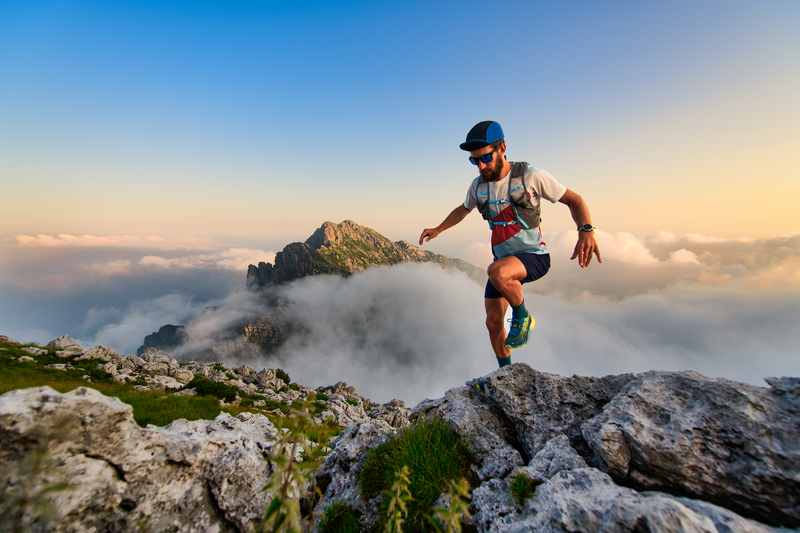
95% of researchers rate our articles as excellent or good
Learn more about the work of our research integrity team to safeguard the quality of each article we publish.
Find out more
MINI REVIEW article
Front. Chem. , 08 November 2022
Sec. Electrochemistry
Volume 10 - 2022 | https://doi.org/10.3389/fchem.2022.1067327
This article is part of the Research Topic Defect Chemistry in Electrocatalysis View all 6 articles
Electrocatalytic CO2 reduction is a promising strategy for converting the greenhouse gas CO2 into high value-added products and achieving carbon neutrality. The rational design of electrocatalysts for CO2 reduction is of great significance. Defect chemistry is an important category for enhancing the intrinsic catalytic performance of electrocatalysts. Defect engineering breaks the catalytic inertia inherent in perfect structures by imparting unique electronic structures and physicochemical properties to electrocatalysts, thereby improving catalytic activity. Recently, various defective nanomaterials have been studied and show great potential in electrocatalytic CO2 reduction. There is an urgent need to gain insight into the effect of defects on catalytic performance. Here, we summarized the recent research advances on the design of various types of defects, including carbon-based materials (intrinsic defects, heteroatom doping and single-metal-atom sites) and metal compounds (vacancies, grain boundaries, and lattice defects). The major challenges and prospects of defect chemistry in electrocatalytic CO2 reduction are also proposed. This review is expected to be instructive in the development of defect engineering for CO2 reduction catalysts.
With the acceleration of industrialization, the emissions of greenhouse gas CO2 have also increased, which has led to serious environmental pollution and energy crisis (Yan et al., 2017). It is desirable to find a mild way to convert CO2 while producing high value-added fuels. Electrocatalytic reduction of CO2 is considered one of the ideal ways to achieve CO2 conversion, which involves a multi-proton coupled electron transfer process (Jin et al., 2021). However, the highly stable structure of CO2 makes it difficult to be activated to participate in the reduction reaction. In addition, the competitive hydrogen evolution reaction (HER) in the CO2 reduction reaction (CO2RR) process also reduces the selectivity of the desired product (Wang et al., 2018b). Therefore, there is an urgent need to find efficient catalysts to reduce the activation energy of CO2 and enhance the selectivity of target products (Yang C. et al., 2021).
It is well known that the performance of electrocatalysts is closely related to the charge transport capacity and intrinsic catalytic activity of the active sites (Zou and Wang, 2021). Tuning the electronic structure and optimizing the reaction interface are effective strategies to improve the catalytic performance (Lu et al., 2022). Among the various regulation strategies, defect engineering is an effective way to break the balance of local electron distribution and regulate the electron structure, which leads to unique physicochemical properties and excellent catalytic performance (Gong et al., 2009; Liu et al., 2019; Wang Q. et al., 2019; Xie et al., 2020). Obviously, the activity of CO2RR greatly depends on the defect chemistry of the employed catalysts (Xue et al., 2020). In general, defect engineering can be widely applied to various types of catalysts, such as intrinsic defects, heteroatom doping and single-metal-atom sites in carbon-based catalysts; and vacancies, grain boundaries and lattice defects in metal compounds (Wang et al., 2021a).
This review aims at providing a detailed discussion on defect chemistry of electrocatalysts that have been discovered and developed to date for CO2RR. We first discuss the effects of defects on carbon-based catalysts including intrinsic defects, heteroatom doping and single-metal-atom sites. Then, we discuss the role of defect engineering in tuning the local atomic and electronic structure of metal compounds, including vacancies, grain boundaries and lattice defects. Finally, the present challenges and future prospects are proposed to give some guidance for the optimization of defect engineering in CO2 reduction electrocatalysts.
The intrinsic defects exist widely in carbon materials, which are formed by lattice distortion and atom deletion. The geometric configuration of intrinsic carbon defects mainly includes edges, vacancies, holes, topological defects, etc. The zigzag and armchair edges make the carbon materials full of unpaired π electrons, which can enhance the local electron density, accelerate electron transfer and lower the formation energy barriers of key intermediates. It was demonstrated that the mechanical ball milling method has the generality of producing more edge defects in the graphitic carbon skeleton (Dong et al., 2019). Meanwhile, chemical etching is an effective strategy to introduce defects on the surface of carbon materials (Li et al., 2019a). Dong et al. used NH3 thermal-treatment to adequately remove pyrrolic-N and pyridinic-N atoms and introduce a high density of defects (Figure 1A) (Dong et al., 2020). As a consequence, the obtained carbon materials possessed a Faradaic efficiency (FE) of 95.2% for CO production (Figure 1B), outperforming that of most metal-free CO2RR electrocatalysts. DFT calculations revealed that the edge pentagonal carbons with the lowest free energy dominated the active sites for CO2RR. This work provided a facile approach for introducing the intrinsic defects to enhance the electrocatalytic activity of carbon materials.
FIGURE 1. (A) Schematic illustration of the synthetic route of 3D topologically defected porous carbon particles. (B) Faradaic efficiencies of CO (gray) and H2 (red) and the partial current of CO on 3D topologically defected porous carbon particles under a range of applied potentials. [Reproduced from (Dong et al., 2020) with permission from the Royal Society of Chemistry]. (C) Defect models used for theoretical calculations: zigzag edge (upper left), armchair edge (upper right), octagonal (lower left), and pentagonal (lower right). (D) DFT calculations for CO2RR activities of different defects. [Reproduced with permission from (Wang et al., 2019)]. (E) FE for CO production at different applied potentials. [Reproduced from (Xue et al., 2019) with permission from the Royal Society of Chemistry]. (F) Schematic of the overall synthesis procedure of Ni-NG-900 catalyst. [Reproduced with permission from (Boppella et al., 2022)]. (G) Free energy diagram of the CO2RR process for CO production on various samples. [Reproduced with permission from (Hao et al., 2022)].
In addition to ammonia treatment and etching strategies, denitrification is also a common strategy for constructing topological defects. For instance, Wang et al. prepared two types of carbon materials by the high temperature removal of heteroatoms (Wang et al., 2019). They revealed that the CO2RR performance of carbon-based catalysts was positively correlated with the content of intrinsic carbon defects. They found that the defective porous carbons without heteroatom dopants also show impressive CO2RR activity. The results of synchrotron and theoretical calculations revealed that the sp2 defects, such as octagonal and pentagonal, are essential for the enhancement of CO2RR performance (Figures 1C,D).
Daiyan et al. (2018) prepared nitrogen-removed mesoporous carbons by carrying out different heat treatments on nitrogen-doped mesoporous carbon. With the removal of the original nitrogen species, the topologically defective structure of the catalyst was formed. When applied to CO2 reduction, the metal-free mesoporous carbon catalyst exhibited a CO FE of 80% and a CO partial current density of −2.9 mA cm−2 at a low overpotential of 0.49 V. The authors demonstrated that the defect sites are the main active sites for CO2RR. Similarly, Chen et al. prepared carbon-based electrocatalysts with topological defects from silk cocoons for CO2RR (Chen et al., 2020b). The resulting electrocatalyst was able to achieve a CO FE of −89% and maintained high selectivity during the 10 days test. Theoretical calculations show that topological defects containing pentagonal carbon rings are the main active sites for accelerated electron transfer in CO2 reduction.
The introduction of topological defects will break the electronic symmetry of the aromatic ring, and the adjacent carbon atoms can be optimized as the active sites for CO2 reduction. Although the construction of intrinsic defects has been well developed, the accurate and quantitative description of certain intrinsic defects is still in its infancy. Some straightforward and simple protocols to precisely quantify the number of defects is highly demanded (Sebastian et al., 2022).
By introducing a small number of other atoms into the original carbon structure, the electronegativity distribution on the surface of the original carbon structure is changed, i.e., the doping of heteroatoms. The introduction of heteroatoms will optimize the intrinsic electronic structure of carbon materials and greatly improve their electrocatalytic activity. The heteroatoms doped into carbon materials are usually nitrogen (N) (Li et al., 2018; Li et al., 2019b; Ning et al., 2022), phosphorus (P) (Chen et al., 2020a), boron (B) (Du et al., 2022), and sulfur (S) atoms (Wang et al., 2020a).
Among them, nitrogen atom doping is the most widely studied. The N doping can be divided into four configurations due to its different locations: graphitic N, pyridinic N, pyrrolic N and N oxide. In the earlier study of N doped carbon materials, Wu et al. (2015) developed N-doped carbon nanotube (NCNT) arrays as efficient electrocatalysts for CO2 reduction. The authors claimed that the structural nature and defect density of N in CNTs determined the catalytic activity. These studies demonstrated the vital role of various configurations of N-doped carbon materials in CO2RR. Wang et al., 2021b fabricated the N-doped porous carbon (NPC) from poly (aniline-co-pyrrole) copolymer with a pyridinic-N content of 2.86 wt% in the presence of salt templets. The optimized catalyst exhibited excellent CO2RR performance with a CO FE of 95.3%, exceeding the performance of most previous N doped carbon electrocatalysts. The experimental and theoretical calculation revealed that the pyridinic-N species contributed significantly to CO2RR.
Besides N, other types of heteroatoms doping/codoping have also been studied. Nakata et al. (2014) used a boron-doped diamond (BDD) electrode to produce formaldehyde under ambient conditions in seawater. This approach broke the traditional limit of the low yield of high-order products, and also suppressed the hydrogen generation. The BDD electrodes with p-type surfaces exhibited a high FE (74%) for formaldehyde, which was helpful for practical applications. The superior performance was attributed to the sp3-bonded carbon of the BDD. These results have great inspiration to achieve high efficiency and low-cost CO2 transformation. Li et al. (2016) reported the S-doped and dual S, N-doped polymer-derived carbons could be used as catalysts for CO2RR. The S, N-doped carbons were obtained by treating the polymer-derived carbon with urea, which showed better catalytic activity towards CO2 reduction than the single S-doped carbons. Xue et al. (2019) synthesized a P and N coordinated fullerene-like carbons (N, P-FC) via a facile soft template pyrolysis method, which showed an impressive activity for CO production in CO2RR (Figure 1E).
Highly dispersed single-metal-atom sites in carbon skeleton can significantly improve the electrocatalytic performance of CO2RR (Wang et al., 2020b; Huang et al., 2022). Single-metal-atom catalysts have received extensive attention due to their high atomic utilization rate and excellent catalytic activity (Zhao et al., 2021). Unlike intrinsically defective or heteroatom-doped active sites, these highly dispersed metal species are generally considered to be directly available as active sites. A large body of research relating to single-metal-atom catalysts has focused on metal-nitrogen-carbon (M-N-C) materials in recent years. MNx constituted the catalytic active sites for the majority of M-N-C materials (Guo et al., 2020), which is similar to the MN4 sites in the macrocylic N-C complexes. For example, metalloporphyrin is a highly effective homogeneous electrocatalyst for CO2RR. Thus, MNx sites existing in solid carbon materials are also considered as active sites for CO2RR.
Research on single-metal-atom catalysts applied to CO2RR can be traced back to 2015. Varela et al. found that the metal-doped nitrogenated carbon, such as Fe-N-C, Mn-N-C and Fe, Mn-N-C showed high CO2RR performance (Varela et al., 2015). Undeniably, for M-N-C catalysts, the category of metal elements and the coordination number of the metal and nitrogen atoms are of key importance for the active sites in CO2RR.
The immobilization of transition metal-nitrogen-carbon complexes has been studied for decades. Various methods and techniques have been generated in this area as well. Meshitsuka et al. are among the earliest researchers who successfully immobilized CoPc and NiPc onto the surface of graphite electrodes (Meshitsuka et al., 1974). In recent years, there has been an increasing number of reports on transition M-N-C complexes as catalysts applied to CO2RR involving various metal centers and supports. However, it is difficult to control the number or thickness of catalyst layers for M-N-C materials prepared by absorption or immobilization methods, which may result in insufficient or too dense active centers and hinder the diffusion of CO2. Therefore, many researchers have been exploring other methods to prepare heterogeneous M-N-C catalysts. A metal-organic framework (MOF) is a polymer consisting of metal-organic ligands on an open framework with a coordinated, porous heterogeneous network structure.
Zhao et al. prepared Ni SAs/N-C catalyst containing Ni-N single-atom sites by ionic exchange of MOFs, showing a superior CO2RR performance with a CO production turnover frequency (TOF) of 5,273 h−1 (Zhao et al., 2017). Ye et al. (2017) synthesized Fe-N-C catalyst by surface functionalization of ZIF-8 with ammonium ferric citrate. The highly exposed Fe-N-C presented an excellent performance for CO2RR, achieving a CO FE of up to 93% at −0.43 V vs. RHE. Gu et al. (2019) prepared a monodisperse Fe3+ single-atom catalyst by controlling the pyrolytic process. The onset potential for CO production was only 80 mV. The partial current density of CO reached 94 mA cm−2 at an ultra-low overpotential of 340 mV. The authors revealed that the active sites were monodisperse Fe3+ particles coordinated with the pyrrolic N on the carbon support using the in-situ synchrotron X-ray absorption spectroscopy. Similarly, Boppella et al. (2022) developed soft-template assisted technology to synthesize pyrrolic N-stabilized single Ni atom electrocatalyst (Figure 1F). The results of the control experiments showed that the synergistic interaction between Ni-Nx and metal free defect sites could effectively promote CO2RR activity.
Furthermore, Lu et al. (2021) developed highly dispersed indium (In) atoms on an N-doped carbon (In-N-C) as a highly efficient catalyst for formic acid production. Hao et al., 2022 synthesized dual-single-atom catalysts consisting of atomically dispersed CuN4 and NiN4 bimetallic sites using electrospun carbon nanofibers. The optimal catalyst exhibited excellent CO FE of 99.6% in a wide potential range (−0.78 to −1.18 V vs. RHE) with a high turnover frequency of 2,870 h−1. The authors revealed that the electronegativity offset of the bimetal atoms induced the strong electron interactions, thereby accelerating the *COOH adsorption and decreasing water dissociation kinetics (Figure 1G). Wang et al. synthesized a Co-N-C catalyst dominated with isolated CoN4 sites (CoN4-CNT) (Wang et al., 2022). When employed in CO2RR, CoN4-CNT exhibited both excellent selectivity (FECO = 99.4%) and activity (jCO = −24.8 mA cm−2) at a low overpotential of 0.49 V in H-type cell, and the FECO remained above 90% with increasing current density from 50 to 600 mA cm−2 in the flow cell.
Single-metal-atom catalysts have become a research hotspot in various catalytic fields, but there are still some doubts about the exact location of their catalytic active center. This is because there are many potential active sites in single-metal-atom catalysts, such as single-metal sites, heteroatoms, intrinsic carbon defects, etc., and it becomes more difficult to quantitatively describe these defects (Qin et al., 2019b). Therefore, more efforts are needed to accurately construct single-metal-atom catalysts with high purity and good homogeneity and to establish a linear relationship between defect density and catalytic activity.
The active centers of metal-based electrocatalysts are often located in defective sites as well. The reasonable introduction of appropriate defects is necessary to enhance the electrocatalytic activity. The charge distribution and intermediate adsorption of the defective active site depend on its microstructure, which is of great significance for the CO2RR process (Gong et al., 2019). Owning to the crucial roles of defects sites in creating new functionalities of metal compounds, atomic-scale identification and quantification is very urgent. In addition to some common evaluation techniques such as Raman spectroscopy, electron-spin resonance spectroscopy, and infrared spectroscopy, etc., the recent rise of deep machine learning may also provide a feasible route to quantify defects (Taherimakhsousi et al., 2020; Yang et al., 2021). According to the different microstructures, the defects of metal compounds can be divided into vacancies, grain boundaries, lattice defects and so on.
Thanks to the lower formation energy, oxygen vacancies are the most common anion defects in metal oxides. The physicochemical properties of metal oxides are changed by the presence of oxygen vacancies, such as the activation of the electron-deficient carbon atoms in CO2, thereby promoting CO2RR (Cao et al., 2022; Wang et al., 2022). For example, Zeng’s group reported a facile H2 plasma treatment to produce oxygen vacancies-riched ZnO nanosheets (Geng et al., 2018). The obtained catalyst exhibited a CO FE of 83% while maintaining a current density of 16.1 mA cm−2 at −1.1 V vs. RHE. DFT calculations revealed that the charge density of ZnO near the valence band maximum increased due to the presence of oxygen vacancies, which promoted the activation of CO2. Li et al. (2020) synthesized oxygen vacancy-rich SnOx nanosheets supported by carbon foam via solvothermal coupled plasma etching strategy (Figure 2A). The optimized electrode exhibited a high FE (86%) and a high partial current density (30 mA cm−2) for formate production (Figure 2B). The oxygen vacancies greatly increased the electrochemical surface area and boosted the adsorption and activation of CO2. Numerous studies have shown that the presence of oxygen vacancies can improve the efficiency of CO2RR by enhancing CO2 adsorption and facilitating its conversion to intermediates (Gao et al., 2017; Wang et al., 2018a; Han et al., 2021; Teh et al., 2021). Consequently, quantitative characterization of oxygen vacancies is thus important to the understanding and application of vacancies-rich catalysts. Lei et al. (2022) found that ZnO nanoparticles with oxygen vacancies can trigger the luminol-H2O2 system to emit strong chemiluminescence (CL), and the CL intensity is strongly dependent on the oxygen vacancies of ZnO nanoparticles. Based on this characteristic, the authors proposed one method for quantifying the oxygen defects in ZnO. In addition to oxygen vacancies, sulfur defects also play a vital role in modifying the electronic structure and reducing the reaction energy barrier of CO2RR (Kang et al., 2019; Cheng et al., 2020). Qin et al. prepared a catalyst of CNTs coated with CdS and found that the S-vacancies generated in the CO2RR process (Qin et al., 2019a). Interestingly, there was a positive correlation between the content of S vacancies and CO formation. DFT calculations indicated that the presence of S-vacancies changed the electron density of the CdS-CNTs catalyst and reduced the energy barrier of CO production.
FIGURE 2. (A) Schematic illustration of the fabrication process of the oxygen vacancy-enriched SnOx nanosheets grown on carbon foam. (B) FE of formate for various electrodes at different electrolytic potentials. (Reproduced from (Li et al., 2020) with permission from the Royal Society of Chemistry). (C) Free energy diagram for the pathways of CO2 conversion into formate on a perfect ZnS and VZn-ZnS surface. [Reproduced with permission from (Pang et al., 2019)]. (D) ECSA normalized CH4 partial current density. [Reproduced with permission from (Choi et al., 2019)]. (E) TEM image of a BiNW showing a twisted structure. (F) HRTEM image of the BiNW showing a grain boundary with crystal lattice dislocation (inset is an FFT image of the square area). (G) Faradaic efficiencies of formate on Cu foam@BiNW, Cu foam@Bi and Bi plate. [Reproduced from (Zhang et al., 2019) with permission from the Royal Society of Chemistry].
In addition to anion vacancies, cation vacancies can also greatly affect the physicochemical properties of metal compounds (Yan et al., 2022). Thanks to their unique electron and orbital distribution, cation vacancies-rich materials also exhibit excellent electrocatalytic performance. For example, Pang et al. (2019) developed a cation vacancy-rich ZnS catalyst, achieving a high selectivity (>85%) of formate in CO2RR. Both experimental and calculation results have demonstrated that the surface Zn vacancies lower the barrier of CO2RR and suppress the HER (Figure 2C). Xia’s group reported the synthesis of ZnIn2S4 nanosheets with abundant Zn vacancies by ultrasonication strategy (Wang et al., 2021d). The obtained catalyst exhibited a partial current density of 245 mA cm−2 and a FE of 94% for formate production in a flow cell. The results revealed that the Zn vacancies with large electrochemically active surface area contributed to the formation of formate.
As mentioned above, both cations and anions vacancies can further enhance the performance of CO2RR. In addition to vacancies, the grain boundaries in polycrystalline materials may also alter the intrinsic physical and chemical properties of the material. For example, Tang et al. (2020) synthesized a series of copper twin boundaries on polished Cu electrodes. The atoms at grain boundaries greatly contribute to methane production. Both the experiment and DFT studies confirmed the twin boundaries facilitate the conversion of absorbed CO* into CH4. Furthermore, Choi et al., 2019 prepared unique star decahedron Cu nanoparticles (SD-Cu NPs) with twin boundaries and multiple stacking faults, exhibiting a low onset potential for methane formation in CO2RR. The DFT calculations revealed that the twin boundaries significantly reduced the formation energy of *CHO, thereby boosting the formation of CH4 at low overpotentials (Figure 2D). Wang et al., 2021c prepared carbon sustained SnO2-Bi2O3 hollow nanofibers as Janus catalyst for formate production. The common boundary of the two components synergistically promotes intrinsic activity. All these suggest that grain boundaries may promote the charge density distribution or change the local electronic environment, thus improving the electrocatalytic performance of metal-based catalysts.
Lattice defects, such as lattice dislocations, expansion and distortion, may significantly alter the physicochemical properties of the metal compounds. The unique electronic structure and enhanced electrical conductivity provided by lattice defects help accelerate charge transport during the CO2RR, thereby increasing catalytic activity. For instance, Zhang’s group synthesized in situ AgCo surface alloy electrocatalysts by cold H2-plasma activation method (Zhang et al., 2020). The as-prepared alloy exhibited high FE of ethanol (72.3%) when used as a CO2RR electrocatalyst. The experimental and DFT results revealed that the distortion of the Ag lattice reduced the energy barrier for the intermediate formation and enhanced the C-C coupling. Zhou et al., 2020 used a supercritical CO2-assisted strategy to fabricate intralayer [Bi2O2]2+ structural distortion in BiOCl. Thanks to the abundant intralayer [Bi2O2]2+ structural distortions in thin nanoplate of BiOCl, the catalyst showed a high selectivity of formate (92%) production in a broad potential range from −0.6 to −0.9 V vs. RHE. This work provides a new route to synthesize two-dimensional materials with crystal distortions for electrocatalytic applications.
Zhang et al. (2019) developed a facile in situ electrochemical transformation strategy to synthesize copper foam supported lattice-dislocated Bi nanowires (Cu foam@BiNW). The obtained Cu foam@BiNW showed an enhanced CO2RR performance with a FE for formate of 95% at −0.69 V vs. RHE (Figures 2E–G). The high CO2RR activity is attributed to the lattice dislocations on the twisted Bi nanowires and the porous structure. They also synthesized a defect-rich Bi catalyst from Bi2S3 through a one-pot hydrothermal reaction (Zhang et al., 2018). When applied to CO2RR, the as-prepared catalyst exhibited the maximum FE of 84% for formate production at an overpotential of 670 mV. The authors revealed that the lattice defects associated with the Bi2S3-derived Bi might have a positive effect on CO2RR.
The application of defective catalysts in electrocatalytic CO2 reduction has become a new research hotspot and has attracted extensive attention in recent years. Defect engineering provides an efficient strategy to tune the surface physicochemical properties of electrocatalysts and leads to a substantial improvement in catalytic performance. In this review, we have briefly summarized recent research progress in defect engineering of electrocatalysts applied to CO2 reduction. Various strategies for adjusting and modifying the surface defects of catalysts are summarized, including intrinsic defects, heteroatom doping, single-metal-atom sites, vacancies, grain boundaries and lattice defects. These defects can adjust the electronic structure of the active center of the electrocatalyst, thus affecting the adsorption, activation and conversion of CO2. Although significant progress has been made in existing studies of catalytic defects, there are still a series of difficulties and challenges remains to be considered.
1 Precise construction of defective sites. It is difficult to precisely introduce a specific defect into the skeleton or lattice of a material. In fact, it is difficult to synthesize catalysts with only one type of defect. For example, there are often multiple types of intrinsic defects in carbon materials, such as edge sites, vacancies, topological defects, and possibly accompanying heteroatoms, etc. Similarly, in metal-based defective materials, lattice dislocations, expansion and distortion are often present simultaneously. In order to determine the specific effects of defect species on electrocatalytic reactions, more controllable and accurate construction methods should be developed. In particular, attempts should be advocated to develop new methods for the large-scale construction of defective materials that can control defect types and density.
2 Advanced characterization techniques to identify defects. Defects often bring unexpected effects. For example, the electronic structure and surface state at the defect site show different properties from the matrix structure. Thus, it becomes more important to characterize them in depth and detail. Although there are many effective techniques and methods for the qualitative characterization of defective sites, the quantitative description of defective sites has always faced some challenges. Therefore, it is highly desirable to accurately characterize defects qualitatively and quantitatively, which will help us in defect site design and predicting the effect of defects on catalytic performance.
3 Stability of defective sites during electrocatalytic reactions. A non-negligible issue during catalytic reactions is the evolution of defective active centers in a complex electrochemical environment. Namely, the stability of the defective sites is an important parameter for practical applications. Changes in defects during the reaction process can significantly increase the complexity of the study, as structural changes often lead to changes in the reaction mechanism. Many unstable defective structures tend to undergo irreversible reconstruction or phase transitions when driven by electric fields, which requires in situ or operando observation of the defect sites during reaction process.
4 Mechanism of defect-sites catalyzed CO2 reduction. Although significant advances have been made in defect engineering for CO2RR, the mechanistic study of defective sites on the CO2 reduction process is still in its initial stage. The adsorption and transformation behavior of CO2 molecules and intermediates at defective sites, and the C-C coupling mechanism of multi-carbon products need to be further clarified. Advanced theoretical simulations and characterizations can help us to understand the active sites and dissect the reaction process, and to explore the structure-activity relationships and reaction mechanisms to a certain extent. Considering the disparity between theoretical calculations and experimental studies, the sophisticated construction of simulation models may be a key step in breaking the gap between theory and reality, and thus deserves more attention.
In summary, we have described the development of defect engineering in the design of CO2RR electrocatalysts. It is obvious that exploring the nature of defects is important for understanding the defective active center and its reaction mechanism in catalyzing CO2 reduction. At this urgent time of increasing carbon emissions, efforts are needed to develop promising defect-rich catalysts that can be prepared on a large scale for high value-added conversion and utilization of CO2. Although there are still many shortcomings in the current CO2 reduction system, we believe that the combination of precisely controlled construction methods, advanced characterization techniques, scientific experimental studies and rigorous model simulations will further accelerate the commercial application of defective electrocatalysts for CO2RR.
HL and XH contributed the conception of the study. HL and RL produced and wrote the article. HL, RL, JN, and KG edited the article. All authors contributed to the article and approved the submitted version.
This work was partly supported by the National Natural Science Foundation of China (Nos. 22108003, 52072002, and 51872005); the Natural Science Foundation of Anhui Provincial Education Department (No. KJ2020A0254); the Research Fund for Young Teachers of Anhui University of Technology (No. QZ202007); and the Wanjiang Scholar Program. The authors would also like to acknowledge the financial support from the Anhui International Research Center of Energy Materials Green Manufacturing and Biotechnology.
The authors declare that the research was conducted in the absence of any commercial or financial relationships that could be construed as a potential conflict of interest.
All claims expressed in this article are solely those of the authors and do not necessarily represent those of their affiliated organizations, or those of the publisher, the editors and the reviewers. Any product that may be evaluated in this article, or claim that may be made by its manufacturer, is not guaranteed or endorsed by the publisher.
Boppella, R., Austeria P, M., Kim, Y., Kim, E., Song, I., Eom, Y., et al. (2022). Pyrrolic N-stabilized monovalent Ni single-atom electrocatalyst for efficient CO2 reduction: Identifying the role of pyrrolic–N and synergistic electrocatalysis. Adv. Funct. Mat. 32, 2202351. doi:10.1002/adfm.202202351
Cao, S., Hu, Y., Yang, C., Li, J., Chen, H., Wei, S., et al. (2022). Constructing surface vacancy to activate the stuck MXenes for high-performance CO2 reduction reaction. J. CO2 Util. 62, 102074. doi:10.1016/j.jcou.2022.102074
Chen, C., Sun, X., Yan, X., Wu, Y., Liu, H., Zhu, Q., et al. (2020a). Boosting CO2 electroreduction on N, P-Co-doped carbon aerogels. Angew. Chem. Int. Ed. 59, 11123–11129. doi:10.1002/anie.202004226
Chen, M., Wang, S., Zhang, H., Zhang, P., Tian, Z., Lu, M., et al. (2020b). Intrinsic defects in biomass-derived carbons facilitate electroreduction of CO2. Nano Res. 13, 729–735. doi:10.1007/s12274-020-2683-2
Cheng, L., Li, Y., Chen, A., Zhu, Y., and Li, C. (2020). Impacts on carbon dioxide electroreduction of cadmium sulfides via continuous surface sulfur vacancy engineering. Chem. Commun. 56, 563–566. doi:10.1039/C9CC08330H
Choi, C., Cheng, T., Flores Espinosa, M., Fei, H., Duan, X., Goddard, W. A., et al. (2019). A highly active star decahedron Cu nanocatalyst for hydrocarbon production at low overpotentials. Adv. Mat. 31, 1805405. doi:10.1002/adma.201805405
Daiyan, R., Tan, X., Chen, R., Saputera, W. H., Tahini, H. A., Lovell, E., et al. (2018). Electroreduction of CO2 to CO on a mesoporous carbon catalyst with progressively removed nitrogen moieties. ACS Energy Lett. 3, 2292–2298. doi:10.1021/acsenergylett.8b01409
Dong, Y., Zhang, Q., Tian, Z., Li, B., Yan, W., Wang, S., et al. (2020). Ammonia thermal treatment toward topological defects in porous carbon for enhanced carbon dioxide electroreduction. Adv. Mat. 32, e2001300. doi:10.1002/adma.202001300
Dong, Y., Zhang, S., Du, X., Hong, S., Zhao, S., Chen, Y., et al. (2019). Boosting the electrical double‐layer capacitance of graphene by self‐doped defects through ball‐milling. Adv. Funct. Mat. 29, 1901127. doi:10.1002/adfm.201901127
Du, J., Fiorani, A., Inagaki, T., Otake, A., Murata, M., Hatanaka, M., et al. (2022). A new pathway for CO2 reduction relying on the self-activation mechanism of boron-doped diamond cathode. JACS Au 2, 1375–1382. doi:10.1021/jacsau.2c00081
Gao, S., Sun, Z., Liu, W., Jiao, X., Zu, X., Hu, Q., et al. (2017). Atomic layer confined vacancies for atomic-level insights into carbon dioxide electroreduction. Nat. Commun. 8, 14503. doi:10.1038/ncomms14503
Geng, Z., Kong, X., Chen, W., Su, H., Liu, Y., Cai, F., et al. (2018). Oxygen vacancies in ZnO nanosheets enhance CO2 electrochemical reduction to CO. Angew. Chem. Int. Ed. 57, 6054–6059. doi:10.1002/anie.201711255
Gong, K., Du, F., Xia, Z., Durstock, M., and Dai, L. (2009). Nitrogen-doped carbon nanotube Arrays with high electrocatalytic activity for oxygen reduction. Science 323, 760–764. doi:10.1126/science.1168049
Gong, Q., Ding, P., Xu, M., Zhu, X., Wang, M., Deng, J., et al. (2019). Structural defects on converted bismuth oxide nanotubes enable highly active electrocatalysis of carbon dioxide reduction. Nat. Commun. 10, 2807. doi:10.1038/s41467-019-10819-4
Gu, J., Hsu, C. S., Bai, L., Chen, H. M., and Hu, X. (2019). Atomically dispersed Fe3+ sites catalyze efficient CO2 electroreduction to CO. Science 364, 1091–1094. doi:10.1126/science.aaw7515
Guo, Z., Xiao, N., Li, H., Wang, Y., Li, C., Liu, C., et al. (2020). Achieving efficient electroreduction CO2 to CO in a wide potential range over pitch-derived ordered mesoporous carbon with engineered Ni-N sites. J. CO2 Util. 38, 212–219. doi:10.1016/j.jcou.2020.01.020
Han, H., Jin, S., Park, S., Kim, Y., Jang, D., Seo, M. H., et al. (2021). Plasma-induced oxygen vacancies in amorphous MnOx boost catalytic performance for electrochemical CO2 reduction. Nano Energy 79, 105492. doi:10.1016/j.nanoen.2020.105492
Hao, J., Zhuang, Z., Hao, J., Wang, C., Lu, S., Duan, F., et al. (2022). Interatomic electronegativity offset dictates selectivity when catalyzing the CO2 reduction reaction. Adv. Energy Mat. 12, 2200579. doi:10.1002/aenm.202200579
Huang, Y., Rehman, F., Tamtaji, M., Li, X., Huang, Y., Zhang, T., et al. (2022). Mechanistic understanding and design of non-noble metal-based single-atom catalysts supported on two-dimensional materials for CO2 electroreduction. J. Mat. Chem. A 10, 5813–5834. doi:10.1039/d1ta08337f
Jin, S., Hao, Z., Zhang, K., Yan, Z., and Chen, J. (2021). Advances and challenges for the electrochemical reduction of CO2 to CO: From fundamentals to industrialization. Angew. Chem. Int. Ed. 60, 20627–20648. doi:10.1002/anie.202101818
Kang, S., Han, S., and Kang, Y. (2019). Unveiling electrochemical reaction pathways of CO2 reduction to CN species at S-vacancies of MoS2. ChemSusChem 12, 2671–2678. doi:10.1002/cssc.201900779
Lei, J., Liu, W., Jin, Y., and Li, B. (2022). Oxygen vacancy-dependent chemiluminescence: A facile approach for quantifying oxygen defects in ZnO. Anal. Chem. 94, 8642–8650. doi:10.1021/acs.analchem.2c00359
Li, C., Wang, Y., Xiao, N., Li, H., Ji, Y., Guo, Z., et al. (2019a). Nitrogen-doped porous carbon from coal for high efficiency CO2 electrocatalytic reduction. Carbon 151, 46–52. doi:10.1016/j.carbon.2019.05.042
Li, H., Xiao, N., Hao, M., Song, X., Wang, Y., Ji, Y., et al. (2018). Efficient CO2 electroreduction over pyridinic-N active sites highly exposed on wrinkled porous carbon nanosheets. Chem. Eng. J. 351, 613–621. doi:10.1016/j.cej.2018.06.077
Li, H., Xiao, N., Wang, Y., Li, C., Ye, X., Guo, Z., et al. (2019b). Nitrogen-doped tubular carbon foam electrodes for efficient electroreduction of CO2 to syngas with potential-independent CO/H2 ratios. J. Mat. Chem. A 7, 18852–18860. doi:10.1039/C9TA05904K
Li, H., Xiao, N., Wang, Y., Liu, C., Zhang, S., Zhang, H., et al. (2020). Promoting the electroreduction of CO2 with oxygen vacancies on a plasma-activated SnOx/carbon foam monolithic electrode. J. Mat. Chem. A 8, 1779–1786. doi:10.1039/C9TA12401B
Li, W., Seredych, M., Rodriguez-Castellon, E., and Bandosz, T. J. (2016). Metal-free nanoporous carbon as a catalyst for electrochemical reduction of CO2 to CO and CH4. ChemSusChem 9, 606–616. doi:10.1002/cssc.201501575
Liu, S., Yang, H. B., Su, X., Ding, J., Mao, Q., Huang, Y. Q., et al. (2019). Rational design of carbon-based metal-free catalysts for electrochemical carbon dioxide reduction: A review. J. Energy Chem. 36, 95–105. doi:10.1016/j.jechem.2019.06.013
Lu, P., Tan, X., Zhao, H., Xiang, Q., Liu, K., Zhao, X., et al. (2021). Atomically dispersed indium sites for selective CO2 electroreduction to formic acid. ACS Nano 15, 5671–5678. doi:10.1021/acsnano.1c00858
Lu, Y., Zhou, L., Wang, S., and Zou, Y. (2022). Defect engineering of electrocatalysts for organic synthesis. Nano Res. doi:10.1007/s12274-022-4858-5
Meshitsuka, S., Ichikawa, M., and Tamaru, K. (1974). Electrocatalysis by metal phthalocyanines in the reduction of carbon dioxide. J. Chem. Soc. Chem. Commun. 158, 158–159. doi:10.1039/C39740000158
Nakata, K., Ozaki, T., Terashima, C., Fujishima, A., and Einaga, Y. (2014). High-yield electrochemical production of formaldehyde from CO2 and seawater. Angew. Chem. Int. Ed. 53, 871–874. doi:10.1002/anie.201308657
Ning, H., Guo, Z., Wang, W., Wang, X., Yang, Z., Ma, Z., et al. (2022). Ammonia etched petroleum pitch-based porous carbon as efficient catalysts for CO2 electroreduction. Carbon Lett. 32, 807–814. doi:10.1007/s42823-021-00316-4
Pang, H., Meng, X., Li, P., Chang, K., Zhou, W., Wang, X., et al. (2019). Cation vacancy-initiated CO2 photoreduction over ZnS for efficient formate production. ACS Energy Lett. 4, 1387–1393. doi:10.1021/acsenergylett.9b00711
Qin, B., Li, Y., Wang, H., Yang, G., Cao, Y., Yu, H., et al. (2019a). Efficient electrochemical reduction of CO2 into CO promoted by sulfur vacancies. Nano Energy 60, 43–51. doi:10.1016/j.nanoen.2019.03.024
Qin, X., Zhu, S., Xiao, F., Zhang, L., and Shao, M. (2019b). Active sites on heterogeneous single-iron-atom electrocatalysts in CO2 reduction reaction. ACS Energy Lett. 4, 1778–1783. doi:10.1021/acsenergylett.9b01015
Sebastian, F. L., Zorn, N. F., Settele, S., Lindenthal, S., Berger, F. J., Bendel, C., et al. (2022). Absolute quantification of sp3 defects in semiconducting single-wall carbon nanotubes by Raman spectroscopy. J. Phys. Chem. Lett. 13, 3542–3548. doi:10.1021/acs.jpclett.2c00758
Taherimakhsousi, N., Macleod, B. P., Parlane, F. G. L., Morrissey, T. D., Booker, E. P., Dettelbach, K. E., et al. (2020). Quantifying defects in thin films using machine vision. npj Comput. Mat. 6, 111. doi:10.1038/s41524-020-00380-w
Tang, C., Shi, J., Bai, X., Hu, A., Xuan, N., Yue, Y., et al. (2020). CO2 reduction on copper’s twin boundary. ACS Catal. 10, 2026–2032. doi:10.1021/acscatal.9b03814
Teh, W. J., Piqué, O., Low, Q. H., Zhu, W., Calle-Vallejo, F., and Yeo, B. S. (2021). Toward efficient tandem electroreduction of CO2 to methanol using anodized titanium. ACS Catal. 11, 8467–8475. doi:10.1021/acscatal.1c01725
Varela, A. S., Ranjbar Sahraie, N., Steinberg, J., Ju, W., Oh, H. S., and Strasser, P. (2015). Metal-doped nitrogenated carbon as an efficient catalyst for direct CO2 electroreduction to CO and hydrocarbons. Angew. Chem. Int. Ed. 54, 10758–10762. doi:10.1002/anie.201502099
Wang, C., Liu, Y., Ren, H., Guan, Q., Chou, S., and Li, W. (2022). Diminishing the uncoordinated N species in Co-N-C catalysts toward highly efficient electrochemical CO2 reduction. ACS Catal. 12, 2513–2521. doi:10.1021/acscatal.1c05029
Wang, G., Liu, M., Jia, J., Xu, H., Zhao, B., Lai, K., et al. (2020a). Nitrogen and sulfur Co-doped carbon nanosheets for electrochemical reduction of CO2. ChemCatChem 12, 2203–2208. doi:10.1002/cctc.201902326
Wang, J., Tan, H. Y., Zhu, Y., Chu, H., and Chen, H. M. (2021a). Linking the dynamic chemical state of catalysts with the product profile of electrocatalytic CO2 reduction. Angew. Chem. Int. Ed. 60, 17254–17267. doi:10.1002/anie.202017181
Wang, Q., Lei, Y., Wang, D., and Li, Y. (2019). Defect engineering in earth-abundant electrocatalysts for CO2 and N2 reduction. Energy Environ. Sci. 12, 1730–1750. doi:10.1039/c8ee03781g
Wang, W., Ma, Z., Fei, X., Wang, X., Yang, Z., Wang, Y., et al. (2022). Joint tuning the morphology and oxygen vacancy of Cu2O by ionic liquid enables high-efficient CO2 reduction to C2 products. Chem. Eng. J. 436, 135029. doi:10.1016/j.cej.2022.135029
Wang, W., Shang, L., Chang, G., Yan, C., Shi, R., Zhao, Y., et al. (2019). Intrinsic carbon-defect-driven electrocatalytic reduction of carbon dioxide. Adv. Mat. 31, 1808276. doi:10.1002/adma.201808276
Wang, X., Li, X., Ding, S., Chen, Y., Liu, Y., Fang, M., et al. (2021b). Constructing ample active sites in nitrogen-doped carbon materials for efficient electrocatalytic carbon dioxide reduction. Nano Energy 90, 106541. doi:10.1016/j.nanoen.2021.106541
Wang, X., Pan, Y., Ning, H., Wang, H., Guo, D., Wang, W., et al. (2020b). Hierarchically micro- and meso-porous Fe-N4O-doped carbon as robust electrocatalyst for CO2 reduction. Appl. Catal. B Environ. 266, 118630. doi:10.1016/j.apcatb.2020.118630
Wang, X., Wang, W., Zhang, J., Wang, H., Yang, Z., Ning, H., et al. (2021c). Carbon sustained SnO2-Bi2O3 hollow nanofibers as Janus catalyst for high-efficiency CO2 electroreduction. Chem. Eng. J. 426, 131867. doi:10.1016/j.cej.2021.131867
Wang, Y., Chen, Z., Han, P., Du, Y., Gu, Z., Xu, X., et al. (2018a). Single-atomic Cu with multiple oxygen vacancies on ceria for electrocatalytic CO2 reduction to CH4. ACS Catal. 8, 7113–7119. doi:10.1021/acscatal.8b01014
Wang, Y., Han, P., Lv, X., Zhang, L., and Zheng, G. (2018b). Defect and interface engineering for aqueous electrocatalytic CO2 reduction. Joule 2, 2551–2582. doi:10.1016/j.joule.2018.09.021
Wang, Z., Qi, R., Liu, D., Zhao, X., Huang, L., Chen, S., et al. (2021d). Exfoliated ultrathin ZnIn2S4 nanosheets with abundant zinc vacancies for enhanced CO2 electroreduction to formate. ChemSusChem 14, 852–859. doi:10.1002/cssc.202002785
Wu, J., Yadav, R. M., Liu, M., Sharma, P. P., Tiwary, C. S., Ma, L., et al. (2015). Achieving highly efficient, selective, and stable CO2 reduction on nitrogen-doped carbon nanotubes. ACS Nano 9, 5364–5371. doi:10.1021/acsnano.5b01079
Xie, C., Yan, D., Li, H., Du, S., Chen, W., Wang, Y., et al. (2020). Defect chemistry in heterogeneous catalysis: Recognition, understanding, and utilization. ACS Catal. 10, 11082–11098. doi:10.1021/acscatal.0c03034
Xue, D., Xia, H., Yan, W., Zhang, J., and Mu, S. (2020). Defect engineering on carbon-based catalysts for electrocatalytic CO2 reduction. Nano-Micro Lett. 13, 5. doi:10.1007/s40820-020-00538-7
Xue, X., Yang, H., Yang, T., Yuan, P., Li, Q., Mu, S., et al. (2019). N, P-coordinated fullerene-like carbon nanostructures with dual active centers toward highly-efficient multi-functional electrocatalysis for CO2RR, ORR and Zn-air battery. J. Mat. Chem. A 7, 15271–15277. doi:10.1039/C9TA03828K
Yan, D., Li, Y., Huo, J., Chen, R., Dai, L., and Wang, S. (2017). Defect chemistry of nonprecious-metal electrocatalysts for oxygen reactions. Adv. Mat. 29, 1606459. doi:10.1002/adma.201606459
Yan, D., Xia, C., Zhang, W., Hu, Q., He, C., Xia, B. Y., et al. (2022). Cation defect engineering of transition metal electrocatalysts for oxygen evolution reaction. Adv. Energy Mat., 2202317. doi:10.1002/aenm.202202317
Yang, C., Lu, Y., Zhang, L., Kong, Z., Yang, T., Tao, L., et al. (2021). Defect engineering on CeO2‐based catalysts for heterogeneous catalytic applications. Small Struct. 2, 2100058. doi:10.1002/sstr.202100058
Yang, S.-H., Choi, W., Cho, B. W., Agyapong-Fordjour, F. O.-T., Park, S., Yun, S. J., et al. (2021). Deep learning-assisted quantification of atomic dopants and defects in 2D materials. Adv. Sci. 8, 2101099. doi:10.1002/advs.202101099
Ye, Y., Cai, F., Li, H., Wu, H., Wang, G., Li, Y., et al. (2017). Surface functionalization of ZIF-8 with ammonium ferric citrate toward high exposure of Fe-N active sites for efficient oxygen and carbon dioxide electroreduction. Nano Energy 38, 281–289. doi:10.1016/j.nanoen.2017.05.042
Zhang, Q., Tao, S., Du, J., He, A., Yang, Y., and Tao, C. (2020). A cold plasma-activated in situ AgCo surface alloy for enhancing the electroreduction of CO2 to ethanol. J. Mat. Chem. A 8, 8410–8420. doi:10.1039/d0ta01262a
Zhang, X., Sun, X., Guo, S.-X., Bond, A. M., and Zhang, J. (2019). Formation of lattice-dislocated bismuth nanowires on copper foam for enhanced electrocatalytic CO2 reduction at low overpotential. Energy Environ. Sci. 12, 1334–1340. doi:10.1039/C9EE00018F
Zhang, Y., Li, F., Zhang, X., Williams, T., Easton, C. D., Bond, A. M., et al. (2018). Electrochemical reduction of CO2 on defect-rich Bi derived from Bi2S3 with enhanced formate selectivity. J. Mat. Chem. A 6, 4714–4720. doi:10.1039/C8TA00023A
Zhao, C., Dai, X., Yao, T., Chen, W., Wang, X., Wang, J., et al. (2017). Ionic exchange of metal–organic frameworks to access single nickel sites for efficient electroreduction of CO2. J. Am. Chem. Soc. 139, 8078–8081. doi:10.1021/jacs.7b02736
Zhao, S., Xiao, N., Li, H., Guo, Z., Bai, J., Xiao, J., et al. (2021). A nickel-nitrogen-doped carbon foam as monolithic electrode for highly efficient CO2 electroreduction. J. CO2 Util. 49, 101549. doi:10.1016/j.jcou.2021.101549
Zhou, Y., Yan, P., Jia, J., Zhang, S., Zheng, X., Zhang, L., et al. (2020). Supercritical CO2-constructed intralayer [Bi2O2]2+ structural distortion for enhanced CO2 electroreduction. J. Mat. Chem. A 8, 13320–13327. doi:10.1039/d0ta04163g
Keywords: defects chemistry, defects engineering, CO2 electroreduction, carbon-based materials, metal compounds
Citation: Li H, Li R, Niu J, Gan K and He X (2022) Defect chemistry of electrocatalysts for CO2 reduction. Front. Chem. 10:1067327. doi: 10.3389/fchem.2022.1067327
Received: 11 October 2022; Accepted: 25 October 2022;
Published: 08 November 2022.
Edited by:
Dafeng Yan, Huazhong University of Science and Technology, ChinaReviewed by:
Dunfeng Gao, Dalian Institute of Chemical Physics (CAS), ChinaCopyright © 2022 Li, Li, Niu, Gan and He. This is an open-access article distributed under the terms of the Creative Commons Attribution License (CC BY). The use, distribution or reproduction in other forums is permitted, provided the original author(s) and the copyright owner(s) are credited and that the original publication in this journal is cited, in accordance with accepted academic practice. No use, distribution or reproduction is permitted which does not comply with these terms.
*Correspondence: Hongqiang Li, aHFsaTIwMjBAYWh1dC5lZHUuY24=; Xiaojun He, eGpoZUBhaHV0LmVkdS5jbg==
Disclaimer: All claims expressed in this article are solely those of the authors and do not necessarily represent those of their affiliated organizations, or those of the publisher, the editors and the reviewers. Any product that may be evaluated in this article or claim that may be made by its manufacturer is not guaranteed or endorsed by the publisher.
Research integrity at Frontiers
Learn more about the work of our research integrity team to safeguard the quality of each article we publish.