrate can greatly improve the catalytic activity. The choice of the substrate has little influence on the activity, and a linear relationship between the current density and loading is identified. (Zou et al., 2015) J. Xu and co-workers designed and successfully synthesized ferromagnetic Co3−xFexO4 spinels using sulfurization. (Wu et al., 2021) Benefitting from limited oxyhydroxide layer and the stable configuration of Co3−xFexO4/Co(Fe)OxHy, the reconstructed Co(Fe)OxHy owns an order of magnitude higher catalytic activity than the directly synthesized Co (Fe) oxyhydroxides. (Wu et al., 2021)
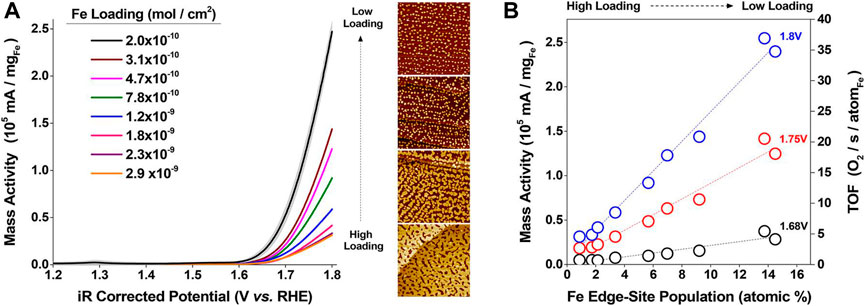
FIGURE 2. (A) OER voltammograms of 2L-Fe2O3/Au under different loadings in N2 purged 0.1 M KOH (Kauffman et al., 2019). (B) TOF and mass activity versus the relative population of edge-site located Fe atoms (Kauffman et al., 2019). Copyright 2019, American Chemical Society.
Cobalt oxides have been used as water oxidation catalysts for more than 70 years. (Hunter et al., 2016; Yu et al., 2022) Co2O3, CoO2, and CoO were first found feasible under anodic bias by initial electrochemical characterization in alkaline conditions. (El Wakkad and Hickling, 1950) Some newly developed structures have shown greater prospects, such as Co3O4. (Dangwal Pandey et al., 2012) Dismukes and co-workers synthesized lithium cobalt oxide having two polymorphs. The results revealed that the existence of a cubic core of Co4O4 indicates the activity of water oxidation. (Gardner et al., 2012) The synthesized pure cubic 400-Li2Co2O4 per bulk cobalt atom has a TOF value of 1.0 × 10−3 s−1 (Figures 3A,B). The TOF has reached 1.9 × 10−3 s−1 after normalization to the amount of cobalt that is accessible on the surface. The electrochemical characterization of membrane electrode assemblies (MEAs) reveals that the current density of the MEA containing cubic 400-Li2Co2O4 is 50 times higher than of the current for the MEA only with Nafion (Figure 3C). Wang et al. successfully synthesized Co3O4 nanoparticles with controllable size and found that the absence of ligands can lead to high activity. (Grzelczak et al., 2013) The tendency that the activity increases with the decrease in particle size reported in the article explicitly demonstrated that water oxidation was primarily dependent on the availability of the surface cobalt atoms. (Grzelczak et al., 2013) Müller et al. successfully synthesized composition- and size-controlled Co3O4 nanoparticles without surfactants. (Blakemore et al., 2013) The obtained cobalt oxide nanoparticles delivered the highest TOF (0.21 mol O2 (mol Cosurface)−1 s−1), and the overpotential of their Co3O4 nanoparticles obtained is 314 mV at 0.5 mA cm−2. Meanwhile, the mass activity of 10 A m−2g−1 is achieved at 500 mV overpotential. (Blakemore et al., 2013) More recently, Yeo et al. reported an iron (III) ion-adsorbed amorphous cobalt oxide as high-efficient OER catalysts. (Gong et al., 2017) The synergetic effect of Fe3+ and CoOx reduces the overpotential of up to 69 mV at 10 mAcm−2. The catalyst has a low overpotential of only 309 mV and can catalyze the OER process at 10 mAcm−2. The stability of the obtained catalyst was enhanced compared with that of the CoOx catalyst, and the Tafel slope of the synthesized catalyst was 27.6 mVdec−1. (Gong et al., 2017)
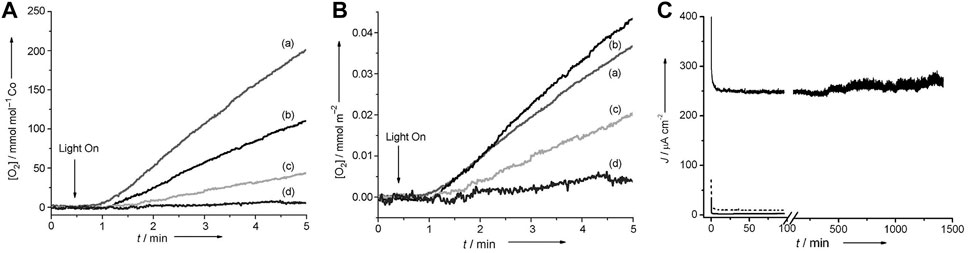
FIGURE 3. (A) Concentration of dissolved O2 measured by a Clark-type electrode at 23°C, pH 5.8 for lithium cobalt oxides synthesized at (A) 400°C, (B) 500°C, (C) 600°C, and (D) 700°C, and (B) the total surface area of a synthesized catalyst. (C) Bulk electrolysis of the Nafion-only control (solid gray line), 700-LiCoO2 (dashed gray line), and 400-Li2Co2O4 (solid black line). (Gardner et al., 2012) Copyright 2012, Wiley-VCH.
In 1966, nickel oxides were first identified as water oxidation catalysts by Bode et al. (1966). Also, further deep studies were made in the following two decades (Oliva et al., 1982). Owing to the high OER catalytic activity in alkaline media, the application of nickel oxides in electrocatalysis has attracted great attention recently (Yu et al., 2022) (Młynarek et al., 1984). A detailed study of iron-doped nickel oxides by Boettcher et al. Trotochaud et al. (2014) underscored the essentiality of impurity influences on the performance of electrocatalysts (Figure 4). (Trotochaud et al., 2014) Lyons and co-workers investigated the mechanisms of nickel oxide electrodes and revealed the importance of Ni (III) or Ni (IV) species and proposed a more general physisorbed peroxide mechanism. (Lyons and Brandon, 2008) They used the electrode as the hole acceptor throughout instead of invoking multiple oxidation states of nickel. (Lyons and Brandon, 2008) Ma et al. reported a high catalytic active single-atom W6+-doped α-Ni(OH)2 OER electrocatalyst. (Yan et al., 2019) Overpotential values of 237 mV and 267 mV were obtained at 10 mAcm−2 and 80 mAcm−2, respectively. Also, a low Tafel slope of 33 mVdec−1 was obtained in a 1 M KOH electrolyte. (Yan et al., 2019)
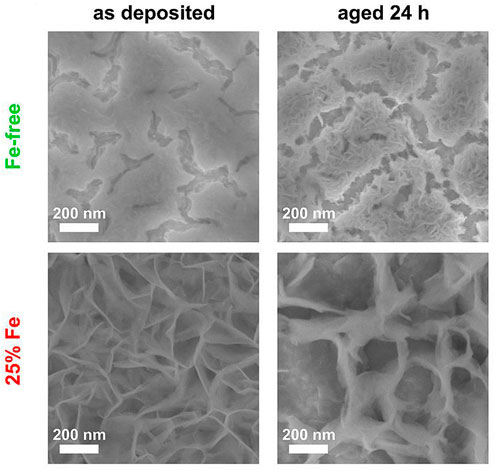
FIGURE 4. SEM images of synthesized Ni(OH)2 (left) and Ni0.75Fe0.25(OH)2 (right) catalysts as-deposited and after 24 h of aging in 40°C 1 M KOH. (Trotochaud et al., 2014) Copyright 2014, American Chemical Society.
2.2 Metal-free carbon-based materials
Carbon-based metal-free electrocatalysts have been considered promising OER catalysts because of their intriguing properties like nature abundance and chemical stability. (An et al., 2022) In 2009, carbon nanotubes doped with nitrogen were found to have excellent OER catalytic activity without fuel crossover and poisoning in alkaline media. (Gong et al., 2009) More recently, these new types of catalysts were discovered to be superior to metal catalysts for the OER. (Zhang et al., 2015)
Dai and co-workers successfully synthesized a high-efficiency electron-acceptor by absorbing buckminsterfullerene (C60) onto single-walled carbon nanotubes (SWCNTs). (Gao et al., 2019) The obtained metal-free C60-SWCNT electrocatalysts were shown to be excellent for the OER process over a wide pH range from acidic to alkaline. (Gao et al., 2019) The current density (10 mAcm−2) of C60-SWCNT is 50, 5.3, and 1.2 times higher than that of the pure C60, SWCNTs and commercial RuO2 at 1.69 V potential, respectively. (Gao et al., 2019) Wu et al. synthesized a carbon quantum dot/graphene composite electrocatalyst with a simple novel two-step procedure (Figure 5A). (Zhao et al., 2019a) The corresponding Tafel slope of the obtained hybrid is 44 mV dec−1. Moreover, the stability test of obtained hybrids was carried out by CV scanning for 2000 cycles, and negligible change was observed. Such good electrocatalytic performance is attributed to various defect sites exposed by small-sized graphene flakes and carbon quantum dots, as well as the fast charge transfer rate and high active surface area. (Zhao et al., 2019a) Dai et al. reported an easy method with mass production to synthesize N and P co-doped 3D mesoporous carbon foams as bifunctional electrocatalysts for oxygen reduction and OER (Figure 5B). (Zhang et al., 2015) The obtained catalyst demonstrated lower onset potentials and higher currents than those of the Pt/C electrode. Furthermore, the material exhibits a lower onset potential than the RuO2 nanoparticle reference. Wang et al. successfully introduced the sp-hybridized nitrogen (sp-N) into graphdiyne, which exhibits potential for the OER. (Zhao et al., 2019b) In their research, heteroelements N and S were introduced into few-layer acetylenic groups in graphdiyne by a one-pot facile method, and the ratios of the N atoms and the S atoms were able to be tuned. (Zhao et al., 2019b) The heteroelement co-doped sample exhibits catalytic activity to those individually doped (either N or S doping) samples and most reported metal-free catalysts. (Zhao et al., 2019b) L. Xin and co-workers built a large library of single-atom catalysts by characterization and analysis of 37 monometallic elements. (Han et al., 2022) By employing an in situ method, they presented a unified set of principles on the state of oxidization, the number of coordinates, the length of the bond, and the coordination element of single atoms for the preparation of single-atom catalysts. They utilized the built single-atom-catalyst library to create complex multi-metallic single-atom phases with up to 12 different elements. (Han et al., 2022) The synthesized 12-metal single-atom catalyst exhibits higher current density, mass activity, and turnover frequency than those of the mixed and individual monometallic single-atom catalysts at the same potentials. (Han et al., 2022) OER catalysis of the 12-metal single-atom catalyst was performed at a constant current of 10 mAcm−2 for 130 h, and no appreciable potential change was observed.
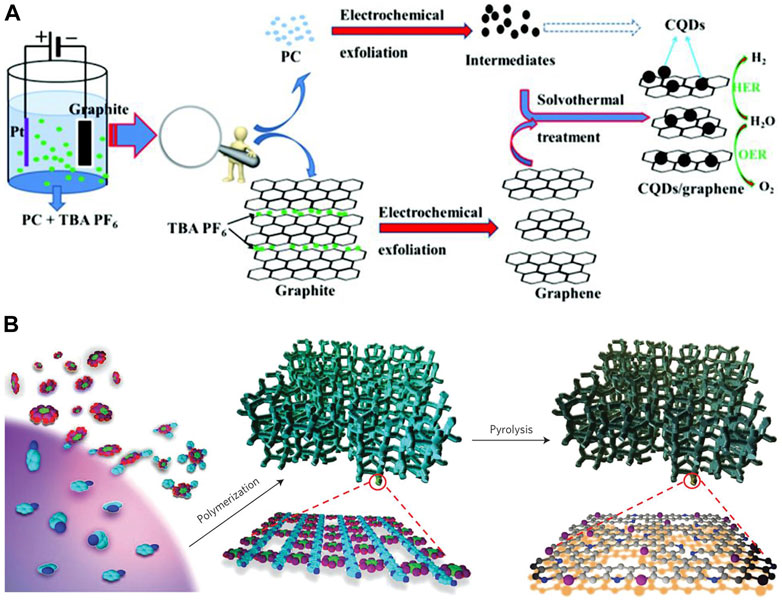
FIGURE 5. (A) Probable formation route of the carbon quantum dot/graphene heterostructure. (Zhao et al., 2019a) Copyright 2019, the Royal Society of Chemistry. (B) Preparation of porous carbon co-doped with N and P electrocatalysts. (Zhang et al., 2015) Copyright 2015, Macmillan Publishers Limited.
2.3 Non-precious metal and carbon hybrids
The poor stability and low conductivity of transition metals (TMs) have severely impeded their use in large-scale H2 production. As is known, carbon nanotubes and graphites have remarkable conductivity and good chemical/thermal stability. The integration of carbon nanomaterials with TM could, therefore, circumvent the aforementioned issues on TM-based electrocatalysts (Figure 6). (Hou et al., 2015; Wang et al., 2015; Jiang et al., 2018; Hu et al., 2019) Therefore, TM/carbon hybrids are emerging as new efficient OER electrocatalysts. Usually, these composites demonstrated both the attributes of the respective components (e.g., TM or carbon) and novel promising properties achieved through the combination of carbon nanomaterials and TM, such as Ni@ nitrogen-doped graphene [Mo3S13]2-@ graphene. (Xu et al., 2017; Ouyang et al., 2018) The new attributes of these hybrids make them promising alternatives for the next-generation, low-cost, and efficient OER catalysts rather than a precious metal electrochemical catalyst.
Zhang and co-workers successfully achieved a mesoporous polyhedral structure of graphite carbon enclosing CoTe2 nanocrystals based on a single heat treatment involving tellurizing and carbonizing simultaneously. (Liu et al., 2017) The texture of the hybrid provides a simple path for the OER with increased activity and strong stability. The hybrid showed an overpotential of 300 mV at 10 mAcm−2. Moreover, the graphitic carbon matrix provides a high electrical conductivity and rich access to active sites besides interfacing with the confined nanocrystalline CoTe2 in an intensive way for the enhancement of the OER. (Liu et al., 2017) Huang and co-workers proposed a general and rational strategy for a group of monodispersed TMs embedded in graphene. (Fei et al., 2018) They have established that the resulting M@graphene (M = Fe, Co, Ni) hybrids lead to minimum distortion of the 2D graphene lattice. More importantly, the existence of the well-defined MN4C4 moieties in different holey graphene frameworks provides a desirable model system for determining and quantifying the relationship between the atomistic structure of the metal centers and its catalytic properties. Owing to the high activity of the intrinsic activity of Ni embedded in nitrogen-doped holey graphene frameworks, the density of the active sites increases as the metal loading increases. (Fei et al., 2018) Zhu et al. developed an effective bifunctional electrocatalyst based on porphyra-derived S-doped Fe-N-C. (Zhang et al., 2019) The resultant hybrid exhibits a low Tafel slope of 59 mV dec−1 and an overpotential of 410 mV at 10 mAcm−2 in a 0.1 M KOH medium. Further investigation revealed that S-doping could optimize the charge and spin distribution of the Fe–N–C hybrid, resulting in good activities in the OER, which mainly attributes to the Fe-Nx and Fe-N3|S sites individually. (Zhang et al., 2019) G. Gomes and co-workers designed an easy one-step sustainable protocol to synthesize nickel/nickel oxide@carbon bifunctional electrocatalysts from the wastes of cauliflower leaves. The electrocatalytic performance of the resultant hybrid was found dependent on the pyrolysis temperature. (Hoang et al., 2020) At 10 mA cm−2, the obtained hybrids require overpotentials of 346 mV and moderate Tafel slopes of 70 mV dec−1 for an OER in a 0.1 M KOH electrolyte. The synthesized electrocatalyst needs only 1.688 V to reach a current density of 10 mA cm−2 when incorporated into a two-electrode water electrolyzer. Medeiros et al. reported on the synthesis, structural, morphological, and electrochemical characterization of Ni-NiO/carbon nanofibers for an OER catalytic process by solution blow spinning. (Silva et al., 2021) The XPS analysis demonstrated that a small fraction of the species of nickel present in the fiber surface is sufficient to promote a good performance in the OER process. The performance of the Ni-NiO/C_An electrode was not affected although the size of the nanoparticles slightly exceeds that of the electrode. (Silva et al., 2021) Also, the active species of Ni3+ were found to be the main factor affecting the catalysis performance.
3 Summary and perspectives
It is essential to identify the active sites and their coordination structure for OER catalysts as those attributes are closely associated with the catalyst activity and stability. Several major types of active sites are classified: defects, electron deficient site, metal center, etc. The debate over the active site of electrocatalysts underlines the complication of recognizing the real active sites despite various experimental techniques and computational methods being employed. One possibility is to use an in situ technique to monitor catalyst evolution during the OER to provide significant insight into active sites.
Material instability and operational instability are considered the two main types of catalyst instability that occurred in the catalytic system and have great influences on the stable catalytic action. Material instability is usually caused by material corrosion regardless of the test conditions. Future OER catalyst applications require the development of materials that can be operated under severe working conditions. For metal oxides, material stability can be improved through structure design and composition adjustment. For carbon-based catalysts, material stability can possibly be improved by increasing the degree of graphitization. Operational instability arises during electrochemical tests and is usually caused by potential-induced element dissolution and/or structure damage. One possible way to increase the operational instability is to use lower operation potential. However, low operation potential needs substantial improvements in the catalyst activity; hence, the design of a high active OER is highly desirable for the future commercial development of water electrolysis.
Author contributions
DT and FX contributed to the conception and design of the manuscript. DT and HX organized the database. DT wrote the whole draft of the manuscript. All authors contributed to manuscript revision, read, and approved the submitted version.
Conflict of interest
The authors declare that the research was conducted in the absence of any commercial or financial relationships that could be construed as a potential conflict of interest.
Publisher’s note
All claims expressed in this article are solely those of the authors and do not necessarily represent those of their affiliated organizations, or those of the publisher, the editors, and the reviewers. Any product that may be evaluated in this article, or claim that may be made by its manufacturer, is not guaranteed or endorsed by the publisher.
References
An, F., Bao, X., Deng, X., Ma, Z., and Wang, X. (2022). Carbon-based metal-free oxygen reduction reaction electrocatalysts: Past, present and future. Carbon N. Y. 37, 1–54. doi:10.1016/j.carbon.2022.04.053
Blakemore, J. D., Gray, H. B., Winkler, J. R., and Müller, A. M. (2013). Co3O4 nanoparticle water-oxidation catalysts made by pulsed-laser ablation in liquids. ACS Catal. 3, 2497–2500. doi:10.1021/cs400639b
Bode, H., Dehmelt, K., and Witte, J. J. (1966). Zur kenntnis der nickelhydroxidelektrode—I. Über das nickel (II)-hydroxidhydrat. Electrochim. Acta 11, 1079–1087. doi:10.1016/0013-4686(66)80045-2
Cai, Z., Bu, X., Wang, P., Ho, J. C., Yang, J., and Wang, X. (2019). Recent advances in layered double hydroxide electrocatalysts for the oxygen evolution reaction. J. Mat. Chem. A Mat. 7, 5069–5089. doi:10.1039/c8ta11273h
Carmo, M., Fritz, D. L., Mergel, J., and Stolten, D. (2013). A comprehensive review on PEM water electrolysis. Int. J. Hydrogen Energy 38, 4901–4934. doi:10.1016/j.ijhydene.2013.01.151
Dangwal Pandey, A., Jia, C., Schmidt, W., Leoni, M., Schwickardi, M., Schüth, F., et al. (2012). Size-controlled synthesis and microstructure investigation of Co3O4 nanoparticles for low-temperature CO oxidation. J. Phys. Chem. C 116, 19405–19412. doi:10.1021/jp306166g
Ding, X., Li, W., Kuang, H., Qu, M., Cui, M., Zhao, C., et al. (2019). An Fe stabilized metallic phase of NiS 2 for the highly efficient oxygen evolution reaction. Nanoscale 11, 23217–23225. doi:10.1039/c9nr07832k
Doyle, R. L., and Lyons, M. E. G. (2016). “The oxygen evolution reaction: Mechanistic concepts and catalyst design,” in Photoelectrochemical solar fuel production Midtown Manhattan, NY, USA: Springer Cham, 41–104. doi:10.1007/978-3-319-29641-8_2
Ďurovič, M., Hnát, J., and Bouzek, K. (2021). Electrocatalysts for the hydrogen evolution reaction in alkaline and neutral media. A comparative review. J. Power Sources 493, e229708. doi:10.1016/j.jpowsour.2021.229708
Eftekhari, A. J. (2017). Electrocatalysts for hydrogen evolution reaction. Int. J. Hydrogen Energy 42, 11053–11077. doi:10.1016/j.ijhydene.2017.02.125
Eftekhari, A. (2017). Tuning the electrocatalysts for oxygen evolution reaction. Mat. Today Energy 5, 37–57. doi:10.1016/j.mtener.2017.05.002
El Wakkad, S., and Hickling, A. (1950). The anodic behaviour of metals. Part VI.-Cobalt. Trans. Faraday Soc. 46, 820–824. doi:10.1039/tf9504600820
Fabbri, E., Nachtegaal, M., Binninger, T., Cheng, X., Kim, B. J., Durst, J., et al. (2017). Dynamic surface self-reconstruction is the key of highly active perovskite nano-electrocatalysts for water splitting. Nat. Mat. 16, 925–931. doi:10.1038/nmat4938
Fei, H., Dong, J., Feng, Y., Allen, C. S., Wan, C., Volosskiy, B., et al. (2018). General synthesis and definitive structural identification of MN4C4 single-atom catalysts with tunable electrocatalytic activities. Nat. Catal. 1, 63–72. doi:10.1038/s41929-017-0008-y
Gao, J., Tao, H., and Liu, B. J. (2021). Progress of nonprecious-metal_based electrocatalysts for oxygen evolution in acidic media. Adv. Mat. 33, 2003786. doi:10.1002/adma.202003786
Gao, R., Dai, Q., Du, F., Yan, D., and Dai, L. (2019). C60-Adsorbed single-walled carbon nanotubes as metal-free, pH-universal, and multifunctional catalysts for oxygen reduction, oxygen evolution, and hydrogen evolution. J. Am. Chem. Soc. 141, 11658–11666. doi:10.1021/jacs.9b05006
Gardner, G. P., Go, Y. B., Robinson, D. M., Smith, P. F., Hadermann, J., Abakumov, A., et al. (2012). Structural requirements in lithium cobalt oxides for the catalytic oxidation of water. Angew. Chem. Int. Ed. Engl. 51, 1648–1651. doi:10.1002/ange.201107625
Gong, K., Du, F., Xia, Z., Durstock, M., and Dai, L. J. (2009). Nitrogen-doped carbon nanotube arrays with high electrocatalytic activity for oxygen reduction. Science 323, 760–764. doi:10.1126/science.1168049
Gong, L., Chng, X. Y., Du, Y., Xi, S., and Yeo, B. S. (2017). Enhanced catalysis of the electrochemical oxygen evolution reaction by iron(III) ions adsorbed on amorphous cobalt oxide. ACS Catal. 8, 807–814. doi:10.1021/acscatal.7b03509
Grzelczak, M., Zhang, J., Pfrommer, J., Hartmann, J., Driess, M., Antonietti, M., et al. (2013). Electro- and photochemical water oxidation on ligand-free Co3O4 nanoparticles with tunable sizes. ACS Catal. 3, 383–388. doi:10.1021/cs3007523
Han, L., Cheng, H., Liu, W., Li, H., Ou, P., Lin, R., et al. (2022). A single-atom library for guided monometallic and concentration-complex multimetallic designs. Nat. Mat. 21, 681–688. doi:10.1038/s41563-022-01252-y
Hauch, A., Ebbesen, S. D., Jensen, S. H., and MjjoMC, M. (2008). Highly efficient high temperature electrolysis. J. Mat. Chem. 18, 2331–2340. doi:10.1039/b718822f
Hoang, V. C., Dinh, K. N., and Gomes, V. G. (2020). Hybrid Ni/NiO composite with N-doped activated carbon from waste cauliflower leaves: A sustainable bifunctional electrocatalyst for efficient water splitting. Carbon 157, 515–524. doi:10.1016/j.carbon.2019.09.080
Hou, Y., Cui, S., Wen, Z., Guo, X., Feng, X., and Chen, J. J. S. (2015). Strongly coupled 3D hybrids of N-doped porous carbon nanosheet/CoNi alloy-encapsulated carbon nanotubes for enhanced electrocatalysis. Small 11, 5940–5948. doi:10.1002/smll.201502297
Hu, Q., Li, G., Han, Z., Wang, Z., Huang, X., Yang, H., et al. (2019). Recent progress in the hybrids of transition metals/carbon for electrochemical water splitting. J. Mat. Chem. A Mat. 7, 14380–14390. doi:10.1039/c9ta04163j
Huang, J., Li, Y., Zhang, Y., Rao, G., Wu, C., Hu, Y., et al. (2019). Identification of key reversible intermediates in self-reconstructed nickel-based hybrid electrocatalysts for oxygen evolution. Angew. Chem. Int. Ed. Engl. 131, 17619–17625. doi:10.1002/ange.201910716
Hunter, B. M., Gray, H. B., and Muller, A. M. (2016). Earth-abundant heterogeneous water oxidation catalysts. Chem. Rev. 116, 14120–14136. doi:10.1021/acs.chemrev.6b00398
Huynh, M., Shi, C., Billinge, S. J., and Nocera, D. G.(2015). Nature of activated manganese oxide for oxygen evolution. J. Am. Chem. Soc. 137, 14887–14904. doi:10.1021/jacs.5b06382
Jamesh, M-I., and Harb, M. (2021). Tuning the electronic structure of the earth-abundant electrocatalysts for oxygen evolution reaction (OER) to achieve efficient alkaline water splitting – A review. J. Energy Chem. 56, 299–342. doi:10.1016/j.jechem.2020.08.001
Jiang, P., Chen, J., Wang, C., Yang, K., Gong, S., Liu, S., et al. (2018). Tuning the activity of carbon for electrocatalytic hydrogen evolution via an iridium-cobalt alloy core encapsulated in nitrogen-doped carbon cages. Adv. Mat. 30, 1705324. doi:10.1002/adma.201705324
Kauffman, D. R., Deng, X., Sorescu, D. C., Nguyen-Phan, T-D., Wang, C., Marin, C. M., et al. (2019). Edge-enhanced oxygen evolution reactivity at ultrathin, Au-supported Fe2O3 electrocatalysts. ACS Catal. 9, 5375–5382. doi:10.1021/acscatal.9b01093
Kim, D., Oh, L. S., Park, J. H., Kim, H. J., Lee, S., and Lim, E. (2022). Perovskite-based electrocatalysts for oxygen evolution reaction in alkaline media: A mini review. Front. Chem. 10, 1024865. doi:10.3389/fchem.2022.1024865
Li, L., Wang, P., Shao, Q., and Huang, X. (2021). Recent progress in advanced electrocatalyst design for acidic oxygen evolution reaction. Adv. Mat. 33, e2004243. doi:10.1002/adma.202004243
Liang, Q., Brocks, G., and Bieberle-Hütter, A. (2021). Oxygen evolution reaction (OER) mechanism under alkaline and acidic conditions. J. Phys. Energy 3, e026001. doi:10.1088/2515-7655/abdc85
Liu, J., Wang, Z., David, J., Llorca, J., Li, J., Yu, X., et al. (2018). Colloidal Ni2− xCoxP nanocrystals for the hydrogen evolution reaction. J. Mat. Chem. A Mat. 6, 11453–11462. doi:10.1039/c8ta03485k
Liu, M., Lu, X., Guo, C., Wang, Z., Li, Y., Lin, Y., et al. (2017). Architecting a mesoporous N-doped graphitic carbon framework encapsulating CoTe2 as an efficient oxygen evolution electrocatalyst. ACS Appl. Mat. Interfaces 9, 36146–36153. doi:10.1021/acsami.7b09897
Lu, X. F., Chen, Y., Wang, S., Gao, S., and Lou, X. W. (2019). Interfacing manganese oxide and cobalt in porous graphitic carbon polyhedrons boosts oxygen electrocatalysis for Zn–air batteries. Adv. Mat. 31, 1902339. doi:10.1002/adma.201902339
Lu, X. F., Gu, L. F., Wang, J. W., Wu, J. X., Liao, P. Q., and Li, G.(2017). Bimetal-organic framework derived CoFe2O4/C porous hybrid nanorod arrays as high-performance electrocatalysts for oxygen evolution reaction. Adv. Mat. 29, 1604437. doi:10.1002/adma.201604437
Lyons, M. E. G., and Brandon, M. P. (2008). The oxygen evolution reaction on passive oxide covered transition metal electrodes in aqueous alkaline solution. Part 1-Nickel. Int. J. Electrochem Sci. 3, 1386–1424.
Lyu, F., Wang, Q., Choi, S. M., and Yin, Y. (2019). Noble-metal-free electrocatalysts for oxygen evolution. Small 15, e1804201. doi:10.1002/smll.201804201
Marschall, R., and Wang, L. J. (2014). Non-metal doping of transition metal oxides for visible-light photocatalysis. Catal. Today 225, 111–135. doi:10.1016/j.cattod.2013.10.088
Młynarek, G., Paszkiewicz, M., and Radniecka, A. (1984). The effect of ferric ions on the behaviour of a nickelous hydroxide electrode. J. Appl. Electrochem. 14, 145–149. doi:10.1007/bf00618733
Naimi, Y., and Antar, A. (2018). Hydrogen generation by water electrolysis. Adv. Hydrogen Gener. Technol 86, 89–104. doi:10.5772/intechopen.76814
Oliva, P., Leonardi, J., Laurent, J., Delmas, C., Braconnier, J., Figlarz, M., et al. (1982). Review of the structure and the electrochemistry of nickel hydroxides and oxy-hydroxides. J. Power Sources 8, 229–255. doi:10.1016/0378-7753(82)80057-8
Ouyang, Y., Li, Q., Shi, L., Ling, C., and Wang, J. J. (2018). Molybdenum sulfide clusters immobilized on defective graphene: A stable catalyst for the hydrogen evolution reaction. J. Mat. Chem. A Mat. 6, 2289–2294. doi:10.1039/c7ta09828f
Park, J., Lee, S., and Kim, S. (2022). Recent advances in amorphous electrocatalysts for oxygen evolution reaction. Front. Chem. 10, 1030803. doi:10.3389/fchem.2022.1030803
Peng, Z., Yang, S., Jia, D., Da, P., He, P., Al-Enizi, A. M., et al. (2016). Homologous metal-free electrocatalysts grown on three-dimensional carbon networks for overall water splitting in acidic and alkaline media. J. Mat. Chem. A Mat. 4, 12878–12883. doi:10.1039/c6ta04426c
Pletcher, D., and Li, X. (2011). Prospects for alkaline zero gap water electrolysers for hydrogen production,Int. J. Hydrogen Energy 36, 15089–15104. doi:10.1016/j.ijhydene.2011.08.080
Plevová, M., Hnát, J., and Bouzek, K. (2021). Electrocatalysts for the oxygen evolution reaction in alkaline and neutral media. A comparative review. J. Power Sources 507, e230072. doi:10.1016/j.jpowsour.2021.230072
Reier, T., Nong, H. N., Teschner, D., Schlögl, R., and Strasser, P. (2017). Electrocatalytic oxygen evolution reaction in acidic environments - reaction mechanisms and catalysts. Adv. Energy Mat. 7, e1601275. doi:10.1002/aenm.201601275
Silva, V. D., Raimundo, R. A., Simões, T. A., Loureiro, F. J. A., Fagg, D. P., Morales, M. A., et al. (2021). Nonwoven Ni–NiO/carbon fibers for electrochemical water oxidation. Int. J. Hydrogen Energy 46, 3798–3810. doi:10.1016/j.ijhydene.2020.10.156
Stelmachowski, P., Duch, J., Sebastian, D., Lazaro, M. J., and Kotarba, A. (2021). Carbon-based composites as electrocatalysts for oxygen evolution reaction in alkaline media. Mat. (Basel) 14, e4984. doi:10.3390/ma14174984
Sun, Y., Dall’Agnese, C., Zhang, C., Yang, L., Jin, X., Dall’Agnese, Y., et al. (2022). Applications of MXenes and their composites in catalysis and photoelectrocatalysis. Mxenes and their Composites. Amsterdam, Netherlands: Elsevier, 449–498. doi:10.1016/B978-0-12-823361-0.00007-1
Tahir, M., Pan, L., Idrees, F., Zhang, X., Wang, L., Zou, J. J., et al. (2017). Electrocatalytic oxygen evolution reaction for energy conversion and storage: A comprehensive review. Nano Energy 37, 136–157. doi:10.1016/j.nanoen.2017.05.022
Todoroki, N., and Wadayama, T. (2019). Heterolayered Ni-Fe hydroxide/oxide nanostructures generated on a stainless-steel substrate for efficient alkaline water splitting. ACS Appl. Mat. Interfaces 11, 44161–44169. doi:10.1021/acsami.9b14213
Trotochaud, L., Young, S. L., Ranney, J. K., and Boettcher, S. W. J. JotA. C. S. (2014). Nickel–iron oxyhydroxide oxygen-evolution electrocatalysts: The role of intentional and incidental iron incorporation. J. Am. Chem. Soc. 136, 6744–6753. doi:10.1021/ja502379c
Wang, T., Cao, X., and Jiao, L. (2021). Ni2P/NiMoP heterostructure as a bifunctional electrocatalyst for energy-saving hydrogen production. eScience 1, 69–74. doi:10.1016/j.esci.2021.09.002
Wang, W., Wang, Z., Hu, Y., Liu, Y., and Chen, S. (2022). A potential-driven switch of activity promotion mode for the oxygen evolution reaction at Co3O4/NiOxHy interface. eScience 2, 438–444. doi:10.1016/j.esci.2022.04.004
Wang, Y. J., Zhao, N., Fang, B., Li, H., Bi, X. T., and Wang, H. (2015). Carbon-supported Pt-based alloy electrocatalysts for the oxygen reduction reaction in polymer electrolyte membrane fuel cells: Particle size, shape, and composition manipulation and their impact to activity. Chem. Rev. 115, 3433–3467. doi:10.1021/cr500519c
Wei, C., and Xu, Z. J. (2018). The comprehensive understanding of as an evaluation parameter for electrochemical water splitting. New York, NY, USA: Wiley Online Library. doi:10.1002/smtd.201800168
Wu, T., Ren, X., Sun, Y., Sun, S., Xian, G., Scherer, G. G., et al. (2021). Spin pinning effect to reconstructed oxyhydroxide layer on ferromagnetic oxides for enhanced water oxidation. Nat. Commun. 12, 3634. doi:10.1038/s41467-021-23896-1
Wu, Z. P., Lu, X. F., Zang, S. Q., and Lou, X. W. (2020). Non-noble-metal-based electrocatalysts toward the oxygen evolution reaction. Adv. Funct. Mat. 30, e1910274. doi:10.1002/adfm.201910274
Xie, X., Du, L., Yan, L., Park, S., Qiu, Y., Sokolowski, J., et al. (2022). Oxygen evolution reaction in alkaline environment: Material challenges and solutions. Adv. Funct. Mat. 32, e2110036. doi:10.1002/adfm.202110036
Xu, D., Stevens, M. B., Cosby, M. R., Oener, S. Z., Smith, A. M., Enman, L. J., et al. (2018). Earth-abundant oxygen electrocatalysts for alkaline anion-exchange-membrane water electrolysis: Effects of catalyst conductivity and comparison with performance in three-electrode cells. ACS Catal. 9, 7–15. doi:10.1021/acscatal.8b04001
Xu, Y., Tu, W., Zhang, B., Yin, S., Huang, Y., Kraft, M., et al. (2017). Nickel nanoparticles encapsulated in few-layer nitrogen-doped graphene derived from metal–organic frameworks as efficient bifunctional electrocatalysts for overall water splitting. Adv. Mat. 29, 1605957. doi:10.1002/adma.201605957
Yan, J., Kong, L., Ji, Y., White, J., Li, Y., Zhang, J., et al. (2019). Single atom tungsten doped ultrathin alpha-Ni(OH)2 for enhanced electrocatalytic water oxidation. Nat. Commun. 10, 2149. doi:10.1038/s41467-019-09845-z
Yu, M., Budiyanto, E., and Tuysuz, H. (2022). Principles of water electrolysis and recent progress in cobalt-nickel-and iron-based oxides for the oxygen evolution reaction. Angew. Chem. Int. Ed. Engl. 61, e202103824. doi:10.1002/anie.202103824
Zeradjanin, A. R., Masa, J., Spanos, I., and Schlögl, R. (2021). Activity and stability of oxides during oxygen evolution reaction from mechanistic controversies toward relevant electrocatalytic descriptors. Front. Energy Res. 8, 8. doi:10.3389/fenrg.2020.613092
Zhang, J., Zhang, M., Zeng, Y., Chen, J., Qiu, L., Zhou, H., et al. (2019). Single Fe atom on hierarchically porous S, N-codoped nanocarbon derived from porphyra enable boosted oxygen catalysis for rechargeable Zn-air batteries. Small 15, e1900307. doi:10.1002/smll.201900307
Zhang, J., Zhao, Z., Xia, Z., and Dai, L. J. (2015). A metal-free bifunctional electrocatalyst for oxygen reduction and oxygen evolution reactions. Nat. Nanotechnol. 10, 444–452. doi:10.1038/nnano.2015.48
Zhao, M., Zhang, J., Xiao, H., Hu, T., Jia, J., and Wu, H. (2019). Facile in situ synthesis of a carbon quantum dot/graphene heterostructure as an efficient metal-free electrocatalyst for overall water splitting. Chem. Commun. 55, 1635–1638. doi:10.1039/c8cc09368g
Zhao, Y., Yang, N., Yao, H., Liu, D., Song, L., Zhu, J., et al. (2019). Stereodefined codoping of sp-N and S atoms in few-layer graphdiyne for oxygen evolution reaction. J. Am. Chem. Soc. 141, 7240–7244. doi:10.1021/jacs.8b13695
Zhou, S., Liu, N., Wang, Z., and Zhao, J. J., (2017). Nitrogen-doped graphene on transition metal substrates as efficient bifunctional catalysts for oxygen reduction and oxygen evolution reactions. ACS Appl. Mat. Interfaces 9, 22578–22587. doi:10.1021/acsami.7b05755
Zou, S., Burke, M. S., Kast, M. G., Fan, J., Danilovic, N., and Boettcher, S. W. (2015). Fe (Oxy)hydroxide oxygen evolution reaction electrocatalysis: Intrinsic activity and the roles of electrical conductivity, substrate, and dissolution. Chem. Mat. 27, 8011–8020. doi:10.1021/acs.chemmater.5b03404
Keywords: OER electrocatalyst, electrochemical water splitting, noble-metal-free electrocatalyst, water electrolysis, anion exchange membrane electrolysis
Citation: Tan D, Xiong H, Zhang T, Fan X, Wang J and Xu F (2022) Recent progress in noble-metal-free electrocatalysts for alkaline oxygen evolution reaction. Front. Chem. 10:1071274. doi: 10.3389/fchem.2022.1071274
Received: 16 October 2022; Accepted: 17 November 2022;
Published: 08 December 2022.
Edited by:
Xiaopeng Han, Tianjin University, ChinaReviewed by:
Jingqi Chi, Qingdao University of Science and Technology, ChinaZhaohui Xiao, Hainan University, China
Copyright © 2022 Tan, Xiong, Zhang, Fan, Wang and Xu. This is an open-access article distributed under the terms of the Creative Commons Attribution License (CC BY). The use, distribution or reproduction in other forums is permitted, provided the original author(s) and the copyright owner(s) are credited and that the original publication in this journal is cited, in accordance with accepted academic practice. No use, distribution or reproduction is permitted which does not comply with these terms.
*Correspondence: Deming Tan, dGFuZGVtaW5nQGNkdS5lZHUuY24=; Fei Xu, ZmVpeHVAbndwdS5lZHUuY24=