- 1Department of Stomatology, China-Japan Union Hospital of Jilin University, Changchun, China
- 2Tumor Hospital of Jilin Province, Changchun, China
- 3Key Laboratory of Polymer Ecomaterials, Changchun Institute of Applied Chemistry, Chinese Academy of Sciences, Changchun, China
In recent years, the environmental problems accompanying the extensive application of biomedical polymer materials produced from fossil fuels have attracted more and more attentions. As many biomedical polymer products are disposable, their life cycle is relatively short. Most of the used or overdue biomedical polymer products need to be burned after destruction, which increases the emission of carbon dioxide (CO2). Developing biomedical products based on CO2 fixation derived polymers with reproducible sources, and gradually replacing their unsustainable fossil-based counterparts, will promote the recycling of CO2 in this field and do good to control the greenhouse effect. Unfortunately, most of the existing polymer materials from renewable raw materials have some property shortages, which make them unable to meet the gradually improved quality and property requirements of biomedical products. In order to overcome these shortages, much time and effort has been dedicated to applying nanotechnology in this field. The present paper reviews recent advances in nanocomposites of CO2 fixation derived reproducible polymers for biomedical applications, and several promising strategies for further research directions in this field are highlighted.
Introduction
With the increases in total population and social productivity, the world’s energy consumption and associated greenhouse gas emissions have increased rapidly (Bauer and Menrad, 2019; Maamoun, 2019; Miyamoto and Takeuchi, 2019; Iglina et al., 2022). Since the industrial revolution in the 1700s, the amount of greenhouse gases emitted into the atmosphere has increased year by year, which has intensified greenhouse effect and caused a series of problems. As the main component of greenhouse gases, CO2 released by the combustion of fossil fuels (coal, oil, and natural gas) and products derived from them, plays a leading role in greenhouse effect. In order to avoid the disasters caused by the intensification of greenhouse effect, researchers with different backgrounds have made great efforts in curbing the CO2 emissions (Rahman et al., 2017; Pan et al., 2018; Chowdhury and Loganathan, 2019; Xu et al., 2019; Xu et al., 2021).
From the view of front-line medical workers, a lot of biomedical polymer products used in our daily work should share some responsibility for the world’s CO2 emissions. Due to their excellent characteristics and ability to reduce the costs, biomedical polymers have been widely used in the fields of tissue engineering, artificial organs, drug synthesis, diagnostic instruments, medical protective items, medical consumables, and packaging materials (Liechty et al., 2010; Mora-Huertas et al., 2010; Tian et al., 2012; Zhang L. et al., 2021; Feng et al., 2021; Hao et al., 2021; Chen W. H. et al., 2022). As shown in Figure 1, except for some large-scale medical equipment, long-life tissue engineering devices and artificial organs, most of the biomedical polymer products have a relatively short life cycle. Most of those used or overdue medical products need to be burned after destruction, which increases the emission of CO2. According to the statistics, there are nearly 100 kinds of biomedical polymer materials (Smith and Gambhir, 2017; Shaghaleh et al., 2018; Lyu et al., 2019), and more than 1800 related medical products in the world. From 2011 to 2019, the global production and marketing scale of biomedical polymer materials had increased from 4.391 to 6.771 million tons, with a compound annual growth rate of 5.6%. It means that annual CO2 emission by treatment of the biomedical polymer waste will be over 20 million tons. Considering the strict process conditions and high defect rate in the production of medical products, the contribution of greenhouse gas emissions in this field will be even greater. As it is urgent to control the greenhouse effect, the recycling of CO2 in biomedical polymer deserves more attention.
Since the outbreak of novel coronavirus disease 2019 (COVID-19), consumption of biomedical materials related to epidemic prevention has soared. As shown in Figure 2A, the production capacity of medical masks in China increased by over 100% after the COVID-19 epidemic. The data released by the Chinese general administration of customs shows that: a large number of medical supplies, including over 220 billion face masks, 2.31 billion protective suits, 289 million pairs of goggles, 2.92 billion pairs of surgical gloves, as well as 1.08 billion COVID-19 virus detection kits were exported abroad from China in 2020 (Figure 2B). Although these epidemic prevention products have played important roles in the global fight against the COVID-19, and have saved countless lives, the environmental problems caused by massive use of them, especially the increase in CO2 emissions deserve more attentions.
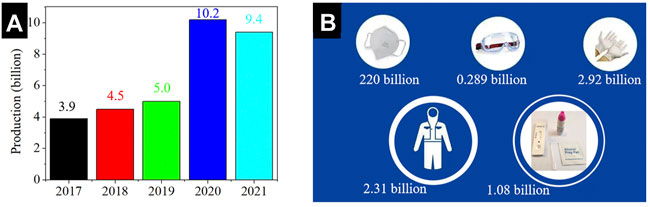
FIGURE 2. (A) Changes in China’s face mask production from 2017 to 2021. (B) Epidemic prevention supplies exported from China to other countries in 2020.
There are many different ways for classification of biomedical polymer materials (Luckachan and Pillai, 2011; Carrion et al., 2021; Wendels and Avérous, 2021; Chen W. H. et al., 2022; Joseph et al., 2022). According to their degradation behavior, they could be divided into biodegradable and nonbiodegradable biomedical polymer materials (Tian et al., 2012; Pang et al., 2014a; Pang et al., 2014b; Pang et al., 2014c; Sun et al., 2014; Sun et al., 2015b). Based on the ways in which they respond to heat, biomedical polymer materials could be classified as thermoplastics, thermosets and elastomers. Considering their origins, they could also be classified into bio-based and fossil fuel-based biomedical polymer materials (as shown in Table 1) (Endres and Siebert-Raths, 2011; Annabi et al., 2014; Sun et al., 2017; Hacker et al., 2019). In general, the bio-based polymer materials (also known as naturally-derived polymers) produced from reproducible plants, animals, and microorganisms, have less contribution to the greenhouse effect than their fossil fuel-based counterparts (Cui et al., 2011; Jayaramudu et al., 2013; Malwela and Ray, 2015; Jammalamadaka and Tappa, 2018; Sun Y. et al., 2019). As these naturally-derived biomedical polymer materials are fundamentally derived from photosynthesis of plants or other natural CO2 fixation reactions, their carbon footprints are much smaller than materials from unsustainable petrochemical raw materials.
As shown by Figure 3, there are three kinds of CO2 fixation derived reproducible polymers, including naturally-derived polymers, synthetic renewable polymers and CO2-based polymers. Synthetic renewable polymers are a group of polymeric materials produced by polymerization of monomers derived from raw materials of naturally-based molecules or macromolecules (Hu C. Y. et al., 2018; Duan et al., 2018; Duan et al., 2019; Yang et al., 2019; Zhou et al., 2019; Duan et al., 2021b; Huang et al., 2021). CO2-based polymers are produced by polymerization between CO2 gas and other bio-based or fossil fuel-based chemicals (Hu C. et al., 2018; Li et al., 2018; Duan et al., 2021a).
As the production of CO2 fixation derived reproducible polymers consumes CO2, their carbon footprints would be relatively small. Therefore, developing biomedical products based on these CO2 fixation derived polymers with reproducible sources, and gradually replacing their unsustainable petrochemical products-based counterparts, will promote the recycling of CO2 in this field and reduce the carbon footprints of them. However, compared with the fossil fuel-based biomedical polymers, most of the existing CO2 fixation derived polymers from renewable raw materials or CO2-based polymer materials, have some shortages in their application properties, which make them unable to meet the gradually improved quality and property requirements of biomedical products.
In the past few decades, nanotechnology has been proved to be effective in improving the properties of traditional materials (Bosco et al., 2015; Li et al., 2016; Sun et al., 2017; Zheng et al., 2017; Iravani, 2020; Allahyari et al., 2021; Fang et al., 2021; Sajjadi et al., 2021; Tamburaci and Tihminlioglu, 2021; Wang et al., 2021; Iravani, 2022). And many scientists have been engaged in developing new kinds of eco-friendly biomedical polymers by adding various nanomaterials into CO2 fixation derived polymers. As shown by Figure 4, natural and synthetic CO2 fixation derived polymers could be produced from CO2 fixation process. In order to improve their application performance, more and more nanocomposites of these CO2 fixation derived reproducible polymers have been prepared by nano-modification. These nanocomposites have wide prospects for production of eco-friendly biomedical polymer products. After being used or overdue, most of the biomedical products produced by nanocomposites of these CO2 fixation derived reproducible biomedical polymers would be burnt. The CO2 gas released by burning of them could be used to produce new reproducible polymers, so as to realize cyclic utilization of CO2. Focusing on the reduction of greenhouse gas emissions in biomedical polymer materials, this short review mainly presented recent advances in the research on nanocomposites of CO2 fixation derived biomedical polymers, including naturally-derived polymers (Section 2), synthetic renewable polymers (Section 3) and CO2-based polymers (Section 4). Moreover, potential trends, challenges and promising strategies for further research directions in this field are also highlighted.
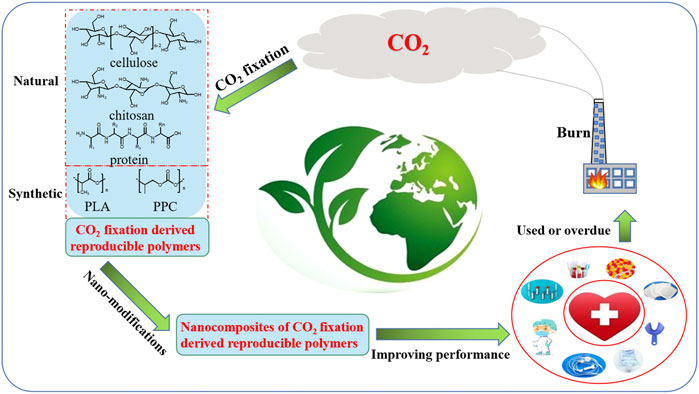
FIGURE 4. CO2 circulation in production and application of CO2 fixation derived reproducible biomedical polymers.
Naturally-derived polymers
Naturally-derived polymers generated from living organisms have a long history of being used as biomedical materials. As early as about 3500 BC, the ancient Egyptians began to use cotton fibers or horsehair to suture wounds. Mexican Indians of the same period could use wood chips to repair the injured skull. Since the 1950s, the rapid developed synthetic polymer materials have replaced natural polymer materials in a variety of application fields. However, based on their excellent biocompatibility and biodegradability, naturally-derived polymers still have irreplaceable applications in the field of biomedical materials. Up to now, research on applying naturally-derived polymers in biomedical products preparation is still attractive all over the world (Kokubo and Takadama, 2006; MacDonald et al., 2008; Henkel et al., 2013; Kang et al., 2014; Nguyen et al., 2017; Nurwito and Maulida, 2018; Barroso et al., 2020; Ramanathan et al., 2020; Weller et al., 2020; Neacsu et al., 2021a; Neacsu et al., 2021b; Carrion et al., 2021). As shown by Figure 5, naturally-derived polymer materials could be divided into two groups including protein-based biomaterials (e.g., collagen, gelatin, silk fibrin, etc.) and polysaccharide-based biomaterials (e.g., cellulose, chitin/chitosan, starch, alginate, hyaluronic acid derivatives, etc.). In this section, recent progress in research on nanocomposites of typical naturally-derived polymers and their applications in biomedical products will be presented.
Collagen
Collagen, the general name of a series of proteins with molecular weight (Mw) ∼300,000, is the most abundant protein in animal body and the major component of skin and skeletal muscle tissues (Chen et al., 2008; Arias et al., 2018; Le Corre-Bordes et al., 2018; Bi et al., 2019; Dippold et al., 2019; Ibrahim et al., 2020; García-Hernández et al., 2021; Hernández-Rangel and Martin-Martinez, 2021). To date, over 20 different types of collagen have been detected in human body, the most common being collagen types I-IV. As most of the collagen molecules have a chain length of sub-micrometer scale, they are a group of natural biomedical nanomaterials. Collagen undergoes enzymatic degradation in the body, resulting in the production of the corresponding amino acids. Due to its unique physicochemical, mechanical, and biological properties, as well as enzymatic degradation, biocompatibility, osteoconductivity, collagen has been extensively researched for biomedical applications (Szentivanyi et al., 2009; Di Maggio et al., 2011; Metwally et al., 2020). Additionally, it has been demonstrated that the rate of collagen deterioration could be controlled by enzymatic pretreatment or by adding cross-links to the polymer chains.
Since collagen and substituted hydroxyapatite are the major solid components of human bone, nanocomposites of collagen and calcium phosphate have been intensively studied as bionic materials for bone replacement and regeneration (Szentivanyi et al., 2009; Henkel et al., 2013). Collagen-hydroxyapatite nanocomposites have gained much recognition as bone grafts, not only due to their composition and structural similarity with natural bone, but also because of their unique functional properties and superior mechanical strength. Incorporating collagen into nanocomposites could provide more cell-recognition sites and result in fast replacement by new bone (Di Maggio et al., 2011; Metwally et al., 2020). Even without osteogenic supplement in culture medium, collagen-hydroxyapatite nanocomposites are very efficient in inducing rapid mineralization by cells.
In addition, collagen-hydroxyapatite based nanocomposites are currently on the market for a wide range of applications. Collagraft®, a composite made of fibrous collagen, hydroxyapatite and tricalcium phosphate, has been approved by FDA for using as biodegradable synthetic bone grafts. Floseal®, exploiting the high thrombogenic properties of collagen, is a high-viscosity gel hemostatic agent consisting of collagen particles and tropical bovine-derived thrombin. Duragen® suture-free sutures were developed for dural repair and regeneration. Collagen-based wound dressings, like Biobrane® and Promogran®, Sulmycin®-Implan gentamicin delivery vehicle, as well as three-dimensional collagen matrix grafts, all show the high research value of collagen in clinical medicine.
Fibrin
When thrombin transforms fibrinogen, fibrin, a natural polymer involved in the coagulation process would be created. Fibrin is primarily protein with molecular weight ∼360 kDa composed of pairs of polypeptide chains. Its structure consists of a central structural domain composed of fibrin peptide E, two pairs of fibrin peptide A&B molecules, and two terminal structural domains of fibrin peptide D. Based on its good biocompatibility, biodegradability, injectability, and properties to promote cell adhesion and proliferation, fibrin is one of the earliest biopolymers being used as biomedical materials. In recent years, studies on fibrin related to wound healing have been prompted by the fibrous network qualities that naturally generate blood coagulants (Zanetti et al., 2013; Whelan et al., 2014). Currently marketed product Bioseed® is a fibrin-based product for the treatment of chronic wounds made by mixing keratin-forming cells with fibrin. By using autologous plasma, Cryoseal® (Thermogenesis, United States) allows the preparation of hemostatic tissue sealants for the treatment of burn wound care. In addition to the coagulation effect, fibrin networks are used as a sealer on the scaffold surface to improve cell adhesion, proliferation, and encapsulation of multiple cell types in fibrin scaffolds or gels (Yücel et al., 2003). Mittermayr et al. added angiogenesis-stimulating substances to fibrin scaffolds to improve regeneration of ischemic tissue (Mittermayr et al., 2016). Although fibrin with weak mechanical characteristics is susceptible to hydrolysis by hydrolases, its mechanical qualities can be strengthened by combining it with other polymers to create complexes.
Gelatin
Gelatin is a kind of denatured collagen obtained by hydrolysis of collagen extracted from animal tissues. Therefore, the mechanical properties, swelling behavior and other physicochemical properties of gelatin are determined by the kind of collagen recovered and the technique of transformation. Similar to other naturally-derived polymers, gelatin exhibits excellent biocompatibility, biodegradability and limited immunogenicity. Gelatin has been widely used as a low-cost naturally-derived polymers in medical and pharmaceutical applications (Foox and Zilberman, 2015; Yue et al., 2015; Li et al., 2020; Rajabi et al., 2021). Moreover, many oral capsules and vaccine stabilizers are based on gelatin. Being used as drug delivery vehicles, gelatin could be designed into various carrier systems, including hydrogels, microspheres, nanoparticles, and nanofibers, making it suitable for drug delivery to different organs. Gelatin nanoparticles, for instance, are more suitable for drug delivery applications within the brain (Xavier Mendes et al., 2021). Nanocomposites of gelatin blended with other complexes are currently under numerous investigations for improving the potential applications in tissue engineering. For example, Elsayed et al. used the fabricated bionic electrospun gelatin fiber scaffold for the mid-membrane equivalent of tissue engineered vascular grafts, and the scaffold exhibited good smooth muscle cell proliferation capabilities (Elsayed et al., 2016).
Chitin/chitosan
Composed of renewable, biodegradable carbohydrates, chitin and chitosan are the second abundant biopolymers in nature (Niaounakis, 2014; Fernandes et al., 2022). Based on their excellent cell attachment and growth abilities, chitin and chitosan made up of N-acetyl glucosamine and N-aminoglucose monomers, have been widely applied in biomedicine (scaffolds, gels, particles, films, etc.) (Qin et al., 2018; Wu et al., 2018; Xu et al., 2018; Yu et al., 2018). The positive charges of chitosan enhanced its capacity to adsorb negatively charged cells. Being used as wound dressing material, chitosan exhibits outstanding hemostatic and antimicrobial properties. Cui et al. (2011) found that chitosan-collagen hydrogels containing staphylococcin not only could promote the burn wound healing, but also showed antimicrobial efficacy against methicillin-resistant Staphylococcus aureus . Based on the hemostatic function of chitosan, products such as HemCon® bandages (HemConMedical Technologies, Inc.) and Celox gauze (MedTrade) have been created and commercialized for the treatment of localized wound bleeding (Bennett, 2017). Chitosan has also been widely used in the field of tissue engineering scaffolds. Its regenerative capacity as a scaffold can be further enhanced by loading the structure with some bioactive compounds. It has been found that filamentous protein/chitosan-based scaffolds, used for repairing myofascial defects in the abdominal wall, showed sustained integration and similar mechanical strength with adjacent natural tissues (Gobin et al., 2006). Colloidal particles formed by chitosan could encapsulate a variety of biomedical molecules, which give them excellent potential in applications as drug delivery systems. Khangtragool’s experiments had successfully used chitosan as drug delivery vehicle for vancomycin application in the rabbit body eye. However, chitosan has mechanical weaknesses and needs to be used in combination with other materials for bone tissue engineering applications (Sharifianjazi et al., 2022). Isikli et al. demonstrated that: compared to pure chitosan scaffolds, nanocomposite scaffolds of hydroxyapatite/chitosan had improved Young’s modulus and compressive strength, while promoted cell attachment and value-added capacity of the scaffold (Isikli et al., 2012). Tamburaci et al. created composite scaffolds for the regeneration of bone tissue by adding diatomaceous earth into chitosan membranes (Iravani, 2020). This kind of diatomaceous earth-doped chitosan composite membranes were proved to have increased swelling behavior, protein adsorption capacity and surface crudity. Moreover, the composite membranes showed excellent biocompatibility by boosting the proliferation of Saos-2 cells and alkaline phosphatase activity.
Starch
Starch is one of the major carbohydrates commonly present in plant tubers and seed endosperm, such as potato, maize and wheat, etc., (Duan et al., 2011; Ismail et al., 2013). Taking advantage of the low price, easy availability, biocompatibility and biodegradability, starch nanocrystals have been widely used in biomedical field. In tissue engineering applications, adding starch to hydroxyapatite could not only enhance the material’s capacity for osteointegration, but also improve its thermal-mechanical properties and cell adhesion behaviors. Mahdieh et al. (2016) created a kind of novel nanocomposite by combining thermoplastic starch and poly (vinyl alcohol) (PVA) with nanoscale magnesium olivine and vitamin E, respectively. Compared with standard starch-PVA matrix, the novel nanocomposite showed better biological and mechanical properties, lower rate of degradation and increased secondary osteoblast proliferation. Moreover, chemically modified starch could be used for drug delivery. The nonionic nature of starch makes it easy to co-blend with other polymers, thereby increasing the pore size and water absorption capacity of the co-blended polymer (Ngoenkam et al., 2010). de Oliveira Cardoso et al. (2017) prepared hydrogels of gellan gum and starch retrograded blends with or without ketoprofen. By changing the concentrations of polymer and cross-linkers, properties of the starch hydrogels could be controlled. The hydrogels with adjustable mechanical and structural properties were promising materials for drug delivery of different therapeutic needs (Ghavimi et al., 2015). In order to overcome the limited shear and heat resistance of starch, SPCL has been prepared by blending starch with the biodegradable polycaprolactone (PCL). Addition of PCL could reduce the stiffness and high water-sensitivity of starch, and made improvement of SPCL in processability, degradability, mechanical properties and cell proliferation (Labet et al., 2007). Additionally, addition of starch could enhance the biodegradability of PCL, and significantly reduce the production cost of the final product (Morgan et al., 2013).
Cellulose
As the most abundant natural polymer on earth, cellulose is considered as the most important renewable biological resource, with great economic value in the world (Neo and Yang, 2015; Basu et al., 2018). Cellulose is a linear polymer composed of β-D-glucopyranose units (disaccharides) linked by glycosidic β-(1,4) bonds. Nanofibrillar networks formed by plant cellulose have a thickness of about 20–100 nm. Whereas bacterial cellulose nanofibrils are 20–50 nm thick. Bacterial cellulose usually shows lower crystallinity, and is better at storing water than plant cellulose (Lavoine et al., 2012; Olson et al., 2012). Additionally, the plant cellulose’s glycan polymers are resistant to biotransformation, which restricts its application fields (Loh et al., 2018; Mishra et al., 2018). Therefore, bacterial cellulose is the subject of extensive investigation currently. Since the 1980s, bacterial cellulose has been used as a natural polymer for wound dressing. Fu et al. (2013) applied a mixture of bacterial cellulose hydrogel and acrylic acid solution in a mouse allograft wound model, and the bacterial cellulose hydrogel exhibited good cell carrier properties and accomplished allograft wound healing in the model. Nanofibrillar products such as Biofill® (Fibrocel, Brazil) or Nanocell® (Thai Nano Cellulose Co. Ltd., Thailand) based on bacterial cellulose are widely used in clinical settings for various types of wounds, such as chronic ulcers, donor partial adjuvants, and wounds following skin cancer (Muangman et al., 2011; Islam et al., 2021). In addition, bacterial cellulose also could be used in medicine delivery systems. Silver nanoparticle-based bacterial cellulose (Ag-BC) has antibacterial effect against both Gram-positive and Gram-negative microorganisms (Yadav et al., 2015). In the domains of orthopedics, otology, ophthalmology, urology, and neurosurgery (Meyer and Palmer, 1934; Bodin et al., 2010; Wang et al., 2010; Kowalska-Ludwicka et al., 2013; Martínez Ávila et al., 2014). Bacterial cellulose is widely applied as a scaffold for guided tissue regeneration. In the realm of tissue engineering, it has an indispensable status.
Hyaluronic acid
Hyaluronic acid (HA) was first isolated from the vitreous fluid of the eye by Meyer and Palmer in 1934 (Monteiro et al., 2014). As an anionic linear polymer composed of D-glucuronic acid and N-acetyl-D-glucosamine, HA could be found in all living organisms. Similar to other natural polymers, HA’s biocompatibility and biodegradability make it one of the most widely used carbohydrate-based natural polymers in the field of tissue engineering. In the past two decades, HA has been involved in a wide range of biological activities, including the control of cell motility and adhesion, promotion of wound healing, and drug delivery in tissue engineering scaffolds. HA binded to CD44 and HA-mediated motor receptor (RHAMM) could be used as cell surface receptors. Internalization triggered by CD44-HA binding can prevent CD44 overexpression in cancer cells, making it possible to distribute anticancer drugs (Simman et al., 2018). Numerous biomedical materials based on HA derivatives have been commercialized, including esterified derivatives like ethyl/benzyl esters (HYAFF®) and cross-linked HA gels that have been extensively investigated for use as wound dressings. Mucoadhesive HA solutions (SYNVISC® and ORTHOVISC®) are also used in clinical settings as synovial fluid substitutes for the purpose of reducing pain and improving joint mobility in patients with osteoarthritis. The AMVISC® vitreous fluid replacement for ophthalmic applications is based on HA, which protects sensitive eye tissue during eye surgery. In the field of tissue regeneration, the HA-based scaffold Hyalomatrix® (Anika therapy) can be employed as a dermal substitute for severe surgical wounds, which can promote capillary growth and attract fibroblasts to grow inward after delivery to the wound bed (Faga et al., 2013; Dwivedi et al., 2020).
Alginate
Alginate, also known as sodium alginate, is an anionic polysaccharide found in the cell wall and intercellular space of algae. It is a block copolymer composed of two different ratios and arrangements of glycuronates ((1,4)-linked β-d-mannuronic acid and α-l-guluronic acid), which allows aqueous solutions of alginate to exhibit a non-Newtonian behavior similar to glycosaminoglycans. Due to its excellent properties including non-toxicity, biocompatibility, non-immunogenicity, straightforward gelation, controlled degradability and cheap in price, alginate has been used in a wide range of biomedical applications. Alginate gels could encapsulate molecules or cells because of their gentle characteristics (Lim and Sun, 1980; Gasperini et al., 2014). In the 1980s, alginate containing encapsulated pancreatic cells was first implanted into humans (O’Meara et al., 2015). Commercial products based on alginate derivatives, such as Kaltostat® and AlgiSite®, are frequently used in wound care, mainly for the treatment of venous leg ulcers, donor wound dressings, pressure ulcers, and so on (Lee and Mooney, 2012; Dumville et al., 2014; Brenner et al., 2015; Khoshzaban et al., 2018; Langa et al., 2018). Alginate hydrogels can also be used as scaffolds and matrices for the capture and delivery of bioactive molecules or cells, and are widely used for tissue regeneration. Alginate can also be used as a drug delivery system, and encapsulation of particular proteins and bioactive substances in alginate gels can enhance their targeting.
Synthetic renewable polymers
Synthetic renewable polymers are a group of polymeric materials produced by biologically or chemically polymerization process (Modjarrad and Ebnesajjad, 2013; Takeuchi et al., 2013; Sun et al., 2015a; Duan et al., 2015; Sun et al., 2016; Duan et al., 2017a; Duan et al., 2017b; Ricklefs et al., 2017; Yang et al., 2022). The production of monomers for this kind of polymer consumes naturally-based molecules or macromolecules (e.g., sugar, starch, cellulose, proteins, etc.) as raw materials. Originated from photosynthesis CO2 fixation process of plant or microorganisms, their carbon footprints are lower than traditional fossil fuel-based polymers. The premise of discussing the impact of a material on greenhouse effect is that its production and application should reach a certain scale. So far, the most intensively studied synthetic renewable polymers, which are expected to make significant contributions to decarbonization, namely polyhydroxyalkanoates (PHAs) and polylactic acid (PLA) related polymer materials.
Poly (hydroxyalkanoates)
In the past few decades, as a group of intracellular polyesters synthesized by different microorganisms, PHAs have been developed rapidly as a kind of new biosynthetic renewable polymers. PHAs are the largest group of biopolymers, and new types of them can be manufactured by biosynthesis with genetically modified bacteria. As shown by Figure 6, based on the feed stocks such as cane/sugar beet sucrose, corn starch-based glucose, plant oils (soybean oil, palm oil, corn oil, etc.) and animal lipids, PHAs with different monomeric building blocks could be produced by biosynthesis process catalyzed by enzymes in living cells. The easy availability, biocompatibility and biodegradability of PHAs make them suitable for biomedical applications, such as absorbable sutures, drug delivery systems, scaffolds and implants to support stem cell proliferation materials (Pourmollaabbassi et al., 2016). Lim et al. compared the properties of different PHA scaffolds in the field of bone tissue engineering, showing well bioactivity and the ability to induce bone regeneration (Lim and Sun, 1980). Bagdadi’s experiments proved the excellent performance of poly-3-hydroxyoctanoate in cardiac tissue engineering (Bagdadi et al., 2018). In addition, PHAs can be applied as wound dressings for skin defect repair. Shishatskaya et al. (2016) employed poly (3-hydroxybutyrate- co-4-hydroxybutyrate) solutions as experimental wound dressings and discovered that they promoted wound tissues healing and vascularization. It has also been found that PHA alone can stimulate cell cycle progression, reduce mitochondrial reactive oxygen species (ROS) production, decrease neuronal mortality, and inhibit neuronal membrane potential changes for the treatment of neurodegenerative illness and seizures control (Yum et al., 2012). Due to its high degradation rate, PHAs could be utilized as a substitute for disposable plastics such as medical appliances, medical packaging and medical trash-bags (Lee and Mooney, 2012; Lim et al., 2017). Although the market prices of these products are not high, the total consumption of them is huge, which are of great significance for decarbonation and environmental protection.
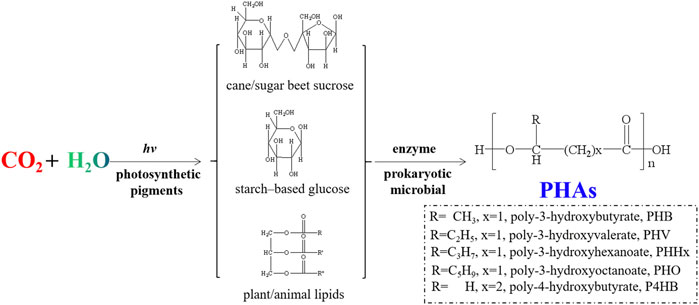
FIGURE 6. Schematic illustration for synthetic route of PHAs with different monomeric building blocks.
Polylactic acid
PLA is one of the most widely utilized synthetic renewable polymers in the biomedical field. As a hydrolyzable aliphatic semi-crystalline polyester, PLA can be prepared both by direct polymerization of lactic acid and by ring opening polymerization (ROP) of lactide (Figure 7) (Langa et al., 2018). The monomers of PLA could be generated by fermentation of carbohydrates (maltose, sucrose, lactose, etc.), which makes it renewable and sustainable. PLA with different stereoisomeric structures show distinct properties (Abd Razak et al., 2012; Khemani and Scholz, 2012; Pang et al., 2014b; Pang et al., 2014c; Sun et al., 2014; Sun et al., 2015b; Rokutanda et al., 2015; Hadasha and Bezuidenhout, 2017; Zhang et al., 2017). For example, poly (D-lactic acid) (PDLA) and poly (l-lactic acid) (PLLA) are semi-crystalline materials, and poly (D, l-lactic acid) (PDLLA) and meso-PLA are amorphous materials (Giménez et al., 2018).
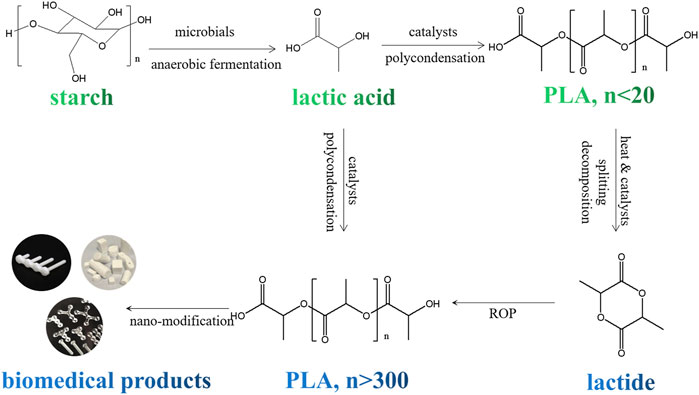
FIGURE 7. Schematic illstration for preparation process of PLA based biomedical products from renewable raw materials.
Despite its complicated molecular stereo structure, PLA shows many advantages in terms of chemical and mechanical properties, biocompatibility and biodegradability. A variety of PLA-based biomedical products have been developed, including surgical clips for surgical instruments, sutures, adhesives for wound closure, bone staples and plates, regenerative scaffolds and drug delivery systems, even medical devices and packaging, etc. Among them, PDLLA is widely used as biomedical coating for orthopedic materials because of its excellent mechanical stability and biocompatibility. Low molecular weight PDLLA can be bonded to antibiotics and other drugs for local drug delivery. Because of its nontoxicity, biocompatibility, and biodegradability, PLLA has been widely explored for cardiac tissues.
Gimenez’s experiments used PLLA scaffolds with overexpression of connexin43 (CX43) to line diaphragm myoblasts (DM) in a model of acute coronary artery occlusion in sheep. The results showed that PLLA plate inoculated with DM overexpressing CX43 could reduce the infarct size, decrease the fibrosis degree of infarct boundary, and induce cardiovascular regeneration, so as to improve cardiac function (Arya et al., 2018). PLLA has relatively high tensile strength, low elongation, and superior modulus (∼4.8 GPa). And it takes time from months to years to for PLLA derided products to lose mechanical integrity. These properties make PLA more suitable for load-bearing roles (e.g. orthopedic fixation). Numerous PLA-based orthopedic products are available on the market, such as Bio-Anchor® (Conmed), Maniscal Stinger ® (Linvatec) and Phantom Suture Anchor® (DePuy). The ability of PLLA to form high-strength fibers can also be used to develop absorbable row sutures and scaffold materials to replace non-degradable fibers. Meanwhile, PLLA has applications in tissue engineering, for example, Sculptra®, an injectable product of PLLA, helps HIV patients who are losing face fat.
PLA related polymers
In the field of biomedical applications, poly (lactic-co-glycolic acid) (PLGA), copolymer of PLA and poly (glycolic acid) (PGA), is considered to be the most representative PLA related polymer materials (Mulinti et al., 2018). The degradation and mechanical properties of PLGA can be adjusted by varying the ratio of lactic acid (or lactide) and glycolic acid (or glycolide). With excellent properties of biocompatibility, non-cytotoxicity and processability, PLGA has been widely used for biomedical and tissue engineering applications (Lanao et al., 2013). For example, PLGA can be utilized as various drug delivery vehicles (microspheres, nanospheres or nanofibers) for the controlled release of drugs, such as for the treatment of cancer and the release of hormone-based drugs. Zamani et al. (2015) developed a novel SDF-1α delivery system by using coaxial electrospraying. The SDF-1α incorporated PLGA particles they achieved had distinct core-shell structure, with the shell thickness of ∼300 nm. Their experiment results showed that the as-prepared core-shell particles could realize controlled release of the SDF-1α for 40 days Moreover, as one of the potential candidates for application in tissue engineering, a large number of researchers have applied PLGA in scaffold development studies. Liu et al. (2012) showed that nanocomposite material of silver nanoparticle/PLGA stainless steel alloy had strong antibacterial and osteoinductive properties, indicating its potential for bone regeneration applications. Lin et al. (2019) applied a tyramine-modified bilayer PLGA scaffold in a porcine model and found that chondrocytes in the scaffold with small and large pores in the upper and lower parts, respectively, had the ability to promote cartilage regeneration and articular cartilage repair. So far, there are many marketable products based on PLGA. For example, Vicryl®, the most popular clinically used absorbable suture, is prepared by PLGA copolymer with 90% glycolic acid and 10% l-lactic acid. Vicryl Rapid®, the irradiated version of Vicryl®, shows to be quick in degradation. By increasing the l-lactic acid/glycolic acid ratio in PLGA, ANACRYL® provides absorbable sutures with slow degradation rate. In all, depending on the application site, sutures with varying degradation rates can be selected to achieve the best healing effect. Tissue-engineered skin grafts (Dermagraft®) are the products marketed based on PLGA polymers for use as skin replacement materials.
Due to its biodegradability, the use of PLA related polymer materials in packaging materials and other disposable products has already received significant attentions (Sinclair, 1996; Haugaard et al., 2002; Frederiksen et al., 2003). Recently, more and more research works related to development of PLA-based nanocomposites for applications in biomedical devices, packages, and consumable products have been reported. Nonato et al. reported the preparation of PLA nanocomposites containing ZnO nanofibers by solvent-cast 3D printing method. This kind of PLA/ZnO nanocomposites were expected to be applicable in medical packaging applications (Nonato et al., 2019). Using hydroxyapatite with micro- and nano-particles as filler, Zaharescu et al. studied the effect of particle size on stability of PLA-based composites. It had been demonstrated that composites with nanosized filler showed better stability under gamma-irradiation. The results of this work would do good to developing long shelf-life PLA-based products for medical wears, face masks and other disposible devices (Zaharescu et al., 2020). Focusing on developing new materials for food and drug containers, packaging items, medical devices, Aversa et al. studied the wear resistance property of injection moulded PLA-talc composites detailedly. By constructing PLA super-networks of oriented and pyknotic crystals with the assistance of ductile poly (butylene adipate-co-terephthalate) (PBAT) nano-fibrils, Zhou et al. prepared PLA composite film with excellent gas barrier performance, high strength, and toughness, which showed promising prospect to be applied in the field of pharmaceutical packaging (Zhou et al., 2016). Antimicrobial and photodegradation properties of PLA/CaO nanocomposites were studied by Loyo et al. (2022). Results of their experiments showed that incorporating CaO nanoparticles into the PLA could be an applicable strategy for development of eco-friendly new medical packaging and devices. Zhao et al. (2019) studied effect of sterilization methods on commercially available biodegradable polyesters, focusing on their potential applications for single-used medical products. Their experiments demonstrates that, by choosing a suitable sterilization process, PLA could have potential to be used for production of transparent medical devices (the barrel of syringes or microfluidic chips), while PLA/PBAT blends might be applied in preparing non-transparent medical packaging.
CO2-based polymers
Among the various strategies for CO2 fixation, to produce CO2-based polymers offers significant potential (Hu C. et al., 2018; Li et al., 2018; Duan et al., 2021a). The CO2-based polymers usually are prepared by ring-opening copolymerization (ROCP) of CO2 and epoxides (Figure 8), under the assistance of certain catalysts (Li et al., 2018). Most of the CO2-based polymers produced by this way are aliphatic polycarbonates, which could be used for replacing the petroleum plastics in some applications such food and medical packaging, agricultural films, trash bags, etc. Moreover, low molecular weights CO2-based polymers could be used to synthesis poly (ether carbonate) polyols. Replacing petrochemical polyether polyols with these CO2-based polymers derived poly (ether carbonate) polyols in polyurethane manufacturing would lead to significant reduction in greenhouse gas emissions (∼20%). Currently, the annual requirement for polyols in field of for polyurethanes production is ∼3–4 Mt (Hemamalini and Dev, 2018; Jafari et al., 2018; Bauer and Menrad, 2019; Gibb, 2019; Shokraei et al., 2019; Heath et al., 2020). Therefore, the replacement of polyether polyols by poly (ether carbonate) polyols of CO2-based polymers could add value to waste CO2 emissions, and do good to decarbonization.
Poly (propylene carbonate)
PPC is an amorphous aliphatic polycarbonate produced by alternating copolymerization of carbon dioxide and propylene oxide (Fu et al., 2000; Liu et al., 2015). The low price of propylene oxide and CO2 has made PPC one of the most extensively researched and promising eco-friendly synthetic polymers. Since PPC is non-toxic, non-polluting, highly transparent, renewable and degradable, barrier, malleable and superior electrical conductivity, it can be used for preparation of various biomedical products (Dånmark et al., 2011; Weems et al., 2021). However, with side chains of -CH3 on its main molecular chain, PPC has drawbacks of poor dimensional stability and a low glass transition temperature (Tg), which restrict its usage in high-temperature resistance application fields. On the other hand, the degradation products of PPC in vivo are water and carbon dioxide, which can avoid the adverse effects similar to that produced by PLA and PLGA and have less inflammatory reactions. This facilitates the develop of PPC as a scaffold for tissue engineering applications (Liu et al., 2015). Manavitehrani et al. developed a gas foam porous scaffold designed based on PPC, starch and bio-glass. Both in vitro and in vivo experiments showed well biocompatibility and tissue infiltration. And in a joint implantation model, bone tissue integration was observed (Manavitehrani et al., 2019). In another experiment, PLA, tricalcium phosphate (TCP) nanoparticles and PPC were mixed to form a novel polymer scaffold named for PPTE for short. By inoculating mouse osteoblasts onto the PPTE composite, the researchers had found that the PPTE composite could promote attachment, proliferation, and mineralization of osteoblast. Meanwhile, PPTE composites also could control the production of bone bridge proteins, and promote the activity of phosphatase, making them viable materials for applications in bone regeneration (Fang et al., 2015). Compared to conventional degradable biopolymers, PPC exhibits better flexibility and maneuverability, making it an ideal carrier for drug delivery and a polymeric drug retardation material (Li et al., 2004; Manavitehrani et al., 2019). Using sirolimus-eluting PPC mesh in a rat jugular vein to abdominal aorta autograft model, it showed that PPC mesh could decrease arteriovenous graft stenosis and suppress MMP-2 and MMP-9 expression (Sun H. et al., 2019). In a recent study, single/double epoxy-capped PPC and chitosan were combined to form chitosan/PPC nanoparticles (CS/PPC NPs). The polymer was then tested for chemical and antibacterial properties. Experiment results revealed that the CS/PPC NPs had a high level of antibacterial activity against Escherichia coli and Staphylococcus aureus, providing a novel strategy for the manufacture of novel nano-size antibiotics (Quan et al., 2021).
Because of its good water barrier and low gas permeability, PPC shows strong and long-lasting antibacterial activity. These features make PPC suitable for preparing medical packaging, dressings and other devices (Quan et al., 2021). In addition, PPC can be used as bone fixation materials, surgical sutures, and as “additives” to improve the properties of other polymers (Yang et al., 2014; Jing et al., 2015; Manavitehrani et al., 2017; Chen X. et al., 2022). Yang et al. applied PPC to improve the performance of PLA. The elastic composite of β-TCP/PPC/PLA showed non-toxic to osteoblast-like cells. The tensile strength of the composite material was close to 1MPa, and the elongation at break (%) was ∼1100%. The modified PLA-derived composite showed advanced mechanical and biological properties (Yang et al., 2014). In the field of dentistry, sodium fluoride-PPC (NaF-PPC) composite strips obtained by adding filler of NaF into melting PPC, exhibited excellent remineralization and antibacterial properties, and might be used for preventing dental caries (Chen X. et al., 2022). As the most promising CO2-based polymers that can be used in numerous biomedical applications, PPC and its derive nanocomposites merits further exploration.
Poly (cyclohexene carbonate)
Based on ROCP of CO2 and cyclohexane oxide, poly (cyclohexene carbonate) (PCHC) is another kind of most common and well-studied CO2-based polymers. PCHC contains a six-member ring structure, which is more rigid and has better thermal performance than PPC (Welle et al., 2007; Liu et al., 2021). At room temperature, it is a brittle material and has high Tg (>100°C). It is a unique epoxide/CO2 copolymer that can be used at high temperature. The hydrophobic cyclohexane groups around the main chain of PCHC molecular make it more hydrophobic and less biodegradable. These factors make PCHC more difficult to be applied directly. However, composites of PCHC combined with other materials have been widely tested for application in drug-loaded nanoparticles, hydrogels, pore-forming substances, biodegradable surfactants, and regenerative scaffolds, etc (Liu et al., 2021). Alexander et al. prepared custom tissue stents of PCHC by electrostatic spinning technology (Welle et al., 2007). Zhang et al. used cationic PCHC as drug carriers to wrap therapeutic siRNAs. Serving as a siRNA carrier, PCHC showed good biodegradability, negligible cytotoxicity, and high transfection efficiency. It had been demonstrated that PCHC/siRNA nanocomposites had promising potential in pancreatic cancer treatment (Zhang X. et al., 2021).
Poly (ethylene carbonate)
As a well-studied fatty acid polycarbonate, poly (ethylene carbonate) (PEC) obtained by ROCP of ethylene oxide and CO2, shows unique surface erosion caused degradation both in vitro and in vivo. As a result, drug release behavior of PEC-based delivery systems usually is directly correlated with polymer mass loss, which would improve the predictability of the drug release profile these drug delivery systems (Bohr et al., 2016). On the other hand, PEC degradation could be triggered by some special enzymes and cells. This feature would do good to realize the drug release at particular locations in vivo. Priemel et al. (2018) had found that adding PEC to PLA microparticles could increase rifampicin release and enhance antibacterial activity. Macrophage induced surface degradation of PEC was investigated in vitro. Degradation of PEC with molecular weight of 41 kDa was slower than that of PEC with molecular weight of 200 kDa, which was proved to be attributed to macrophage induced surface degradation. And this work indicated that on-demand drug delivery induced by macrophages can be achieved with PEC-based drug delivery systems (Chu et al., 2014). As PEC has a short degradation period, typically completed within 2–4 weeks, it is difficult for PEC to be used in long-term drug delivery (Bohr et al., 2016). By embedding anti-oxidants into the PEC polymer matrix to scavenge the free radicals produced by phagocytes, Selvakumar et al. (2016) had slowed down the degradation rate of PEC. This work revealed the degradation mechanism of PEC, and provided a theoretical basis for developing long-term drug delivery systems with controlled release behaviors.
Poly (butyl carbonate)
Poly (butyl carbonate) (PBC), derived from monomers of epoxy butane and CO2, has good qualities of elasticity, tensile strength, elongation at break over 400%, Tg of −32°C. Despite of PBC’s good biocompatibility (Cai et al., 2017), its poor thermal stability and low melting temperature (∼55°C) have restrict its applications. Thus method of blending PBC with other polymers, has been frequently utilized to enhance the qualities of PBC-derived materials for a variety of applications (Wang et al., 2012; Wu et al., 2015; Li et al., 2019a). PBC has been transformed into biodegradable nanofiber membranes by numerous researchers for biomedical applications. For example, PBC/PLA/chitosan composite nanofiber membranes prepared by electrostatic spinning technique, had exhibited outstanding hydrophilicity, excellent antibacterial activity, and mechanical properties (Gu et al., 2019). In another work, axial electrostatic spinning was utilized to create core-shell PVA/PBC composite nano-fibers, which were then used as drug carriers of doxorubicin (DOX). The attachment and proliferate of SKOV3 ovarian cells could be inhibited by this DOX-loaded PVA/PBC core-shell nano-fibers, which indicated that as prepared nanocomposites have the potential to be used in tissue engineering and chemotherapy (Yan et al., 2016). Likewise, PBC can potentially be used as drug delivery vehicles for targeted therapies (Liu et al., 2007; Gu et al., 2018). Gu et al. (2018) prepared biodegradable nanofibers made of PBC, PLA, and graphene oxide (GO), and employed this PBC/PLA/GO composite nanofiber as a carrier for anti-tumor drugs. The findings indicated that the PBC/PLA/GO nanofiber matrix had the dual function of supporting cell imaging and drug delivery, which was promising for up-coming biomedical applications. PBC can also be added to other biodegradable polymers to enhance their mechanical and chemical characteristics. For instance, Wu et al. indicated that melt-blending PBC and PPC in an intermittent mixer might enhance PPC’s processing qualities and serve as a toughening agent (Wu et al., 2015). With good overall performance and low-cost, PBC is considered to be one of the most promising new biodegradable polycarbonates and deserves further development and utilization.
Other CO2-based polymers
In addition to the common polycarbonates mentioned above, there are numerous o CO2-based polymers that have applications in the biomedical field. Poly (trimethylene carbonate) (PTMC) has excellent biocompatibility, biodegradability and flexibility, and is very attractive for applications as nanocarriers of pharmaceuticals. It had been found to have greater kinetic stability and enhanced drug transport, exhibiting excellent chemotherapeutic efficacy against various cancers (Wang et al., 2013; Jiang et al., 2014; Li et al., 2019b; Zhou et al., 2020; Zou et al., 2020). In addition, PTMC can be used as hydrogels or 3D printed scaffolds for tissue engineering, such as vascular grafts and bone regeneration scaffolds (Song et al., 2011; Schüller-Ravoo et al., 2013). In a recent study, fabricated custom scaffolds for orbital floor repair by stereolithography using 40wt% hydroxyapatite in PTMC resin, which promoted vascular neovascularization and bone morphogenesis in the orbital floor (Guillaume et al., 2020). Poly (limonene carbonate) (PLC), generated from limonene oxide and carbon dioxide, is then widely used in coatings and is a promising bio-based alternative (Li et al., 2017; Li et al., 2021). Poly (propylene carbonate maleate) (PPCMA) is a new kind of CO2-based polymers produced by ternary polymerization of PO, CO2, and maleic anhydride. PPCMA’s primary chain contains a double bond that gives it versatility and practicality, enabling its widespread application in numerous research domains. Liu et al. (2011) encapsulated PPCMA by microencapsulation and controlled drug methanesulfonic acid. The utility of PPCMA for controlled release of drug pazufloxacin mesylate revealed the potential of PPCMA as a long-term drug sustained release carrier. Additionally, absorbable CO2-based polymers can be developed as tracers to direct imaging agents to certain areas in applications of medical imaging, anti-cancer immunity therapy, antimicrobial materials, etc (Ding et al., 2012; Voo et al., 2015; Zou et al., 2016; Zou et al., 2017; Lee et al., 2020; Liang et al., 2021).
Conclusion
In this review, focusing on the reduction of greenhouse gas emissions by biomedical polymer products, recent advances in studies on nanocomposites of CO2 fixation derived reproducible polymers have been reviewed. The CO2 fixation derived renewable polymers have been divided into three groups, namely naturally-derived polymers, synthetic renewable polymers and CO2-based polymers. As most of the CO2 fixation derived renewable polymers are biocompatible, non-cytotoxic, biodegradable and eco-friendly, there are more and more scientists engaged in researches on developing new kinds of biomedical materials by preparing nanocomposites of them. So far, great majority of research works in this field have been focused on high value-added biomedical applications, including tissue engineering, drug delivery, cancer treatment and organ transplantation, etc. However, the overall consumption of biomedical materials in these field is relatively small, which has a less important impact on reduction of CO2 emissions. In comparison, the researches on eco-friendly materials applied for disposable biomedical products, including diagnostic consumables, medical protective items (protective suits, face masks, goggles, surgical gloves, etc.), other medical consumables (sample tubes, drug packaging, medicine containers, syringe, infusion devices, etc.) and medical packaging materials, are obviously less than the former direction. Unfortunately, the raw materials of these low-cost disposable biomedical products, which have been ignored by scientists, are mainly petroleum-based polymers. Most of these consumable products have relatively short life cycle, and play a leading role in environmental pollution caused by biomedical polymer materials. Therefore, the authors suggest that more attentions and efforts should be given to develop new materials with small carbon footprint, based on CO2 fixation derived reproducible polymers for these short-life biomedical products in future.
Author contributions
XL and ZL: conceptualization, original draft preparations; ZJ, ZL, and ZS: literature survey, writing; XL: funding acquisition; DX, YY, and ZS: review and editing. All authors have read and agreed to the published version of the manuscript.
Funding
This research was supported by the Jilin Provincial Key Research and Development Project of Jilin Provincial Science and Technology Department, China (Grant No. 20200404176YY), and the Opening Foundation of Xi’an Modern Chemistry Research Institute (Grant No. 204J20211406).
Acknowledgments
The authors are grateful to finacial support of Jilin Provincial Department of science and technology and Xi’an Modern Chemistry Research Institute.
Conflict of interest
The authors declare that the research was conducted in the absence of any commercial or financial relationships that could be construed as a potential conflict of interest.
Publisher’s note
All claims expressed in this article are solely those of the authors and do not necessarily represent those of their affiliated organizations, or those of the publisher, the editors and the reviewers. Any product that may be evaluated in this article, or claim that may be made by its manufacturer, is not guaranteed or endorsed by the publisher.
References
Abd Razak, S. I., Ahmad Sharif, N., and Abdul Rahman, W. (2012). Biodegradable polymers and their bone applications: A review. Int. J. Basic Appl. Sci. 12, 31–49. doi:10.1.1.418.8617/123101-8585IJBAS-IJENS
Allahyari, S., Zahednezhad, F., Khatami, M., Hashemzadeh, N., Zakeri-Milani, P., and Trotta, F. (2021). Cyclodextrin nanosponges as potential anticancer drug delivery systems to be introduced into the market, compared with liposomes. J. Drug Deliv. Sci. Technol. 67, 102931. doi:10.1016/j.jddst.2021.102931
Annabi, N., Tamayol, A., Uquillas, J. A., Akbari, M., Bertassoni, L. E., Cha, C., et al. (2014). 25th anniversary article: Rational design and applications of hydrogels in regenerative medicine. Adv. Mat. 26 (1), 85–124. doi:10.1002/adma.201303233
Arias, L. S., Pessan, J. P., Vieira, A. P. M., Lima, T. M. T. d., Delbem, A. C. B., and Monteiro, D. R. (2018). Iron oxide nanoparticles for biomedical applications: A perspective on synthesis, drugs, antimicrobial activity, and toxicity. Antibiotics 7 (2), 46. doi:10.3390/antibiotics7020046
Arya, G., Kumari, R. M., Sharma, N., Gupta, N., Chandra, R., and Nimesh, S. (2018). “Polymeric nanocarriers for site-specific gene therapy,” in Drug targeting and stimuli sensitive drug delivery systems (Elsevier), 689–714. doi:10.1016/B978-0-12-813689-8.00018-5
Bagdadi, A. V., Safari, M., Dubey, P., Basnett, P., Sofokleous, P., Humphrey, E., et al. (2018). Poly (3‐hydroxyoctanoate), a promising new material for cardiac tissue engineering. J. Tissue Eng. Regen. Med. 12 (1), e495–e512. doi:10.1002/term.2318
Barroso, A., Mestre, H., Ascenso, A., Simões, S., and Reis, C. (2020). Nanomaterials in wound healing: From material sciences to wound healing applications. Nano Sel. 1 (5), 443–460. doi:10.1002/nano.202000055
Basu, A., Heitz, K., Strømme, M., Welch, K., and Ferraz, N. (2018). Ion-crosslinked wood-derived nanocellulose hydrogels with tunable antibacterial properties: Candidate materials for advanced wound care applications. Carbohydr. Polym. 181, 345–350. doi:10.1016/j.carbpol.2017.10.085
Bauer, A., and Menrad, K. (2019). Standing up for the Paris Agreement: Do global climate targets influence individuals’ greenhouse gas emissions? Environ. Sci. Policy 99, 72–79. doi:10.1016/j.envsci.2019.05.015
Bennett, B. L. (2017). Bleeding control using hemostatic dressings: Lessons learned. Wilderness Environ. Med. 28 (2), S39–S49. doi:10.1016/j.wem.2016.12.005
Bi, C., Li, X., Xin, Q., Han, W., Shi, C., Guo, R., et al. (2019). Effect of extraction methods on the preparation of electrospun/electrosprayed microstructures of tilapia skin collagen. J. Biosci. Bioeng. 128 (2), 234–240. doi:10.1016/j.jbiosc.2019.02.004
Bodin, A., Bharadwaj, S., Wu, S., Gatenholm, P., Atala, A., and Zhang, Y. (2010). Tissue-engineered conduit using urine-derived stem cells seeded bacterial cellulose polymer in urinary reconstruction and diversion. Biomaterials 31 (34), 8889–8901. doi:10.1016/j.biomaterials.2010.07.108
Bohr, A., Water, J. J., Wang, Y., Arnfast, L., and Beck-Broichsitter, M. (2016). Potential of surface-eroding poly (ethylene carbonate) for drug delivery to macrophages. Int. J. Pharm. X. 511 (2), 814–820. doi:10.1016/j.ijpharm.2016.07.075
Bosco, R., Iafisco, M., Tampieri, A., Jansen, J. A., Leeuwenburgh, S. C., and Van Den Beucken, J. J. (2015). Hydroxyapatite nanocrystals functionalized with alendronate as bioactive components for bone implant coatings to decrease osteoclastic activity. Appl. Surf. Sci. 328, 516–524. doi:10.1016/j.apsusc.2014.12.072
Brenner, M., Hilliard, C., Peel, G., Crispino, G., Geraghty, R., and O'Callaghan, G. (2015). Management of pediatric skin-graft donor sites: A randomized controlled trial of three wound care products. J. Burn Care Res. 36 (1), 159–166. doi:10.1097/BCR.0000000000000161
Cai, X., Yang, X., Zhang, H., and Wang, G. (2017). Modification of biodegradable poly(butylene carbonate) with 1, 4-cyclohexanedimethylene to enhance the thermal and mechanical properties. Polym. Degrad. Stab. 143, 35–41. doi:10.1016/j.polymdegradstab.2017.06.018
Carrion, C. C., Nasrollahzadeh, M., Sajjadi, M., Jaleh, B., Soufi, G. J., and Iravani, S. (2021). Lignin, lipid, protein, hyaluronic acid, starch, cellulose, gum, pectin, alginate and chitosan-based nanomaterials for cancer nanotherapy: Challenges and opportunities. Int. J. Biol. Macromol. 178, 193–228. doi:10.1016/j.ijbiomac.2021.02.123
Chen, Z., Mo, X., He, C., and Wang, H. (2008). Intermolecular interactions in electrospun collagen–chitosan complex nanofibers. Carbohydr. Polym. 72 (3), 410–418. doi:10.1016/j.carbpol.2007.09.018
Chen, W. H., Chen, Q. W., Chen, Q., Cui, C., Duan, S., Kang, Y., et al. (2022a). Biomedical polymers: Synthesis, properties, and applications. Sci. China Chem. 65, 1010–1075. doi:10.1007/s11426-022-1243-5
Chen, X., Zhao, S., Chu, S., Liu, S., Yu, M., Li, J., et al. (2022b). A novel sustained release fluoride strip based Poly (propylene carbonate) for preventing caries. Eur. J. Pharm. Sci. 171, 106128. doi:10.1016/j.ejps.2022.106128
Chowdhury, H., and Loganathan, B. (2019). Third-generation biofuels from microalgae: A review. Curr. Opin. Green Sustain. Chem. 20, 39–44. doi:10.1016/j.cogsc.2019.09.003
Chu, D., Beck-Broichsitter, M., Curdy, C., Riebesehl, B., and Kissel, T. (2014). Feasibility of macrophage mediated on-demand drug release from surface eroding poly (ethylene carbonate). Int. J. Pharm. X. 465 (1-2), 1–4. doi:10.1016/j.ijpharm.2014.02.005
Cui, F., Li, G., Huang, J., Zhang, J., Lu, M., Lu, W., et al. (2011). Development of chitosan-collagen hydrogel incorporated with lysostaphin (CCHL) burn dressing with anti-methicillin-resistant Staphylococcus aureus and promotion wound healing properties. Drug Deliv. (Lond). 18 (3), 173–180. doi:10.3109/10717544.2010.509363
Dånmark, S., Finne-Wistrand, A., Schander, K., Hakkarainen, M., Arvidson, K., Mustafa, K., et al. (2011). In vitro and in vivo degradation profile of aliphatic polyesters subjected to electron beam sterilization. Acta Biomater. 7 (5), 2035–2046. doi:10.1016/j.actbio.2011.02.011
de Oliveira Cardoso, V. M., Cury, B. S. F., Evangelista, R. C., and Gremião, M. P. D. (2017). Development and characterization of cross-linked gellan gum and retrograded starch blend hydrogels for drug delivery applications. J. Mech. Behav. Biomed. Mat. 65, 317–333. doi:10.1016/j.jmbbm.2016.08.005
Di Maggio, N., Piccinini, E., Jaworski, M., Trumpp, A., Wendt, D. J., and Martin, I. (2011). Toward modeling the bone marrow niche using scaffold-based 3D culture systems. Biomaterials 32 (2), 321–329. doi:10.1016/j.biomaterials.2010.09.041
Ding, X., Yang, C., Lim, T. P., Hsu, L. Y., Engler, A. C., Hedrick, J. L., et al. (2012). Antibacterial and antifouling catheter coatings using surface grafted PEG-b-cationic polycarbonate diblock copolymers. Biomaterials 33 (28), 6593–6603. doi:10.1016/j.biomaterials.2012.06.001
Dippold, D., Cai, A., Hardt, M., Boccaccini, A. R., Horch, R. E., Beier, J. P., et al. (2019). Investigation of the batch-to-batch inconsistencies of collagen in PCL-collagen nanofibers. Mater. Sci. Eng. C 95, 217–225. doi:10.1016/j.msec.2018.10.057
Duan, B., Sun, P., Wang, X., and Yang, C. (2011). Preparation and properties of starch nanocrystals/carboxymethyl chitosan nanocomposite films. Starch/Starke. 63 (9), 528–535. doi:10.1002/star.201000136
Duan, R., Sun, Z., Pang, X., Hu, C., Shao, H., Chen, X., et al. (2015). Non-symmetrical aluminium salen complexes: Synthesis and their reactivity with cyclic ester. Polymer 77, 122–128. doi:10.1016/j.polymer.2015.09.036
Duan, R., Hu, C., Li, X., Pang, X., Sun, Z., Chen, X., et al. (2017a). Air-stable salen–iron complexes: Stereoselective catalysts for lactide and ε-caprolactone polymerization through in situ initiation. Macromolecules 50 (23), 9188–9195. doi:10.1021/acs.macromol.7b01766
Duan, R., Qu, Z., Pang, X., Zhang, Y., Sun, Z., Zhang, H., et al. (2017b). Ring‐opening polymerization of lactide catalyzed by bimetallic salen‐type titanium complexes. Chin. J. Chem. 35 (5), 640–644. doi:10.1002/cjoc.201600580
Duan, R., Hu, C., Sun, Z., Pang, X., and Chen, X. (2018). Zinc and magnesium complexes bearing oxazoline-derived ligands and their application for ring opening polymerization of cyclic esters. ACS omega 3 (9), 11703–11709. doi:10.1021/acsomega.8b01997
Duan, R., Hu, C., Sun, Z., Zhang, H., Pang, X., and Chen, X. (2019). Conjugated tri-nuclear salen-Co complexes for the copolymerization of epoxides/CO2: Cocatalyst-free catalysis. Green Chem. 21 (17), 4723–4731. doi:10.1039/C9GC02045D
Duan, R., Hu, C., Zhou, Y., Huang, Y., Sun, Z., Zhang, H., et al. (2021a). Propylene oxide cycloaddition with carbon dioxide and homopolymerization: Application of commercial beta zeolites. Ind. Eng. Chem. Res. 60 (3), 1210–1218. doi:10.1021/acs.iecr.1c00080
Duan, R., Zhou, Y., Huang, Y., Sun, Z., Zhang, H., Pang, X., et al. (2021b). A trinuclear salen–Al complex for copolymerization of epoxides and anhydride: Mechanistic insight into a cocatalyst-free system. Chem. Commun. 57 (1), 133–136. doi:10.1039/D0CC06874H
Dumville, J. C., Keogh, S. J., Stubbs, N., Walker, R. M., and Fortnam, M. (2014). Alginate dressings for treating pressure ulcers. Cochrane Database Syst. Rev. 8, CD011277. Article number: CD011277. doi:10.1002/14651858.CD011277.pub2
Dwivedi, R., Pandey, R., Kumar, S., and Mehrotra, D. (2020). Poly hydroxyalkanoates (PHA): Role in bone scaffolds. J. Oral Biol. Craniofac. Res. 10 (1), 389–392. doi:10.1016/j.jobcr.2019.10.004
Elsayed, Y., Lekakou, C., Labeed, F., and Tomlins, P. (2016). Fabrication and characterisation of biomimetic, electrospun gelatin fibre scaffolds for tunica media-equivalent, tissue engineered vascular grafts. Mater. Sci. Eng. C 61, 473–483. doi:10.1016/j.msec.2015.12.081
Endres, H. J., and Siebert-Raths, A. (2011). Engineering biopolymers markets, manufacturing, properties and applications Munich: Hanser publisher 71148, 3–15.
Faga, A., Nicoletti, G., Brenta, F., Scevola, S., Abatangelo, G., and Brun, P. (2013). Hyaluronic acid three‐dimensional scaffold for surgical revision of retracting scars: A human experimental study. Int. Wound J. 10 (3), 329–335. doi:10.1111/j.1742-481X.2012.00981.x
Fang, H. W., Kao, W. Y., Lin, P. I., Chang, G. W., Hung, Y. J., and Chen, R. M. (2015). Effects of polypropylene carbonate/poly (d, l-lactic) acid/tricalcium phosphate elastic composites on improving osteoblast maturation. Ann. Biomed. Eng. 43 (8), 1999–2009. doi:10.1007/s10439-014-1236-9
Fang, H., Guo, Z., Chen, J., Lin, L., Hu, Y., Li, Y., et al. (2021). Combination of epigenetic regulation with gene therapy-mediated immune checkpoint blockade induces anti-tumour effects and immune response in vivo. Nat. Commun. 12 (1), 6742. doi:10.1038/s41467-021-27078-x
Feng, X., Xu, W., Xu, X., Li, G., Ding, J., and Chen, X. (2021). Cystine proportion regulates fate of polypeptide nanogel as nanocarrier for chemotherapeutics. Sci. China Chem. 64 (2), 293–301. doi:10.1007/s11426-020-9884-6
Fernandes, M., Padrão, J., Ribeiro, A. I., Fernandes, R. D., Melro, L., Nicolau, T., et al. (2022). Polysaccharides and metal nanoparticles for functional textiles: A review. Nanomaterials 12 (6), 1006. doi:10.3390/nano12061006
Foox, M., and Zilberman, M. (2015). Drug delivery from gelatin-based systems. Expert Opin. Drug Deliv. 12 (9), 1547–1563. doi:10.1517/17425247.2015.1037272
Frederiksen, C. S., Haugaard, V. K., Poll, L., and Miquel Becker, E. (2003). Light-induced quality changes in plain yoghurt packed in polylactate and polystyrene. Eur. Food Res. Technol. 217 (1), 61–69. doi:10.1007/s00217-003-0722-3
Fu, K., Pack, D. W., Klibanov, A. M., and Langer, R. (2000). Visual evidence of acidic environment within degrading poly (lactic-co-glycolic acid)(PLGA) microspheres. Pharm. Res. 17 (1), 100–106. doi:10.1023/a:1007582911958
Fu, L., Zhang, J., and Yang, G. (2013). Present status and applications of bacterial cellulose-based materials for skin tissue repair. Carbohydr. Polym. 92 (2), 1432–1442. doi:10.1016/j.carbpol.2012.10.071
García‐Hernández, A. B., Morales‐Sánchez, E., Calderón‐Domínguez, G., Salgado‐Cruz, M. d. l. P., Farrera‐Rebollo, R. R., Vega‐Cuellar, M. Á., et al. (2021). Hydrolyzed collagen on PVA‐based electrospun membranes: Synthesis and characterization. J. Appl. Polym. Sci. 138 (41), 51197. doi:10.1002/app.51197
Gasperini, L., Mano, J. F., and Reis, R. L. (2014). Natural polymers for the microencapsulation of cells. J. R. Soc. Interface 11 (100), 20140817. doi:10.1098/rsif.2014.0817
Ghavimi, S. A. A., Ebrahimzadeh, M. H., Solati-Hashjin, M., and Abu Osman, N. A. (2015). Polycaprolactone/starch composite: Fabrication, structure, properties, and applications. J. Biomed. Mat. Res. A 103 (7), 2482–2498. doi:10.1002/jbm.a.35371
Giménez, C. S., Olea, F. D., Locatelli, P., Dewey, R. A., Abraham, G. A., Montini Ballarin, F., et al. (2018). Effect of poly (l-lactic acid) scaffolds seeded with aligned diaphragmatic myoblasts overexpressing connexin-43 on infarct size and ventricular function in sheep with acute coronary occlusion. Artif. Cells Nanomed. Biotechnol. 46, S717–S724. doi:10.1080/21691401.2018.1508029
Gobin, A. S., Butler, C. E., and Mathur, A. B. (2006). Repair and regeneration of the abdominal wall musculofascial defect using silk fibroin-chitosan blend. Tissue Eng. 12 (12), 3383–3394. doi:10.1089/ten.2006.12.3383
Gu, X., Cao, R., Li, F., Li, Y., Jia, H., and Yu, H. (2018). Graphene oxide as a nanocarrier for controlled loading and targeted delivery of Typhonium giganteum drugs. J. Chem. 2018, 1–7. doi:10.1155/2018/6325870
Gu, X., Cao, R., Li, Y., Liu, S., Wang, Z., Feng, S., et al. (2019). Three-component antibacterial membrane of poly (butylene carbonate), poly (lactic acid) and chitosan prepared by electrospinning. Mat. Technol. (N. Y. N. Y). 34 (8), 463–470. doi:10.1080/10667857.2019.1576822
Guillaume, O., Geven, M. A., Varjas, V., Varga, P., Gehweiler, D., Stadelmann, V. A., et al. (2020). Orbital floor repair using patient specific osteoinductive implant made by stereolithography. Biomaterials 233, 119721. doi:10.1016/j.biomaterials.2019.119721
Hacker, M. C., Krieghoff, J., and Mikos, A. G. (2019). “Synthetic polymers,” in Principles of regenerative medicine (Elsevier), 559–590. doi:10.1016/B978-0-12-809880-6.00033-3
Hadasha, W., and Bezuidenhout, D. (2017). “Synthetic polymers,” in Principles of regenerative medicine (Elsevier), 51–77. Industrial Applications of Poly (lactic acid).
Hao, K., Guo, Z., Lin, L., Sun, P., Li, Y., Tian, H., et al. (2021). Covalent organic framework nanoparticles for anti-tumor gene therapy. Sci. China Chem. 64 (07), 1235–1241. doi:10.1007/s11426-021-9998-x
Haugaard, V., Weber, C., Danielsen, B., and Bertelsen, G. (2002). Quality changes in orange juice packed in materials based on polylactate. Eur. Food Res. Technol. 214, 423–428. doi:10.1007/s00217-001-0474-x
Heath, D. E., Guelcher, S. A., and Cooper, S. L. (2020). “Polyurethanes,” in Biomaterials science (Elsevier), 103–107. doi:10.1016/B978-0-12-816137-1.00010-6
Hemamalini, T., and Dev, V. R. G. (2018). Comprehensive review on electrospinning of starch polymer for biomedical applications. Int. J. Biol. Macromol. 106, 712–718. doi:10.1016/j.ijbiomac.2017.08.079
Henkel, J., Woodruff, M. A., Epari, D. R., Steck, R., Glatt, V., Dickinson, I. C., et al. (2013). Bone regeneration based on tissue engineering conceptions—A 21st century perspective. Bone Res. 1 (1), 216–248. doi:10.4248/BR201303002
Hernández‐Rangel, A., and Martin‐Martinez, E. S. (2021). Collagen based electrospun materials for skin wounds treatment. J. Biomed. Mat. Res. A 109 (9), 1751–1764. doi:10.1002/jbm.a.37154
Hu, C. Y., Duan, R. L., Yang, J. W., Dong, S. J., Sun, Z. Q., Pang, X., et al. (2018a). Enolic schiff base zinc amide complexes: Highly active catalysts for ring-opening polymerization of lactide and ε-caprolactone. Chin. J. Polym. Sci. 36 (10), 1123–1128. doi:10.1007/s10118-018-2129-4
Hu, C., Duan, R., Yang, S., Pang, X., and Chen, X. (2018b). CO2 controlled catalysis: Switchable homopolymerization and copolymerization. Macromolecules 51 (12), 4699–4704. doi:10.1021/acs.macromol.8b00696
Huang, Y., Hu, C., Zhou, Y., Duan, R., Sun, Z., Wan, P., et al. (2021). Monomer controlled switchable copolymerization: A feasible route for the functionalization of poly (lactide). Angew. Chem. Int. Ed. 60 (17), 9274–9278. doi:10.1002/anie.202017088
Ibrahim, A., Hassan, D., Kelany, N., Kotb, S., and Soliman, M. (2020). Validation of three different sterilization methods of tilapia skin dressing: Impact on microbiological enumeration and collagen content. Front. Vet. Sci. 7, 597751. doi:10.3389/fvets.2020.597751
Iglina, T., Iglin, P., and Pashchenko, D. (2022). Industrial CO2 capture by algae: A review and recent advances. Sustainability 14 (7), 3801. doi:10.3390/su14073801
Iravani, S. (2020). Nano- and biosensors for the detection of SARS-CoV-2: Challenges and opportunities. Mat. Adv. 1 (9), 3092–3103. doi:10.1039/D0MA00702A
Iravani, S. (2022). Nanophotocatalysts against viruses and antibiotic-resistant bacteria: Recent advances. Crit. Rev. Microbiol. 48 (1), 67–82. doi:10.1080/1040841X.2021.1944053
Isikli, C., Hasirci, V., and Hasirci, N. (2012). Development of porous chitosan–gelatin/hydroxyapatite composite scaffolds for hard tissue‐engineering applications. J. Tissue Eng. Regen. Med. 6 (2), 135–143. doi:10.1002/term.406
Islam, S. U., Ul-Islam, M., Ahsan, H., Ahmed, M. B., Shehzad, A., Fatima, A., et al. (2021). Potential applications of bacterial cellulose and its composites for cancer treatment. Int. J. Biol. Macromol. 168, 301–309. doi:10.1016/j.ijbiomac.2020.12.042
Ismail, H., Irani, M., and Ahmad, Z. (2013). Starch-based hydrogels: Present status and applications. Int. J. Polym. Mat. 62 (7), 411–420. doi:10.1080/00914037.2012.719141
Jafari, A., Hassanajili, S., Karimi, M. B., Emami, A., Ghaffari, F., and Azarpira, N. (2018). Effect of organic/inorganic nanoparticles on performance of polyurethane nanocomposites for potential wound dressing applications. J. Mech. Behav. Biomed. Mat. 88, 395–405. doi:10.1016/j.jmbbm.2018.09.001
Jammalamadaka, U., and Tappa, K. (2018). Recent advances in biomaterials for 3D printing and tissue engineering. J. Funct. Biomater. 9 (1), 22. doi:10.3390/jfb9010022
Jayaramudu, J., Reddy, G. S. M., Varaprasad, K., Sadiku, E., Ray, S. S., and Rajulu, A. V. (2013). Preparation and properties of biodegradable films from Sterculia urens short fiber/cellulose green composites. Carbohydr. Polym. 93 (2), 622–627. doi:10.1016/j.carbpol.2013.01.032
Jiang, T., Li, Y., Lv, Y., Cheng, Y., He, F., and Zhuo, R. (2014). Biodegradable amphiphilic block-graft copolymers based on methoxy poly (ethylene glycol)-b-(polycarbonates-g-polycarbonates) for controlled release of doxorubicin. J. Mat. Sci. Mat. Med. 25 (1), 131–139. doi:10.1007/s10856-013-5057-4
Jing, X., Mi, H., Peng, J., Peng, X., and Turng, L. (2015). Electrospun aligned poly(propylene carbonate) microfibers with chitosan nanofibers as tissue engineering scaffolds. Carbohydr. Polym. 117, 941–949. doi:10.1016/j.carbpol.2014.10.025
Joseph, X., Akhil, V., Arathi, A., and Mohanan, P. (2022). Nanobiomaterials in support of drug delivery related issues. Mater. Sci. Eng. B 279, 115680. doi:10.1016/j.mseb.2022.115680
Kang, M. L., Ko, J. Y., Kim, J. E., and Im, G. I. (2014). Intra-articular delivery of kartogenin-conjugated chitosan nano/microparticles for cartilage regeneration. Biomaterials 35 (37), 9984–9994. doi:10.1016/j.biomaterials.2014.08.042
Khemani, K., and Scholz, C. (2012). “Introduction and overview of degradable and renewable polymers and materials,” in Degradable polymers and materials: principles and practice. 2nd Edition (Washington DC: ACS Publications), 3–10. doi:10.1021/bk-2012-1114.ch001
Khoshzaban, A., Keyhanvar, P., Delrish, E., Najafi, F., Keshel, S. H., Watanabe, I., et al. (2018). Alginate microcapsules as nutrient suppliers: An in vitro study. Cell J. 20 (1), 25–30. doi:10.22074/cellj.2018.4508
Kokubo, T., and Takadama, H. (2006). How useful is SBF in predicting in vivo bone bioactivity? Biomaterials 27 (15), 2907–2915. doi:10.1016/j.biomaterials.2006.01.017
Kowalska-Ludwicka, K., Cala, J., Grobelski, B., Sygut, D., Jesionek-Kupnicka, D., Kolodziejczyk, M., et al. (2013). Modified bacterial cellulose tubes for regeneration of damaged peripheral nerves. Arch. Med. Sci. 9 (3), 527–534. doi:10.5114/aoms.2013.33433
Labet, M., Thielemans, W., and Dufresne, A. (2007). Polymer grafting onto starch nanocrystals. Biomacromolecules 8 (9), 2916–2927. doi:10.1021/bm700468f
Lanao, R. P. F., Jonker, A. M., Wolke, J. G. C., Jansen, J. A., van Hest, J. C., and Leeuwenburgh, S. C. (2013). Physicochemical properties and applications of Poly(lactic-co-glycolic acid) for use in bone regeneration. Tissue Eng. Part B Rev. 19, 380–390. doi:10.1089/ten.TEB.2012.0443
Langa, P., Wardowska, A., Zieliński, J., Podolak-Popinigis, J., Sass, P., Sosnowski, P., et al. (2018). Transcriptional profile of in vitro expanded human epidermal progenitor cells for the treatment of non-healing wounds. J. Dermatol. Sci. 89, 272–281. doi:10.1016/j.jdermsci.2017.12.003
Lavoine, N., Desloges, I., Dufresne, A., and Bras, J. (2012). Microfibrillated cellulose–its barrier properties and applications in cellulosic materials: A review. Carbohydr. Polym. 90 (2), 735–764. doi:10.1016/j.carbpol.2012.05.026
Le Corre-Bordes, D., Hofman, K., and Hall, B. (2018). Guide to electrospinning denatured whole chain collagen from hoki fish using benign solvents. Int. J. Biol. Macromol. 112, 1289–1299. doi:10.1016/j.ijbiomac.2018.02.088
Lee, A. L., Yang, C., Gao, S., Wang, Y., Hedrick, J. L., and Yang, Y. Y. (2020). Biodegradable cationic polycarbonates as vaccine adjuvants. ACS Appl. Mat. Interfaces 12 (47), 52285–52297. doi:10.1021/acsami.0c09649
Lee, K. Y., and Mooney, D. J. (2012). Alginate: Properties and biomedical applications. Prog. Polym. Sci. 37 (1), 106–126. doi:10.1016/j.progpolymsci.2011.06.003
Li, X., Meng, Y., Chen, G., and Li, R. (2004). Thermal properties and rheological behavior of biodegradable aliphatic polycarbonate derived from carbon dioxide and propylene oxide. J. Appl. Polym. Sci. 94 (2), 711–716. doi:10.1002/app.20938
Li, Z., Xing, D., Wang, C., Xin, Y., Liu, J., and Sun, Z. (2016). Recent progress in application of PEGylated nanocarriers for antitumor drug delivery systems. Drug Future 41 (3), 177–184. doi:10.1358/dof.2016.041.03.2464359
Li, C., Johansson, M., Sablong, R. J., and Koning, C. E. (2017). High performance thiol-ene thermosets based on fully bio-based poly (limonene carbonate) s. Eur. Polym. J. 96, 337–349. doi:10.1016/j.eurpolymj.2017.09.034
Li, X., Hu, C., Pang, X., Duan, R., and Chen, X. (2018). One-pot copolymerization of epoxides/carbon dioxide and lactide using a ternary catalyst system. Catal. Sci. Technol. 8 (24), 6452–6457. doi:10.1039/C8CY01856A
Li, Y., Han, C., Yu, Y., and Huang, D. (2019a). Morphological, thermal, rheological and mechanical properties of poly (butylene carbonate) reinforced by stereocomplex polylactide. Int. J. Biol. Macromol. 137, 1169–1178. doi:10.1016/j.ijbiomac.2019.07.068
Li, Y., Wei, J., Wei, Y., Cheng, L., Guo, B., Meng, F., et al. (2019b). Apolipoprotein E peptide-guided disulfide-cross-linked micelles for targeted delivery of sorafenib to hepatocellular carcinoma. Biomacromolecules 21 (2), 716–724. doi:10.1021/acs.biomac.9b01419
Li, Z., Zhang, S., Chen, Y., Ling, H., Zhao, L., Luo, G., et al. (2020). Gelatin methacryloyl‐based tactile sensors for medical wearables. Adv. Funct. Mat. 30 (49), 2003601. doi:10.1002/adfm.202003601
Li, C., Johansson, M., Buijsen, P., Dijkstra, G., Sablong, R. J., and Koning, C. E. (2021). Limonene-derived polycarbonates as biobased UV-curable (powder) coating resins. Prog. Org. Coat. 151, 106073. doi:10.1016/j.porgcoat.2020.106073
Liang, Z. C., Yang, C., Ding, X., Hedrick, J. L., Wang, W., and Yang, Y. Y. (2021). Carboxylic acid-functionalized polycarbonates as bone cement additives for enhanced and sustained release of antibiotics. J. Control. Release 329, 871–881. doi:10.1016/j.jconrel.2020.10.018
Liechty, W. B., Kryscio, D. R., Slaughter, B. V., and Peppas, N. A. (2010). Polymers for drug delivery systems. Annu. Rev. Chem. Biomol. Eng. 1, 149–173. doi:10.1146/annurev-chembioeng-073009-100847
Lim, F., and Sun, A. M. (1980). Microencapsulated islets as bioartificial endocrine pancreas. Science 210 (4472), 908–910. doi:10.1126/science.6776628
Lim, J., You, M., Li, J., and Li, Z. (2017). Emerging bone tissue engineering via Polyhydroxyalkanoate (PHA)-based scaffolds. Mater. Sci. Eng. C 79, 917–929. doi:10.1016/j.msec.2017.05.132
Lin, T. H., Wang, H. C., Cheng, W. H., Hsu, H. C., and Yeh, M. L. (2019). Osteochondral tissue regeneration using a tyramine-modified bilayered PLGA scaffold combined with articular chondrocytes in a porcine model. Int. J. Mol. Sci. 20 (2), 326. doi:10.3390/ijms20020326
Liu, Y., Huang, K., Peng, D., Liu, S., and Wu, H. (2007). Preparation of poly (butylene‐co‐ε‐caprolactone carbonate) and their use as drug carriers for a controlled delivery system. J. Polym. Sci. A. Polym. Chem. 45 (11), 2152–2160. doi:10.1002/pola.21981
Liu, Y., Peng, D., Huang, K., Liu, S., and Liu, Z. (2011). Preparation and properties of poly (propylene carbonate maleate) microcapsules for controlled release of pazufloxacin mesilate. J. Appl. Polym. Sci. 122 (5), 3248–3254. doi:10.1002/app.34351
Liu, Y., Zheng, Z., Zara, J. N., Hsu, C., Soofer, D. E., Lee, K. S., et al. (2012). The antimicrobial and osteoinductive properties of silver nanoparticle/poly (DL-lactic-co-glycolic acid)-coated stainless steel. Biomaterials 33 (34), 8745–8756. doi:10.1016/j.biomaterials.2012.08.010
Liu, Q., Wu, L., Jackstell, R., and Beller, M. (2015). Using carbon dioxide as a building block in organic synthesis. Nat. Commun. 6 (1), 5933. doi:10.1038/ncomms6933
Liu, G. L., Wu, H. W., Lin, Z. I., Liao, M. G., Su, Y. C., Chen, C. K., et al. (2021). Synthesis of functional CO2-based polycarbonates via dinuclear nickel nitrophenolate-based catalysis for degradable surfactant and drug-loaded nanoparticle applications. Polym. Chem. 12 (9), 1244–1259. doi:10.1039/D0PY01755H
Loh, E. Y. X., Mohamad, N., Fauzi, M. B., Ng, M. H., Ng, S. F., and Mohd Amin, M. C. I. (2018). Development of a bacterial cellulose-based hydrogel cell carrier containing keratinocytes and fibroblasts for full-thickness wound healing. Sci. Rep. 8 (1). doi:10.1038/s41598-018-21174-7
Loyo, C., Moreno-Serna, V., Fuentes, J., Amigo, N., Sepúlveda, F. A., Ortiz, J. A., et al. 2 (2022). PLA/CaO nanocomposites with antimicrobial and photodegradation properties. Polym. Degrad. Stab. 197, 109865. doi:10.1016/j.polymdegradstab.2022.109865
Luckachan, G. E., and Pillai, C. (2011). Biodegradable polymers-a review on recent trends and emerging perspectives. J. Polym. Environ. 19 (3), 637–676. doi:10.1007/s10924-011-0317-1
Lyu, Z., Ding, L., Huang, A. T., Kao, C. L., and Peng, L. (2019). Poly (amidoamine) dendrimers: Covalent and supramolecular synthesis. Mat. Today Chem. 13, 34–48. doi:10.1016/j.mtchem.2019.04.004
Maamoun, N. (2019). The Kyoto protocol: Empirical evidence of a hidden success. J. Environ. Econ. Manage. 95, 227–256. doi:10.1016/j.jeem.2019.04.001
MacDonald, R. A., Voge, C. M., Kariolis, M., and Stegemann, J. P. (2008). Carbon nanotubes increase the electrical conductivity of fibroblast-seeded collagen hydrogels. Acta biomater. 4 (6), 1583–1592. doi:10.1016/j.actbio.2008.07.005
Mahdieh, Z., Bagheri, R., Eslami, M., Amiri, M., Shokrgozar, M. A., and Mehrjoo, M. (2016). Thermoplastic starch/ethylene vinyl alcohol/forsterite nanocomposite as a candidate material for bone tissue engineering. Mater. Sci. Eng. C 69, 301–310. doi:10.1016/j.msec.2016.06.043
Malwela, T., and Ray, S. S. (2015). Enzymatic degradation behavior of nanoclay reinforced biodegradable PLA/PBSA blend composites. Int. J. Biol. Macromol. 77, 131–142. doi:10.1016/j.ijbiomac.2015.03.018
Manavitehrani, I., Fathi, A., Wang, Y., Maitz, P. K., Mirmohseni, F., Cheng, T. L., et al. (2017). Fabrication of a biodegradable implant with tunable characteristics for bone implant applications. Biomacromolecules 18 (6), 1736–1746. doi:10.1021/acs.biomac.7b00078
Manavitehrani, I., Le, T. Y., Daly, S., Wang, Y., Maitz, P. K., Schindeler, A., et al. (2019). Formation of porous biodegradable scaffolds based on poly (propylene carbonate) using gas foaming technology. Mater. Sci. Eng. C 96, 824–830. doi:10.1016/j.msec.2018.11.088
Martínez Ávila, H., Schwarz, S., Feldmann, E. M., Mantas, A., von Bomhard, A., Gatenholm, P., et al. (2014). Biocompatibility evaluation of densified bacterial nanocellulose hydrogel as an implant material for auricular cartilage regeneration. Appl. Microbiol. Biotechnol. 98 (17), 7423–7435. doi:10.1007/s00253-014-5819-z
Metwally, S., Ferraris, S., Spriano, S., Krysiak, Z. J., Kaniuk, Ł., Marzec, M. M., et al. (2020). Surface potential and roughness controlled cell adhesion and collagen formation in electrospun PCL fibers for bone regeneration. Mat. Des. 194, 108915. doi:10.1016/j.matdes.2020.108915
Meyer, K., and Palmer, J. W. (1934). The polysaccharide of the vitreous humor. J. Biol. Chem. 107 (3), 629–634. doi:10.1016/S0021-9258(18)75338-6
Mishra, S., Singh, P. K., Dash, S., and Pattnaik, R. (2018). Microbial pretreatment of lignocellulosic biomass for enhanced biomethanation and waste management. Biotech. 8 (11), 458. doi:10.1007/s13205-018-1480-z
Mittermayr, R., Slezak, P., Haffner, N., Smolen, D., Hartinger, J., Hofmann, A., et al. (2016). Controlled release of fibrin matrix-conjugated platelet derived growth factor improves ischemic tissue regeneration by functional angiogenesis. Acta Biomater. 29, 11–20. doi:10.1016/j.actbio.2015.10.028
Miyamoto, M., and Takeuchi, K. (2019). Climate agreement and technology diffusion: Impact of the Kyoto Protocol on international patent applications for renewable energy technologies. Energy policy 129, 1331–1338. doi:10.1016/j.enpol.2019.02.053
Modjarrad, K., and Ebnesajjad, S. (2013). Handbook of polymer applications in medicine and medical devices. Elsevier.
Monteiro, I., Gabriel, D., Timko, B., Hashimoto, M., Karajanagi, S., Tong, R., et al. (2014). A two-component pre-seeded dermal–epidermal scaffold. Acta Biomater. 10 (12), 4928–4938. doi:10.1016/j.actbio.2014.08.029
Mora-Huertas, C. E., Fessi, H., and Elaissari, A. (2010). Polymer-based nanocapsules for drug delivery. Int. J. Pharm. X. 385 (1-2), 113–142. doi:10.1016/j.ijpharm.2009.10.018
Morgan, J. L., Strumillo, J., and Zimmer, J. (2013). Crystallographic snapshot of cellulose synthesis and membrane translocation. Nature 493 (7431), 181–186. doi:10.1038/nature11744
Muangman, P., Opasanon, S., Suwanchot, S., and Thangthed, O. (2011). A case report: Efficiency of microbial cellulose dressing in partial thickness burn wounds. J. Am. Coll. Certif. Wound Specialists 3 (1), 16–19. doi:10.1016/j.jcws.2011.03.003
Mulinti, P., Brooks, J. E., Lervick, B., Pullan, J. E., and Brooks, A. E. (2018). “10 Strategies to improve the hemocompatibility of biodegradable biomaterials,” in Hemocompatibility of biomaterials for clinical applications. Editor C. A. Siedlecki (Cambridge, England: Woodhead Publishing), 253–278. doi:10.1016/B978-0-08-100497-5.00017-3
Neacsu, I. A., bCAaLeau, S. A., cCAb, , Marin, S. t., Holban, A. M., Vasile, B. S., et al. (2021a). Collagen-carboxymethylcellulose biocomposite wound-dressings with antimicrobial activity. Materials 14, 1153. doi:10.3390/ma14051153
Neacsu, I. A., Leau, S. A., Marin, S., Holban, A. M., Vasile, B. S., Nicoara, A. I., et al. (2021b). Collagen-carboxymethylcellulose biocomposite wound-dressings with antimicrobial activity. Materials 14 (5), 1153. doi:10.3390/ma14051153
Neo, K. R. S., and Yang, K. L. (2015). Continuous hydrolysis of carboxymethyl cellulose with cellulase aggregates trapped inside membranes. Enzyme Microb. Technol. 78, 34–39. doi:10.1016/j.enzmictec.2015.06.005
Ngoenkam, J., Faikrua, A., Yasothornsrikul, S., and Viyoch, J. (2010). Potential of an injectable chitosan/starch/β-glycerol phosphate hydrogel for sustaining normal chondrocyte function. Int. J. Pharm. X. 391 (1-2), 115–124. doi:10.1016/j.ijpharm.2010.02.028
Nguyen, T. V., Nguyen, T. T. H., Wang, S. L., Vo, T. P. K., and Nguyen, A. D. (2017). Preparation of chitosan nanoparticles by TPP ionic gelation combined with spray drying, and the antibacterial activity of chitosan nanoparticles and a chitosan nanoparticle–amoxicillin complex. Res. Chem. Intermed. 43 (6), 3527–3537. doi:10.1007/s11164-016-2428-8
Niaounakis, M. (2014). Biopolymers: Processing and products. William andrew. Kidlington, UK: Elsevier.
Nonato, R., Mei, L., Bonse, B., Chinaglia, E., and Morales, A. (2019). Nanocomposites of PLA containing ZnO nanofibers made by solvent cast 3D printing: Production and characterization. Eur. Polym. J. 114, 271–278. doi:10.1016/j.eurpolymj.2019.02.026
Nurwito, B. S., and Maulida, H. N. (2018). Electrospun fibers as a wound dressing material using combination of cellulose acetate/collagen seeding stem cell. Asian J. Microbiol. Biotechnol. Environ. Sci. 20, s43–s47.
Olson, D. G., McBride, J. E., Shaw, A. J., and Lynd, L. R. (2012). Recent progress in consolidated bioprocessing. Curr. Opin. Biotechnol. 23 (3), 396–405. doi:10.1016/j.copbio.2011.11.026
O’Meara, S., Martyn‐St James, M., and Adderley, U. (2015). Alginate dressings for venous leg ulcers. Cochrane db. Syst. Rev. 8. doi:10.1002/14651858
Pan, S. Y., Chiang, P. C., Pan, W., and Kim, H. (2018). Advances in state-of-art valorization technologies for captured CO2 toward sustainable carbon cycle. Crit. Rev. Environ. Sci. Technol. 48 (5), 471–534. doi:10.1080/10643389.2018.1469943
Pang, X., Duan, R., Li, X., and Chen, X. (2014a). Bimetallic salen–aluminum complexes: Synthesis, characterization and their reactivity with rac-lactide and ε-caprolactone. Polym. Chem. 5 (12), 3894–3900. doi:10.1039/C3PY01774E
Pang, X., Duan, R., Li, X., Gao, B., Sun, Z., Wang, X., et al. (2014b). Bimetallic Schiff-base aluminum complexes based on pentaerythrityl tetramine and their stereoselective polymerization of racemic lactide. RSC Adv. 4 (43), 22561–22566. doi:10.1039/c4ra02092h
Pang, X., Duan, R., Li, X., Sun, Z., Zhang, H., Wang, X., et al. (2014c). Highly stereoselective bimetallic complexes for lactide and ε-caprolactone polymerization. RSC Adv. 4 (100), 57210–57217. doi:10.1039/C4RA11126E
Pourmollaabbassi, B., Karbasi, S., and Hashemibeni, B. (2016). Evaluate the growth and adhesion of osteoblast cells on nanocomposite scaffold of hydroxyapatite/titania coated with poly hydroxybutyrate. Adv. Biomed. Res. 5, 156. doi:10.4103/2277-9175.188486
Priemel, P. A., Wang, Y., Bohr, A., Water, J. J., Yang, M., and Mørck Nielsen, H. (2018). Poly (ethylene carbonate)-containing polylactic acid microparticles with rifampicin improve drug delivery to macrophages. J. Pharm. Pharmacol. 70 (8), 1009–1021. doi:10.1111/jphp.12937
Qin, H., Li, J., He, B., Sun, J., Li, L., and Qian, L. (2018). Novel wearable electrodes based on conductive chitosan fabrics and their application in smart garments. Materials 11 (3), 370. doi:10.3390/ma11030370
Quan, Z., Luo, C., Zhu, B., Zhao, C., Yang, M., Bjørås, M., et al. (2021). Synthesis and antimicrobial activities of chitosan/polypropylene carbonate-based nanoparticles. RSC Adv. 11 (17), 10121–10129. doi:10.1039/D0RA09257F
Rahman, F. A., Aziz, M. M. A., Saidur, R., Bakar, W. A. W. A., Hainin, M., Putrajaya, R., et al. (2017). Pollution to solution: Capture and sequestration of carbon dioxide (CO2) and its utilization as a renewable energy source for a sustainable future. Renew. Sustain. Energy Rev. 71, 112–126. doi:10.1016/j.rser.2017.01.011
Rajabi, N., Rezaei, A., Kharaziha, M., Bakhsheshi-Rad, H. R., Luo, H., RamaKrishna, S., et al. (2021). Recent advances on bioprinted gelatin methacrylate-based hydrogels for tissue repair. Tissue Eng. Part A 27 (11-12), 679–702. doi:10.1089/ten.tea.2020.0350
Ramanathan, G., Seleenmary Sobhanadhas, L. S., Sekar Jeyakumar, G. F., Devi, V., Sivagnanam, U. T., and Fardim, P. (2020). Fabrication of biohybrid cellulose acetate-collagen bilayer matrices as nanofibrous spongy dressing material for wound-healing application. Biomacromolecules 21 (6), 2512–2524. doi:10.1021/acs.biomac.0c00516
Ricklefs, M., Korossis, S., Haverich, A., and Schilling, T. (2017). Polymeric scaffolds for bioartificial cardiovascular prostheses. Scaffolds Tissue Eng. Mat., Technol. Clin. Appl. Chapter 12, 267–291. doi:10.5772/intechopen.71846
Rokutanda, S., Yanamoto, S., Yamada, S. I., Naruse, T., Inokuchi, S., and Umeda, M. (2015). Application of polyglycolic acid sheets and fibrin glue spray to bone surfaces during oral surgery: A case series. J. Oral Maxillofac. Surg. 73 (5), 1017.e1–1017.e6. e1016. doi:10.1016/j.joms.2015.01.014
Sajjadi, M., Nasrollahzadeh, M., Jaleh, B., Soufi, G. J., and Iravani, S. (2021). Carbon-based nanomaterials for targeted cancer nanotherapy: Recent trends and future prospects. J. Drug Target. 29 (7), 716–741. doi:10.1080/1061186X.2021.1886301
Schüller‐Ravoo, S., Teixeira, S. M., Feijen, J., Grijpma, D. W., and Poot, A. A. (2013). Flexible and elastic scaffolds for cartilage tissue engineering prepared by stereolithography using poly (trimethylene carbonate)‐based resins. Macromol. Biosci. 13 (12), 1711–1719. doi:10.1002/mabi.201300399
Selvakumar, M., Pawar, H. S., Francis, N. K., Das, B., Dhara, S., and Chattopadhyay, S. (2016). Excavating the role of aloe vera wrapped mesoporous hydroxyapatite frame ornamentation in newly architectured polyurethane scaffolds for osteogenesis and guided bone regeneration with microbial protection. ACS Appl. Mat. Interfaces 8 (9), 5941–5960. doi:10.1021/acsami.6b01014
Shaghaleh, H., Xu, X., and Wang, S. (2018). Current progress in production of biopolymeric materials based on cellulose, cellulose nanofibers, and cellulose derivatives. RSC Adv. 8 (2), 825–842. doi:10.1039/C7RA11157F
Sharifianjazi, F., Khaksar, S., Esmaeilkhanian, A., Bazli, L., Eskandarinezhad, S., Salahshour, P., et al. (2022). Advancements in fabrication and application of chitosan composites in implants and dentistry: A review. Biomolecules 12 (2), 155. doi:10.3390/biom12020155
Shishatskaya, E. I., 2, Nikolaeva, E. D., Vinogradova, O. N., and Volova, T. G.2 (2016). Experimental wound dressings of degradable PHA for skin defect repair. J. Mat. Sci. Mat. Med. 27, 165–169. doi:10.1007/s10856-016-5776-4
Shokraei, N., Asadpour, S., Shokraei, S., Nasrollahzadeh Sabet, M., Faridi‐Majidi, R., and Ghanbari, H. (2019). Development of electrically conductive hybrid nanofibers based on CNT‐polyurethane nanocomposite for cardiac tissue engineering. Microsc. Res. Tech. 82 (8), 1316–1325. doi:10.1002/jemt.23282
Simman, R., Mari, W., Younes, S., and Wilson, M. (2018). Use of hyaluronic acid–based biological bilaminar matrix in wound bed preparation: A case series. Eplasty 18, 151–161.
Sinclair, R. (1996). The case for polylactic acid as a commodity packaging plastic. J. Macromol. Sci. Part A 33 (5), 585–597. doi:10.1080/10601329608010880
Smith, B. R., and Gambhir, S. S. (2017). Nanomaterials for in vivo imaging. Chem. Rev. 117 (3), 901–986. doi:10.1021/acs.chemrev.6b00073
Song, Y., Wennink, J. W., Kamphuis, M. M., Sterk, L. M., Vermes, I., Poot, A. A., et al. (2011). Dynamic culturing of smooth muscle cells in tubular poly (trimethylene carbonate) scaffolds for vascular tissue engineering. Tissue Eng. Part A 17 (3-4), 381–387. doi:10.1089/ten.tea.2009.0805
Sun, Z., Zhang, H., Pang, X., Bian, X., Chen, W., and Chen, X. (2014). Synthesis, characterization and application of methyl 3, 5-disulfo-benzoate dipotassium dihydrate as nucleating agent for Poly (L-lactide). Chem. Res. Chin. Univ. 30 (2), 333–338. doi:10.1007/s40242-013-3275-4
Sun, Z., Duan, R., Zhang, H., Pang, X., Wang, X., and Chen, X. (2015a). Highly stereoselective polymerization of racemic lactide by bimetallic schiff base complexes. J. Renew. Mat. 3 (2), 82–90. doi:10.7569/jrm.2014.634133
Sun, Z., Zhang, B., Bian, X., Feng, L., Zhang, H., Duan, R., et al. (2015b). Synergistic effect of PLA–PBAT–PLA tri-block copolymers with two molecular weights as compatibilizers on the mechanical and rheological properties of PLA/PBAT blends. RSC Adv. 5 (90), 73842–73849. doi:10.1039/c5ra11019j
Sun, Z., Duan, R., Yang, J., Zhang, H., Li, S., Pang, X., et al. (2016). Bimetallic Schiff base complexes for stereoselective polymerisation of racemic-lactide and copolymerisation of racemic-lactide with ε-caprolactone. RSC Adv. 6 (21), 17531–17538. doi:10.1039/c6ra00289g
Sun, Z., Duan, R., Xing, D., Pang, X., Li, Z., and Chen, X. (2017). Recent advances in application of poly-epsilon-caprolactone and its derivative copolymers for controlled release of anti-tumor drugs. Curr. Cancer Drug Targets 17 (5), 445–455. doi:10.2174/1568009617666170109150430
Sun, H., Liu, C. Z., Liu, C., Tang, M., Cao, G., Zhang, Q., et al. (2019a). Employing the sirolimus-eluting poly (propylene carbonate) mesh for the prevention of arteriovenous graft stenosis in rats. J. Cardiovasc. Pharmacol. Ther. 24 (3), 269–277. doi:10.1177/1074248418806060
Sun, Y., Zhao, Y. Q., Zeng, Q., Wu, Y. W., Hu, Y., Duan, S., et al. (2019b). Dual-functional implants with antibacterial and osteointegration-promoting performances. ACS Appl. Mat. Interfaces 11 (40), 36449–36457. doi:10.1021/acsami.9b14572
Szentivanyi, A., Assmann, U., Schuster, R., and Glasmacher, B. (2009). Production of biohybrid protein/PEO scaffolds by electrospinning. Materwiss. Werksttech. 40 (1‐2), 65–72. doi:10.1002/mawe.200800376
Takeuchi, J., Suzuki, H., Murata, M., Kakei, Y., Ri, S., Umeda, M., et al. (2013). Clinical evaluation of application of polyglycolic acid sheet and fibrin glue spray for partial glossectomy. J. Oral Maxillofac. Surg. 71 (2), e126–e131. doi:10.1016/j.joms.2012.08.012
Tamburaci, S., and Tihminlioglu, F. (2021). Development of Si doped nano hydroxyapatite reinforced bilayer chitosan nanocomposite barrier membranes for guided bone regeneration. Mater. Sci. Eng. C 128, 112298. doi:10.1016/j.msec.2021.112298
Tian, H., Tang, Z., Zhuang, X., Chen, X., and Jing, X. (2012). Biodegradable synthetic polymers: Preparation, functionalization and biomedical application. Prog. Polym. Sci. 37 (2), 237–280. doi:10.1016/j.progpolymsci.2011.06.004
Voo, Z. X., Khan, M., Narayanan, K., Seah, D., Hedrick, J. L., and Yang, Y. Y. (2015). Antimicrobial/antifouling polycarbonate coatings: Role of block copolymer architecture. Macromolecules 48 (4), 1055–1064. doi:10.1021/ma5022488
Wang, J., Gao, C., Zhang, Y., and Wan, Y. (2010). Preparation and in vitro characterization of BC/PVA hydrogel composite for its potential use as artificial cornea biomaterial. Mater. Sci. Eng. C 30 (1), 214–218. doi:10.1016/j.msec.2009.10.006
Wang, J., Zheng, L., Li, C., Zhu, W., Zhang, D., Xiao, Y., et al. (2012). Fully biodegradable blends of poly (butylene succinate) and poly (butylene carbonate): Miscibility, thermal properties, crystallization behavior and mechanical properties. Polym. Test. 31 (1), 39–45. doi:10.1016/j.polymertesting.2011.09.005
Wang, X., Zhuang, Y., and Dong, L. (2013). Study of biodegradable polylactide/poly (butylene carbonate) blend. J. Appl. Polym. Sci. 127 (1), 471–477. doi:10.1002/app.37735
Wang, Y., Pisapati, A. V., Zhang, X. F., and Cheng, X. (2021). Recent developments in nanomaterial‐based shear‐sensitive drug delivery systems. Adv. Healthc. Mat. 10 (13), 2002196. doi:10.1002/adhm.202002196
Weems, A. C., Arno, M. C., Yu, W., Huckstepp, R. T., and Dove, A. P. (2021). 4D polycarbonates via stereolithography as scaffolds for soft tissue repair. Nat. Commun. 12 (1), 3771. doi:10.1038/s41467-021-23956-6
Welle, A., Kröger, M., Döring, M., Niederer, K., Pindel, E., and Chronakis, I. S. (2007). Electrospun aliphatic polycarbonates as tailored tissue scaffold materials. Biomaterials 28 (13), 2211–2219. doi:10.1016/j.biomaterials.2007.01.024
Weller, C. D., Team, V., and Sussman, G. (2020). First-line interactive wound dressing update: A comprehensive review of the evidence. Front. Pharmacol. 11, 155. doi:10.3389/fphar.2020.00155
Wendels, S., and Avérous, L. (2021). Biobased polyurethanes for biomedical applications. Bioact. Mat. 6 (4), 1083–1106. doi:10.1016/j.bioactmat.2020.10.002
Whelan, D., Caplice, N., and Clover, A. (2014). Fibrin as a delivery system in wound healing tissue engineering applications. J. Control. Release 196, 1–8. doi:10.1016/j.jconrel.2014.09.023
Wu, D., Li, W., Zhao, Y., Deng, Y., Zhang, H., Zhang, H., et al. (2015). Thermal, mechanical and rheological properties of biodegradable poly (propylene carbonate) and poly (butylene carbonate) blends. Chin. J. Polym. Sci. 33 (3), 444–455. doi:10.1007/s10118-015-1597-z
Wu, J., Su, C., Jiang, L., Ye, S., Liu, X., and Shao, W. (2018). Green and facile preparation of chitosan sponges as potential wound dressings. ACS Sustain. Chem. Eng. 6 (7), 9145–9152. doi:10.1021/acssuschemeng.8b01468
Xavier Mendes, A., Moraes Silva, S., O’Connell, C. D., Duchi, S., Quigley, A. F., Kapsa, R. M., et al. (2021). Enhanced electroactivity, mechanical properties, and printability through the addition of graphene oxide to photo-cross-linkable gelatin methacryloyl hydrogel. ACS Biomater. Sci. Eng. 7 (6), 2279–2295. doi:10.1021/acsbiomaterials.0c01734
Xu, Q., Ke, X., Shen, L., Ge, N., Zhang, Y., Fu, F., et al. (2018). Surface modification by carboxymethy chitosan via pad-dry-cure method for binding Ag NPs onto cotton fabric. Int. J. Biol. Macromol. 111, 796–803. doi:10.1016/j.ijbiomac.2018.01.091
Xu, C., Wang, H., Li, X., Zhou, W., Wang, C., and Wang, S. (2019). Explosion characteristics of a pyrolysis biofuel derived from rice husk. J. Hazard. Mat. 369, 324–333. doi:10.1016/j.jhazmat.2019.01.101
Xu, G., Li, Y., Hou, W., Wang, S., and Kong, F. (2021). Effects of substrate type on enhancing pollutant removal performance and reducing greenhouse gas emission in vertical subsurface flow constructed wetland. J. Environ. Manage. 280, 111674. doi:10.1016/j.jenvman.2020.111674
Yadav, V., Sun, L., Panilaitis, B., and Kaplan, D. L. (2015). In vitro chondrogenesis with lysozyme susceptible bacterial cellulose as a scaffold. J. Tissue Eng. Regen. Med. 9 (12), E276–E288. doi:10.1002/term.1644
Yan, E., Cao, M., Wang, Y., Meng, Y., Zheng, H., Hao, X., et al. (2016). Degradable polyvinyl alcohol/poly (butylene carbonate) core–shell nanofibers for chemotherapy and tissue engineering. Mat. Lett. 167, 13–17. doi:10.1016/j.matlet.2015.12.113
Yang, T. I., Lin, P. L., Chang, G. W., Tseng, Y. C., Yu, Z. Y., Yang, C. B., et al. (2014). A new class of biocompatible tricalcium phosphate/polypropylene carbonate/polylactic acid nanocomposites with controlled flexibility and biodegradability. Curr. Nanosci. 10 (2), 194–199. doi:10.2174/1573413709666131129000432
Yang, Z., Hu, C., Duan, R., Sun, Z., Zhang, H., Pang, X., et al. (2019). Salen‐manganese complexes and their application in the ring‐opening polymerization of lactide and ϵ‐caprolactone. Asian J. Org. Chem. 8 (3), 376–384. doi:10.1002/ajoc.201800695
Yang, Z., Hu, C., Cui, F., Pang, X., Huang, Y., Zhou, Y., et al. (2022). One‐pot precision synthesis of AB, ABA and ABC block copolymers via switchable catalysis. Angew. Chem. Int. Ed. Engl. 61 (12), e202117533. doi:10.1002/anie.202117533
Yu, Y., Shen, M., Song, Q., and Xie, J. (2018). Biological activities and pharmaceutical applications of polysaccharide from natural resources: A review. Carbohydr. Polym. 183, 91–101. doi:10.1016/j.carbpol.2017.12.009
Yücel, E. A., Oral, O., Olgaç, V., and Oral, C. K. (2003). Effects of fibrin glue on wound healing in oral cavity. J. Dent. (Shiraz). 31 (8), 569–575. doi:10.1016/S0300-5712(03)00113-1
Yue, K., Trujillo-de Santiago, G., Alvarez, M. M., Tamayol, A., Annabi, N., and Khademhosseini, A. (2015). Synthesis, properties, and biomedical applications of gelatin methacryloyl (GelMA) hydrogels. Biomaterials 73, 254–271. doi:10.1016/j.biomaterials.2015.08.045
Yum, M. S., Ko, T. S., and Kim, D. W. (2012). β-Hydroxybutyrate increases the pilocarpine-induced seizure threshold in young mice. Brain Dev. 34 (3), 181–184. doi:10.1016/j.braindev.2011.05.012
Zaharescu, T., Tardei, C., Râpă, M., and Iordoc, M. (2020). Size particle effects on the thermal stability of poly (lactic acid)/hydroxyapatite hybrids for biodegradable package. Ceram. Int. 46 (6), 7288–7297. doi:10.1016/j.ceramint.2019.11.223
Zamani, M., Prabhakaran, M. P., San Thian, E., and Ramakrishna, S. (2015). Controlled delivery of stromal derived factor-1α from poly lactic-co-glycolic acid core–shell particles to recruit mesenchymal stem cells for cardiac regeneration. J. Colloid Interface Sci. 451, 144–152. doi:10.1016/j.jcis.2015.04.005
Zanetti, A. S., Sabliov, C., Gimble, J. M., and Hayes, D. J. (2013). Human adipose‐derived stem cells and three‐dimensional scaffold constructs: A review of the biomaterials and models currently used for bone regeneration. J. Biomed. Mat. Res. 101 (1), 187–199. doi:10.1002/jbm.b.32817
Zhang, L., Li, X., Yu, X., Li, Y., Sun, A., Huang, C., et al. (2017). Construction of vascularized pacemaker tissues by seeding cardiac progenitor cells and endothelial progenitor cells into Matrigel. Life Sci. 179, 139–146. doi:10.1016/j.lfs.2017.05.007
Zhang, L., Zhang, C., Zhuang, Z. N., Li, C. X., Pan, P., Zhang, C., et al. (2021a). Bio-inspired nanoenzyme for metabolic reprogramming and anti-inflammatory treatment of hyperuricemia and gout. Sci. China Chem. 64 (4), 616–628. doi:10.1007/s11426-020-9923-9
Zhang, X., Lin, Z. I., Yang, J., Liu, G. L., Hu, Z., Huang, H., et al. (2021b). Carbon dioxide-derived biodegradable and cationic polycarbonates as a new siRNA carrier for gene therapy in pancreatic cancer. Nanomaterials 11 (9), 2312. doi:10.3390/nano11092312
Zhao, Y., Zhu, B., Wang, Y., Liu, C., and Shen, C. (2019). Effect of different sterilization methods on the properties of commercial biodegradable polyesters for single-use, disposable medical devices. Mater. Sci. Eng. C 105, 110041. doi:10.1016/j.msec.2019.110041
Zheng, X., Hui, J., Li, H., Zhu, C., Hua, X., Ma, H., et al. (2017). Fabrication of novel biodegradable porous bone scaffolds based on amphiphilic hydroxyapatite nanorods. Mater. Sci. Eng. C 75, 699–705. doi:10.1016/j.msec.2017.02.103
Zhou, S. Y., Huang, H. D., Ji, X., Yan, D. X., Zhong, G. J., Hsiao, B. S., et al. (2016). Super-robust polylactide barrier films by building densely oriented lamellae incorporated with ductile in situ nanofibrils of poly (butylene adipate-co-terephthalate). ACS Appl. Mat. Interfaces 8 (12), 8096–8109. doi:10.1021/acsami.6b00451
Zhou, Y., Hu, C., Zhang, T., Xu, X., Duan, R., Luo, Y., et al. (2019). One-pot synthesis of diblock polyesters by catalytic terpolymerization of lactide, epoxides, and anhydrides. Macromolecules 52 (9), 3462–3470. doi:10.1021/acs.macromol.9b00001
Zhou, C., Xia, Y., Wei, Y., Cheng, L., Wei, J., Guo, B., et al. (2020). GE11 peptide-installed chimaeric polymersomes tailor-made for high-efficiency EGFR-targeted protein therapy of orthotopic hepatocellular carcinoma. Acta Biomater. 113, 512–521. doi:10.1016/j.actbio.2020.06.020
Zou, Y., Fang, Y., Meng, H., Meng, F., Deng, C., Zhang, J., et al. (2016). Self-crosslinkable and intracellularly decrosslinkable biodegradable micellar nanoparticles: A robust, simple and multifunctional nanoplatform for high-efficiency targeted cancer chemotherapy. J. Control. Release 244, 326–335. doi:10.1016/j.jconrel.2016.05.060
Zou, Y., Wei, Y., Wang, G., Meng, F., Gao, M., Storm, G., et al. (2017). Nanopolymersomes with an ultrahigh iodine content for high‐performance X‐ray computed tomography imaging in vivo. Adv. Mat. 29 (10), 1603997. doi:10.1002/adma.201603997
Keywords: nanocomposites, carbon dioxide fixation, reproducible, biomedical polymers, decarbonization
Citation: Liu X, Jiang Z, Xing D, Yang Y, Li Z and Sun Z (2022) Recent progress in nanocomposites of carbon dioxide fixation derived reproducible biomedical polymers. Front. Chem. 10:1035825. doi: 10.3389/fchem.2022.1035825
Received: 03 September 2022; Accepted: 20 September 2022;
Published: 07 October 2022.
Edited by:
Svetlana Tretsiakova, Ulster University, United KingdomReviewed by:
Dongfang Zhou, Southern Medical University, ChinaPiotr Rychter, Jan Długosz University, Poland
Copyright © 2022 Liu, Jiang, Xing, Yang, Li and Sun. This is an open-access article distributed under the terms of the Creative Commons Attribution License (CC BY). The use, distribution or reproduction in other forums is permitted, provided the original author(s) and the copyright owner(s) are credited and that the original publication in this journal is cited, in accordance with accepted academic practice. No use, distribution or reproduction is permitted which does not comply with these terms.
*Correspondence: Zhiwen Jiang, amlhbmd6dzIwQG1haWxzLmpsdS5lZHUuY24=; Zhiying Li, emh5aW5nbGkyMDEyQHNpbmEuY29t