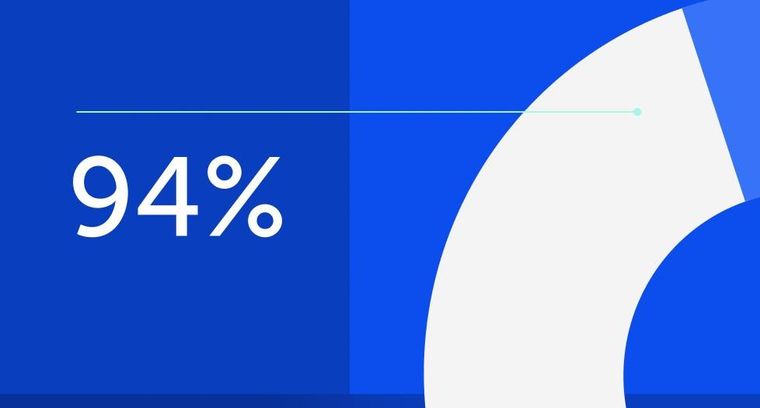
94% of researchers rate our articles as excellent or good
Learn more about the work of our research integrity team to safeguard the quality of each article we publish.
Find out more
ORIGINAL RESEARCH article
Front. Chem., 05 December 2022
Sec. Analytical Chemistry
Volume 10 - 2022 | https://doi.org/10.3389/fchem.2022.1050423
This article is part of the Research TopicSurface Enhanced Raman Scattering: Theory and ApplicationsView all 8 articles
The adsorption behaviour of the 5-fluorouracil (5FU) on small gold clusters AuN with N = 6, 8, 20 was evaluated by means of density functional theory using the PBE-D3 functional in combination with a mixed basis set, i.e. cc-pVDZ-PP for gold atoms and cc-pVTZ for non-metal elements. The binding energies between 5FU and gold clusters were determined in the range of 16–24 and 11–19 kcal/mol in gas-phase and aqueous media, respectively. The corresponding Gibbs energies were found to be around -7 to -10 kcal/mol in vacum and sigificantly reduced to -1 to -6 kcal/mol in water solution, indicating that both the association and dissociation processes are likely spontaneous. An analysis on the charge density difference tends to confirm the existence of a charge transfer from the 5FU molecule to Au atoms. Analysis of the surface-enhanced Raman scattering (SERS) spectra of 5FU adsorbed on the Au surfaces shows that the stretching vibrations of N−H and C=O bonds play a major role in the SERS phenomenon. A mechanism for the drug releasing from the gold surfaces is also proposed. The process is triggered by either the low pH in cancerous tumors or the presence of cysteine residues in protein matrices.
The 5-fluorouracil molecule, denoted as 5FU in Figure 1, which is commercialized under the trade name Adrucil, is one of the most widely used drugs in the cytotoxic chemotherapy to treat different cancers such as those of the colon, stomach, pancreas, breast, and esophagus (Vodenkova et al., 2020). The practical use of this drug has been well documented in both in vivo and in vitro studies (Al-Jumayli et al., 2022; Khodarahmi et al., 2022), increasing the importance of the compound in the field of medicinal chemistry (Li et al., 2021). In view of its widespread use, it is necessary to focus on its increasing biocompatibility and reduction of its undesirable therapeutic effects. Some common side effects including loss of appetite, low blood cell count, hair loss, inflammation of the mouth and skin, etc. Have been found (de Oliveira et al., 2021; Solhjoo et al., 2021). When topically used, it can cause skin irritation, especially during pregnancy (Oliveira et al., 2021). Therefore, it is extremely important to use the highly biocompatible, hydrophilic carriers to reduce such inherently damaged issues (Frydenberg et al., 2020; Wang et al., 2021). In addition to an improvement of the therapeutic efficacy of 5FU and a reduction of adverse events, it is also crucial to quickly detect its presence in a biological environment by a simple detection method (Yuksel et al., 2022).
Drug carriers play an important role in reaching the target area in the body as well as improving the therapeutic effect by adjustment of the optimal dose (Román et al., 2018; Zahed et al., 2018). While most polar drugs do not access cell membranes, nanomaterials can help solving this problem because of their high surface-to-volume ratio and low density. Furthermore, the small size, high stability and easily suitable surface modification properties of these nanomaterials also encourage their use as drug carriers (Al-Musawi et al., 2019; Horo et al., 2019).
For instance, the ability of the boron-fullerene B40 and its exohedral metallic derivatives (Au@B40 and Au4@B40) to carry 5FU was recently evaluated by DFT computations (Afarideh et al., 2018). Up to four Au atoms can be attached on the outer surface of the B40 cage at once to obtain the unique capability for delivering this molecule. Each Au atom has the potential to interact with one 5FU molecule when using only one B40 molecule. Due to the low binding energies and charge transfer, the 5FU drug can easily be detached from B40, Au@B40, and Au4@B40 at room temperature. The Ni-doped (8,0) boron nitride nanotube (Ni-BNNT) was employed as a 5FU adsorbent and sensor because it improved adsorption ability (up to −17 kcal/mol) against 5FU (Sanad et al., 2019). Additionally, a large charge transfer occurs between 5FU and Ni-BNNT (Aal, 2022). Due to their advantageous characteristics, the pure and encapsulated B36N36 nanoclusters have been recommended as potential new delivery systems and detectors for 5FU drugs. An important stage is the nature of the interactions between drugs and various nano-based materials that have been the subject of numerous recent reports (Chen et al., 2022; Nguyen et al., 2022; Shakerzadeh et al., 2022; Yuksel et al., 2022).
Of the currently known nanomaterials such as carbon nanotubes (Minhas et al., 2021), boron nitride nanotubes (Kaur et al., 2019; Zarghami Dehaghani et al., 2021), chitin-chitosan (Sargazi-Avval et al., 2021), silica (Mirhaji et al., 2018), ninosome, the gold-based nanoparticles (Manzano and Vallet-Regí, 2020; Onishi et al., 2020; Siddique and Chow, 2020) are becoming attractive owing to their high biocompatibility, facile synthesis with many different sizes and shapes (Du et al., 2018; Khoshnevisan et al., 2018). These unique properties and applications in medicine have been discussed thoroughly in several recent reports (Elahi et al., 2018; Freitas de Freitas et al., 2018; Lee et al., 2020). In fact, gold nanoparticles have been approved as appropriate materials for many essential applications in biomedicine, biosensors and imaging (Bai et al., 2020; Bansal et al., 2020; Zhang et al., 2020). In this context, it is important to elucidate the interaction mechanism, as well as the binding nature, of the drug and gold nanoparticles (Sotnikov et al., 2019; Taghizadeh et al., 2019; Nhat et al., 2020a; Chen et al., 2021; Hang et al., 2021). It has been found that the interactions are mainly stabilized by a charge transfer from the electron-rich atoms of ligands to Au metals (Nhat et al., 2020b; Si et al., 2021). In some other cases, the gold atoms can act as a proton acceptor giving rise to nonconventional hydrogen bonds (Pakiari and Jamshidi, 2007; Nhat et al., 2020a). Nevertheless the interaction mechanism of gold nanoparticles is not well understood yet. From a methodological viewpoint, a question of interest is as to whether the particle surface could be modelled by some small-size clusters.
A majority of 80–85% of the administered 5FU drug is quickly converted to dihydroflourouracil during 5FU metabolism (Kryachko and Remacle, 2005). Furthermore, a tiny fraction (1–3%) of the drug given is transformed into fluorodeoxyuridine monophosphate and fluorouridine triphosphate, thereby inhibiting DNA synthesis and RNA processing. Urine excretes the remaining 12–19% of the unmetabolized 5FU form (Si et al., 2021). The concentration of 5FU in untreated wastewater was evaluated to be close to 18 ng/L (Tendwa et al., 2021) The ambient 5FU cytostatic drug concentration amounts to ∼0.21 ng/L (Mazzuca et al., 2016). Numerous analytical methods (Rowney et al., 2009) have been used to detect 5-fluorouracil in blood sample, such as polarography (Franquet-Griell et al., 2015), amperometric (Dos Santos et al., 2022), photoluminescence (Ibrahim et al., 2018), high-performance liquid chromatography (Hanif et al., 2018; Liu et al., 2019), and gas chromatography-mass spectrometry (Gui et al., 2013). These techniques are effective, but they are costly due to the large testing costs, have limited throughput and take a long detection time because the sample must undergo complicated treatment procedures before analysis. Therefore, it is now important to devise a quick, economic and convenient approach for the 5FU detection.
The surface-enhanced Raman scattering (SERS) phenomenon occurs when a Raman active molecule is adsorbed on the surface of a nanoparticle, considerably enhancing its Raman intensities (Schlücker, 2014). The electromagnetic (EM) and charge transfer (CT) mechanisms emerge now as the two main explanations for the enhancing mechanism of SERS. According to the EM approach, the enhancement is mostly due to interactions between molecules and the massive surface resonance plasmons that an incoming laser causes to form on the substrate’s surface (Mullot et al., 2009). Following the CT approach, the increase also involves a significant charge transfer as during the associated electronic resonance transition between the molecules and the substrate (Schlücker, 2014). For the detection of a single molecule, the SERS technique appears to be a highly sensitive analytical technique because of its high enhancement efficiency (Weiss and Haran, 2001).
In this context, we set out to carry out in present study quantum chemical calculations with the aim to evaluate the binding mechanism between gold nanoparticles and the 5FU molecule by using the gold AuN clusters with N = 6, 8, 20 as models for the gold surfaces. In addition, the surface enhanced Raman scattering (SERS) mechanism and the applicability of gold nanoparticles for detection of the 5FU molecule are also elucidated.
All electronic structure calculations in this study are performed using density functional theory (DFT) with the PBE-D3 functional (Perdew et al., 1996; Grimme et al., 2010) including the dispersion correction (D3) in conjunction with the effective core potential (ECP) cc-pVDZ-PP (Peterson, 2003) for gold atoms and the cc-pVTZ basis set for other atoms using the Gaussian 16 package (Frisch et al., 2019). In the cc-pVDZ-PP basis set, the inner electrons along with the nucleus are considered as an inert core, and those on 5 s, 5p, 5 days, and 6Is orbitals of the Au atom are taken as valence electrons. The interaction between valence electrons and inert core is included in the pseudopotential which also takes care of the relativistic effects of the heavy gold atoms. This allows us to reduce greatly the number of electrons to be treated, and thereby computing times, but still describe properly the interactions between gold atoms and biomolecules (Nhat et al., 2020a; Nhat et al., 2020b). In addition, the Integral Equation Formalism-Polarizable Continuum Model (IEF-PCM) (Tomasi et al., 2005) is employed to investigate the effects of aqueous solvents. The density of states (DOS) are simulated by using the GaussSum program (O'boyle et al., 2008). The charge density difference (CDD) maps and energy decomposition analyses are obtained by using the Multiwfn 3.8 software (Lu and Chen, 2012).
The binding energies (Eb) is simply defined as the energy difference between those of the AuN-5FU complexes with respect to the free 5FU drug molecule and the AuN (N = 6, 8, 20) species:
where
The Gibbs free energy of the interaction are calculated as follow:
where
The energies of frontier states such as HOMO and LUMO, and the derivative HOMO–LUMO band gap (Eg) are also computed to examine the electronic responses during adsorptions. The Eg is a useful factor for determining the kinetic reactivity of materials (Hadipour et al., 2015), and its change upon the adsorption process can be used to evaluate the sensitivity of absorption.
Several theoretical and experimental studies (Scanlan and Hillier, 1984; Hadipour et al., 2015; Wielińska et al., 2019) have previously been conducted to determine the chemical structure of 5FU drug and at least eight different tautomers have been located (cf. Figure 2). Accordingly, the molecule exhibits a planar structure, containing a six-membered N-heterocylic ring (pyrimidine ring) with a fluorine substituent at the C5 position. This modification process is completely similar to that of DNA bases as a result of the hydrogen transfer within oxygen and nitrogen atoms (Pascu et al., 2005; Markova et al., 2010).
FIGURE 2. Optimized structures of 5FU and its tautomers. Values (kcal/mol) given in parentheses represent relative energies of 5FU tautomers at the PBE-D3/cc-pVTZ + ZPE level in vacuum/water solution with respect to 5FU.
Generally, the proton transfer in the 5FU molecule results in eight possible tautomers (Figure 2), including one di-keto (5FU), four keto-enols (5FU_1, 5FU_2, 5FU_7 and 5FU_8), and three di-enols (5FU_3, 5FU_4 and 5FU_5). The two rotational conformations (5FU_7 and 5FU_8) are also found upon rotation of the O−H group around the C-O group (5FU_1, 5FU_2). This is similar to that of 5FU_4 and 5FU_5 isomers. At the PBE-D3/cc-pVTZ level, the 5FU structure is 9–16 kcal/mol more stable than its tautomeric forms (gas-phase values). Such an energy gap is reduced to 1–8 kcal/mol in the aqueous solution. These computed results are fully consistent with both theoretical and experimental results previously reported (Scanlan and Hillier, 1984; Markova et al., 2010). The predicted distance of the C−F bond is 1.341 Å, which is comparable to the experimental value of 1.348 Å (Rastogi et al., 2000). The bond lengths of C2 = O and C4 = O are computed to be 1.220 and 1.221 Å, respectively, being somewhat longer than the experimental values of 1.212 and 1.211 Å (Rastogi and Palafox, 2011).
As reported earlier, the Au6 and Au8 clusters have planar structures, while the Au20 cluster gas prefers a pyramidal shape in their ground states (Rastogi and Palafox, 2011). To determine the favored binding sites between the gold clusters and the 5FU molecule, an analysis of NBO charge distribution (Rastogi et al., 2000) is first caried out and presented in Figure 3. The corner Au atoms (blue color) are found to bear a more positive charge than others (red color). According to previous studies (Nhat et al., 2017; Nhat et al., 2020b), the 5FU molecule is expected to interact with gold clusters by anchoring its electron-rich sites on the positively charged atoms of gold surfaces. In addition, the binding is in part stabilized due to the formation of some nonconventional X−H···Au hydrogen bonds. To evaluate the dynamic stability of the gold cluste-5FU complexes we carry out Born-Oppenheimer molecular dynamics simulations for Au6-5FU and Au8-5FU systems at 300 K. Simulation results that are collected in Figure S3 (SI file), show the connectivity between atoms in these systems remains unchanged during the simulation time, indicating that they are dynamically stable.
FIGURE 3. NBO charge distributions in AuN (N = 6, 8, 20) clusters. Color range given in a.u. Are: blue: more positive than 0.06; red: more negative than -0.06.
Four structures of AuN-5FU (N = 6, 8, 20) complexes obtained at the PBE-D3/cc-pVTZ/cc-pVDZ-PP level are displayed in Figure 4−6. Accordingly, the most stable structure of Au6-5FU complexes contains an Au−O bond constructed by anchoring the oxygen atom of the C2 = O group on the corner of Au6. This structure is almost planar with an Au−O bond length of 2.317 Å. The oxygen atom of the C4 = O group is the next preferred site for Au6 attack, resulting in the Au6-5FU_2 complex. This form is ∼1.0 kcal/mol less stable than Au6-5FU_1. The two remaining structures, namely Au6-5FU_3 and Au6-5FU_4, that can be considered as rotational isomers of Au6-5FU_1 and Au6-5FU_2, respectively, are lying around 2–5 kcal/mol above. A perpendicular anchor type between the Au6 cluster and the 5FU molecule is not found.
FIGURE 4. Low-lying structures of the Au6-5FU complex in gas-phase. Values given in parentheses are relative energies (kcal/mol) with respect to the most stable form Au6-5FU_1. Yellow, cyan, blue, white-gray, red, and magenta are used to represent the Au, C, N, H, O, and F atoms, respectively.
As in Au6-5FU, the oxygen atom of the C2 = O and C4 = O carbonyl groups is also the most preferred site for 5FU binding to Au8 (Figure 5). The lowest-energy Au8-5FU_1 has a binding energy of −24 kcal/mol, as compared to a corresponding value of −17 kcal/mol obtained for Au6-5FU_1 (Figure 4). This structure is stabilized mainly due to interaction of 2 C=O bonds with the Au8 cluster. The next stable complex Au8-5FU_2 is ∼3.4 kcal/mol higher in energy than Au8-5FU_1. Noticably, we find a new type of adsorption, i.e. Au8-5FU_3, in which the 5FU molecule is lying parallelly on the gold surface. However, such interaction induces a slight change in geometry of Au8 cluster. Similar to the Au6-5FU system, the C4 = O group is not a preferred site for binding to the gold atoms. Indeed, this binding pattern (Au8-5FU_4) has a relative energy of ∼9 kcal/mol higher than the most stable form.
FIGURE 5. Low-lying structures of the Au8-5FU complex in gas phase. Values indicated in parentheses are the relative energies (kcal/mol) with respect to the most stable isomer Au8-5FU_1. Yellow, cyan, blue, white-gray, red, and magenta are used to represent the Au, C, N, H, O, and F atoms, respectively.
For the interaction between the pyramidal Au20 and 5FU, four stable structures are identified and presented in Figure 6. The most stable form Au20-5FU_1 has a binding energy of around −17 kcal/mol. The next stable complex Au20-5FU_2 is formed by anchoring the O atom of the C4 = O group on a corner of Au20 is computed to be ∼2.3 kcal/mol less stable than Au20-5FU_1. The structure Au20-5FU_3 is also rather stable as located at ∼3 kcal/mol above Au20-5FU_1 and is basically considered as a rotational isomer of the latter. On the contrary, the resulting complexes that are formed by adsorption of 5FU on an Au atom at the edge of the Au20 cluster are much less stable, being ∼5 kcal/mol higher in energy.
FIGURE 6. Low-lying structures of the Au20-5FU complex in the gas phase. Values indicated in parentheses are the relative energies (kcal/mol) with respect to the most stable structure Au20-5FU_1. Yellow, cyan, blue, white-gray, red, and magenta are used to represent the Au, C, N, H, O, and F atoms, respectively.
Table 1 presents the calculated binding energy (Eb) and Gibbs free energy (∆G) for the 5FU adsorption on gold clusters obtained at the PBE-D3/cc-pVTZ/cc-pVDZ-PP level. The adsorption processes are exothermic indicating all stabilizing interactions. However, due to entropic effects, i.e. entropy is decreased during the adsorption, the Gibbs free energies turn out to be less negative as compared to corresponding binding enthalpies. In particular, the higher reactivity toward the 5FU molecules of Au8 in comparison with Au6 and Au20 clusters correlates with its smaller HOMO–LUMO gap and lower stability (Nhat et al., 2020a).
TABLE 1. Relative energy (RE), binding energy (Eb) and Gibbs free energy (ΔG) for the 5FU adsorption on gold clusters AuN with N = 6, 8, 20 in their optimized complexes (PBE-D3/cc-pVTZ/cc-pVDZ-PP, kcal/mol).
When including the influence of an aqueous solvent, both Eb and ΔG values become less negative than those computed in vacuum. The numerical data obtained for interactions of 5FU molecule and AuN (N = 6, 8, 20) clusters in a water environment presented in Table 1 show that the Au8 cluster also has the highest affinity with the 5FU molecule.
In the most stable complexes AuN-5FU_1 (N = 6, 8, 20), the bond lengths of Au−O amount to 2.317, 2.359 (2.417), and 2.404 Å in gas phase and 2.329, 2.337 (2.370), and 2.397 Å in water, respectively. Thus it can be seen that the bidentate formation of Au−O bonds in Au8-5FU_1 correlates well with its largest bonding energy. Overall, the bond lengths are predicted to be slightly longer than the sum of the covalent radii of oxygen (0.73 Å) and gold (1.44 Å) atoms (Pakiari and Jamshidi, 2007).
For deeper insights into the interaction mechanisms, we now consider the energies of frontier orbitals, namely the HOMO and LUMO, in both isolated components and resulting products. The change of the HOMO−LUMO energy gap (ΔEg) of gold clusters during the 5FU adsoption is computed using the following equation:
where
In gas-phase, the band gap of Au8 is predicted to be 1.4 eV (Table 2), which is significantly smaller than the corresponding values of 2.1 and 1.8 eV for Au6 and Au20, respectively. The result is thus correlates well with the largest binding energy of 5FU to Au8 cluster as analyzed above. Energies of frontier orbitals in addition provide us with important information on the binding mechanism. During the adsorption, a charge transfer could be processed from the 5FU molecule to the gold atoms, or reversedly depending on the energy gap between frontier states of adsorbent and adsorbate.
TABLE 2. Energies (eV) of frontier orbitals (HOMO and LUMO), their band gap (Eg) and change of Eg upon 5FU adsorption on gold clusters.
In the gas phase, the energy difference between the HOMO of 5FU and LUMO of gold clusters amounts to about 1.7–2.3 eV, being smaller than that involving the HOMO of gold clusters and LUMO of 5FU, which ranges from 3.3 to 3.50 eV. This leads to a decrease in the band gaps of the gold clusters after adsorption with 5FU (Figure 7A). Thus, the charge densities are mainly transferred from the HOMO of the 5FU molecule to the LUMO of a gold cluster. Such a prediction is also elucidated by the charge density difference (CDD) map analysis (Figure 7B). The CDD is computed by the folowing equation:
where
FIGURE 7. Density of states, HOMO and LUMO (A) and charge density difference maps (B) for the most stable complexes, AuN-5FU_1. The Fermi energy (EFermi) level is corrected to the zero reference. Isovalue is equal to 0.01 au for MOs and to 0.005 au for CDDs.
A topological analysis for the most complexes AuN-5FU_1 (N = 6, 8, 20) is performed and shown in Supplementary Table S1 and Supplementary Figure S1 (Supplementary File S1). The presence of bond critical points (BCPs) between Au, O and H atoms involved in intermolecular interactions in their complexes, as shown in Supplementary Figure S1, suggests that they occur. Two interactions between the gold and O, H atoms Au⋅⋅⋅O, Au⋅⋅⋅H are mainly responsible for these complex stabilization (Supplementary Figure S1). Such interactions have electron densities ρr that are quite low, 0.014–0.020 a.u. For Au⋅⋅⋅H and 0.049–0.053 a.u. For Au⋅⋅⋅O, indicating very weak interactions. As a result, for example Au8-5FU_1 complex, intermolecular interaction energy (Eint) of these bonds around -11 kcal/mol (see in SI file). The Laplacian of electron density (
The energy decomposition analysis (EDA) is considered as an effective method for a quantitative interpretation of chemical bonding in terms of three main components (Morokuma, 1971; Ziegler and Rauk, 1977; Zhao et al., 2018). Accordingly, the instantaneous interaction energy ΔEint between two fragments A and B in a molecule A–B is partitioned in three terms including 1) the quasi-classical electrostatic interaction ΔEelstat between the fragments; 2) the repulsive exchange (Pauli) interaction ΔEPauli between electrons of the two fragments having the same spin, and 3) the orbital (covalent) interaction ΔEorb which comes from the orbital relaxation and the orbital mixing between the fragments. The latter term can be decomposed into contributions from orbitals with different symmetry which make it possible to distinguish between σ, π, and δ bonding. Thus, ΔEint is calculated according to the following expression (Liu, 2007):
Our EDA analysis results are performed using Multiwfn software (Lu and Chen, 2012). For example, the interaction energy of Au8-5FU complex is -24 kcal/mol. The orbital interaction energy -41 kcal/mol significantly stabilizes the product; while the steric term (sum of electrostatic interaction energy, Pauli repulsion energy and change in exchange-correlation energy), destabilizes the adduct by 17 kcal/mol (see Supplementary Table S2 in SI file).
Analyses of the vibrational signatures and the surface-enhanced Raman scattering (SERS) spectra are the effective tools for the rationalization of the drug binding mechanism on nanoparticles surfaces. In principle, Raman spectroscopy allows us to detect vibrational features of functional groups in organic compounds. However, when molecules are present at very low concentrations, this technique is naturally restricted due to the intrinsically weak Raman intensity. In this situation, the Raman intensities are often magnified by the use of the SERS techniques (Mohammadi et al., 2018). In order to interpret the SERS phenomenon, both chemical and electromagnetic enhancement mechanisms are frequently taken into accounts (Farquharson et al., 2008). A number of theoretical and experimental studies on the Raman and SERS spectra of 5FU molecule have been carried out (Farquharson et al., 2005; Cialla et al., 2014). In the present study, both Raman and SERS spectra of the 5FU molecule and its complexes in the range of 400–3600 cm−1 are simulated and illustrated in Figure 8, while details of some significant signals are provided in Table 3. The experimental data for free 5FU are also included in Table 3 for a comparison.
FIGURE 8. Raman signatures of 5FU (A) and its SERS spectra on AuN (N = 6, 8, 20) surfaces (B–D) in aqueous solution.
TABLE 3. Selected Raman frequencies (in cm−1) and intensities (in arbitrary unit) for 5FU and its complexes Au6-5FU_1 computed in aqueous solution.
The Raman spectrum of 5FU is basically characterized by typical signals in the regions of 400–800, 1000–1800 and 3000–3600 cm−1. Several peaks located above 3000 cm−1 are due to the ν(C−H), ν(N1−H) and ν(N3−H) stretching modes. In particular, the calculated ν(C−H) stretching at 3156 cm−1 can be assigned to the experimental value of 3067 cm−1 (Farquharson et al., 2008). Both ν(N1−H) and ν(N3−H) modes are predicted to stretch at 3547 and 3503 cm−1. Because the H atom in the N3−H bond is involved in an intermolecular hydrogen bond, the wavenumber at 3503 cm−1 of ν(N3−H) is smaller than that of ν(N1−H), which is consistent with the experimental data of 3022 (N3−H bond) and 3146 cm−1 (N1−H bond) (Pavel et al., 2006). However, the calculated results of these bond stretchings are larger than the experimental counterpart (Rastogi et al., 2000).
Other important bands are related to the C=O stretching vibrations. As shown in Figure 8, these modes are observed at 1719 and 1675 cm−1, which are comparable to the experimental values of 1725 cm−1 and 1672 cm−1 (Rastogi et al., 2000). On the contrary, the peaks centered at 1652 and 1224 cm−1 that arise from the C=C and C−F stretching modes, agree well with the measured values at 1658 and 1224 cm−1, respectively (Rastogi et al., 2000). In particular, the highest peak observed at 731 cm−1 is due to the pyrimidine ring in-plane-deformation, and could accordingly be assigned to the recorded signal at 738 cm−1 (Rastogi and Palafox, 2011).
Some significant variations in the SERS spectra are identified when compared with the normal Raman one as illustrated Figures 8B–D. For example, the N1−H stretching mode in the Au6-5FU_1 complex is strongly shifted to a lower energy region near 3188 cm−1, as compared to a corresponding value centered at 3548 cm−1 in free 5FU. The C=O stretching of carbonyl groups also changes significantly. Indeed, the peaks associated with the C2 = O and C4 = O bonds are significantly shifted, in opposite directions, from 1719 to 1675 cm−1 in free 5FU to 1620 (decreased by 99 cm−1) and 1697 cm−1 (increased by 22 cm−1) in Au6-5FU_1. Other vibrations including the N1−H bending β(N1−H), C6−H bending β(C6−H) and pyrimidine ring in-plane-deformation δ(ring), that are observed at 1399, 1318 and 737 cm−1 in Au6-5FU_1. However, they are slightly changed following adsorption on gold surfaces as they are not directly involved in the interactions (Table 3). More importantly, significant modifications of the intensities are also observed. In the SERS spectra, the N−H stretching modes turn out to be the highest intensity bands, rather than the overlapped bands around 800 cm−1 arising from the in-plane-deformation of pyrimidine ring as in free 5FU. Such vibrations that are now centered at 3200–3400 cm−1, represent the most significant increase. Gold nanoparticles synthetized using polyethylene glycol surfactants have been used to detect several drugs such as rhodamine, 5FU and doxorubicin. It has been shown that both ν(C=O) and β(N1−H) signals are significantly enhanced when coated on the gold substrate (Rastogi and Palafox, 2011).
The drug releasing from the carrier in the target cells is one of the most important steps of a drug delivery process. In a human body, the drug can be separated from the carrier by either external or internal inducements such as the pH change or amino acid residues such as glutathione and cysteine (Rastogi et al., 2000). As a result of an extensive lactic production, tumor cells are typically more acidic than the normal ones. Indeed, the pH of cancer cells is normally smaller than 6 as compared to values around 7.4 in blood (Swietach et al., 2014). In an acidic media, protons can attack any rich-electron site of the 5FU molecule, but it appears that the C2 = O bond emerges as the most preferred site for protonation (Figure 9A). Therefore, we now examine the effects of protons on the stability of AuN-5FU products by protonation of the C2 = O, and then carry out geometry optimizations for the resulting AuN-5FU(+H+) complexes. As shown in Figure 9B, interactions between the 5FU molecule and gold atoms are now mainly predominated by weaker H-bonds, with a binding energy of around −8.0 kcal/mol as compared to corresponding values from −11 to −19 kcal/mol in an aqueous environment. It is expected that the 5FU drug binding to the carrier turns out to be much more breakable in an acidic environment, and thereby it is able to be released faster.
FIGURE 9. Optimal structures of 5FU in an acidic environment (A) and their complexes with gold clusters (B). Values given in parentheses are binding energies (kcal/mol, PBE-D3/cc-pVTZ/pVDZ-PP + ZPE).
Another factor contributing crucially to drug release is an internal force related to amino acids present in the protein matrices (Ghosh et al., 2008). Some sulfur based-amino acid constituents such as cysteine and methionine are found to be the most preferred binding sites for metal particles (Ghosh et al., 2008; Swietach et al., 2014). Cysteine is a relatively strong acid with the acid dissociation constants of pK1 = 1.7 and pK2 = 8.3 (O'Neil, 2013). Therefore, in biological systems, it likely emerges in its deprotonated forms by a proton cleavage of either the carboxyl or thiol group (Eckhardt et al., 2013). For further insights into the drug release mechanism from the gold surfaces, we consider the following ligand exchange reaction:
The resulting complexes of AuN clusters with the deprotonated form of cysteine, denoted as Cys(−H+), are illustrated in Figure 10. In agreement with recent reports (Nhat et al., 2020a; Si et al., 2021; Le Guével et al., 2011), these systems are mainly stabilized due to a covalent bond between the S-atom of the thiol group and the corner Au atoms. In aqueous solution, the binding energies with Cys (−H+) are predicted to be around −45, −47, and −42 kcal/mol for Au6, Au8 and Au20, respectively, that are much more negative as compared to the corresponding values obtained for a 5FU adsorption. Under neutral condition, the largest binding energies of 5FU to AuN cluster are situated in the range of −19 to −11 kcal/mol (Table 1), but significantly reduced to −8 kcal/mol in an acidic solution (Figure 9). The results clearly indicate that the gold affinity of cysteine residues is much stronger than that of the 5FU molecule. As a result, a drug release from a gold surface is expected to be spontaneous within the cancer cells upon protonation due to this internal inducement.
FIGURE 10. Resulting complexes of deprotonated cysteine Cys (−H+) with gold clusters AuN (N = 6, 8, 20). Binding energies (kcal/mol) are computed at the PBE-D3/cc-pVTZ/cc-pVDZ-PP + ZPE level.
In this theoretical study we attempted to elucidate the adsorption behaviours of the 5FU cancer drug on the Au nanostructured surfaces using three small gold clusters AuN (N = 6, 8, 20) as model reactants. The structures, energetics and spectoscopic properties were analyzed thoroughly by employing a functional with dispersion correction, namely the PBE-B3. The effects of solvent in an aqueous solutiuon were also evaluated using the IEF-PCM model.
In both vacuum and aqueous solution, the 5FU molecule tends to bind with gold atoms via the oxygen atom of the C2 = O group. The resulting complexes are also partially stabilized by some weak N−H∙∙∙Au couplings. Nonetheless, in an acidic environment, the Au⋅⋅⋅H−X with X = O, C interaction turns out to be predominant, instead of the stronger gas phase covalent Au−O bond. Indeed, the largest binding energy is greatly declined from −24 kcal/mol in gas phase to −19 and −8 kcal/mol in neutral and acidic media, respectively. Following 5FU adsorption, the band gap of gold clusters is greatly modified giving rise to a significant change in the electronic properties. Analysis of the frontier MOs showed that a charge transfer from the 5FU molecule to the gold clusters constitutes the main ingredient of the 5FU−Au interaction. A mechanism for drug release from the gold surface has also been proposed and elucidated. Accordingly, such a process is triggered by either the cysteine residues present in protein matrices or the low pH in cancerous tissues.
An examination on the SERS spectra also allowed us to verify the preferable orientation of 5FU molecules on the gold surface. As being directly oriented to the gold surface, the N−H stretching mode turns out to be the most dominant factor responsible for the SERS enhancement of the drug molecules. These results show that it is possible to use SERS spectroscopy to detect the 5FU molecule in an aqueous medium through the use of gold nanoparticles.
The original contributions presented in the study are included in the article/Supplementary Material, further inquiries can be directed to the corresponding author.
NS carried out computations, analysed the results and wrote the first draft. PN analysed the results and edited the draft. MN supervised the work, analysed the results and finalized the manuscript.
NTS was funded by Vingroup JSC and supported by the Master, PhD Scholarship Programme of Vingroup Innovation Foundation (VinIF), Institute of Big Data, code VinIF.2021.TS.058. The authors declare that the funder was not involved in the study design, collection, analysis, interpretation of data, the writing of this article, or the decision to submit it for publication.
NS is grateful to the Mississippi Center for Supercomputer United States for computing resources. MN thanks KU Leuven Belgium for support for open access publication, and is thankful to Van Lang University, Vietnam.
The authors declare that the research was conducted in the absence of any commercial or financial relationships that could be construed as a potential conflict of interest.
All claims expressed in this article are solely those of the authors and do not necessarily represent those of their affiliated organizations, or those of the publisher, the editors and the reviewers. Any product that may be evaluated in this article, or claim that may be made by its manufacturer, is not guaranteed or endorsed by the publisher.
The Supplementary Material for this article can be found online at: https://www.frontiersin.org/articles/10.3389/fchem.2022.1050423/full#supplementary-material
Aal, S. A. (2022). DFT study of the therapeutic potential of borospherene and metalloborospherenes as a new drug-delivery system for the 5-fluorouracil anticancer drug. J. Mol. Liq. 360, 119457. doi:10.1016/j.molliq.2022.119457
Afarideh, B., Rajabibazl, M., Omidi, M., Yaghmaee, B., Rahimpour, A., Khodabakhshi, R., et al. (2018). Anticancer activity of graphene oxide/5-FU on CT26 Ds-Red adenocarcinoma cell line. Orient. J. Chem. 34 (4), 2002–2007. doi:10.13005/ojc/3404038
Al-Jumayli, M., Choucair, K., Al-Obaidi, A., Park, R., Bansal, A., Baranda, J., et al. (2022). Pre-operative carboplatin/paclitaxel versus 5-fluorouracil (5-fu)-based chemoradiotherapy for older adults with esophageal cancer. Anticancer Res. 42 (1), 59–66. doi:10.21873/anticanres.15457
Al-Musawi, S., Hadi, A. J., Hadi, S. J., and Hindi, N. K. K. (2019). Preparation and characterization of folated chitosan-magnetic nanocarrier for 5-fluorouracil drug delivery and studying its effect in bladder cancer therapy. J. Glob. Pharm. Technol. 11, 628–637.
Bai, X., Wang, Y., Song, Z., Feng, Y., Chen, Y., Zhang, D., et al. (2020). The basic properties of gold nanoparticles and their applications in tumor diagnosis and treatment. Int. J. Mol. Sci. 21 (7), 2480. doi:10.3390/ijms21072480
Bansal, S. A., Kumar, V., Karimi, J., Singh, A. P., and Kumar, S. (2020). Role of gold nanoparticles in advanced biomedical applications. Nanoscale Adv. 2 (9), 3764–3787. doi:10.1039/d0na00472c
Chen, F., Si, P., de la Zerda, A., Jokerst, J. V., and Myung, D. (2021). Gold nanoparticles to enhance ophthalmic imaging. Biomater. Sci. 9 (2), 367–390. doi:10.1039/d0bm01063d
Chen, J., Qiu, M., Zhang, S., Li, B., Li, D., Huang, X., et al. (2022). A calcium phosphate drug carrier loading with 5-fluorouracil achieving a synergistic effect for pancreatic cancer therapy. J. Colloid Interface Sci. 605, 263–273. doi:10.1016/j.jcis.2021.07.080
Cialla, D., Pollok, S., Steinbrücker, C., Weber, K., and Popp, J. (2014). SERS-based detection of biomolecules. Nanophotonics 3 (6), 383–411. doi:10.1515/nanoph-2013-0024
de Oliveira, B. E., Amorim, O. H. J., Lima, L. L., Rezende, R. A., Mestnik, N. C., Bagatin, E., et al. (2021). 5-Fluorouracil, innovative drug delivery systems to enhance bioavailability for topical use. J. Drug Deliv. Sci. Technol. 61, 102155. doi:10.1016/j.jddst.2020.102155
Dos Santos, A. M., Junior, A. G. T., Carvalho, S. G., and Chorilli, M. (2022). An updated review on properties, nanodelivery systems, and analytical methods for the determination of 5-fluorouracil in pharmaceutical and biological samples. Curr. Pharm. Des. 28, 1501–1512. doi:10.2174/1381612828666220509150918
Du, Y., Xia, L., Jo, A., Davis, R. M., Bissel, P., Ehrich, M. F., et al. (2018). Synthesis and evaluation of doxorubicin-loaded gold nanoparticles for tumor-targeted drug delivery. Bioconjugate Chem. 29 (2), 420–430. doi:10.1021/acs.bioconjchem.7b00756
Eckhardt, S., Brunetto, P. S., Gagnon, J., Priebe, M., Giese, B., and Fromm, K. M. (2013). Nanobio silver: Its interactions with peptides and bacteria, and its uses in medicine. Chem. Rev. 113 (7), 4708–4754. doi:10.1021/cr300288v
Elahi, N., Kamali, M., and Baghersad, M. H. (2018). Recent biomedical applications of gold nanoparticles: A review. Talanta 184, 537–556. doi:10.1016/j.talanta.2018.02.088
Farquharson, S., Gift, A., Shende, C., Inscore, F., Ordway, B., Farquharson, C., et al. (2008). Surface-enhanced Raman spectral measurements of 5-fluorouracil in saliva. Molecules 13 (10), 2608–2627. doi:10.3390/molecules13102608
Farquharson, S., Shende, C., Inscore, F. E., Maksymiuk, P., and Gift, A. (2005). Analysis of 5-fluorouracil in saliva using surface-enhanced Raman spectroscopy. J. Raman Spectrosc. 36 (3), 208–212. doi:10.1002/jrs.1277
Franquet-Griell, H., Gómez-Canela, C., Ventura, F., and Lacorte, S. (2015). Predicting concentrations of cytostatic drugs in sewage effluents and surface waters of Catalonia (NE Spain). Environ. Res. 138, 161–172. doi:10.1016/j.envres.2015.02.015
Freitas de Freitas, L., Varca, G. H. C., dos Santos Batista, J. G., and Benévolo Lugão, A. (2018). An overview of the synthesis of gold nanoparticles using radiation technologies. Nanomaterials 8 (11), 939. doi:10.3390/nano8110939
Frisch, M. J., Trucks, G. W., Schlegel, H. B., Scuseria, G. E., Robb, M. A., Cheeseman, J. R., et al. (2019). Gaussian 16, rev. C.01. Wallingford CT: Gaussian, Inc.
Frydenberg, H., Harsem, N. K., Ofigsbø, Å., Skoglund, H., Brændengen, M., Kaasa, S., et al. (2020). Chemotherapy during pregnancy for advanced colon cancer: A case report. Clin. Colorectal Cancer 19 (2), 141–144. doi:10.1016/j.clcc.2020.02.013
Ghosh, P., Han, G., De, M., Kim, C. K., and Rotello, V. M. (2008). Gold nanoparticles in delivery applications. Adv. Drug Deliv. Rev. 60 (11), 1307–1315. doi:10.1016/j.addr.2008.03.016
Grimme, S., Antony, J., Ehrlich, S., and Krieg, H. (2010). Fundamentals of time-dependent density functional theory. J. Chem. Phys. 132, 1541041–15410419.
Gui, R., Wan, A., and Jin, H. (2013). Retracted Article: Facile synthesis of quantum dots/mesoporous silica/quantum dots core/shell/shell hybrid microspheres for ratiometric fluorescence detection of 5-fluorouracil in human serum. Analyst 138 (20), 5956–5964. doi:10.1039/c3an01089a
Hadipour, N. L., Ahmadi Peyghan, A., and Soleymanabadi, H. (2015). Theoretical study on the Al-doped ZnO nanoclusters for CO chemical sensors. J. Phys. Chem. C 119 (11), 6398–6404. doi:10.1021/jp513019z
Hang, N. T. N., Si, N. T., Nguyen, M. T., and Nhat, P. V. (2021). Adsorption/desorption behaviors and SERS chemical enhancement of 6-mercaptopurine on a nanostructured gold surface: The Au20 cluster model. Molecules 26 (17), 5422. doi:10.3390/molecules26175422
Hanif, M., Akhtar, M. F., Naeem, S., Wahid, M., Shehzad, M. A., Saadullah, M., et al. (2018). Development and validation of a new HPLC method for the detection of 5-fluorouracil in mobile phase and in plasma. Curr. Pharm. Anal. 14 (1), 3–7. doi:10.2174/1573412912666160922124552
Horo, H., Das, S., Mandal, B., and Kundu, L. M. (2019). Development of a photoresponsive chitosan conjugated prodrug nano-carrier for controlled delivery of antitumor drug 5-fluorouracil. Int. J. Biol. Macromol. 121, 1070–1076. doi:10.1016/j.ijbiomac.2018.10.095
Ibrahim, R. A. A-Z., Al-Hakeim, H. K., and Suhail, F. S. A. (2018). Anticancer drug 5-Fluorouracil in aqueous solution by differential pulse polarography: An assessment of optimum conditions. Nano Biomed. Eng. 10 (2), 117–128. doi:10.5101/nbe.v10i2.p117-128
James, A. M., and Lord, M. P. (1992). Macmillan's chemical and physical data. London, United Kingdom: Macmillan.
Kaur, J., Gill, G. S., and Jeet, K. (2019). “Applications of carbon nanotubes in drug delivery: A comprehensive review,” in Characterization and biology of nanomaterials for drug delivery (Amsterdam, Netherlands: Elsevier), 113–135.
Khodarahmi, M., Abbasi, H., Kouchak, M., Mahdavinia, M., Handali, S., and Rahbar, N. (2022). Nanoencapsulation of aptamer-functionalized 5-Fluorouracil liposomes using alginate/chitosan complex as a novel targeting strategy for colon-specific drug delivery. J. Drug Deliv. Sci. Technol. 71, 103299. doi:10.1016/j.jddst.2022.103299
Khoshnevisan, K., Daneshpour, M., Barkhi, M., Gholami, M., Samadian, H., and Maleki, H. (2018). The promising potentials of capped gold nanoparticles for drug delivery systems. J. Drug Target. 26 (7), 525–532. doi:10.1080/1061186x.2017.1387790
Kryachko, E., and Remacle, F. (2005). Three-gold clusters form nonconventional hydrogen bonds O–H⋯ Au and N–H⋯ Au with formamide and formic acid. Chem. Phys. Lett. 404 (1-3), 142–149. doi:10.1016/j.cplett.2005.01.061
Le Guével, X., Hötzer, B., Jung, G., Hollemeyer, K., Trouillet, V., and Schneider, M. (2011). Formation of fluorescent metal (Au, Ag) nanoclusters capped in bovine serum albumin followed by fluorescence and spectroscopy. J. Phys. Chem. C 115 (22), 10955–10963. doi:10.1021/jp111820b
Lee, K. X., Shameli, K., Yew, Y. P., Teow, S-Y., Jahangirian, H., Rafiee-Moghaddam, R., et al. (2020). <p>Recent developments in the facile bio-synthesis of gold nanoparticles (AuNPs) and their biomedical applications</p>. Int. J. Nanomedicine 15, 275–300. doi:10.2147/ijn.s233789
Li, J., Lu, F., Shao, X., and You, B. (2021). 5-FU@ DHA-UIO-66-NH2 potentiates chemotherapy sensitivity of breast cancer cells through a microRNA let-7a-dependent mechanism. Ann. Transl. Med. 9, 1761. doi:10.21037/atm-21-5978
Li, Q., Wang, J., Ding, Q., Chen, M., and Ma, F. (2017). Coupling effect on charge-transfer mechanism of surface-enhanced resonance Raman scattering. J. Raman Spectrosc. 48 (4), 560–569. doi:10.1002/jrs.5077
Liu, H., He, Y., and Cao, K. (2021). Flexible surface-enhanced Raman scattering substrates: A review on constructions, applications, and challenges. Adv. Mat. Interfaces 8 (21), 2100982. doi:10.1002/admi.202100982
Liu, L., Anwar, S., Ding, H., Xu, M., Yin, Q., Xiao, Y., et al. (2019). Electrochemical sensor based on F, N-doped carbon dots decorated laccase for detection of catechol. J. Electroanal. Chem. 840, 84–92. doi:10.1016/j.jelechem.2019.03.071
Liu, S. (2007). Steric effect: A quantitative description from density functional theory. J. Chem. Phys. 126 (24), 244103. doi:10.1063/1.2747247
Lu, T., and Chen, F. (2012). Multiwfn: A multifunctional wavefunction analyzer. J. Comput. Chem. 33 (5), 580–592. doi:10.1002/jcc.22885
Manzano, M., and Vallet-Regí, M. (2020). Mesoporous silica nanoparticles for drug delivery. Adv. Funct. Mat. 30 (2), 1902634. doi:10.1002/adfm.201902634
Markova, N., Enchev, V., and Ivanova, G. (2010). Tautomeric equilibria of 5-fluorouracil anionic species in water. J. Phys. Chem. A 114 (50), 13154–13162. doi:10.1021/jp1063879
Mazzuca, F., Borro, M., Botticelli, A., Mazzotti, E., Marchetti, L., Gentile, G., et al. (2016). Pre-treatment evaluation of 5-fluorouracil degradation rate: Association of poor and ultra-rapid metabolism with severe toxicity in a colorectal cancer patients cohort. Oncotarget 7 (15), 20612–20620. doi:10.18632/oncotarget.7991
Minhas, M. U., Abdullah, O., Sohail, M., Khalid, I., Ahmad, S., Khan, K. U., et al. (2021). Synthesis of novel combinatorial drug delivery system (nCDDS) for co-delivery of 5-fluorouracil and leucovorin calcium for colon targeting and controlled drug release. Drug Dev. Industrial Pharm. 47 (12), 1952–1965. doi:10.1080/03639045.2022.2072514
Mirhaji, E., Afshar, M., Rezvani, S., and Yoosefian, M. (2018). Boron nitride nanotubes as a nanotransporter for anti-cancer docetaxel drug in water/ethanol solution. J. Mol. Liq. 271, 151–156. doi:10.1016/j.molliq.2018.08.142
Mohammadi, A., Nicholls, D. L., and Docoslis, A. (2018). Improving the surface-enhanced Raman scattering performance of silver nanodendritic substrates with sprayed-on graphene-based coatings. Sensors 18 (10), 3404. doi:10.3390/s18103404
Morokuma, K. (1971). Molecular orbital studies of hydrogen bonds. III. C= O··· H–O hydrogen bond in H2CO··· H2O and H2CO··· 2H2O. J. Chem. Phys. 55 (3), 1236–1244. doi:10.1063/1.1676210
Mullot, J-U., Karolak, S., Fontova, A., Huart, B., and Levi, Y. (2009). Development and validation of a sensitive and selective method using GC/MS-MS for quantification of 5-fluorouracil in hospital wastewater. Anal. Bioanal. Chem. 394 (8), 2203–2212. doi:10.1007/s00216-009-2902-x
Nguyen, C-H., Banh, K-S., Dang, C-H., Nguyen, C-H., and Nguyen, T-D. (2022). β-cyclodextrin/alginate nanoparticles encapsulated 5-fluorouracil as an effective and safe anticancer drug delivery system. Arabian J. Chem. 15 (6), 103814. doi:10.1016/j.arabjc.2022.103814
Nhat, P. V., Nguyen, P. T. N., and Si, N. T. (2020). A computational study of thiol-containing cysteine amino acid binding to Au6 and Au8 gold clusters. J. Mol. Model. 26 (3), 58. doi:10.1007/s00894-020-4312-0
Nhat, P. V., Si, N. T., Leszczynski, J., and Nguyen, M. T. (2017). Another look at structure of gold clusters Aun from perspective of phenomenological shell model. Chem. Phys. 493, 140–148. doi:10.1016/j.chemphys.2017.06.009
Nhat, P. V., Si, N. T., Tram, N. T. T., Duong, L. V., and Nguyen, M. T. (2020). Elucidating the binding mechanism of thione-containing mercaptopurine and thioguanine drugs to small gold clusters. J. Comput. Chem. 41 (19), 1748–1758. doi:10.1002/jcc.26216
Nițică, Ș., Moldovan, A. I., Toma, V., Moldovan, C. S., Berindan-Neagoe, I., Știufiuc, G., et al. (2018). PEGylated gold nanoparticles with interesting plasmonic properties synthesized using an original, rapid, and easy-to-implement procedure. J. Nanomater. 2018, 1–7. doi:10.1155/2018/5954028
O'boyle, N. M., Tenderholt, A. L., and cclib, Langner K. M. (2008). cclib: A library for package-independent computational chemistry algorithms. J. Comput. Chem. 29 (5), 839–845. doi:10.1002/jcc.20823
O'Neil, M. J. (2013). The merck index: An encyclopedia of chemicals, drugs, and biologicals. London, United Kingdom: RSC Publishing.
Oliveira, M. M. B., de Araújo, A. A., Ribeiro, S. B., de Sales Mota, P. C. M., Marques, V. B., da Silva Martins Rebouças, C., et al. (2021). Losartan improves intestinal mucositis induced by 5-fluorouracil in mice. Sci. Rep. 11 (1), 23241–23312. doi:10.1038/s41598-021-01969-x
Onishi, H., Nagai, T., and Machida, Y. (2020). Applications of chitin, chitosan, and their derivatives to drug carriers for microparticulated or conjugated drug delivery systems. Applications of chitin and chitosan. Florida, United States: CRC Press, 205–231.
Pakiari, A., and Jamshidi, Z. (2007). Interaction of amino acids with gold and silver clusters. J. Phys. Chem. A 111 (20), 4391–4396. doi:10.1021/jp070306t
Pascu, M. L., Brezeanu, M., Voicu, L., Staicu, A., Carstocea, B., and Pascu, R. A. (2005). 5-fluorouracil as a phosensitiser. Vivo 19 (1), 215–220.
Pavel, I., Cota, S., Kiefer, W., and Cîntă-Pînzaru, S. (2006). SERS substrate-dependent interaction of the anticarcinogenic drug 5-fluorouracil with silver. Part. Sci. Technol. 24 (3), 301–309. doi:10.1080/02726350600840639
Perdew, J. P., Burke, K., and Ernzerhof, M. (1996). Generalized gradient approximation made simple. Phys. Rev. Lett. 77 (18), 3865–3868. doi:10.1103/physrevlett.77.3865
Peterson, K. A. (2003). Systematically convergent basis sets with relativistic pseudo-potentials. I. Correlation consistent basis sets for the post-d group 13–15 elements. J. Chem. Phys. 119 (21), 11099–11112. doi:10.1063/1.1622923
Rastogi, V., Jain, V., Yadav, R., Singh, C., and Palafox, M. A. (2000). Fourier transform Raman spectrum and ab initio and density functional computations of the vibrational spectrum, molecular geometry, atomic charges and some molecular properties of the anticarcinogenic drug 5-fluorouracil. J. Raman Spectrosc. 31 (7), 595–603. doi:10.1002/1097-4555(200007)31:7<595::aid-jrs582>3.0.co;2-9
Rastogi, V., and Palafox, M. A. (2011). Vibrational spectra, tautomerism and thermodynamics of anticarcinogenic drug: 5-Fluorouracil. Spectrochimica Acta Part A Mol. Biomol. Spectrosc. 79 (5), 970–977. doi:10.1016/j.saa.2011.04.008
Román, G., Noseda Grau, E., Brizuela, G., Juan, A., and Simonetti, S. (2018). A first-principles study of pristine and Al-doped activated carbon interacting with 5-Fluorouracil anticancer drug. Eur. Phys. J. E 41 (9), 107–116. doi:10.1140/epje/i2018-11718-4
Rowney, N. C., Johnson, A. C., and Williams, R. J. (2009). Cytotoxic drugs in drinking water: A prediction and risk assessment exercise for the thames catchment in the United Kingdom. Environ. Toxicol. Chem. 28 (12), 2733–2743. doi:10.1897/09-067.1
Sanad, M. F., Shalan, A. E., Bazid, S. M., Serea, E. S. A., Hashem, E. M., Nabih, S., et al. (2019). A graphene gold nanocomposite-based 5-FU drug and the enhancement of the MCF-7 cell line treatment. RSC Adv. 9 (53), 31021–31029. doi:10.1039/c9ra05669f
Sargazi-Avval, H., Yoosefian, M., Ghaffari-Moghaddam, M., Khajeh, M., and Bohlooli, M. (2021). Potential applications of armchair, zigzag, and chiral boron nitride nanotubes as a drug delivery system: Letrozole anticancer drug encapsulation. Appl. Phys. A 127 (4), 257–7. doi:10.1007/s00339-021-04402-2
Scanlan, M. J., and Hillier, I. H. (1984). An ab initio study of tautomerism of uracil, thymine, 5-fluorouracil, and cytosine. J. Am. Chem. Soc. 106 (13), 3737–3745. doi:10.1021/ja00325a005
Schlücker, S. (2014). Surface-Enhanced Raman spectroscopy: Concepts and chemical applications. Angew. Chem. Int. Ed. 53 (19), 4756–4795. doi:10.1002/anie.201205748
Shakerzadeh, E., Zborowski, K. K., Anota, E. C., and Nguyen, M. T. (2022). Pristine and alkali and alkaline Earth metals encapsulated B36N36 nanoclusters as prospective delivery agents and detectors for 5-fluorouracil anticancer drug. Appl. Organom. Chemis. 36 (7), e6721. doi:10.1002/aoc.6721
Si, N. T., Nhung, N. T. A., Bui, T. Q., Nguyen, M. T., and Nhat, P. V. (2021). Gold nanoclusters as prospective carriers and detectors of pramipexole. RSC Adv. 11 (27), 16619–16632. doi:10.1039/d1ra02172a
Siddique, S., and Chow, J. C. (2020). Gold nanoparticles for drug delivery and cancer therapy. Appl. Sci. 10 (11), 3824. doi:10.3390/app10113824
Solhjoo, A., Sobhani, Z., Sufali, A., Rezaei, Z., Khabnadideh, S., and Sakhteman, A. (2021). Exploring pH dependent delivery of 5-fluorouracil from functionalized multi-walled carbon nanotubes. Colloids Surfaces B Biointerfaces 205, 111823. doi:10.1016/j.colsurfb.2021.111823
Sotnikov, D. V., Berlina, A. N., Ivanov, V. S., Zherdev, A. V., and Dzantiev, B. B. (2019). Adsorption of proteins on gold nanoparticles: One or more layers? Colloids Surfaces B Biointerfaces 173, 557–563. doi:10.1016/j.colsurfb.2018.10.025
Swietach, P., Vaughan-Jones, R. D., Harris, A. L., and Hulikova, A. (2014). The chemistry, physiology and pathology of pH in cancer. Phil. Trans. R. Soc. B 369 (1638), 20130099. doi:10.1098/rstb.2013.0099
Taghizadeh, S., Alimardani, V., Roudbali, P. L., Ghasemi, Y., and Kaviani, E. (2019). Gold nanoparticles application in liver cancer. Photodiagnosis Photodyn. Ther. 25, 389–400. doi:10.1016/j.pdpdt.2019.01.027
Tendwa, M. B., Chebon-Bore, L., Lobb, K., Musyoka, T. M., and Bishop Ö, T. (2021). Force field parameters for Fe2+4S2−4 clusters of dihydropyrimidine dehydrogenase, the 5-fluorouracil cancer drug deactivation protein: A step towards in silico pharmacogenomics studies. Molecules 26 (10), 2929. doi:10.3390/molecules26102929
Tomasi, J., Mennucci, B., and Cammi, R. (2005). Quantum mechanical continuum solvation models. Chem. Rev. 105 (8), 2999–3094. doi:10.1021/cr9904009
Vodenkova, S., Buchler, T., Cervena, K., Veskrnova, V., Vodicka, P., and Vymetalkova, V. (2020). 5-fluorouracil and other fluoropyrimidines in colorectal cancer: Past, present and future. Pharmacol. Ther. 206, 107447. doi:10.1016/j.pharmthera.2019.107447
Wang, C., Shen, L., and Wu, L. (2021). Adsorption and sensing of an anticancer drug on the boron nitride nanocones; a computational inspection. Comput. Methods Biomechanics Biomed. Eng. 24 (2), 151–160. doi:10.1080/10255842.2020.1815716
Weiss, A., and Haran, G. (2001). Time-dependent single-molecule Raman scattering as a probe of surface dynamics. J. Phys. Chem. B 105 (49), 12348–12354. doi:10.1021/jp0126863
Wielińska, J., Nowacki, A., and Liberek, B. (2019). 5-fluorouracil—Complete insight into its neutral and ionised forms. Molecules 24 (20), 3683. doi:10.3390/molecules24203683
Yuksel, N., Kose, A., and Fellah, M. F. (2022). A Density Functional Theory study for adsorption and sensing of 5-Fluorouracil on Ni-doped boron nitride nanotube. Mater. Sci. Semicond. Process. 137, 106183. doi:10.1016/j.mssp.2021.106183
Zahed, F. M., Hatamluyi, B., Lorestani, F., and Es’haghi, Z. (2018). Silver nanoparticles decorated polyaniline nanocomposite based electrochemical sensor for the determination of anticancer drug 5-fluorouracil. J. Pharm. Biomed. Analysis 161, 12–19. doi:10.1016/j.jpba.2018.08.004
Zarghami Dehaghani, M., Yousefi, F., Sajadi, S. M., Tajammal Munir, M., Abida, O., Habibzadeh, S., et al. (2021). Theoretical encapsulation of fluorouracil (5-fu) anti-cancer chemotherapy drug into carbon nanotubes (cnt) and boron nitride nanotubes (bnnt). Molecules 26 (16), 4920. doi:10.3390/molecules26164920
Zhang, L., Mazouzi, Y., Salmain, M., Liedberg, B., and Boujday, S. (2020). Antibody-gold nanoparticle bioconjugates for biosensors: Synthesis, characterization and selected applications. Biosens. Bioelectron. 165, 112370. doi:10.1016/j.bios.2020.112370
Zhao, L., von Hopffgarten, M., Andrada, D. M., and Frenking, G. (2018). Energy decomposition analysis. WIREs Comput. Mol. Sci. 8 (3), e1345. doi:10.1002/wcms.1345
Keywords: 5-fluorouracil, 5FU, SERS spectra, gold clusters, binding mechanism, binding energy, DFT
Citation: Si NT, Nhat PV and Nguyen MT (2022) Binding mechanism and SERS spectra of 5-fluorouracil on gold clusters. Front. Chem. 10:1050423. doi: 10.3389/fchem.2022.1050423
Received: 21 September 2022; Accepted: 21 November 2022;
Published: 05 December 2022.
Edited by:
Václav Ranc, Palacký University, CzechiaReviewed by:
Osvaldo Andres Yáñez Osses, University of the Americas, ChileCopyright © 2022 Si, Nhat and Nguyen. This is an open-access article distributed under the terms of the Creative Commons Attribution License (CC BY). The use, distribution or reproduction in other forums is permitted, provided the original author(s) and the copyright owner(s) are credited and that the original publication in this journal is cited, in accordance with accepted academic practice. No use, distribution or reproduction is permitted which does not comply with these terms.
*Correspondence: Minh Tho Nguyen, bWluaHRoby5uZ3V5ZW5Admx1LmVkdS52bg==
†ORCID: Nguyen Thanh Si, orcid.org/0000-0002-6356-9662; Pham Vu Nhat, orcid.org/0000-0002-1485-6569; Minh Tho Nguyen, orcid.org/0000-0002-3803-0569
Disclaimer: All claims expressed in this article are solely those of the authors and do not necessarily represent those of their affiliated organizations, or those of the publisher, the editors and the reviewers. Any product that may be evaluated in this article or claim that may be made by its manufacturer is not guaranteed or endorsed by the publisher.
Research integrity at Frontiers
Learn more about the work of our research integrity team to safeguard the quality of each article we publish.