- 1Country Enterprise Technology Center of Guizhou Province, Guizhou University, Guiyang, China
- 2International Innovation Center for Forest Chemicals and Materials, Co-Innovation Center of Efficient Processing and Utilization of Forest Resources, College of Materials Science and Engineering, Nanjing Forestry University, Nanjing, China
- 3National Synchrotron Radiation Laboratory, University of Science and Technology of China, Hefei, China
- 4Advanced Light Source, Lawrence Berkeley National Laboratory, Berkeley, CA, United States
5-Hydroxymethylfurfural (HMF) has aroused considerable interest over the past years as an important biomass-derived platform molecule, yielding various value-added products. The conventional HMF conversion requires noble metal catalysts and harsh operating conditions. On the other hand, the electrocatalytic conversion of HMF has been considered as an environmentally benign alternative. However, its practical application is limited by low overall energy efficiency and incomplete conversion. Paired electrolysis and highly efficient electrocatalysts are two viable strategies to address these limitations. Herein, an overview of coupled electrocatalytic HMF hydrogenation or hydrogen evolution reaction (HER) with HMF oxidation as well as the associated electrocatalysts are reviewed and discussed. In this mini-review, a brief introduction of electrocatalytic HMF upgrading is given, followed by the recent advances and challenges of paired electrolysis with an emphasis on the integration HMF electrohydrogenation with HMF electrooxidation. Finally, a perspective for a future sustainable biomass upgrading community based on electrocatalysis is proposed.
Introduction
The rapid development of human civilization and growth of world population result in fiercely global energy demands (Corma et al., 2007; Mika et al., 2018). Due to the declining fossil fuel reserves and the increasing concerns about environmental impacts resulting from fossil fuel combustion, more efforts have been devoted to exploring sustainable energy sources and renewable carbons for organic chemical production (Bozell, 2010; Shi and Zhang, 2016). As the most abundant natural carbon, biomass possesses a great promise in developing carbon-neutral economy (Demirbas, 2001; Hu et al., 2012; Morais et al., 2015). Recently, a biomass-derived chemical, 5-hydroxymethylfurfural (HMF), which is among the Department of Energy’s “Top 10 + 4” list, has been considered as a versatile platform molecule (Bozell and Petersen, 2010; Adeogun et al., 2019; Baga, 2020). Owing to the hydroxymethyl and formyl functional groups attached to the furan ring, further upgrading HMF can generate various high-valued chemicals via oxidation, reduction, hydrogenation, esterification, hydrolysis, and cleavage (Binder and Raines, 2009; Bozell and Petersen, 2010; Du et al., 2011; Rosatella et al., 2011; Alamillo et al., 2012; Balakrishnan et al., 2012; Gallo et al., 2013).
The oxidation of HMF yields valuable chemicals, such as 2,5-diformylfuran (DFF), 5-formyl-furan carboxylic acid (FFCA), 5-hydroxymethyl-2-furan carboxylic acid (HMFCA), and 2,5-furan dicarboxylic acid (FDCA), as shown in Figure 1A (Xiang et al., 2011; Vuyyuru and Strasser, 2012a; Antonyraj et al., 2013). Due to the symmetric structure of functional groups, both DFF and FDCA as monomers have tremendous potentials for synthesizing biomass-derived drugs and antifungal agents, furan-urea resins, and other important polymer materials (Liu and Zhang, 2016; Lei et al., 2020). FDCA can serve as a replacement for the petroleum-derived terephthalic acid, producing polyethylene terephthalate and poly (ethylene 2,5-furandicarbocylate) (Wang J. et al., 2017a). In addition, FDCA has a large potential to take a place of terephthalate and butyleneterephthalate, which are used widely in producing various polyesters (Gandini et al., 2009; Kong et al., 2018). Meanwhile, the reduction products of HMF include 2,5-dimethylfuran (DMF), 2,5-dihydroxymethylfuran (BHMF), 2,5-bishydroxymethyl-tetrahydrofuran (DHMTHF), and 2,5-hexanedione (HD) (Connolly et al., 2010; Kong et al., 2014; Scholz et al., 2014; Roylance and Choi, 2016b; Elangovan et al., 2016; Guo et al., 2016; Perret et al., 2016; Luo et al., 2017). Among these reductive products, BHMF, which has two hydroxymethyl groups fused with the furan ring, can also act as a precursor to form polyesters and polyurethane foams (Lecomte et al., 1998; Gandini, 2011; Delidovich et al., 2016; Hu et al., 2018). Moreover, DMF and DHMTHF have been regarded as promising next-generation biofuels (Goyal et al., 2016; Xia et al., 2018). Specifically, DMF has higher energy density and better miscibility than fuel ethanol, which can be used as a potential high-quality liquid biofuel to replace gasoline derived from fossil fuels (Goyal et al., 2016; Kwon et al., 2016). Furthermore, HD is able to serve as the raw material for the production of paraxylene, which is an important precursor to produce polyethylene terephthalate (PET) as well (Roylance and Choi, 2016b). Apart from the above, HMF itself can also undergo hydroxyl-aldehyde condensation with acetone to produce liquid fuel intermediates (Su et al., 2020).
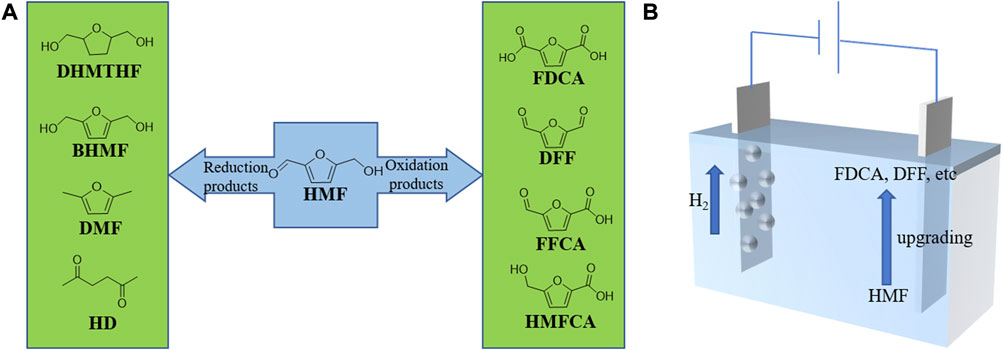
FIGURE 1. (A) Reductive and oxidative products of HMF upgrading. (B) Paired electrolysis of HMF oxidation with HER.
The conventional thermocatalytic valorization of HMF always requires noble metal catalysts (Au, Pd, Pt, and Ru) and harsh operational conditions, such as high temperatures and pressures (Taarning et al., 2008; Casanova et al., 2009; Gorbanev et al., 2009; Davis et al., 2011; Villa et al., 2013; Zhang and Deng, 2015). Additionally, HMF oxidation via conventional approaches relies on using toxic oxidants or organic solvents (CH2Cl2, C6H5CH3, CH3CN, etc.) (Amarasekara et al., 2008; Cao et al., 2017). For instance, Gao et al. utilized toxic organic reagents such as methylene chloride and acetonitrile in the process of oxidizing HMF into FDCA (Gao et al., 2015). On the other hand, the conventional methods for the reduction of HMF usually perform at high H2 pressure and requires catalysts containing precious metals as well (Alamillo et al., 2012; Wang T. et al., 2017b). There are certain safety hazards when using H2 as the proton source for HMF reduction (Bloor et al., 2016). Plus, it is not cost-efficient because H2 is a valuable energy carrier. Therefore, it is necessary to explore economical and environmentally friendly strategies to upgrade HMF (Ohyama et al., 2012; Ohyama et al., 2013; Kong et al., 2014; Yang et al., 2017; Sajid et al., 2018). To date, the electrocatalytic HMF upgrading has attracted a great deal of attention (You et al., 2016b; Zhang L. et al., 2019b; Zhang Y.-R. et al., 2019e; Taitt et al., 2019; Kisszekelyi et al., 2020; Zhou et al., 2021). Electrocatalysis is driven by electricity and can be conducted under aqueous and ambient conditions, which is more environmentally benign. Notably, H2O can be used as oxidant or proton source instead of costly oxidizing agents and H2 gas. More to the point, the reaction rate and product selectivity can be easily controlled by the applied voltage and current (Nilges and Schroeder, 2013; Cha and Choi, 2015; Roylance et al., 2016). In order to achieve higher Faradic efficiency (FE) and overall conversion efficiency, the valorization of HMF can also be paired with other half reactions. From the last 5 years, the research on the paired electrolysis of HMF is mainly focused on coupling the oxidation of HMF with hydrogen evolution reaction (HER), while the combination of HMF reduction with other reactions has received only a few studies. In this mini-review, we summarized the recent advances of paired electrolysis in HMF valorization, which is different from previous reviews. Firstly, we introduce the electrocatalytic oxidation of HMF integrated with HER. Then, the electrochemical reduction strategy of HMF is briefly introduced, followed by emphasizing the upgrading of HMF on both cathode and anode simultaneously. Finally, we discuss the challenges and future directions of paired electrolysis in HMF valorization.
Paired HMF oxidation with HER
H2 is a pollution-free fuel with high energy density, which has been considered as an alternative to fossil fuels (El-Emam and Ozcan, 2019; Xia et al., 2020). Electrocatalytic water splitting to produce clean H2 has gained increasing attention (Yu et al., 2018). However, the anodic reaction, oxygen evolution reaction (OER), is the bottleneck of water splitting, which results in the low energy conversion efficiency because of its sluggish kinetics (Zhang J.-Y. et al., 2019a; You et al., 2019). Besides, the product of OER, O2, does not have a significantly commercial value. Therefore, replacing OER with a thermodynamically more favorable HMF oxidation reaction can not only solve the safety hazard caused by hydrogen and oxygen mixing, but also improve the overall energy conversion efficiency (Chen et al., 2014; Jiao et al., 2015; Yang and Mu, 2021). As an innovative strategy (Figure 1B), the electrocatalytic oxidation of HMF coupled with HER can produce highly valuable products on both anode and cathode simultaneously (You and Sun, 2018; Dubale et al., 2020). Consequently, it is highly attractive to develop efficient electrocatalysts, especially bifunctional catalysts, to integrate HMF oxidation with HER in a single electrolyzer.
Nobel metal catalysts and their alloys have been extensively studied for electrooxidation of HMF due to their high activity in many chemical processes (Kokoh and Belgsir, 2002; Vuyyuru and Strasser, 2012a; Xu et al., 2019; Du et al., 2020; Park et al., 2020). In addition, noble metals such as Pt and Pd have excellent HER performance. Therefore, noble metal catalysts have been applied as bifunctional catalysts in paired electrocatalysis of HMF oxidation and HER. Kim’s group found that the (AuPd)7 alloy had remarkable catalytic performance for electrocatalytic oxidation of HMF and HER in a coupled cell (Park et al., 2020). Although it is hard to realize industrial applications with noble metal catalysts because of their high price, they have contributed a lot to the study of the mechanism of HMF oxidation (Cha and Choi, 2015; Latsuzbaia et al., 2018; Heidary and Kornienko, 2019).
Although noble metals have exhibited excellent catalytic activity for HMF oxidation, they still suffer from high-cost due to their scarcity. Thus, the development of earth-abundant electrocatalysts with high efficiency has become a focus for large scale HMF oxidation integrated with HER. To date, various transition metals ranging from Ni, Co, Cu, Fe to Mn are used to design bifunctional catalysts for HMF oxidation and H2 production (Jiang et al., 2016; You et al., 2017; Liu et al., 2018; Nam et al., 2018; Li S. et al., 2019b). Ni (You et al., 2017) and its nitrides (Zhang N. et al., 2019c), borides (Barwe et al., 2018; Zhang P. et al., 2019d), phosphides (You et al., 2016a; Li M. et al., 2019a), oxides (Choi et al., 2020; Gao et al., 2020; Lu et al., 2020), and hydroxides (Latsuzbaia et al., 2018; Chen et al., 2019) have been reported for the electrochemical oxidation of HMF. Among these catalysts containing nickel, NiN3@C, Ni2P, hp-Ni (3D hierarchically porous nickel-based catalyst), and NiSe@NiOx core-shell nanowires had been used as bifunctional catalysts for both HMF oxidation and HER with high FE (>95%) for FDCA and H2, respectively. Moreover, all of these bifunctional catalysts form high-valent nickel species during electrolysis of HMF oxidation. On the other hand, the electrooxidation of HMF can be paired with HER via different catalysts as well. For instance, Deng et al. synthesized a “Nanoplatelet-on-Nanoarray” nickel-cobalt hydroxide-based catalyst (t-NiCo-MOF) by simple conversion of a bimetallic metal-organic framework (MOF) nanoarray (Deng et al., 2020b). They used t-NiCo-MOF as the anodic catalyst and MoNi4 as the cathodic catalyst to co-generate FDCA and H2 at a low voltage of 1.392V vs. RHE with a high current density of 100 mA/cm2, which was ∼300 mV lower than overall water splitting.
As competent bifunctional electrocatalysts for overall water splitting, Co-based catalysts have also been employed for coupled electrolysis in HMF oxidation and HER (Jiang et al., 2016; Kang et al., 2020). As early as 2016, Sun and co-workers (Jiang et al., 2016) reported an electrodeposited Co-P as the bifunctional electrocatalyst for integrated HMF oxidation and H2 evolution in a membrane-divided electrolyzer, which achieved nearly unity FE and selectivity for both H2 and FDCA production. Co3O4 nanowires have also been studied as bifunctional catalysts for HMF oxidation coupled with HER (Zhou et al., 2019). Surprisingly, the high concentration of HMF (100 mM) was realized by Co3O4 nanowires, which far surpassed the HMF concentration previously reported. In 2018, Weidner et al. investigated a series of cobalt-metalloid alloys (CoX; X = P, Si, B, As, Te) as electrocatalysts for the oxidation of HMF (Weidner et al., 2018). Among them, CoB showed the highest catalytic performance, which not only had high selectivity for FDCA but also suppressed the decomposition of HMF in alkaline electrolyte.
In addition to Ni- and Co-based catalysts, other transition metals, such as Mn-, Fe-, and Cu-based catalysts have also been reported as competitive catalysts for HMF oxidation. Choi and Kubota used MnOx as an anodic catalyst to achieve the conversion of HMF to FDCA in H2SO4 solution (pH = 1), showing the possibility of FDCA production in acidic media, which is beneficial to integrate with HER and solve the problem of incompatible electrolyte (Kubota and Choi, 2018). Only a few Fe containing catalysts display high catalytic activity for HMF oxidation. NiFe layered double hydroxide (LDH) was utilized for HMF oxidation, achieving 99.4% FE for HMF conversion (Liu et al., 2018). Meanwhile, they used the benchmark HER catalyst, Pt, as the cathode to produce H2. Cu has excellent conductivity and is relatively inactive to water oxidation, which may achieve HMF oxidation with higher efficiency when acted as electrocatalysts (Nam et al., 2018). A typical example is that CuxS@NiCo-LDH core-shell nanoarrays, which approached a current density of 10 mA/cm2 at a voltage of 1.34V vs. RHE, yielding nearly unity FE towards both FDCA and H2 (Deng et al., 2020a).
Paired HMF reduction with HMF oxidation
The reduction of HMF is mainly producing biofuels (DMF and DHMTHF), polymer precursors (BHMF and HD), and various organic solvents (Roylance and Choi, 2016b; Goyal et al., 2016; Hu et al., 2018; Xia et al., 2018). The diversity of the products of HMF reduction have obtained extensive research interest due to their wide application prospects (Roylance and Choi, 2016a; Zhang L. et al., 2019b; Zhang Y.-R. et al., 2019e). However, compared with the electrocatalytic oxidation of HMF, the electrochemical reduction of HMF is still at its early stage. In 2013, the electrocatalytic reduction of HMF was first studied by Koper’s group using a series of pure metal electrodes under neutral conditions (Kwon et al., 2013). Subsequently, they investigated the catalytic effects of these metals in acidic solutions and found that the overpotentials for HMF hydrogenation in acidic electrolyte were much lower than that in neutral solutions (Kwon et al., 2015). Among those pure metal electrodes, Ag electrode showed the highest selectivity and conversion for the formation of BHMF. Later, Chio and co-workers modified the silver electrode through galvanic displacement method (Aggd) for electrocatalytic HMF reduction (Roylance et al., 2016). The resulting Aggd approached high yield (99%) and FE (99%) for BHMF at -1.3 V vs. Ag/AgCl in a slightly alkaline solution. However, electrochemical reduction of HMF is typically paired with OER, which has sluggish kinetics and the unvalued product (Han et al., 2020). Therefore, coupling HMF reduction with HMF oxidation is able to obtain two value-added products and avoid the slow kinetics of OER.
Although replacing OER with HMF oxidation is a feasible strategy, there are still several challenges which need to overcome to develop paired electrolysis. For instance, the optimal potentials and current densities of the two half-reactions in paired electrocatalysis are unmatched. The well-developed redox mediators are suitable solutions for mismatched problems in paired electrolysis. It was reported that TEMPO (2,2,6,6-tetramethylpiperidine-1-oxyl) and its derivatives (like ACT, 4-acetamido-TEMPO) can work as redox mediators for the electrocatalytic HMF oxidation in mildly alkaline electrolytes due to their rapid redox kinetics, high solubility in water, remarkable stability, and suitable redox potentials (Cha and Choi, 2015; Cardiel et al., 2019). As aforementioned, Ag-based electrode demonstrated excellent catalytic capacity for electrohydrogenation of HMF to BHMF in the same electrolyte (Roylance et al., 2016). Therefore, Li’s group utilized Ag nanoparticles immobilized on carbon black (Ag/C) as the catalyst for electrocatalytic reduction of HMF to BHMF under cathodic conditions. On the other hand, ACT acted as the redox mediator for HMF oxidation (the mechanism is shown in Figure 2A) on carbon felt electrode (Chadderdon et al., 2019). Thus, the ACT-mediated indirect electrooxidation of HMF is insensitive to the anode potentials. With the precise control of the cathode potentials, it was feasible to couple the electrohydrogenation of HMF to BHMF with the oxidation of HMF to FDCA in a single divided cell. The paired electrolysis of HMF achieved 85% yield for BHMF and 98% yield for FDCA, respectively, as well as a combined electron efficiency of 187%, which is the highest electron efficiency for HMF conversion (Figures 2B,C).
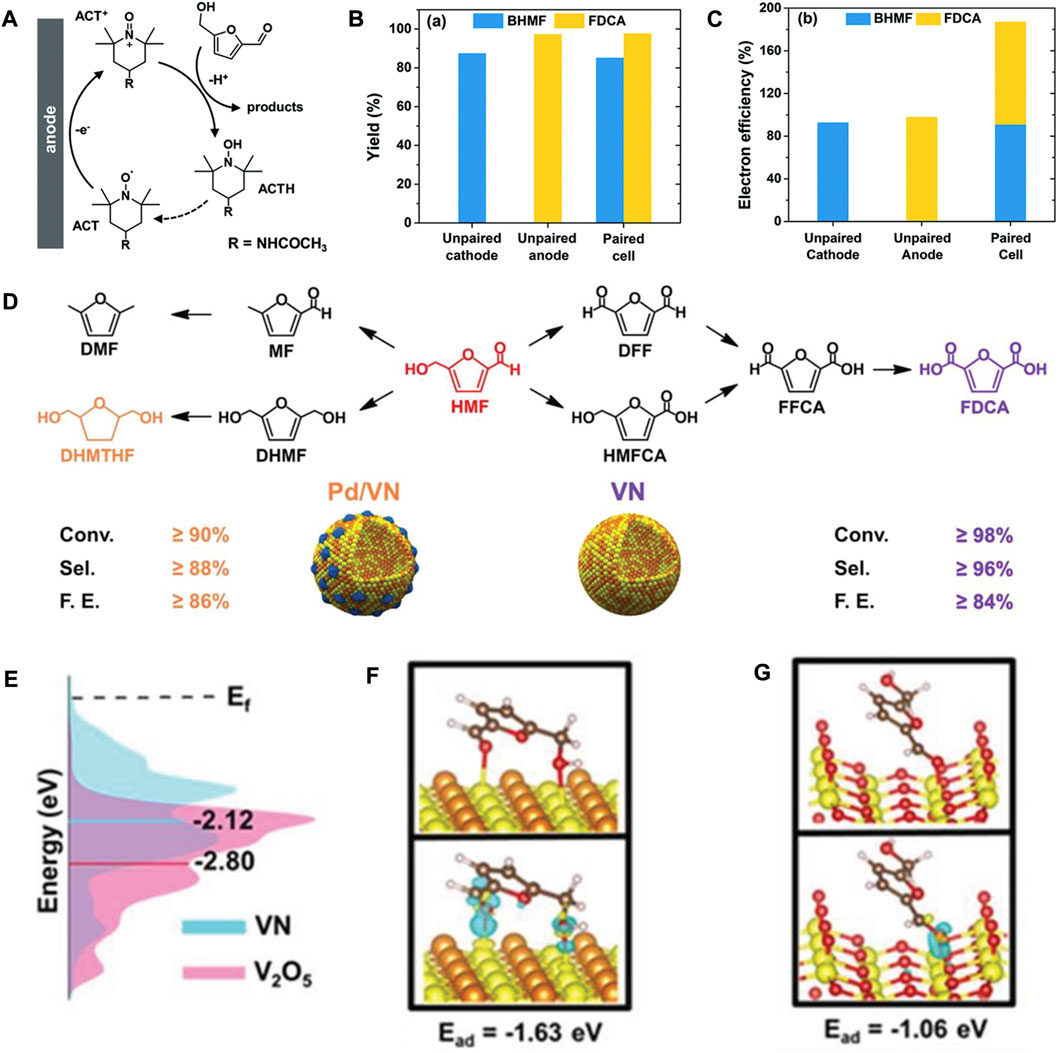
FIGURE 2. (A) Schematic diagram of the ACT-mediated electrocatalytic oxidation of HMF. (B) The yields of BHMF and FDCA in unpaired and paired cells. (C) The electron efficiencies of BHMF and FDCA in unpaired and paired cells. Adapted with permission from Chadderdon et al. (2019). Copyright 2019 Royal Society of Chemistry. (D) Electrocatalytic hydrogenation (left) and electrocatalytic oxidation (right) of HMF over Pd/VN and VN electrocatalysts, respectively. (E) Density of states plots of VN and V2O5. (F,G) Optimized structure (up) and charge density difference (bottom) of HMF on VN and V2O5, respectively. Adapted with permission from Li et al. (2019). Copyright 2019 John Wiley & Sons, Inc.
In the same year, Wang and co-workers successfully fabricated 3D vanadium nitride (VN) and Pd/VN hollow nanospheres (Figure 2D) as the anode and cathode, respectively, to electrocatalytically upgrade HMF into FDCA and DHMTHF in a bipolar membrane-divided electrolyzer (Li S. et al., 2019b). After electrolysis at 100 mA for 3 h, the conversion of HMF oxidation and reduction was 92% and 87%, respectively, with a high combined FE of ≥170%. For the unpaired HMF oxidation catalyzed by 3D VN, high conversion of HMF (≥98%) was obtained with the high selectivity (≥96%) and FE (≥84%) for FDCA after eight cycles. Compared to other vanadium-based catalysts, such as V2O5 and VOOH, the high performance of VN can be assigned to its low d-band center (Figure 2E), which facilitate the chemisorption and activation of HMF on VN surface (Figures 2F,G). For the unpaired electrocatalytic hydrogenation of HMF, the high selectivity (≥88%) and FE (≥86%) for DHMTHF were achieved with the help of 3D hollow Pd/VN. Notably, the Pd/VN catalyzed hydrogenation product is DHMTHF, which is different from the previously reported results. Additionally, the 3D hollow structure of electrocatalyst favors the diffusion and transport of substrates.
Summary and perspective
Recently, paired electrolysis has been widely investigated. In this mini-review, we have overviewed and focused on the recent progress of electrocatalytic oxidation of HMF paired with HER or HMF reduction. In paired cells, value-added products can be obtained on both anode and cathode simultaneously via electrocatalytic oxidation and hydrogenation, achieving a combined efficiency greater than 100%. Ideally, utilization of bifunctional electrocatalysts in paired electrolysis is more attractive due to its low cost and facile cell design. Mediated paired electrolysis is another strategy to solve the potential mismatch issues. In general, HMF can be oxidatively converted into DFF and FDCA over monometallic and bimetallic electrocatalysts, including noble metals and transition metals. The intrinsic nature of electrode has a great effect on the pathway of HMF oxidation. With the respect to the reductive upgrading of HMF, Ag exhibits remarkable selectivity to BHMF in slightly alkaline solutions. Overall, optimizing the performance of electrocatalysts to enhance their selectivity, catalytic activity, and stability, is still the main challenge. Although different electrodes have a strong influence on product selectivity and reaction pathway, other reaction conditions, such as mismatched potential and incompatibilities of electrolytes for the two half-reactions, product separation, and crossover issues will also limit large-scale applications for paired electrolysis. More efforts have been devoted to solve the aforementioned problems for industrial application. Additionally, theoretical simulations and in-situ/ex-situ characterization need to be performed to reveal the reaction mechanisms which are beneficial to design advanced catalysts.
Besides HER and HMF reduction reaction, the oxidation of HMF can also be coupled with CO2 reduction reactions (CO2RR), N2 reduction reactions (N2RR), and other organic reduction reactions (Zhang P. et al., 2019d; Xu et al., 2019; Choi et al., 2020). However, these pair-wise electrolysis studies are limited, and more electro-reductive coupling reactions (such as NO3−, NO reduction, etc.) for HMF should be considered. In addition, photoelectrolysis and bioelectrocatalysis have been also considered as promising alternatives for biomass upgrading (Roylance et al., 2016; Ozcan et al., 2017; Ma et al., 2018; Chen et al., 2020; Meng and Li, 2021; Meng et al., 2022). Moreover, the combination of electrocatalysis and biocatalysis for biomass upgrading can provide yields and selectivity that chemical catalysis cannot achieve (Schmitz et al., 2019).
Author contributions
DQ wrote the original draft of the manuscript. NJ and YH designed the scope and content of this review and edited the manuscript. SH, LC, and YY edited the manuscript. NJ, QG, and HC reviewed the final version of the review. All of the authors discussed the content and reviewed the manuscript.
Funding
This work was supported by the Start-up Funding of the Guizhou University (GZU), the Special Research fund of Natural Science for GZU. YY acknowledge the support from One Hundred Person Project of the Chinese Academy of Sciences (KY2310000043), National Natural Science Foundation of China (22172153), Users with Excellence Program of Hefei Science Center CAS (2021HSC-UE001), and the support from University of Science and Technology of China (USTC).
Conflict of interest
The authors declare that the research was conducted in the absence of any commercial or financial relationships that could be construed as a potential conflict of interest.
The reviewer [HL] declared a shared affiliation with the authors [NJ, DQ, LC, QG, HC] to the handling editor at the time of review.
Publisher’s note
All claims expressed in this article are solely those of the authors and do not necessarily represent those of their affiliated organizations, or those of the publisher, the editors and the reviewers. Any product that may be evaluated in this article, or claim that may be made by its manufacturer, is not guaranteed or endorsed by the publisher.
Supplementary material
The Supplementary Material for this article can be found online at: https://www.frontiersin.org/articles/10.3389/fchem.2022.1055865/full#supplementary-material
References
Adeogun, A. I., Agboola, B. E., Idowu, M. A., and Shittu, T. A. (2019). ZnCl2 enhanced acid hydrolysis of pretreated corncob for glucose production: Kinetics, thermodynamics and optimization analysis. J. Bioresour. Bioprod. 4 (3), 149–158. doi:10.12162/jbb.v4i3.003
Alamillo, R., Tucker, M., Chia, M., Pagan-Torres, Y., and Dumesic, J. (2012). The selective hydrogenation of biomass-derived 5-hydroxymethylfurfural using heterogeneous catalysts. Green Chem. 14 (5), 1413–1419. doi:10.1039/c2gc35039d
Amarasekara, A. S., Green, D., and McMillan, E. (2008). Efficient oxidation of 5-hydroxymethylfurfural to 2, 5-diformylfuran using Mn(III)-salen catalysts. Catal. Commun. 9 (2), 286–288. doi:10.1016/j.catcom.2007.06.021
Antonyraj, C. A., Jeong, J., Kim, B., Shin, S., Kim, S., Lee, K.-Y., et al. (2013). Selective oxidation of HMF to DFF using Ru/γ-alumina catalyst in moderate boiling solvents toward industrial production. J. Industrial Eng. Chem. 19 (3), 1056–1059. doi:10.1016/j.jiec.2012.12.002
Baga, B. (2020). Utilization of waste straw and husks from rice production: A review. J. Bioresour. Bioprod. 5 (3), 143–162. doi:10.1016/j.jobab.2020.07.001
Balakrishnan, M., Sacia, E. R., and Bell, A. T. (2012). Etherification and reductive etherification of 5-(hydroxymethyl)furfural: 5-(alkoxymethyl)furfurals and 2, 5-bis(alkoxymethyl)furans as potential bio-diesel candidates. Green Chem. 14 (6), 1626–1634. doi:10.1039/c2gc35102a
Barwe, S., Weidner, J., Cychy, S., Morales, D. M., Dieckhofer, S., Hiltrop, D., et al. (2018). Electrocatalytic oxidation of 5-(hydroxymethyl)furfural using high-surface-area nickel boride. Angew. Chem. Int. Ed. 57 (35), 11460–11464. doi:10.1002/anie.201806298
Binder, J. B., and Raines, R. T. (2009). Simple chemical transformation of lignocellulosic biomass into furans for fuels and chemicals. J. Am. Chem. Soc. 131 (5), 1979–1985. doi:10.1021/ja808537j
Bloor, L. G., Solarska, R., Bienkowski, K., Kulesza, P. J., Augustynski, J., Symes, M. D., et al. (2016). Solar-driven water oxidation and decoupled hydrogen production mediated by an electron-coupled-proton buffer. J. Am. Chem. Soc. 138 (21), 6707–6710. doi:10.1021/jacs.6b03187
Bozell, J. J. (2010). Connecting biomass and petroleum processing with a chemical bridge. Science 329 (5991), 522–523. doi:10.1126/science.1191662
Bozell, J. J., and Petersen, G. R. (2010). Technology development for the production of biobased products from biorefinery carbohydrates-the US Department of Energy's "Top 10" revisited. Green Chem. 12 (4), 539–554. doi:10.1039/b922014c
Cao, T., Wu, M., Ordomsky, V. V., Xin, X., Wang, H., Metivier, P., et al. (2017). Selective electrogenerative oxidation of 5-hydroxymethylfurfural to 2, 5-furandialdehyde. ChemSusChem 10 (24), 4851–4854. doi:10.1002/cssc.201702119
Cardiel, A. C., Taitt, B. J., and Choi, K.-S. (2019). Stabilities, regeneration pathways, and electrocatalytic properties of nitroxyl radicals for the electrochemical oxidation of 5-hydroxymethylfurfural. ACS Sustain. Chem. Eng. 7 (13), 11138–11149. doi:10.1021/acssuschemeng.9b00203
Casanova, O., Iborra, S., and Corma, A. (2009). Biomass into chemicals: Aerobic oxidation of 5-hydroxymethyl-2-furfural into 2, 5-furandicarboxylic acid with gold nanoparticle catalysts. ChemSusChem 2 (12), 1138–1144. doi:10.1002/cssc.200900137
Cha, H. G., and Choi, K.-S. (2015). Combined biomass valorization and hydrogen production in a photoelectrochemical cell. Nat. Chem. 7 (4), 328–333. doi:10.1038/nchem.2194
Chadderdon, X. H., Chadderdon, D. J., Pfennig, T., Shanks, B. H., and Li, W. (2019). Paired electrocatalytic hydrogenation and oxidation of 5-(hydroxymethyl)furfural for efficient production of biomass-derived monomers. Green Chem. 21 (22), 6210–6219. doi:10.1039/c9gc02264c
Chen, H., Simoska, O., Lim, K., Grattieri, M., Yuan, M., Dong, F., et al. (2020). Fundamentals, applications, and future directions of bioelectrocatalysis. Chem. Rev. 120 (23), 12903–12993. doi:10.1021/acs.chemrev.0c00472
Chen, X., Zhong, X., Yuan, B., Li, S., Gu, Y., Zhang, Q., et al. (2019). Defect engineering of nickel hydroxide nanosheets by ostwald ripening for enhanced selective electrocatalytic alcohol oxidation. Green Chem. 21 (3), 578–588. doi:10.1039/c8gc03451f
Chen, Y. X., Lavacchi, A., Miller, H. A., Bevilacqua, M., Filippi, J., Innocenti, M., et al. (2014). Nanotechnology makes biomass electrolysis more energy efficient than water electrolysis. Nat. Commun. 5, 4036. doi:10.1038/ncomms5036
Choi, S., Balamurugan, M., Lee, K.-G., Cho, K. H., Park, S., Seo, H., et al. (2020). Mechanistic investigation of biomass oxidation using nickel oxide nanoparticles in a CO2-saturated electrolyte for paired electrolysis. J. Phys. Chem. Lett. 11 (8), 2941–2948. doi:10.1021/acs.jpclett.0c00425
Connolly, T. J., Considine, J. L., Ding, Z., Forsatz, B., Jennings, M. N., MacEwan, M. F., et al. (2010). Efficient synthesis of 8-oxa-3-aza-bicyclo [3.2.1] octane hydrochloride. Org. Process Res. Dev. 14 (2), 459–465. doi:10.1021/op9002642
Corma, A., Iborra, S., and Velty, A. (2007). Chemical routes for the transformation of biomass into chemicals. Chem. Rev. 107 (6), 2411–2502. doi:10.1021/cr050989d
Davis, S. E., Houk, L. R., Tamargo, E. C., Datye, A. K., and Davis, R. J. (2011). Oxidation of 5-hydroxymethylfurfural over supported Pt, Pd and Au catalysts. Catal. Today 160 (1), 55–60. doi:10.1016/j.cattod.2010.06.004
Delidovich, I., Hausoul, P. J. C., Deng, L., Pfuetzenreuter, R., Rose, M., and Palkovits, R. (2016). Alternative monomers based on lignocellulose and their use for polymer production. Chem. Rev. 116 (3), 1540–1599. doi:10.1021/acs.chemrev.5b00354
Demirbas, A. (2001). Biomass resource facilities and biomass conversion processing for fuels and chemicals. Energy Convers. Manag. 42 (11), 1357–1378. doi:10.1016/s0196-8904(00)00137-0
Deng, X., Kang, X., Li, M., Xiang, K., Wang, C., Guo, Z., et al. (2020a). Coupling efficient biomass upgrading with H2 production via bifunctional CuxS@NiCo-LDH core-shell nanoarray electrocatalysts. J. Mat. Chem. A Mat. 8 (3), 1138–1146. doi:10.1039/c9ta06917h
Deng, X., Li, M., Fan, Y., Wang, L., Fu, X.-Z., and Luo, J.-L. (2020b). Constructing multifunctional 'Nanoplatelet-on-Nanoarray' electrocatalyst with unprecedented activity towards novel selective organic oxidation reactions to boost hydrogen production. Appl. Catal. B Environ. 278, 119339. doi:10.1016/j.apcatb.2020.119339
Du, Y., Sheng, H., Astruc, D., and Zhu, M. (2020). Atomically precise noble metal nanoclusters as efficient catalysts: A bridge between structure and properties. Chem. Rev. 120 (2), 526–622. doi:10.1021/acs.chemrev.8b00726
Du, Z., Ma, J., Wang, F., Liu, J., and Xu, J. (2011). Oxidation of 5-hydroxymethylfurfural to maleic anhydride with molecular oxygen. Green Chem. 13 (3), 554–557. doi:10.1039/c0gc00837k
Dubale, A. A., Zheng, Y., Wang, H., Huebner, R., Li, Y., Yang, J., et al. (2020). High‐performance bismuth‐doped nickel aerogel electrocatalyst for the methanol oxidation reaction. Angew. Chem. Int. Ed. 59 (33), 13891–13899. doi:10.1002/anie.202004314
El-Emam, R. S., and Ozcan, H. (2019). Comprehensive review on the techno-economics of sustainable large-scale clean hydrogen production. J. Clean. Prod. 220, 593–609. doi:10.1016/j.jclepro.2019.01.309
Elangovan, S., Topf, C., Fischer, S., Jiao, H., Spannenberg, A., Baumann, W., et al. (2016). Selective catalytic hydrogenations of nitriles, ketones, and aldehydes by well-defined manganese pincer complexes. J. Am. Chem. Soc. 138 (28), 8809–8814. doi:10.1021/jacs.6b03709
Gallo, J. M. R., Alonso, D. M., Mellmer, M. A., and Dumesic, J. A. (2013). Production and upgrading of 5-hydroxymethylfurfural using heterogeneous catalysts and biomass-derived solvents. Green Chem. 15 (1), 85–90. doi:10.1039/c2gc36536g
Gandini, A., Silvestre, A. J. D., Neto, C. P., Sousa, A. F., and Gomes, M. (2009). The furan counterpart of poly(ethylene terephthalate): An alternative material based on renewable resources. J. Polym. Sci. A. Polym. Chem. 47 (1), 295–298. doi:10.1002/pola.23130
Gandini, A. (2011). The irruption of polymers from renewable resources on the scene of macromolecular science and technology. Green Chem. 13 (5), 1061–1083. doi:10.1039/c0gc00789g
Gao, L., Deng, K., Zheng, J., Liu, B., and Zhang, Z. (2015). Efficient oxidation of biomass derived 5-hydroxymethylfurfural into 2, 5-furandicarboxylic acid catalyzed by merrifield resin supported cobalt porphyrin. Chem. Eng. J. 270, 444–449. doi:10.1016/j.cej.2015.02.068
Gao, L., Liu, Z., Ma, J., Zhong, L., Song, Z., Xu, J., et al. (2020). NiSe@NiOx core-shell nanowires as a non-precious electrocatalyst for upgrading 5-hydroxymethylfurfural into 2, 5-furandicarboxylic acid. Appl. Catal. B Environ. 261, 118235. doi:10.1016/j.apcatb.2019.118235
Gorbanev, Y. Y., Klitgaard, S. K., Woodley, J. M., Christensen, C. H., and Riisager, A. (2009). Gold-catalyzed aerobic oxidation of 5-hydroxymethylfurfural in water at ambient temperature. ChemSusChem 2 (7), 672–675. doi:10.1002/cssc.200900059
Goyal, R., Sarkar, B., Bag, A., Siddiqui, N., Dumbre, D., Lucas, N., et al. (2016). Studies of synergy between metal-support interfaces and selective hydrogenation of HMF to DMF in water. J. Catal. 340, 248–260. doi:10.1016/j.jcat.2016.05.012
Guo, W., Liu, H., Zhang, S., Han, H., Liu, H., Jiang, T., et al. (2016). Efficient hydrogenolysis of 5-hydroxymethylfurfural to 2, 5-dimethylfuran over a cobalt and copper bimetallic catalyst on N-graphene-modified Al2O3. Green Chem. 18 (23), 6222–6228. doi:10.1039/c6gc02630c
Han, J., Kim, Y., Jackson, D. H. K., Chang, H., Kim, H. W., Lee, J., et al. (2020). Enhanced catalytic performance and changed reaction chemistry for electrochemical glycerol oxidation by atomic-layer-deposited Pt-nanoparticle catalysts. Appl. Catal. B Environ. 273, 119037. doi:10.1016/j.apcatb.2020.119037
Heidary, N., and Kornienko, N. (2019). Operando Raman probing of electrocatalytic biomass oxidation on gold nanoparticle surfaces. Chem. Commun. 55 (80), 11996–11999. doi:10.1039/c9cc06646b
Hu, L., Xu, J., Zhou, S., He, A., Tang, X., Lin, L., et al. (2018). Catalytic advances in the production and application of biomass derived 2, 5-dihydroxymethylfuran. ACS Catal. 8 (4), 2959–2980. doi:10.1021/acscatal.7b03530
Hu, L., Zhao, G., Hao, W., Tang, X., Sun, Y., Lin, L., et al. (2012). Catalytic conversion of biomass-derived carbohydrates into fuels and chemicals via furanic aldehydes. RSC Adv. 2 (30), 11184–11206. doi:10.1039/c2ra21811a
Jiang, N., You, B., Boonstra, R., Rodriguez, I. M. T., and Sun, Y. (2016). Integrating electrocatalytic 5-hydroxymethylfurfural oxidation and hydrogen production via Co-P-derived electrocatalysts. ACS Energy Lett. 1 (2), 386–390. doi:10.1021/acsenergylett.6b00214
Jiao, Y., Zheng, Y., Jaroniec, M., and Qiao, S. Z. (2015). Design of electrocatalysts for oxygen- and hydrogen-involving energy conversion reactions. Chem. Soc. Rev. 44 (8), 2060–2086. doi:10.1039/c4cs00470a
Kang, M. J., Yu, H. J., Kim, H. S., and Cha, H. G. (2020). Deep eutectic solvent stabilised Co-P films for electrocatalytic oxidation of 5-hydroxymethylfurfural into 2, 5-furandicarboxylic acid. New J. Chem. 44 (33), 14239–14245. doi:10.1039/d0nj01426e
Kisszekelyi, P., Hardian, R., Vovusha, H., Chen, B., Zeng, X., Schwingenschlogl, U., et al. (2020). Selective electrocatalytic oxidation of biomass-derived 5-hydroxymethylfurfural to 2, 5-diformylfuran: From mechanistic investigations to catalyst recovery. ChemSusChem 13 (12), 3060. doi:10.1002/cssc.202001276
Kokoh, K. B., and Belgsir, E. T. (2002). Electrosynthesis of furan-2, 5-dicarbaldehyde by programmed potential electrolysis. Tetrahedron Lett. 43 (2), 229–231. doi:10.1016/s0040-4039(01)02106-2
Kong, X., Zhu, Y., Fang, Z., Kozinski, J. A., Butler, I. S., Xu, L., et al. (2018). Catalytic conversion of 5-hydroxymethylfurfural to some value-added derivatives. Green Chem. 20 (16), 3657–3682. doi:10.1039/c8gc00234g
Kong, X., Zhu, Y., Zheng, H., Dong, F., Zhu, Y., and Li, Y.-W. (2014). Switchable synthesis of 2, 5-dimethylfuran and 2, 5-dihydroxymethyltetrahydrofuran from 5-hydroxymethylfurfural over Raney Ni catalyst. RSC Adv. 4 (105), 60467–60472. doi:10.1039/c4ra09550b
Kubota, S. R., and Choi, K.-S. (2018). Electrochemical oxidation of 5-hydroxymethylfurfural to 2, 5-furandicarboxylic acid (FDCA) in acidic media enabling spontaneous FDCA separation. ChemSusChem 11 (13), 2138–2145. doi:10.1002/cssc.201800532
Kwon, Y., Birdja, Y. Y., Raoufmoghaddam, S., and Koper, M. T. M. (2015). Electrocatalytic hydrogenation of 5-hydroxymethylfurfural in acidic solution. ChemSusChem 8 (10), 1745–1751. doi:10.1002/cssc.201500176
Kwon, Y., de Jong, E., Raoufmoghaddam, S., and Koper, M. T. M. (2013). Electrocatalytic hydrogenation of 5-hydroxymethylfurfural in the absence and presence of glucose. ChemSusChem 6 (9), 1659–1667. doi:10.1002/cssc.201300443
Kwon, Y., Schouten, K. J. P., van der Waal, J. C., de Jong, E., and Koper, M. T. M. (2016). Electrocatalytic conversion of furanic compounds. ACS Catal. 6 (10), 6704–6717. doi:10.1021/acscatal.6b01861
Latsuzbaia, R., Bisselink, R., Anastasopol, A., van der Meer, H., van Heck, R., Yague, M. S., et al. (2018). Continuous electrochemical oxidation of biomass derived 5-(hydroxymethyl)furfural into 2, 5-furandicarboxylic acid. J. Appl. Electrochem. 48 (6), 611–626. doi:10.1007/s10800-018-1157-7
Lecomte, J., Finiels, A., Geneste, P., and Moreau, C. (1998). Selective hydroxymethylation of furfuryl alcohol with aqueous formaldehyde in the presence of dealuminated mordenites. Appl. Catal. A General 168 (2), 235–241. doi:10.1016/S0926-860X(97)00349-9
Lei, Q., Li, J., Cao, N., Song, Z., Liu, C.-L., and Dong, W.-S. (2020). Direct production of 2, 5-diformylfuran from fructose catalysed by Mo-based composite oxides in static air. Mol. Catal. 487, 110892. doi:10.1016/j.mcat.2020.110892
Li, M., Chen, L., Ye, S., Fan, G., Yang, L., Zhang, X., et al. (2019a). Dispersive non-noble metal phosphide embedded in alumina arrays derived from layered double hydroxide precursor toward efficient oxygen evolution reaction and biomass upgrading. J. Mat. Chem. A Mat. 7 (22), 13695–13704. doi:10.1039/c9ta03580j
Li, S., Sun, X., Yao, Z., Zhong, X., Coo, Y., Liang, Y., et al. (2019b). Biomass valorization via paired electrosynthesis over vanadium nitride-based electrocatalysts. Adv. Funct. Mat. 29 (42), 1904780. doi:10.1002/adfm.201904780
Liu, B., and Zhang, Z. (2016). One-Pot Conversion of Carbohydrates into furan derivatives via furfural and 5-hydroxylmethylfurfural as intermediates. ChemSusChem 9 (16), 2015–2036. doi:10.1002/cssc.201600507
Liu, W.-J., Dang, L., Xu, Z., Yu, H.-Q., Jin, S., and Huber, G. W. (2018). Electrochemical oxidation of 5-hydroxymethylfurfural with NiFe layered double hydroxide (LDH) nanosheet catalysts. ACS Catal. 8 (6), 5533–5541. doi:10.1021/acscatal.8b01017
Lu, Y., Dong, C.-L., Huang, Y.-C., Zou, Y., Liu, Y., Li, Y., et al. (2020). Hierarchically nanostructured NiO-Co3O4 with rich interface defects for the electro-oxidation of 5-hydroxymethylfurfural. Sci. China Chem. 63 (7), 980–986. doi:10.1007/s11426-020-9749-8
Luo, J., Monai, M., Wang, C., Lee, J. D., Duchon, T., Dvorak, F., et al. (2017). Unraveling the surface state and composition of highly selective nanocrystalline Ni-Cu alloy catalysts for hydrodeoxygenation of HMF. Catal. Sci. Technol. 7 (8), 1735–1743. doi:10.1039/c6cy02647h
Ma, B., Wang, Y., Guo, X., Tong, X., Liu, C., Wang, Y., et al. (2018). Photocatalytic synthesis of 2, 5-diformylfuran from 5-hydroxymethyfurfural or fructose over bimetallic Au-Ru nanoparticles supported on reduced graphene oxides. Appl. Catal. A General 552, 70–76. doi:10.1016/j.apcata.2018.01.002
Meng, Y., and Li, H. (2021). Electrocatalytic oxidation of biomass-derived 5-hydroxymethylfurfural to 2, 5-furandicarboxylic acid coupled with H2 evolution. Curr. Org. Chem. 25 (23), 2810–2814. doi:10.2174/1385272825666210927101643
Meng, Y., Yang, S., and Li, H. (2022). Electro- and photocatalytic oxidative upgrading of bio-based 5-hydroxymethylfurfural. ChemSusChem 15 (13), e202102581. doi:10.1002/cssc.202102581
Mika, L. T., Csefalvay, E., and Nemeth, A. (2018). Catalytic conversion of carbohydrates to initial platform chemicals: Chemistry and sustainability. Chem. Rev. 118 (2), 505–613. doi:10.1021/acs.chemrev.7b00395
Morais, A. R. C., da Costa Lopes, A. M., and Bogel-Lukasik, R. (2015). Carbon dioxide in biomass processing: Contributions to the green biorefinery concept. Chem. Rev. 115 (1), 3–27. doi:10.1021/cr500330z
Nam, D.-H., Taitt, B. J., and Choi, K.-S. (2018). Copper-based catalytic anodes to produce 2, 5-furandicarboxylic acid, a biomass-derived alternative to terephthalic acid. ACS Catal. 8 (2), 1197–1206. doi:10.1021/acscatal.7b03152
Nilges, P., and Schroeder, U. (2013). Electrochemistry for biofuel generation: Production of furans by electrocatalytic hydrogenation of furfurals. Energy Environ. Sci. 6 (10), 2925–2931. doi:10.1039/c3ee41857j
Ohyama, J., Esaki, A., Yamamoto, Y., Arai, S., and Satsuma, A. (2012). Selective hydrogenation of 2-hydroxymethyl-5-furfural to 2, 5-bis(hydroxymethyl)furan over gold sub-nano clusters. RSC Adv. 3 (4), 1033–1036. doi:10.1039/c2ra22190j
Ohyama, J., Esaki, A., Yamamoto, Y., Arai, S., and Satsuma, A. (2013). Selective hydrogenation of 2-hydroxymethyl-5-furfural to 2, 5-bis(hydroxymethyl)furan over gold sub-nano clusters. RSC Adv. 3 (4), 1033–1036. doi:10.1039/c2ra22190j
Ozcan, L., Yalcin, P., Alagoz, O., and Yurdakal, S. (2017). Selective photoelectrocatalytic oxidation of 5-(hydroxymethyl)-2-furaldehyde in water by using Pt loaded nanotube structure of TiO2 on Ti photoanodes. Catal. Today 281, 205–213. doi:10.1016/j.cattod.2016.07.024
Park, M., Gu, M., and Kim, B.-S. (2020). Tailorable electrocatalytic 5-hydroxymethylfurfural oxidation and H2 production: Architecture-performance relationship in bifunctional multilayer electrodes. ACS Nano 14 (6), 6812–6822. doi:10.1021/acsnano.0c00581
Perret, N., Grigoropoulos, A., Zanella, M., Manning, T. D., Claridge, J. B., and Rosseinsky, M. J. (2016). Catalytic response and stability of nickel/alumina for the hydrogenation of 5-hydroxymethylfurfural in water. ChemSusChem 9 (5), 521–531. doi:10.1002/cssc.201501225
Rosatella, A. A., Simeonov, S. P., Frade, R. F. M., and Afonso, C. A. M. (2011). 5-Hydroxymethylfurfural (HMF) as a building block platform: Biological properties, synthesis and synthetic applications. Green Chem. 13 (4), 754–793. doi:10.1039/c0gc00401d
Roylance, J. J., and Choi, K.-S. (2016a). Electrochemical reductive amination of furfural-based biomass intermediates. Green Chem. 18 (20), 5412–5417. doi:10.1039/c6gc01541g
Roylance, J. J., and Choi, K.-S. (2016b). Electrochemical reductive biomass conversion: Direct conversion of 5-hydroxymethylfurfural (HMF) to 2, 5-hexanedione (HD) via reductive ring-opening. Green Chem. 18 (10), 2956–2960. doi:10.1039/c6gc00533k
Roylance, J. J., Kim, T. W., and Choi, K.-S. (2016). Efficient and selective electrochemical and photoelectrochemical reduction of 5-hydroxymethylfurfural to 2, 5-bis(hydroxymethyl)furan using water as the hydrogen source. ACS Catal. 6 (3), 1840–1847. doi:10.1021/acscatal.5b02586
Sajid, M., Zhao, X., and Liu, D. (2018). Production of 2, 5-furandicarboxylic acid (FDCA) from 5-hydroxymethylfurfural (HMF): Recent progress focusing on the chemical-catalytic routes. Green Chem. 20 (24), 5427–5453. doi:10.1039/c8gc02680g
Schmitz, L. M., Rosenthal, K., and Luetz, S. (2019). Enzyme-based electrobiotechnological synthesis. Adv. Biochem. Eng. Biotechnol. 167, 87–134. doi:10.1007/10_2017_33
Scholz, D., Aellig, C., and Hermans, I. (2014). Catalytic transfer hydrogenation/hydrogenolysis for reductive upgrading of furfural and 5-(hydroxymethyl)furfural. ChemSusChem 7 (1), 268–275. doi:10.1002/cssc.201300774
Shi, Y., and Zhang, B. (2016). Correction: Recent advances in transition metal phosphide nanomaterials: Synthesis and applications in hydrogen evolution reaction. Chem. Soc. Rev. 45 (6), 1781. doi:10.1039/c6cs90013e
Su, -.M., Li, -.W., Ma, -.Q., and Zhu, -.B. (2020). Production of jet fuel intermediates from biomass platform compounds via aldol condensation reaction over iron-modified MCM-41 lewis acid zeolite. J. Bioresour. Bioprod. 5 (4), 256–265. doi:10.1016/j.jobab.2020.10.004
Taarning, E., Nielsen, I. S., Egeblad, K., Madsen, R., and Christensen, C. H. (2008). Chemicals from renewables: Aerobic oxidation of furfural and hydroxymethylfurfural over gold catalysts. ChemSusChem 1 (1-2), 75–78. doi:10.1002/cssc.200700033
Taitt, B. J., Nam, D.-H., and Choi, K.-S. (2019). A comparative study of nickel, cobalt, and iron oxyhydroxide anodes for the electrochemical oxidation of 5-hydroxymethylfurfural to 2, 5-furandicarboxylic acid. ACS Catal. 9 (1), 660–670. doi:10.1021/acscatal.8b04003
Villa, A., Schiavoni, M., Campisi, S., Veith, G. M., and Prati, L. (2013). Pd-modified Au on carbon as an effective and durable catalyst for the direct oxidation of HMF to 2, 5-furandicarboxylic acid. ChemSusChem 6 (4), 609–612. doi:10.1002/cssc.201200778
Vuyyuru, K. R., and Strasser, P. (2012a). Oxidation of biomass derived 5-hydroxymethylfurfural using heterogeneous and electrochemical catalysis. Catal. Today 195 (1), 144–154. doi:10.1016/j.cattod.2012.05.008
Wang, J., Liu, X., Jia, Z., Liu, Y., Sun, L., and Zhu, J. (2017a). Synthesis of bio-based poly(ethylene 2, 5-furandicarboxylate) copolyesters: Higher glass transition temperature, better transparency, and good barrier properties. J. Polym. Sci. Part A Polym. Chem. 55 (19), 3298–3307. doi:10.1002/pola.28706
Wang, T., Zhang, J., Xie, W., Tang, Y., Guo, D., and Ni, Y. (2017b). Catalytic transfer hydrogenation of biobased HMF to 2, 5-bis-(hydroxymethyl) furan over Ru/Co3O4. Catalysts 7 (3), 92. doi:10.3390/catal7030092
Weidner, J., Barwe, S., Sliozberg, K., Piontek, S., Masa, J., Apfel, U.-P., et al. (2018). Cobalt-metalloid alloys for electrochemical oxidation of 5-hydroxymethylfurfural as an alternative anode reaction in lieu of oxygen evolution during water splitting. Beilstein J. Org. Chem. 14, 1436–1445. doi:10.3762/bjoc.14.121
Xia, B., Zhang, Y., Shi, B., Ran, J., Davey, K., and Qiao, S.-Z. (2020). Photocatalysts for hydrogen evolution coupled with production of value-added chemicals. Small Methods 4 (7), 2000063. doi:10.1002/smtd.202000063
Xia, H., Xu, S., Hu, H., An, J., and Li, C. (2018). Efficient conversion of 5-hydroxymethylfurfural to high-value chemicals by chemo- and bio-catalysis. RSC Adv. 8 (54), 30875–30886. doi:10.1039/c8ra05308a
Xiang, X., He, L., Yang, Y., Guo, B., Tong, D., and Hu, C. (2011). A one-pot two-step approach for the catalytic conversion of glucose into 2, 5-diformylfuran. Catal. Lett. 141 (5), 735–741. doi:10.1007/s10562-011-0555-9
Xu, G.-R., Batmunkh, M., Donne, S., Jin, H., Jiang, J.-X., Chen, Y., et al. (2019). Ruthenium(iii) polyethyleneimine complexes for bifunctional ammonia production and biomass upgrading. J. Mat. Chem. A Mat. 7 (44), 25433–25440. doi:10.1039/c9ta10267a
Yang, P., Xia, Q., Liu, X., and Wang, Y. (2017). Catalytic transfer hydrogenation/hydrogenolysis of 5-hydroxymethylfurfural to 2, 5-dimethylfuran over Ni-Co/C catalyst. Fuel 187, 159–166. doi:10.1016/j.fuel.2016.09.026
Yang, Y., and Mu, T. (2021). Electrochemical oxidation of biomass derived 5-hydroxymethylfurfural (HMF): Pathway, mechanism, catalysts and coupling reactions. Green Chem. 23 (12), 4228–4254. doi:10.1039/d1gc00914a
You, B., Jiang, N., Liu, X., and Sun, Y. (2016a). Simultaneous H2 generation and biomass upgrading in water by an efficient noble-metal-free bifunctional electrocatalyst. Angew. Chem. Int. Ed. 55 (34), 9913–9917. doi:10.1002/anie.201603798
You, B., Liu, X., Jiang, N., and Sun, Y. (2016b). A general strategy for decoupled hydrogen production from water splitting by integrating oxidative biomass valorization. J. Am. Chem. Soc. 138 (41), 13639–13646. doi:10.1021/jacs.6b07127
You, B., Liu, X., Liu, X., and Sun, Y. (2017). Efficient H2 evolution coupled with oxidative refining of alcohols via a hierarchically porous nickel bifunctional electrocatalyst. ACS Catal. 7 (7), 4564–4570. doi:10.1021/acscatal.7b00876
You, B., and Sun, Y. (2018). Innovative strategies for electrocatalytic water splitting. Acc. Chem. Res. 51 (7), 1571–1580. doi:10.1021/acs.accounts.8b00002
You, B., Tang, M. T., Tsai, C., Abild-Pedersen, F., Zheng, X., and Li, H. (2019). Enhancing electrocatalytic water splitting by strain engineering. Adv. Mat. 31 (17), 1807001. doi:10.1002/adma.201807001
Yu, F., Yu, L., Mishra, I. K., Yu, Y., Ren, Z. F., and Zhou, H. Q. (2018). Recent developments in earth-abundant and non-noble electrocatalysts for water electrolysis. Mater. Today Phys. 7, 121–138. doi:10.1016/j.mtphys.2018.11.007
Zhang, J.-Y., He, T., Wang, M., Qi, R., Yan, Y., Dong, Z., et al. (2019a). Energy-saving hydrogen production coupling urea oxidation over a bifunctional nickel-molybdenum nanotube array. Nano Energy 60, 894–902. doi:10.1016/j.nanoen.2019.04.035
Zhang, L., Zhang, F., Michel, F. C., and Co, A. C. (2019b). Efficient electrochemical hydrogenation of 5-hydroxymethylfurfural to 2, 5-bis(hydroxymethyl)furan on Ag-displaced nanotextured Cu catalysts. ChemElectroChem 6 (18), 4739–4749. doi:10.1002/celc.201900640
Zhang, N., Zou, Y., Tao, L., Chen, W., Zhou, L., Liu, Z., et al. (2019c). Electrochemical oxidation of 5-hydroxymethylfurfural on nickel nitride/carbon nanosheets: Reaction pathway determined by in situ sum frequency generation vibrational spectroscopy. Angew. Chem. Int. Ed. 58 (44), 15895–15903. doi:10.1002/anie.201908722
Zhang, P., Sheng, X., Chen, X., Fang, Z., Jiang, J., Wang, M., et al. (2019d). Paired electrocatalytic oxygenation and hydrogenation of organic substrates with water as the oxygen and hydrogen source. Angew. Chem. Int. Ed. 58 (27), 9155–9159. doi:10.1002/anie.201903936
Zhang, Y.-R., Wang, B.-X., Qin, L., Li, Q., and Fan, Y.-M. (2019e). A non-noble bimetallic alloy in the highly selective electrochemical synthesis of the biofuel 2, 5-dimethylfuran from 5-hydroxymethylfurfural. Green Chem. 21 (5), 1108–1113. doi:10.1039/c8gc03689f
Zhang, Z., and Deng, K. (2015). Recent advances in the catalytic synthesis of 2, 5-furandicarboxylic acid and its derivatives. ACS Catal. 5 (11), 6529–6544. doi:10.1021/acscatal.5b01491
Zhou, B., Dong, C.-L., Huang, Y.-C., Zhang, N., Wu, Y., Lu, Y., et al. (2021). Activity origin and alkalinity effect of electrocatalytic biomass oxidation on nickel nitride. J. Energy Chem. 61, 179–185. doi:10.1016/j.jechem.2021.02.026
Keywords: 5-hydroxymethylfurfural, electrocatalytic hydrogenation, electrocatalytic oxidation, paired electrocatalysis, biomass valorization
Citation: Qu D, He S, Chen L, Ye Y, Ge Q, Cong H, Jiang N and Ha Y (2022) Paired electrocatalysis in 5-hydroxymethylfurfural valorization. Front. Chem. 10:1055865. doi: 10.3389/fchem.2022.1055865
Received: 28 September 2022; Accepted: 12 October 2022;
Published: 21 October 2022.
Edited by:
Ligang Feng, Yangzhou University, ChinaReviewed by:
Hui Xu, Changzhou University, ChinaZonghua Pu, Université du Québec, Canada
Hu Li, Guizhou University, China
Copyright © 2022 Qu, He, Chen, Ye, Ge, Cong, Jiang and Ha. This is an open-access article distributed under the terms of the Creative Commons Attribution License (CC BY). The use, distribution or reproduction in other forums is permitted, provided the original author(s) and the copyright owner(s) are credited and that the original publication in this journal is cited, in accordance with accepted academic practice. No use, distribution or reproduction is permitted which does not comply with these terms.
*Correspondence: Nan Jiang, bmppYW5nQGd6dS5lZHUuY24=; Yang Ha, eWFuZ2hhQGxibC5nb3Y=