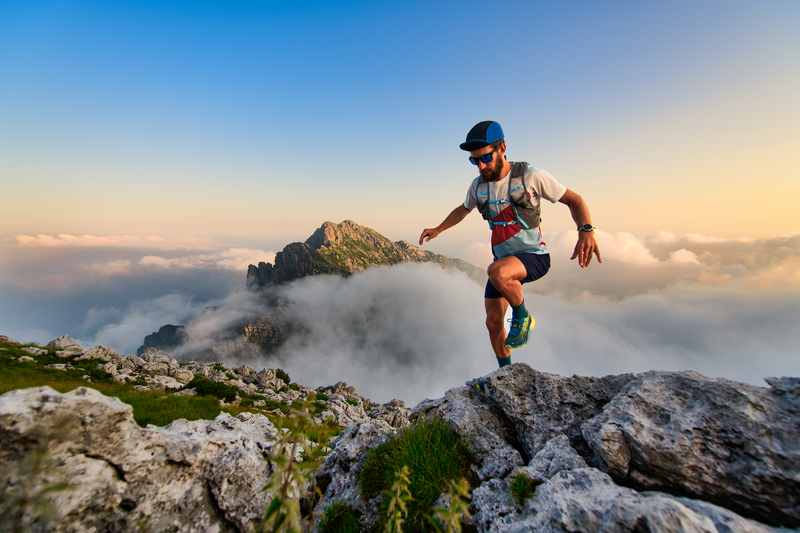
95% of researchers rate our articles as excellent or good
Learn more about the work of our research integrity team to safeguard the quality of each article we publish.
Find out more
ORIGINAL RESEARCH article
Front. Chem. , 30 November 2022
Sec. Electrochemistry
Volume 10 - 2022 | https://doi.org/10.3389/fchem.2022.1069824
This article is part of the Research Topic Photocatalysis and Electrocatalysis for Energy Conversion View all 11 articles
This work prepared an ISAPO-34/SAPO-18 intergrown zeolite using phosphate organoamine as the structure guiding agent. Physical-chemical characterizations by XRD, SEM, TG, and BET showed that the SAPO-34/SAPO-18 presents a cross-stacked cubic block-like microscopic morphology, with characteristic diffusive diffraction peaks at 2θ = 16–18° and 30–33° and a specific surface area of 557 m2 g−1. The series of copper-based catalysts prepared from SAPO-34/SAPO-18 showed a shift of the active temperature window to a lower temperature with increasing copper content. Moreover, the Brønsted acid site decreased significantly due to copper ion exchange and zeolite structure framework damage. Among them, the 1.2 wt% sample showed the widest active temperature window, with a T90 range of 175–435°C. After low-temperature hydrothermal aging treatment, the zeolite structure was eroded and the catalyst activity deteriorated significantly.
Due to the recent increasing seriousness of environmental pollution, theoretical studies have explored methods to relieve this phenomenon based on two-dimensional catalysts (Ren et al., 2022a; Ren et al., 2022b; Ren et al., 2022c; Ren et al., 2022d; Zhang et al., 2022). The gas emissions of internal combustion engines have produced considerable levels of pollution. China steadily ranks first worldwide in the production and sales of internal combustion engines, with domestic sales reaching 46.813 million in 2020. Among these, diesel internal combustion engines comprised 6.341 m units, accounting for 13.5% of the total internal combustion engine sales and showing a steady growth trend. Given this trend, the challenge of exhaust pollutant emission is increasingly prominent and seriously threatens the sustainable development of atmospheric ecological environments. The diesel vehicle emission standards in China mainly follow European emission regulations, for which selective catalytic reduction (SCR) technology provides a necessary way for diesel vehicles to meet VI emission standards (Han et al., 2019). The core catalyst required by ammonia selective catalytic reduction (NH3-SCR) technology, an internationally recognized efficient technology for nitrogen oxides (NOX), is shifted from a vanadium, tungsten, and titanium system (Yang et al., 2014) to a zeolite catalyst system (Chen et al., 2022). The latter is usually composed of zeolite as a carrier of active metal elements (Zheng et al., 2020; Jin et al., 2022; Yuan et al., 2022). The common zeolite skeleton configurations include MFI (Yuan et al., 2016), AEI (Wu et al., 2022), BEA (Lin et al., 2018), LTA (Lin et al., 2021), CHA (Wang et al., 2015; Bergmana et al., 2020; Sun et al., 2020; Zhang et al., 2021a; Bello et al., 2022), AFX (Li et al., 2022a), etc. The zeolite SCR catalyst (Tsukamoto et al., 2019) represented by CHA has advantages including good low-temperature activity, a wide active temperature window, high nitrogen selectivity, green environmental protection due to its unique micropore structure, and suitable surface acidity (Xu et al., 2020; Wang et al., 2021; Zhang et al., 2022a). In recent years, in-depth studies have evaluated the formula, performance, and mechanism of an SCR catalyst with single-structure zeolite. The corresponding intellectual property rights are owned by foreign companies. Polycrystalline/mixed crystal/intergrown zeolite SCR catalysts also show excellent catalytic activity and durability. Zhang et al. (2021b) reported greater catalytic activity, hydrothermal stability, and sulfur aging resistance for a Cu/SAPO-18/34 intergrown zeolite catalyst compared to those for Cu/SAPO-18 and Cu/SAPO-34. As a typical representative, SAPO-18/34 zeolite is a new type of zeolite (Boruntea et al., 2019; Tsuchiya et al., 2020; Li et al., 2022b) composed of AEI and CHA skeleton structure units in stacking faults. It has both the pore canals and acidity of the two crystal phase structures, which usually show better catalytic performance than a single zeolite. Zhao et al. (2016) used triethylamine and N, N-diisopropyl ethylamine as double templates to prepare AEI/CHA intergrown SAPO zeolite and its catalyst, which not only increased the catalytic activity but also significantly reduced the carbon deposition rate. This dual template method is the most common way to prepare symbiotic molecular sieves. However, compared to single templates, it is more difficult to prepare molecular sieves with double templates, and the effects of the proportion, distribution, and chemical state of the templates on the synthesis are more complex. Therefore, the efficient preparation of AEI/CHA symbiotic molecular sieves requires a template.
The present study used phosphate organic amine (Li et al., 2022c) as a template and phosphorus source in a synthesis environment at a pH of 6–7. A SAPO-34/SAPO-18 intergrown zeolite with a hydrogen API-CHA structure was directly prepared and its physicochemical properties and catalytic activity were analyzed by characterization methods including XRD, SEM, and BET. The effects of factors such as active component content and low-temperature hydrothermal inactivation on the performance were studied to provide a reference for the performance research and application of intergrown zeolite SCR catalysts.
The reagents included SAPO-18/SAPO-34 zeolite (self-made, SiO2:P2O5:Al2O3 = 1:3.74:3.81); copper nitrate (analytically pure, Shanghai McLean Biochemical Technology Co., Ltd.); nitric acid (analytically pure, Tianjin Kemio Chemical Reagent Co., Ltd.); deionized water (self-made).
A 0.1N copper nitrate solution was prepared, 200 g of which was weighed and placed in a 500 ml beaker. Next, 10 g of SAPO-18/SAPO-34 zeolite was added to the solution. Nitric acid was then added until pH = 3, stirred, and reacted in a water bath at 80°C. A series of copper-based catalysts were prepared. Those with copper contents of 0.3wt%, 0.8wt%, and 1.2wt% were labeled as 0.3, 0.8, and 1.2, respectively.
XRD characterization was performed using a SmartLab SE X-ray diffractometer (Rigaku Corporation) to analyze the crystal structures of the samples. SEM characterization was performed using an Apero-Lowvac high-resolution field emission scanning electron microscope (Thermo Fisher) to observe the sample microstructure. BET characterization was performed using an ASAP 2460 specific surface area and porosity analyzer (Micrometrics) to analyze the specific surface area, pore volume, and pore size of the test samples. NH3-TPD characterization was performed using an AutoChem II 2920 chemical adsorption instrument (Micrometrics) to analyze the surface acidity characteristics of the samples. TG-DSC characterization was performed using a STA449F5 Jupiter-type synchronous thermal analyzer (NETZSCH) to assess the mass and heat changes of the samples at increasing temperatures.
The catalytic performance was tested in a miniature fixed-bed activity evaluation device, as shown in Figure 1. For these tests, 5 g catalyst powder was fully ground to prepare a 40–60 mesh sample and placed in a quartz reaction tube with a 15 mm inner diameter. Both ends were sealed with quartz cotton to form a catalyst bed. The evaluation device comprised a simulation gas distribution system, a programmed heating device, and a gas analyzer. The simulated tail gas composition was as follows: [NO] = 500 PPM, [NH3] = 500 PPM, [H2O] = 10 vol.%, [O2] = 10 vol.%, with N2 as the equilibrium gas, and an airspeed of 30,000 h−1 (default conditions). The NO conversion rate, NH3 conversion rate, N2O content, and N2 selectivity were calculated according to the following formula:
where NO (out), NO2 (out), N2O (out), and NH3 (out) are the outlet concentrations of NO, NO2, N2O, and NH3 and NO (in) and NH3 (in) are the inlet concentrations of NO and NH3.
FIGURE 1. Equipment diagram for the evaluation of the activity of the NH3-SCR catalyst. 1. Nitric oxide. 2. Ammonia. 3. Nitrogen. 4. Oxygen. 5. Propane. 7. Other Gases. 8. Filter. 9. Globe valve. 10. Mass flowmeter. 11. One-way valve. 12. Mixer. 13. Heating furnace. 14. Reactor. 15. Gas analyzer. 16. Computer.
The formula for the heating program T was as follows:
where the unit of the T is °C; t represents the time with the unit of second.
To investigate the hydrothermal stability of the SAPO-18/SAPO-34 zeolite at low temperature, an SCR catalyst with 1.2% copper content was aged for 100 h at 90°C under 10% water vapor at a space speed of 30,000 h−1. The age and fully dried samples were labeled as 1.2-a. The NH3-SCR catalytic activity of the aged samples was investigated in a micro fixed-bed reactor.
Figure 2A shows the NO conversion curves of the series copper-based SAPO-18/SAPO-34 catalyst in the standard NH3-SCR reaction. With increasing copper content, the ion exchange sites on which copper ions bonded to the molecular sieve as well as the formed active species are generally believed to change, with the temperature window shifting from higher to lower temperatures. The common copper active species in Cu-based zeolite SCR catalysts include Cu2+, [Cu(OH)]+, Cu-O-Cu, CuOx, etc. (Borfecchia et al., 2015; Shan et al., 2019; Liu et al., 2020; Khurana et al., 2022). Figure 2A shows that when the copper content increased from 0.3wt% to 0.8wt%, the range of the active temperature window T90 (the temperature at which the NO conversion rate is 90%) widened from 325–525°C to 170–425°C. Accordingly, the NO ignition temperature T50 (the temperature at which the NO conversion rate is 50%) decreased from 250°C to 160°C. Kwak et al. (2012) reported that at low copper content, the active component copper preferentially occupied the sites in the D6R cage, while some copper ions migrated to the CHA cage when the copper content increased. At a copper content of 1.2wt%, the low-temperature performance of the catalyst decreased slightly and the T50 temperature increased to about 165°C compared to 0.8wt%, and the high-temperature activity increased slightly, with the temperature window widening to 175–435°C. All Cu-based SAPO-18/SAPO-34 catalysts showed excellent nitrogen selectivity of close to 100%, as shown in Figure 2D.
FIGURE 2. NH3-SCR performance of a series of copper-based SCR catalysts. (A) No conversion; (B) NH3 outlet concentration; (C) N2O outlet concentration; (D) N2 selectivity.
Figure 2B shows the outlet ammonia concentrations of the series of copper-based SAPO-18/SAPO-34 catalysts during the activity test. In general, the SAPO-18/SAPO-34 intergrown zeolite showed poor ammonia storage performance, with ammonia escape concentrated at 100°C–300°C. At temperatures above 100°C, the adsorbed NH3 showed excessive desorption. Due to the lower temperature and the poor catalyst activity, NH3 could not fully participate in the selective catalytic reduction reaction, resulting in a sharp increase in its desorption capacity. When the number of catalytic active centers was small, this situation was particularly prominent. The amount of NH3 escape ranked from high to low was as follows: under 0.3wt%> 0.8wt%> 1.2wt%. N2O production showed the opposite trends as those for ammonia escape, as shown in Figure 2C. At lower copper content, less secondary pollutant N2O was generated (Isapour et al., 2022), which may be related to the species of CuOX crystal cluster; however, the overall production was <10 PPM, which met the relevant limit requirements for national emission standards.
Figure 3A shows the XRD pattern of the SAPO-34/SAPO-18 intergrown zeolite. The characteristic diffraction peaks are attributed to 2θ = 9.79°, 13.22°, 16.41°, 18.18°, 19.51°, 21.05°, 23.56°, 24.47°, 25.47°, 26.45°, 31.27°, and 31.61°, respectively. By comparison to the standard spectrum diagram in Figure 3A, the characteristic diffraction peaks of the SAPO-34 zeolite were attributed to 2θ = 9.64°, 13.08°, 14.20°, 16.28°, 18.05°, 19.35°, 20.94°, while the characteristic diffraction peaks of SAPO-18 zeolite were attributed to 2θ = 9.60°, 13.08°, 14.78°, 15.48°, 16.97°, 19.28°, 19.49°, 20.12°, 24.85°, and 26.11°. In contrast, the characteristic diffraction peaks of the SAPO-34/SAPO-18 intergrown zeolite were wide and weak in the range of 2θ = 16–18° and 30–33°, which were not observed in the above two single crystal zeolites. The ellipsoidal CHA structure and the pear-shaped AEI structure have similar skeleton topologies and the hexagonal prism cage (D6R) is key to the connection of the two lattices (Zhao et al., 2017). Where the D6R cage of CHA structural zeolite is straight and parallel, AEI structural zeolite shows a cross-oblique and parallel distribution. Therefore, the SAPO-34/SAPO-18 intergrown zeolite was more inclined to cross-stack on the cubic bulk crystal, with the microstructure shown in Figures 3B,C.
FIGURE 3. (A) XRD patterns; SEM photo of a SAPO-34/SAPO-18 intergrown zeolite: (B) fresh sample (30,000x) and (C) aged sample (20,000x). (D) N2 adsorption-desorption isotherms and porosity distribution curve of a SAPO-34/SAPO-18 intergrown zeolite.
Figure 3B shows the microstructure photos of the SAPO-34/SAPO-18 intergrown zeolite, displaying generally irregular cross-stacked cube blocks. The crystal size is 3–5 μm, and the morphology obviously differs from those of SAPO-34 and SAPO-18 zeolites, which intuitively confirmed the formation of a eutectic structure. The intergrown crystal surface of SAPO-34/SAPO-18 was not smooth, but rather showed defects and damage, possibly because the use of the concentrated sol-gel system to prepare the intergrown zeolite affected the nucleation and growth process. The industrial pure-grade silicon and aluminum sources may have also contributed to the irregular morphology. After low-temperature hydrothermal aging, the defects on the SAPO-34/SAPO-18 crystal surface expanded and showed a tendency for fragmentation, indicating that its structure was damaged, as shown in Figure 3C.
Figure 3D presents the nitrogen physical adsorption test results of the SAPO-34/SAPO-18 intergrown zeolite. The nitrogen isotherm absorption/desorption curve of the intergrown zeolite showed type I isotherm characteristics, as defined by IUPAC, and had a type H4 hysteresis loop. That is, in the interval 0 < P/P0 < 0.01, the adsorption curve rose sharply with increasing relative pressure, indicating that the intergrown zeolite had structural characteristics of microporous materials (Lu et al., 2022). According to the gap width distribution curve, the median pore size was approximately 0.36 nm (Horvath-Kawazoe method); the narrow and sharp distribution reflected the regular lattice and orderly pore distribution of the intergrown zeolite. Moreover, the two-phase symbiosis did not significantly change the skeleton types of the CHA and AEI structural zeolites.
The XRF test results in Table 1 show an elemental composition of SAPO-34/SAPO-18 intergrown zeolite of SiO2:P2O5: Al2O3 = 1:3.74:3.81, which is related to the use of phosphoric acid-organic amine as template agent. The intergrown zeolite had low copper ion exchange efficiency and the copper content was only 0.3wt% after constant exchange at 80°C for 8 h. The copper content increased to 0.8wt% after two more exchanges. After three exchanges, the copper content loading rate was only 1.2 wt%. However, with increasing rounds of ion exchange, the specific surface area of the corresponding zeolite catalyst decreased significantly. The specific surface area of the SAPO-34/SAPO-18 intergrown zeolite was as high as 557 m2 g−1 and decreased to 487 m2 g−1 after three rounds of copper ion exchange. This may occur due to damage to the SAPO-34/SAPO-18 intergrown zeolite structure in the hot water environment used in ion exchange.
Figure 4A shows the test results of the synchronous thermal analysis of the SAPO-34/SAPO-18 intergrown zeolite. The weight loss curve (TG) shows two main weight loss intervals at 40–150°C and 150–300°C and a weight loss rate of about 9 wt%. Below 150°C, the weight loss is obvious (up to 7 wt%), which is related to the rapid evaporation of excessive free water adsorbed by the zeolite. The heat curve (DSC) also reflects the water evaporation and heat absorption at this stage. With increasing temperature, the remaining water bound in the zeolite begins to volatilize and the organic amine template agent undergoes thermal decomposition with heating. The significant slowing of mass and heat changes at 150–300°C were attributed to the new phosphoric acid-organic amine template used in the SAPO-34/SAPO-18 synthesis process effectively avoiding the excessive use of template agent compared to the traditional method. The use of phosphoric acid-organic amine as a structure-diverting agent allowed the accurate and efficient use of organic amine molecules, thus improving environmental protection and economic benefits.
FIGURE 4. (A) TG-DSC curve of a SAPO-34/SAPO-18 intergrown zeolite. (B) NH3-TPD curves of the series of copper-based SCR catalysts.
The surface acidity of zeolite catalysts is generally believed to have an important influence on NH3-SCR performance. The surface acidity and amount of acid in zeolite SCR catalysts are usually characterized by NH3-TPD. Figure 4B shows the temperature-programmed ammonia desorption curves of the series of copper-based SAPO-34/SAPO-18 zeolite catalysts. The three main characteristic peaks were attributed to weak, medium-strong, and strong acid sites, respectively (Pérez-Uriarte et al., 2016; Xu et al., 2017; Shin et al., 2018). The weak acid site at low temperatures corresponded to a weak Lewis acid (T = 220°C), the medium strong acid site at middle temperatures corresponds to strong Lewis acids and some active copper species sites (T = 420°C), and the strong acid adsorption site at high temperatures corresponded to a Brønsted acid (T = 510°C). As shown in Figure 4B, increasing copper content was associated with a decreased acid content of the zeolite catalyst and acid peak intensity to varying degrees. The Brønsted acid position was particularly obvious. Regarding the main factors affecting Brønsted acid sites, 1) during the ion exchange, Cu occupies the hydroxyl site of Si-OH-Al in the six-member ring of zeolite to form Cu2+, with Brønsted acid sites decreasing accordingly (Villamaina et al., 2019). 2) During ion exchange reactions, the high-temperature water environment damages the structure of the SAPO-34/SAPO-18 zeolite, leading to a significant reduction of Brønsted acid sites (Liu et al., 2022). 3) The copper loading amount in the zeolite is exceeded and the active components mainly exist as [Cu(OH)]+ and CuOx clusters (Lee et al., 2021). The generation of CuO may lead to the dealuminization of the zeolite skeleton; that is, the destruction of zeolite Brønsted acid (Si-OH-Al) (Di Iorio et al., 2015; Millan et al., 2021; Negri et al., 2021). Based on the above factors, we further investigated the effect of low-temperature hydrothermal aging treatment on the performance of the NH3-SCR catalyst.
Figure 5A shows the NO conversion curve of a 1.2 wt% sample before and after low-temperature hydrothermal aging. Figure 5A shows significantly decreased catalyst activity with aging. Moreover, the temperature window basically disappears, and the NO ignition temperature exceeds 200°C. The results of crystal phase structure characterization Figure 5B demonstrated that, after aging, the catalyst presents an amorphous state and the characteristic diffraction peak of SAPO-34/SAPO-18 zeolite almost completely disappears. The above results suggest that the crystal phase structure of the copper-based SAPO-34/SAPO-18 zeolite catalyst was destroyed after low-temperature hydrothermal aging treatment, leading to irreversible inactivation (Woo et al., 2018) (Figure 6).
FIGURE 5. Performance changes of the 1.2 wt% copper content catalyst before and after low-temperature hydrothermal aging. (A) NO conversion. (B) XRD patterns.
FIGURE 6 . Schematic diagram of the destruction of zeolite and its catalyst by low-temperature hydrothermal aging treatment.
Ma et al. (2020) reported that the Si-O(H)-Al bond of SAPO-34 zeolite was prone to hydrolysis in low-temperature hydrothermal environments. Woo et al. (2020) believed that the hydrolysis first formed Si (2Al) (2OH) and Si (3Al) (OH), which were finally transformed into silicon clusters. Gao et al. (2013) reported that SAPO-34 zeolite structures with more Si-O(H)-Al bonds showed more serious hydrolysis damage. Wang et al. (2019) studied the effect of SAPO-34 zeolite hydrolysis on active species, in which Cu(OH)+ was transformed into spinel-structured CuAl2O4, with significantly decreased catalytic activity. Leistner et al. (2015) confirmed the loss of the active Cu2+ species lost after the water vapor treatment of Cu-SAPO-34. Zhang et al. (2022b) proposed that the hydrolysis of the Si-O(H)-Al bond and the loss of active copper species jointly induced Cu/SAPO-34 inactivation, with inactivation the easier under a larger proportion of the two.
First, SAPO-18/SAPO-34 intergrown zeolite was prepared using phosphoric acid-organic amine, which has a unique crystal phase structure and microstructure, displaying the typical adsorption characteristics of zeolite microporous materials. Phosphoric acid-organic amines act as both a phosphorus source and a structure-diverting agent. The excessive use of structure-diverting agents can be avoided by use of a synthetic stoichiometric ratio. TG-DSC test results did not show an obvious thermal decomposition phenomenon of organic amines. Secondly, with increasing active component content, the state of copper species changed due to migration, and the active temperature window of the Cu-based SAPO-18/SAPO-34 catalyst shifted toward low temperatures. At copper contents <0.8wt%, the T50 was 160°C, and the T90 range was 170–425°C, showing the optimal performance. Finally, the SAPO-18/SAPO-34 intergrown zeolite showed three main ammonia adsorption sites, and the Brønsted acid sites of the zeolite carrier were lost due to copper occupying the exchange sites during the ion exchange. However, a more important incentive is the structural damage of SAPO zeolite in the low-temperature hydrothermal process. After low-temperature hydrothermal aging treatment, the temperature window of the 1.2wt% Cu content sample almost disappeared, with the crystal phase structure seriously damaged.
The raw data supporting the conclusion of this article will be made available by the authors without undue reservation.
Conceptualization, HY; experimental methods, HY, KR and PW; validation, KR and LW; investigation, HY and PW; writing—original draft preparation, HY, PW and LW; writing—review and editing, KR; funding acquisition, HY. All authors have read and agreed to the published version of the manuscript.
The authors thank the Natural Science Foundation of Jiangsu for support (No. BK20220407).
The authors declare that the research was conducted in the absence of any commercial or financial relationships that could be construed as a potential conflict of interest.
All claims expressed in this article are solely those of the authors and do not necessarily represent those of their affiliated organizations, or those of the publisher, the editors, and the reviewers. Any product that may be evaluated in this article, or claim that may be made by its manufacturer, is not guaranteed or endorsed by the publisher.
Bello, E., Ferri, P., Nero, M., Willhammar, T., Millet, I., Schutze, F. W., et al. (2022). NH3-SCR catalysts for heavy-duty diesel vehicles: Preparation of CHA-type zeolites with low-cost templates. Appl. Catal. B Environ. 303, 120928. doi:10.1016/j.apcatb.2021.120928
Bergmana, S. L., Dahlin, S., Mesilov, V. V., Xiao, Y., Englund, J., Xi, S., et al. (2020). In-situ studies of oxidation/reduction of copper in Cu-CHA SCR catalysts: Comparison of fresh and SO2-poisoned catalysts. Appl. Catal. B Environ. 269, 118722. doi:10.1016/j.apcatb.2020.118722
Borfecchia, E., Lomachenko, K. A., Giordanino, F., Falsig, H., Beato, P., Soldatov, A. V., et al. (2015). Revisiting the nature of Cu sites in the activated Cu-SSZ-13 catalyst for SCR reaction. Chem. Sci. 6, 548–563. doi:10.1039/c4sc02907k
Boruntea, C. R., Lundegaard, L. F., Corma, A., and Vennestrom, P. N. (2019). Crystallization of AEI and AFX zeolites through zeolite-to-zeolite transformations. Microporous Mesoporous Mater. 278, 105–114. doi:10.1016/j.micromeso.2018.11.002
Chen, L., Ren, S., Liu, L., Su, B., Yang, J., Chen, Z., et al. (2022). Catalytic performance over Mn-Ce catalysts for NH3-SCR of NO at low temperature: Different zeolite supports[J]. J. Environ. Chem. Eng. 10, 107167. doi:10.1016/j.jece.2022.107167
Di Iorio, J. R., Bates, S. A., Verma, A. A., Delgass, W. N., Ribeiro, F. H., Miller, J. T., et al. (2015). The dynamic nature of brønsted acid sites in Cu–zeolites during NOx selective catalytic reduction: Quantification by gas-phase Ammonia titration. Top. Catal. 58 (7), 424–434. doi:10.1007/s11244-015-0387-8Topics in Catalysis
Gao, F., Walter, E. D., Washton, N. M., Szanyi, J., and Peden, C. H. F. (2013). Synthesis and evaluation of Cu-SAPO-34 catalysts for ammonia selective catalytic reduction. 1. Aqueous solution ion exchange. ACS Catal. 3, 2083–2093. doi:10.1021/cs4004672
Han, L. P., Cai, S. X., Gao, M., Hasegawa, J. y., Wang, P., Zhang, J., et al. (2019). Selective catalytic reduction of NOx with NH3 by using novel catalysts: State of the art and future prospects. Chem. Rev. 119 (19), 10916–10976. doi:10.1021/acs.chemrev.9b00202
Isapour, G., Wang, A. Y., Han, J., Feng, Y., Gronbeck, H., Creaser, D., et al. (2022). In situ DRIFT studies on N2O formation over Cu-functionalized zeolites during ammonia-SCR. Catal. Sci. Technol. 12, 3921–3936. doi:10.1039/d2cy00247g
Jin, Q. Q., Fang, D., Ye, Y. L., Hou, S., He, F., and Xie, J. (2022). Cu, Co, or Ni species in exchanged Y zeolite catalysts and their denitration performance for selective catalytic reduction by ammonia. Appl. Surf. Sci. 600, 154075. doi:10.1016/j.apsusc.2022.154075
Khurana, I., Albarracin-Caballero, J. D., and Shih, A. J. (2022). Identification and quantification of multinuclear Cu active sites derived from monomeric Cu moieties for dry NO oxidation over Cu-SSZ-13. J. Catal. 413, 1111–1122. doi:10.1016/j.jcat.2022.08.005
Kwak, J. H., Zhu, H. Y., Lee, J. H., Peden, C. H. F., and Szanyi, J. (2012). Two different cationic positions in Cu-SSZ-13. Chem. Commun. 48 (39), 4758–4760. doi:10.1039/c2cc31184d
Lee, H., Song, I., Jeon, S. W., and Kim, D. H. (2021). Mobility of Cu ions in Cu-SSZ-13 determines the reactivity of selective catalytic reduction of NOx with NH3. J. Phys. Chem. Lett. 12 (12), 3210–3216. doi:10.1021/acs.jpclett.1c00181
Leistner, K., and Olsson, L. (2015). Deactivation of Cu/SAPO-34 during low-temperature NH3-SCR[J]. Appl. Catal. B Environ. 165, 192–199. doi:10.1016/j.apcatb.2014.09.067
Li, K. X., Li, Z. G., Ren, X. N., Shao, Y. K., Wang, J. H., Zhang, L., et al. (2022). AEI-CHA symbiotic molecular sieve and catalyst thereof. China. 202210002965.9 [P].
Li, R., Jiang, X. Q., Lin, J. C., Zhang, Z., Huang, Q., Fu, G., et al. (2022). Understanding the influence of hydrothermal treatment on NH3-SCR of NOx activity over Cux-SSZ-16. Chem. Eng. J. 441, 136021. doi:10.1016/j.cej.2022.136021
Li, Z. H., Liu, Y. S., Dou, T., Li, X., Di, C., and Chen, S. L. (2022). Sustainable synthesis of AEI/CHA intergrowth zeolites for methanol-to-olefins conversion. Microporous Mesoporous Mater. 344, 112201. doi:10.1016/j.micromeso.2022.112201
Lin, J. H., Lee, K. H., and Hong, S. B. (2021). Effect of preparation method on NH3-SCR activity of Cu-LTA catalysts. Catal. Today 376, 41–46. doi:10.1016/j.cattod.2020.08.030
Lin, Q. J., Feng, X., Zhang, H. L., Lin, C., Liu, S., Xu, H., et al. (2018). Hydrothermal deactivation over CuFe/BEA for NH3-SCR. J. Industrial Eng. Chem. 65, 40–50. doi:10.1016/j.jiec.2018.04.009
Liu, C., Yasumura, S., Toyao, Ta, Maeno, Z., and Shimizu, K. i. (2022). Mechanism of standard NH3–SCR over Cu-CHA via NO+ and HONO intermediates. J. Phys. Chem. C 126 (28), 11594–11601. doi:10.1021/acs.jpcc.2c03432
Liu, K., Yan, Z. D., Shan, W. P., Shan, Y., Shi, X., and He, H. (2020). Quantitative determination of the Cu species, acid sites and NH3-SCR mechanism on Cu-SSZ-13 and H-SSZ-13 at low temperatures. Catal. Sci. Technol. 10, 1135–1150. doi:10.1039/c9cy02352f
Lu, X. M., Ren, T. S., Cao, P. Z., Wang, Z., Liu, L., He, J., et al. (2022). Construction of high performance binder-free zeolite monolith. Chem. Eng. J. 447, 137558. doi:10.1016/j.cej.2022.137558
Ma, Y., Wu, X. D., Liu, L. P., Cao, L., Ran, R., Si, Z., et al. (2020). Critical roles of Cu(OH)2 in low-temperature moisture-induced degradation of Cu-SAPO-34 SCR catalyst: Correlating reversible and irreversible deactivation. Appl. Catal. B Environ. 278, 119306. doi:10.1016/j.apcatb.2020.119306
Millan, R., Cnudde, P., Speybroeck, V. V., and Boronat, M. (2021). Mobility and reactivity of Cu+ species in Cu-CHA catalysts under NH3-SCR-NOx reaction conditions: Insights from AIMD simulations. JACS Au 1 (10), 1778–1787. doi:10.1021/jacsau.1c00337
Negri, Chiara, Martini, A., Deplano, G., Lomachenko, K. A., Janssens, T. V. W., Borfecchia, E., et al. (2021). Investigating the role of Cu-oxo species in Cu-nitrate formation over Cu-CHA catalysts. Phys. Chem. Chem. Phys. 23, 18322–18337. doi:10.1039/d1cp01754c
Pérez-Uriarte, P., Ateka, A., Aguayo, A. T., and Bilbao, J. (2016). Comparison of HZSM-5 zeolite and SAPO (-18 and -34) based catalysts for the production of light olefins from DME. Catal. Lett. 146, 1892–1902.doi:10.1007/s10562-016-1829-z
Ren, K., Chen, Y., Qin, H., Feng, W., and Zhang, G. (2022). Graphene/biphenylene heterostructure: Interfacial thermal conduction and thermal rectification. Appl. Phys. Lett. 121, 082203. doi:10.1063/5.0100391
Ren, K., Qin, H., Liu, H., Chen, Y., Liu, X., and Zhang, G. Manipulating interfacial thermal conduction of 2D janus heterostructure via a thermo-mechanical coupling[J]. Adv. Funct. Mat., 2022, 32: 2110846, doi:10.1002/adfm.202110846
Ren, K., Wang, K., and Zhang, G. (2022). Atomic adsorption-controlled magnetic properties of a two-dimensional (2D) janus monolayer. ACS Appl. Electron. Mat. 4, 4507–4513. doi:10.1021/acsaelm.2c00740
Ren, K., Yan, Y., Zhang, Z., Sun, M., and Schwingenschlögl, U. (2022). A family of LixBy monolayers with a wide spectrum of potential applications. Appl. Surf. Sci. 604, 154317. doi:10.1016/j.apsusc.2022.154317
Shan, Y. L., Shi, X. Y., Du, J. P., Yu, Y., and He, H. (2019). Cu-Exchanged RTH-type zeolites for NH3-selective catalytic reduction of NOx: Cu distribution and hydrothermal stability. Catal. Sci. Technol. 9, 106–115. doi:10.1039/c8cy01933a
Shin, Y. H., Kweon, S., Park, M. B., and Chae, H. J. (2018). Comparative study of CHA- and AEI-type zeolytic catalysts for the conversion of chloromethane into light olefins. Korean J. Chem. Eng. 35 (7), 1433–1440. doi:10.1007/s11814-018-0050-8
Sun, L. J., Yang, M., Cao, L., Cao, Y., Xu, S., Zhu, D., et al. (2020). Fabrication of Cu-CHA composites with enhanced NH3-SCR catalytic performances and hydrothermal stabilities. Microporous Mesoporous Mater. 309, 110585. doi:10.1016/j.micromeso.2020.110585
Tsuchiya, K., Tsunoji, N., Sasaki, Y., Uemura, M., Onishi, M., Sadakane, M., et al. (2020). Triple-template system for phosphorus-modified AFX/CHA intergrowth zeolite. Microporous Mesoporous Mater. 309, 110540. doi:10.1016/j.micromeso.2020.110540
Tsukamoto, Y., Fukuma, T., and Kusaka, J. (2019). Analysis and modeling of NOx reduction based on the reactivity of Cu active sites and brønsted acid sites in a Cu-chabazite SCR catalyst. SAE Tech. Pap. 24, 0150.
Villamaina, R., Liu, S. J., Nova, I., Tronconi, E., Ruggeri, M. P., Collier, J., et al. (2019). Speciation of Cu cations in Cu-CHA catalysts for NH3-SCR: Effects of SiO2/Al2O3 ratio and Cu-loading investigated by transient response methods. ACS Catal. 9, 8916–8927. doi:10.1021/acscatal.9b02578
Wang, A. Y., Chen, Y., Walter, E. D., Washton, N. M., Mei, D., Varga, T., et al. (2019). Unraveling the mysterious failure of Cu/SAPO-34 selective catalytic reduction catalysts. Nat. Commun. 10, 1137. doi:10.1038/s41467-019-09021-3
Wang, B., Ma, L. J., Han, L. N., Feng, Y., Hu, J., Xie, W., et al. (2021). Assembly-reassembly of coal fly ash into Cu-SSZ-13 zeolite for NH3-SCR of NO via interzeolite transformations. Chem. Eng. Sci. X 10, 100089. doi:10.1016/j.cesx.2021.100089
Wang, D., Jangjou, Y., Liu, Y., Sharma, M. K., Luo, J., Li, J., et al. (2015). A comparison of hydrothermal aging effects on NH3-SCR of NOx over Cu-SSZ-13 and Cu-SAPO-34 catalysts. Appl. Catal. B Environ. 165, 438–445. doi:10.1016/j.apcatb.2014.10.020
Woo, J., Bernin, D., Ahari, H., Shost, M., Zammit, M., and Olsson, L. (2020). Regeneration of Cu/SAPO-34(MO) with H2O only: Too good to be true. Catal. Sci. Technol. 10, 1529–1538. doi:10.1039/c9cy01981b
Woo, J. W., Leistner, K., Bernin, D., Ahari, H., Shost, M., Zammit, M., et al. (2018). Effect of various structure directing agents (SDAs) on low-temperature deactivation of Cu/SAPO-34 during NH3-SCR reaction. Catal. Sci. Technol. 8, 3090–3106. doi:10.1039/c8cy00147b
Wu, Q., Fan, C., Wang, Y., Chen, X., Wang, G., Qin, Z., et al. (2022). Direct incorporating small amount of Ce (III) in Cu-SAPO-18 catalysts for enhanced low-temperature NH3-SCR activity: Influence on Cu distribution and Si coordination. Chem. Eng. J. 435, 134890. doi:10.1016/j.cej.2022.134890
Xu, H. D., Lin, C. L., Lin, Q. J., Feng, X., Zhang, Z., Wang, Y., et al. (2020). Grain size effect on the high-temperature hydrothermal stability of Cu/SAPO-34 catalysts for NH3-SCR. J. Environ. Chem. Eng. 8, 104559. doi:10.1016/j.jece.2020.104559
Xu, Z. Q., Li, J. W., Qian, W. X., Ma, H., Zhang, H., and Ying, W. (2017). Synthesis of core–shell SAPO-34@SAPO-18 composites by the epitaxial growth method and their catalytic properties for the MTO reaction. RSC Adv. 7, 54866–54875. doi:10.1039/c7ra11395a
Yang, J., Lei, S., Yu , , and Xu, G. (2014). Low-cost V−W−Ti SCR catalyst from titanium-bearing blast furnace slag. J. Environ. Chem. Eng. 2, 1007–1010. doi:10.1016/j.jece.2014.03.022
Yuan, E. H., Li, M., Yang, M. H., Huang, X., Zhang, K., Han, W., et al. (2022). Encapsulation of ultra-small Cu–Fe into ZSM-5 zeolites for NH3-SCR with broad reaction-temperature ranges. Microporous Mesoporous Mater. 331, 111675. doi:10.1016/j.micromeso.2021.111675
Yuan, E. H., Zhang, K., Lu, G. X., Mo, Z., and Tang, Z. (2016). Synthesis and application of metal-containing ZSM-5 for the selective catalytic reduction of NOX with NH3. J. Industrial Eng. Chem. 42, 142–148. doi:10.1016/j.jiec.2016.07.030
Zhang, J. Y., Liang, J., Peng, H. G., Mi, Y., Luo, P., Xu, H., et al. (2021). Cost-effective fast-synthesis of chabazite zeolites for the reduction of NOx. Appl. Catal. B Environ. 292, 120163. doi:10.1016/j.apcatb.2021.120163
Zhang, L., Ren, K., Li, J., Cui, Z., and Cheng, H. (2022). The first-principles study of external strain tuning the electronic and optical properties of the 2D MoTe2/PtS2 van der Waals heterostructure. Front. Chem. 10, 934048. doi:10.3389/fchem.2022.934048
Zhang, S. T., Chen, J. W., Meng, Y., Pang, L., Guo, Y., Luo, Z., et al. (2022). Insight into solid-state ion-exchanged Cu-based zeolite (SSZ-13, SAPO-18, and SAPO-34) catalysts for the NH3-SCR reaction: The promoting role of NH4-form zeolite substrates. Appl. Surf. Sci. 571, 151328. doi:10.1016/j.apsusc.2021.151328
Zhang, S. T., Meng, Y., Pang, L., Ding, Q., Chen, Z., Guo, Y., et al. (2022). Understanding the direct relationship between various structure-directing agents and low-temperature hydrothermal durability over CuSAPO-34 during the NH3-SCR reaction. Catal. Sci. Technol. 12, 579–595. doi:10.1039/d1cy02046c
Zhang, S. T., Ming, S. J., Guo, L., Bian, C., Meng, Y., Liu, Q., et al. (2021). Controlled synthesis of Cu-based SAPO-18/34 intergrowth zeolites for selective catalytic reduction of NOx by ammonia. J. Hazard. Mater. 414, 125543. doi:10.1016/j.jhazmat.2021.125543
Zhao, D. P., Zhang, Y., Li, Z., Wang, Y., and Yu, J. (2017). Synthesis of SAPO-18/34 intergrowth zeolites and their enhanced stability for dimethyl ether to olefins. RSC Adv. 7, 939–946. doi:10.1039/c6ra25080g
Zhao, D. P., Zhao, Q. S., Zhang, Y., Shi, T., Yao, H. G., and Yu, J. Q. (2016). Synthesis and characterization of intergrowth structured SAPO-18/SAPO-34. Chem. J. Chin. Univ. 2 (37), 342–348. doi:10.7503/cjcu20150527
Keywords: intergrown structure, copper-based zeolite catalyst, selective catalytic reduction, nitrogen oxides, low-temperature hydrothermal deactivation
Citation: Ye H, Ren K, Wang P and Wang L (2022) The investigation of the NH3-SCR performance of a copper-based AEI-CHA intergrown zeolite catalyst. Front. Chem. 10:1069824. doi: 10.3389/fchem.2022.1069824
Received: 14 October 2022; Accepted: 07 November 2022;
Published: 30 November 2022.
Edited by:
Guangzhao Wang, Yangtze Normal University, ChinaReviewed by:
Weiji Dai, Jiangsu University of Science and Technology, ChinaCopyright © 2022 Ye, Ren, Wang and Wang. This is an open-access article distributed under the terms of the Creative Commons Attribution License (CC BY). The use, distribution or reproduction in other forums is permitted, provided the original author(s) and the copyright owner(s) are credited and that the original publication in this journal is cited, in accordance with accepted academic practice. No use, distribution or reproduction is permitted which does not comply with these terms.
*Correspondence: Hongling Ye, eWhsQGJiYy5lZHUuY24=; Kai Ren, a2FpcmVuQG5qZnUuZWR1LmNu
Disclaimer: All claims expressed in this article are solely those of the authors and do not necessarily represent those of their affiliated organizations, or those of the publisher, the editors and the reviewers. Any product that may be evaluated in this article or claim that may be made by its manufacturer is not guaranteed or endorsed by the publisher.
Research integrity at Frontiers
Learn more about the work of our research integrity team to safeguard the quality of each article we publish.