- Bahir Dar Energy Center, Bahir Dar Institute of Technology, Bahir Dar University, Bahir Dar, Ethiopia
Rechargeable Zn-based batteries (RZBs) have garnered a great interest and are thought to be among the most promising options for next-generation energy storage technologies due to their low price, high levels of safety, adequate energy density and environmental friendliness. However, dendrite formation during stripping/plating prevents rechargeable zinc-based batteries from being used in real-world applications. Dendrite formation is still a concern, despite the fact that inhibitory strategies have been put up recently to eliminate the harmful effects of zinc dendrites. Thus, in order to direct the strategies for inhibiting zinc dendrite growth, it is vital to understand the formation mechanism of zinc dendrites. Hence, for the practical application of zinc-based batteries, is essential to use techniques that effectively prevent the creation and growth of zinc dendrites. The development and growth principles of zinc dendrites are first made clear in this review. The recent advances of solutions to the zinc dendrite problem are then discussed, including strategies to prevent dendrite growth and subsequent creation as much as possible, reduce the negative impacts of dendrites, and create dendrite-free deposition processes. Finally, the challenges and perspective for the development of zinc-based batteries are discussed.
1 Introduction
Numerous significant advancements have been made in the development of electrochemical energy storage systems over the past several years (Goh et al., 2013). Batteries are an example of an electrochemical energy storage technology that can store electric energy as chemical energy and convert the chemical energy to electric energy as needed (Worku et al, 2021a; Khamsanga et al., 2019). Thus, battery technologies can increase the use of renewable energy sources while reducing the use of limited fossil fuels (Li et al., 2016). Battery technology has so far been applied to stationary energy storage and power batteries. As an illustration, lithium-based batteries are typically used as the power source for electric vehicles, mobile phones, laptops, and other mobile devices (Worku et al., 2022). The use of lithium-ion batteries to store renewable energy is currently receiving a lot of interest despite the fact that these batteries pose safety risks, are expensive, and have a low energy density. As a result, numerous high-safety battery types have been suggested and thoroughly studied. Due to their high levels of safety and affordability, green and sustainable energy storage systems made of aluminum, zinc, potassium and sodium have recently gained a lot of attention (Zhong et al., 2021). Due to their plentiful resources, environmental friendliness, and high energy density, zinc-based rechargeable batteries, such as, Zn-Ni batteries, Zn-MnO2 batteries (Sumboja et al., 2015), zinc-ion batteries and zinc-based flow zinc-air batteries, are thought to be the most promising energy storage devices to replace lithium batteries (HabtuGabbiye et al., 2022).
Additionally, zinc has high capacity (5,854 Ah·L−1 and 820 Ah·kg−1), good electrochemical reversibility [0.762 V relative to the standard hydrogen electrode (SHE)], and does not readily corrode even in alkaline conditions (Abbasi et al., 2019). Zinc has a number of other advantages when used as an electrode material, including a high specific energy, a high power density, a low redox potential, nontoxicity, recyclability, and low cost. Zinc is currently one of the most widely utilized electrode materials because zinc-based batteries often have high energy density, low cost, high discharge voltage, and good environmental benignity. Zinc-based batteries are promise for the next wave of energy storage technologies because of these characteristics (Worku et al., 2021b). Notably, zinc oxides and their combinations can also function in addition to pure zinc. However, the issues of dendritic growth, self-corrosion, and morphological change are still unresolved, which has a significant impact on the efficiency of zinc plating and striping and the service life of zinc electrode (Worku et al., 2021c).
The ability to suppress dendrite growth in particular is crucial for improving the Coulombic efficiency (CE) and stability of zinc-based batteries (Hosseini et al., 2018). Due to unequal zinc deposition during the charging process of secondary zinc-based batteries, zinc dendrites are formed. The performance and lifespan of zinc-based batteries are significantly impacted by the presence of zinc dendrites (Fu et al., 2016).
In addition, zinc dendrites readily detach from electrodes in alkaline media, resulting in a decline in battery capacity and efficiency. Additionally, when zinc dendrites continue to grow, they eventually come into direct contact with the anode and cathode, creating a short circuit and the eventual collapse of the battery (Lee et al., 2016). Additionally, the dendritic shape might increase the zinc electrode’s specific surface area, which promotes zinc corrosion and lowers the zinc consumption rate. In addition, zinc dendrites would easily separate from the electrode surface to generate “dead” zinc as a result of the weak adhesion, reducing the battery’s capacity. In recent years, numerous strategies have been proposed to reduce uneven zinc deposition and enhance the cycling performance of zinc-based batteries (Ayele et al., 2021b). Rechargeable zinc-air batteries are still far from becoming commercially available for a number of reasons, one of which is the fact that the zinc anode has a poor CE as a result of the development of zinc dendrites (Zuo et al., 2021). Moreover, for a very long time, Li metal batteries have been plagued by dendrite formation and the dead Li that results from it. Recent research has suggested a novel redox mediator-based approach to lithium restoration. However, using some redox mediators frequently results in the unwanted side effect of significant self-discharge. Chen et al. (2022), reported a selection principle of redox mediators for reactivating dead Li in lithium metal batteries. This approach may both successfully reactivate the dead Li and decrease self-discharge. These strategies include adding additives to the electrolyte or anode metal, optimizing operating parameters, and removing the detrimental effects of dendrite growth. The main concepts behind these techniques can be broken down into three groups (I) approaches to prevent zinc dendrites from forming and growing further as much as possible; (II) approaches to reduce the negative effects caused by zinc dendrites; and (III) approaches to get rid of zinc dendrites and ultimately create a deposition process without them (Chu et al., 2022). The first approach, while currently the most popular way to address the dendritic problem, also has significant downsides, like greater electrode polarization. Although the second and third approaches are more difficult to implement, their effectiveness is thought to be sufficient, and certain concepts and real-world applications have been suggested (K. Wang Anran et al, 2020a). Despite the fact that these techniques have made significant strides in slowing zinc dendrite formation, it is still unknown what causes homogeneous zinc deposition. In order to provide workable inhibitory strategies for morphological control of electrodeposited zinc, additional research and knowledge of the process underlying zinc dendrite formation are therefore required (Worku et al., 2021d). In order to stabilize the Zn anodes, various techniques have been used. These include surface modification, structural design, and electrolyte control (Figure 1). These methods serve to improve the electrochemical performance of RZIBs by successfully suppressing Zn dendrite development and/or side reactions. This review outlines the theories and approaches for addressing zinc dendrite problems and reducing their negative effects (Lu et al., 2018). As a result, it can serve as a thorough reference to guide the advancement and practical use of zinc-based batteries in the future.
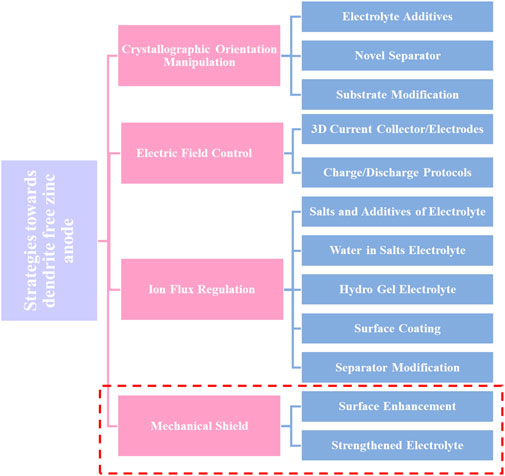
FIGURE 1. Schematic representation of the materials used in RZIBs to stabilize Zn anodes. Reproduced under the terms of the CC-BY Creative Commons Attribution 4.0 International license (Q. Li et al., 2020). Copyright (2022), Energy Materials.
2 Overview of Zn anode
Despite the development of alternate substitutes, Zn anodes remain the most optimal anodes for RZBs due to their incomparable benefits. The issues with Zn anodes may be found in numerous laboratory investigations that often include high anode sources, low current densities, and restricted loading mass in the cathode (Wang et al., 2015). Due to its “hostless” nature and uniform stripping and plating, Zn anodes inevitably experience dendritic difficulties, much like many other metal anodes. Metal anodes based on stripping and plating mechanism witnessed unlimited volume change as opposed to standard graphite anodes based on insertion mechanism since this “hostless” nature can lead to uncontrollable dendrites growth. In addition, there are other problems with the Zn anode aqueous system, such as corrosion, passivation, and hydrogen evolution, which are worse in alkaline electrolytes. Typically, Zn metal is used directly as the anode of RZIBs. Due to their security and affordability, RZIBs with Zn metal anodes have a lot of potential for large-scale energy storage. The anode-electrolyte interface problems are the main reason why their practical performances are still below expectations. The following sections discuss these problems, which mostly concern dendritic formation and side reactions on the surface of zinc anodes (Li et al. 2020a).
3 Dendrite formation and side reactions
3.1 Formation of dendrite
The reaction mechanism of a Zn anode in the mild aqueous electrolyte can be summed up as follows (Wang et al., 2021):
Diffusion, adsorption, growth and nucleation are the usual four phases that Zn2+ goes through during electrodeposition. The Zn anode surface microenvironment can affect these activities. Particularly, Zn anodes’ surfaces are not atomically smooth, which may lead to irregular electric field distribution, heterogeneous ion flux distribution, and various nucleation barrier sites (Guo et al., 2020). Therefore, under the unconstrained 2D Zn2+ diffusion, Zn2+ is more likely to adsorb and accumulate on the higher active sites (Figure 2A). Zn atomic clusters would then develop as a result of the Zn2+ nucleating on these locations. The distribution of the produced Zn atomic clusters on the surface of Zn is heterogeneous, which exacerbates the unequal field distribution (Zeng et al., 2019). Due to the tip effect, these clusters can also act as small protrusions with greater curvature and stimulate Zn dendrite formation (Figures 2B–D). A number of risks would be brought by the expanding Zn dendrites (Zuo et al., 2021). Due to the Zn dendrites’ porous and flimsy 3D shape, fresher Zn could come into contact with aqueous electrolytes, increasing the potential for side reactions (Xie et al., 2020). Additionally, because of the poor connection between the dendrites and anodes, the dendrites are vulnerable to rupturing away from the Zn substrate and turning into “dead Zn.” The insulating byproduct layer and inactive “dead Zn” increase the battery’s internal resistance and polarization (Figures 3A–C). Along with “dead Zn,” certain dendrites may develop continuously until they pierce the separator, which will result in a short circuit (Li et al. 2020b).
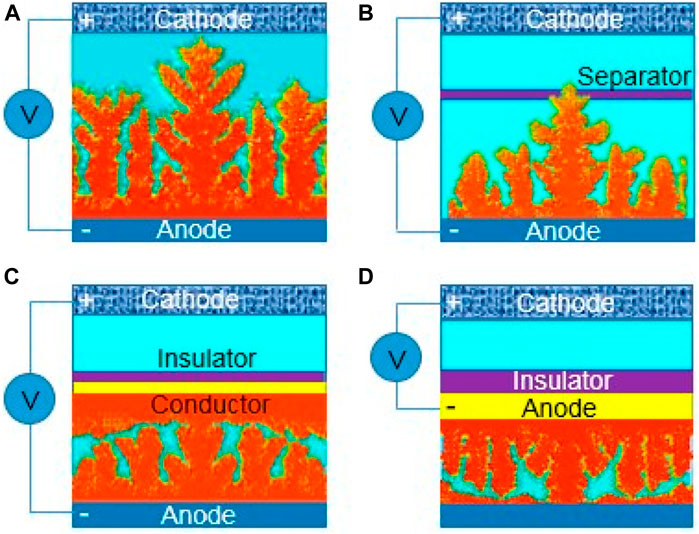
FIGURE 2. Dendrite growth of depositing zinc. (A) Dendrite growth causing short circuit of the batteries, (B) dendrite growth puncturing a separator, (C) partially conductive separator guiding dendrite growth, and (D) insulator encapsulating anode-reversing dendrite growth. Reproduced under the terms of the CC-BY Creative Commons Attribution 4.0 International license (Zhang et al., 2022). Copyright (2022), Energy Materials.
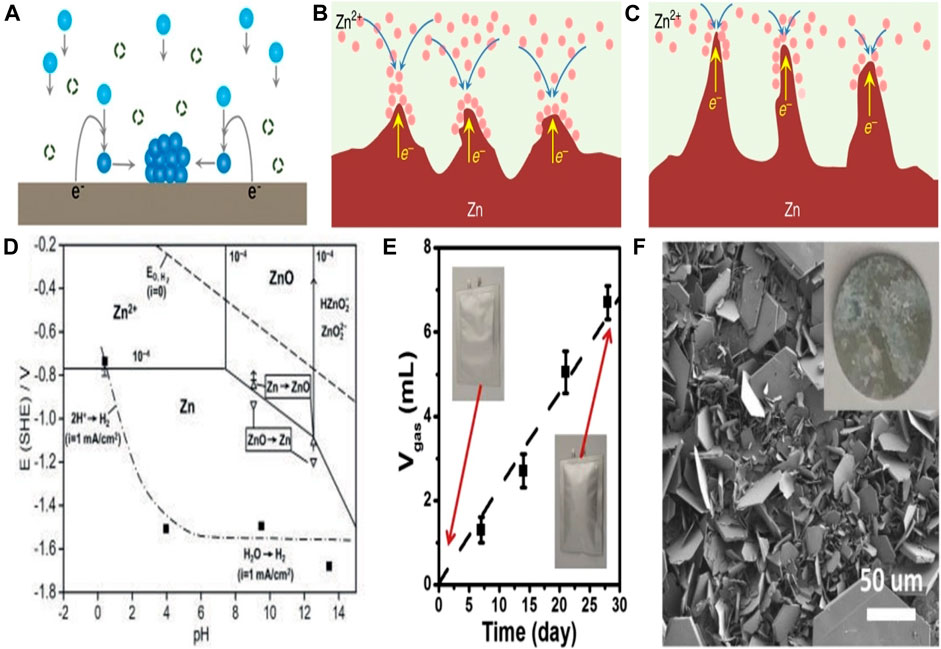
FIGURE 3. Schematic representation of the creation of Zn atomic clusters during unconstrained 2D Zn2+ diffusion. (A) Schematic representation of the tip effect in (B). Dendrite formation is option (C). Pourbaix diagram of the 10–4 M Zn2+ system in Zn/H2O. (D) The gas evolution of a Zn symmetric cell in a 3 M ZnSO4 electrolyte at various resting times. (E) The Zn anode’s surface morphology after 30 days of immersion in 3 M ZnSO4 electrolyte. (F) Reproduced under the terms of the CC-BY Creative Commons Attribution 4.0 International license (Zhang et al., 2022). Copyright (2022), Energy Materials.
3.2 Rechargeable Zn-based batteries side reactions
Other negative issues with Zn anodes include side reactions, such as passivation, corrosion, and HER, in addition to dendritic formation (Liu et al., 2019b). One of these is the Zn anode’s thermodynamic instability in an aqueous solution, which is the main contributor to the development of hydrogen (Li et al., 2022). The reaction of hydrogen evolution can be demonstrated as follows:
Surface corrosion from hydrogen evolution can result from chemical or electrochemical reactions. Normally, electrochemical cells are destroyed by hydrogen evolution because it raises internal pressure and causes the sealing to fail (Han et al., 2020). The Pourbaix diagram (Figure 3D) shows that in the whole pH range, Zn2+/Zn has a lower equilibrium potential than H2O/H2 does. Because of the thermodynamic activity of Zn in an aqueous solution, HER tends to develop on the surface of Zn metal anodes via chemical or electrochemical processes (Figure 3E). As a result, when RZIBs are charged, HER competes with the Zn plating and there would be an associated Zn corrosion process. HER raises the battery’s internal pressure, which may further increase polarization, cause the battery to swell and even rupture. Due to the accumulating OH−, HER also causes a rise in pH at the anode surface. In order to generate byproducts with limited solubility, such as Zn(OH)2, Zn4SO4(OH)6xH2O (ZHS), etc., the continuously rising OH− would further react with Zn2+ and the anion of Zn salts (Figure 3F). These byproducts, which act as electrical insulators and passivate the Zn surface to prevent additional Zn plating or peeling, block the sites. In an alkaline environment, the Zn anode’s passivation usually occurs by generating insulating ZnO on the anode’s surface, which prevents the anode from engaging in further electrochemical action. They can’t stop the additional HER and Zn corrosion on the Zn anodes because they are currently free in the framework (Tian et al., 2021). Zn and electrolytes around anodes are thus continuously consumed, which results in a lower CE. Additionally, the corrosion- and passivation-induced rough and uneven surface may hasten the formation of Zn dendrites (Mo et al., 2022).
3.3 Reducing side reactions
The Zn anode’s surface area grows when Zn dendrites develop. Surface-dependent processes that consume active Zn continuously and significantly lower battery capacity include corrosion reactions and the hydrogen evolution reaction. The gas will result in volume expansion of the batteries when the side reaction of hydrogen evolution takes place in a nearby high-energy location. In addition, insoluble Zn(OH)2 is created and attaches to the metal Zn surface, inducing surface passivation of the fresh Zn, as the local OH− concentration rises. This leads to a poor plating/stripping CE by decreasing the anode’s conductivity, raising the interface impedance, and decreasing the active Zn nucleation sites. The performance and longevity of batteries are put at risk by these irreversible hydrogen evolution, corrosion, and passivation side processes, which fundamentally deplete limited electrolyte and Zn ions (Wang et al, 2020a). A schematic representation representing Zn corrosion, passivation, and hydrogen development can be seen in Figure 4A.
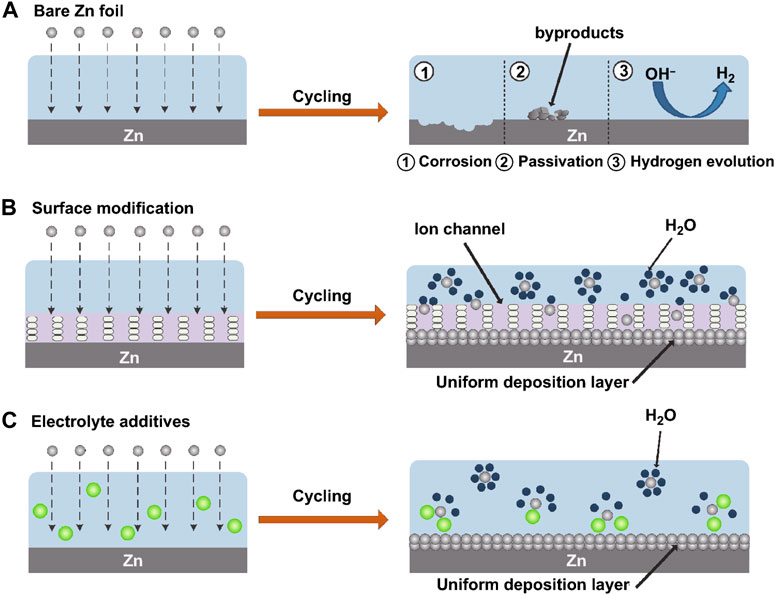
FIGURE 4. The passivation, HER and corrosion, on bare Zn. (A) A schematic illustration of coated Zn’s morphological development. (B) Schematic representation of the Zn ion deposition morphology after electrolyte additions. (C) CC-BY Creative Commons Attribution 4.0 International license was used to permit this reproduction (Li et al., 2022). Copyright (2022), Springer Nature.
3.3.1 Reducing active water
In aqueous electrolytes, Zn2+ and six water molecules combine to generate hydrated Zn2+([Zn(H2O)6]2+), which is the principal cause of side reactions. Before being reduced on the surface of the Zn anode, [Zn(H2O)6]2+ must go through a desolvation process, which inexorably results in direct contact between the Zn anode and water molecules and sets off side reactions. In order to increase the hydrogen evolution potential of metal Zn and lessen the corrosion response, it was discovered that adding atomic groups or solid electrolyte interface layers to the anode surface is advantageous. Additionally, the interfacial layer either directly blocks contact between the electrolyte and the Zn anode or lowers the amount of water molecules that are allowed to desolvate onto the Zn surface (Li et al., 2013). Following the protective layer, the Zn deposition is extremely uniform, as illustrated in Figure 4B.
3.3.2 Modulating coordination status
The parasitic water reduction during Zn deposition is sped up by the high overpotential created by the robust Coulomb contacts between the solvated Zn2+ and its surrounding H2O shell. As a result, a passivation layer and the evolution of H are encouraged. The strength of the connection between the Zn2+ ions and solvated H2O needs to be reduced in order to prevent water reduction and Zn dendrites. A quick and convenient method for improving the electrolyte composition is to add particular chemicals (Figure 4C). Some additions can solvate with Zn ions preferentially, substitute H2O in the Zn2+ solvated sheath, or remove H2O entirely (Liu et al., 2019b).
4 Engineering approaches of Zn metal anodes surface modification
The Zn metal electrode surface’s structure has a significant impact on the electrochemical performance of RZIBs. The surface of Zn metal electrodes has therefore been modified using a variety of techniques. The dominant crystallographic orientation and initial anode surface texture will have a significant impact on the following electrochemical behavior. The mass transfer process, which is primarily driven by the electric field and concertation gradient throughout the battery cycling process, commands the dendrite’s creation. From a mechanical standpoint, the strength of the interaction with physical shielding will have an impact on the dendrite growth. These techniques can be divided into four basic categories: Mechanical shielding, ion flow regulation, electric field control, and manipulation of crystallographic orientation (Tian et al., 2021). These techniques can be divided into several key categories, such as shielding the Zn metal to avoid side reactions, controlling the Zn deposition behavior, and producing a consistent electric field as shown in Figure 5. For future reference, the unexplored mechanical viewpoint that mechanical shielding inhibits dendrite formation is listed below. This section discusses a variety of techniques within each area.
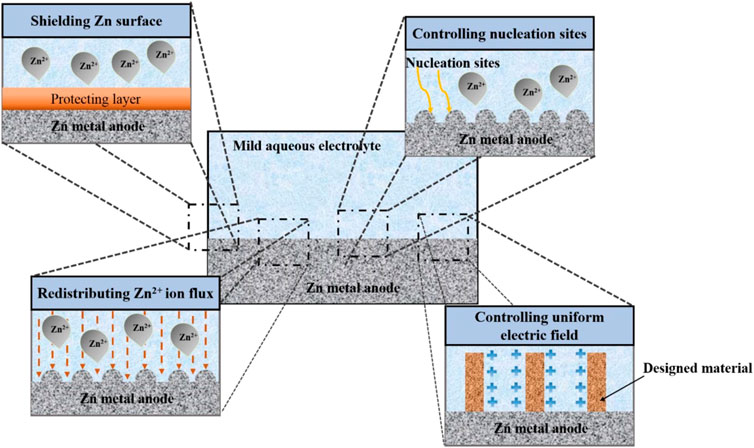
FIGURE 5. Techniques for modifying Zn metal anodes to improve their electrochemical performance. Reproduced with permission (Huy, Hieu, and Hur 2021). Copyright (2021), MDPI.
4.1 Electric field control
The electrochemical reaction is started by the movement of ions in an electric field. The identification of problems with electric field management resulting either from the simple design of electrode structures or from in-situ control of dendrite growth. The local areal current density will be significantly reduced by a well-designed anode structure or a 3D porous nanostructured anode, which will also result in less polarization and uniform deposition. The complex construction of the current collector will change the electric field’s uniform distribution, which will cause lateral growth rather of the vertical accumulation that has been observed in Li metal anodes. The nature of the electric field is also utilized for controlling advance charge/discharge protocols for removing dendrites with the intricate design (Yang et al., 2020).
Another efficient strategy for facilitating highly uniform Zn2- distribution is to encourage uniform electric field distribution. Thus, conductive carbon materials have frequently been added to Zn anodes to increase the electro-active surface area, further homogenize the electric field distribution, and reduce Zn dendritic growth. These materials include porous carbon film, carbon nanotube (CNT) scaffolds, reduced graphene oxide (rGO), carbon black, and graphite. In addition, layer-by-layer self-assembled MXene layers were created on Zn anodes in order to create ultrathin and uniform MXene layers and efficiently homogenize the electric field distribution as shown in Figures 6A,B). In order to shift the Zn2−concentration field and completely eradicate Zn dendrites, Zhi et al. demonstrated that hydrogen-substituted graphene (HsGDY) may be connected with a Zn electrode (Figures 6C–E).
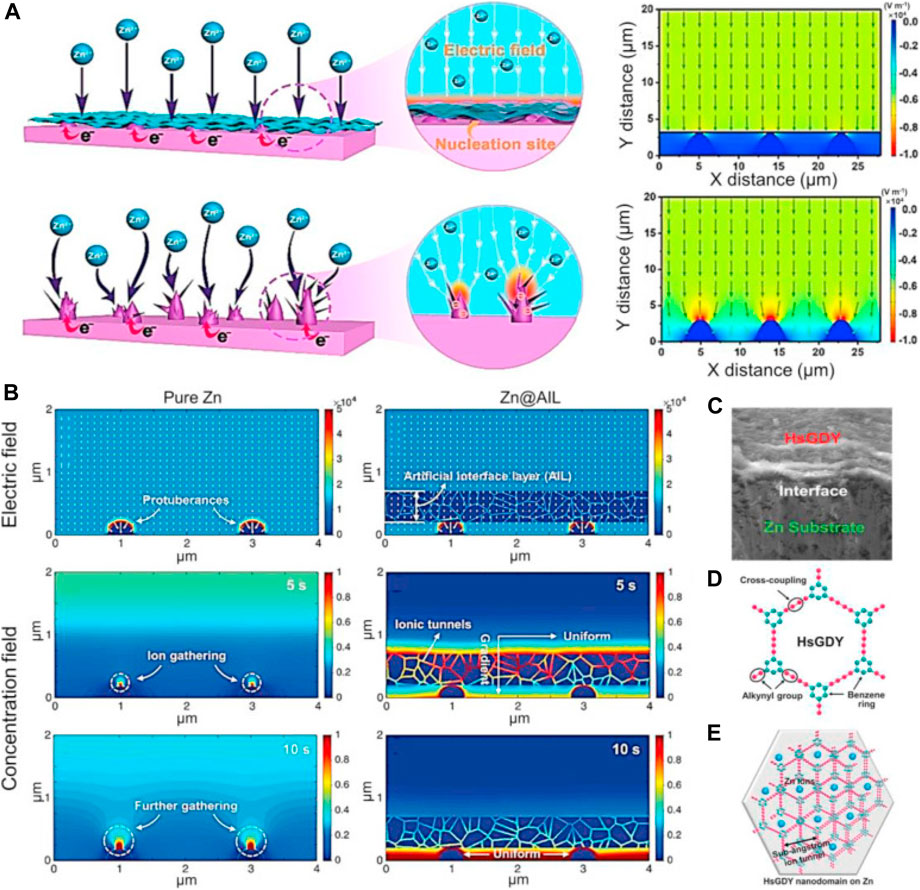
FIGURE 6. (A) Schematic representation of the behavior of zinc plating on pure zinc and zinc coated with MXene, together with the associated models of electric field distributions. Diagrams of the HsGDY and its sub-angstrom ion tunnel are shown in (B) dual-field simulations of pure Zn and Zn@HsGDY, (C) Zn@HsGDY cross-section, and (D,E). CC-BY Creative Commons Attribution 4.0 International license was used to permit this reproduction (Chu et al., 2022). Copyright (2022), Elsevier.
4.1.1 3D current collector/electrodes
Batteries, which focus on a 2D confusion and nucleation, typically use a planar current collector. In comparison to a planar structure, all 3D architectures should pay special attention to their bigger electroactive regions (Cao et al., 2020).
4.1.2 Charge/discharge protocols
Charge/discharge techniques, which are significantly more measurable and cost-effective, introduce specific in situ charge/discharge processes to modify dendrite formation. A recent paper described an electro-healing technique that combined cycling at high current density with a low current density stripping/plating process. Without giving the batteries any extra care, those techniques have a significant impact on applications and will pave the way for battery maintenance. To support the proposal, it is important to investigate the anode’s future long-term cycling impact and its viability with the available infrastructure (Huy, Hieu, and Hur 2021).
4.2 Ion flux regulation
The ion flux in the electrolyte can be influenced by numerous complex parameters, in contrast to the simple electric field control (Yu et al., 2020). The electrolyte, interfacial layer, and separator, along with other mass transfer processes occurring close to the interface while deposition takes place, have a significant impact on the ion movement caused by the electric field. We can get a consistent ion migration behavior and reduced polarization at the dendrite suppression by changing the electrolyte’s constituents and concentration (Wang et al., 2022). Aqueous and nonaqueous electrolytes can be used to classify the electrolyte (including ion liquid electrolytes and organic liquid electrolytes) (Zhang et al., 2022). According to PH, the majority of the electrolytes in RZBs are aqueous-based (including hydrogel and water-in-salt) and are classified as alkaline, neutral, or mildly acid. We concentrate on the aqueous-based electrolytes for RZB in this section. Meanwhile, interfacial modification can work as an ion filter for uniform movement and deposition as well as directly shield the anode from parasitic reaction. For a homogeneous plating, the functionalized separator will also promote uniform ion dispersion (Pan et al., 2018).
4.2.1 Salts and additives
The primary salts and additions are included in the electrolyte components. Two common salts, ZnSO4 and Zn (CF3SO3)2, have variable electrochemical properties depending on their stability, conductivity, and compatibility. To be more precise, Zn(CF3SO3)2 makes a difference in the potential hysteresis between plating and stripping. This is due to the large CF3SO3 anions, which will reduce the solvated sheath surrounding Zn2+ and aid Zn2+ migration. Even though Zn(CF3SO3)2 has a stronger electrochemical performance, ZnSO4 is the salt that offers the greatest potential for cost savings and environmental benefits (Zhu et al., 2021).
4.2.2 Water in salts electrolyte
Better electrolyte concentrations typically result in higher conductivity and lessened polarization in addition to the components. Another benefit of concentration is the destruction of the Zn2+solvation-sheath structure, which is linked to high Zn reversibility and is known as high concentration Zn ion electrolyte (HCZE) or water in salts (WIS) (Pan et al., 2016).
4.2.3 The hydrogel electrolyte
The most promising electrolyte for wearable technology is called a hydrogel electrolyte, which is a network of polymer chains with embedded electrolyte. It can provide improved mechanical strength, ion confinement, and ion dispersion by adding functional groups to the polymer chain. The functionalization can also cause an even deposition at the contact and control ion flux. Hydrogel electrolytes must carefully balance their ion conductivity and mechanical strength in order to withstand large current densities. On the other hand, water retention and stability will also be taken into account when commercialization is being considered (Zhang et al., 2016). Ling et al. (2021), reported Self-healable hydrogel electrolyte for dendrite-free and self-healable zinc-based aqueous batteries, which delivered a high capacity of 304 mAh·g−1 at 0.5 A·g−1 and good cycling stability with a capacity retention of 83.1% (vs. 62.5% with polyacrylamide) after 1,500 charge/discharge cycles at 5.0 A·g−1.
4.2.4 Surface coating
The most effective defense against dendritic problems for metal anodes is surface coating, which is often created through doctor blading, spin coating, and atomic layered deposition (Wang et al., 2018). There are two types of coatings: organic and inorganic. The formal one often serves as a barrier against adverse effects and offers a consistent ion route. Canpeng Li et al., developed a “all-in-one” (AIO) strategy by combining structural design, interface modification, and electrolyte optimization, inheriting the benefits of the 3D zinc anode and gel electrolyte with nearly no hydrogen evolution (Figure 7).
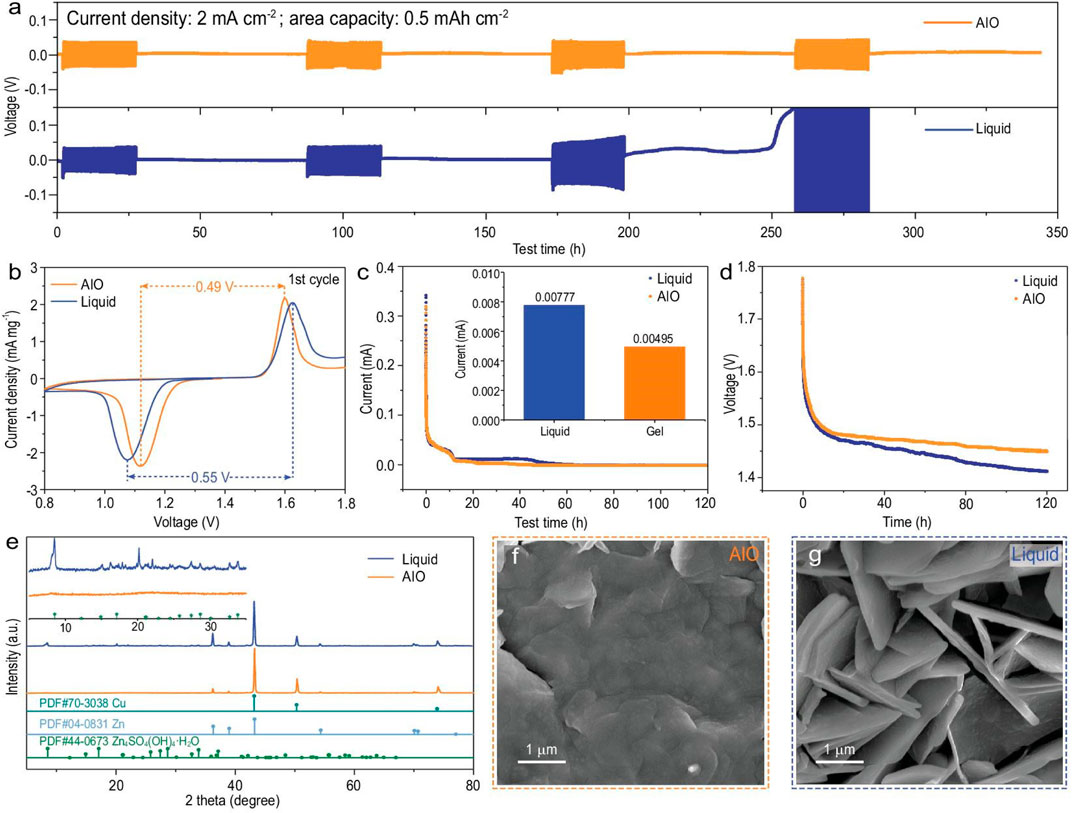
FIGURE 7. (A) Shelving-recovery performance of Cu foam@Zn/Cu foam@Zn symmetric cell (AIO electrode/Cu foam@Zn in AIO system) under 2 mA·cm−2. (B) First cyclic voltammetry curve, (C) float charge current, (D) open circuit potential decays of AIO electrode/α-MnO2 and Cu foam@Zn/α-MnO2 full cell. (E) XRD patterns of the anodes in different full cell systems after 100 cycles at 500 mA·g−1, and the corresponding SEM images of (F) AIO electrode and (G) Cu foam@Zn in 2 M ZnSO4 + 0.1 M MnSO4. Reproduced with permission (Li et al., 2022). Copyright (2022), Oxford University Press.
4.2.5 Separator modification
A separator is a crucial component in the prevention of short circuits. The ion flux will also be redistributed as a result. Currently, there are two main types of separators: nonwoven paper and glass fiber (Jia et al., 2020). Due to its great thickness and lack of economics, glass fiber has a high degree of resilience and chemical inducing to prevent dendrites. The commercialization of RZBs is economically aided by the nonwoven paper, however this paper’s worst property is that it is easily pierceable. A good mechanical property, low operating expenses, and improved ion flux guiding are desirable characteristics in a separator that inhibits dendrite formation (Zhang et al., 2017).
4.3 Mechanical shield
The mechanical elements will surely have an impact on the dendritic formation and short circuit process. There has not been any systematic research or simulation too far for RZBs to estimate this threshold (Li et al., 2020a). The crucial function of physical shielding can be seen in the apparent morphological difference between the deposition in the electric tank and a cell with a separator. The anhydrous property and increased mechanical strength should stabilize the Zn anodes. The problems to be solved in further research will be the low ionic conductivity and poor interfacial contact that are accompanied by high polarization. The operation of mechanical shielding is frequently coordinated with other surface coating, gel and solid-state electrolyte, and enhanced separator methods. The balance between mechanical property and electrochemical property, such as ion conductivity, and other factors, should be taken into consideration while creating robust physical shielding (Wang et al., 2019).
4.4 Crystallographic orientation manipulation
Zn is a crystalline substance, and the preferred crystallographic orientations can be changed throughout the electrodeposition process to control the surface texture. A dense and parallel orientation is what a properly produced Zn anode should have, and this can be accomplished by adjusting the substrate used and the electrodeposition electrolyte additive. While the substrate is modified via epitaxial electrodeposition, the electrolyte additive modifies the crystallographic by chemical contact (Zhang et al., 2020). The following Zn deposition tends to be oriented similarly during the battery cycling process as a result of the lattice match, which contributes to better electrochemical performance. The initial Zn anode can be controlled using straightforward techniques, making it feasible to produce stable Zn anodes in large quantities. However, the efficiency after prolonged cycling needs to be further assessed (Shin et al., 2020).
4.5 Engineering of substrate
4.5.1 Engineered materials and substrate selection
For dendritic suppression, it is crucial to select the right substrate or modified materials. In order to achieve efficient electron transport, the substrate for zinc deposition must be insoluble in aqueous electrolyte. Currently, porous carbon materials, copper foam, nickel foam, and foil are the most often utilized substrates. On the other hand, a crucial aspect of substrates’ characteristics is their zinc nucleation overpotential (Yang et al.). Low nucleation overpotential will result in a smaller potential barrier to overcome, allowing for uniform zinc deposition and improved zinc plating/stripping reversibility. Cu foam has been proven in prior research to have a number of inherent advantages as a substrate, and various modification techniques can further optimize zinc nucleation overpotential. The excellent adsorption ability of zinc atoms is advantageous for the uniform deposition when choosing modified materials. Stronger interactions between zinc and surface particles with strong electron interactions and polarization can both prevent zinc from aggregating on the substrate surface and from flaking off once it has been deposited (Mainar, Blazquez, and Urdampilleta 2017).
4.5.2 Substrate surface engineering
Metal affinity functional groups frequently control the surface of the optimal substrate, resulting in uniform locations for corresponding metal deposition (Qin et al., 2021). Zinc will be deposited uniformly as a result of the improved coatings’ capacity to optimize the electron transport channel, the electric field distribution, the wettability of the interface, and other factors (Cui, Han, and Hu 2021). Moreover, by controlling Zn2+ deposition, a surface coating is designed to provide uniform Zn nucleation and a flat Zn deposition layer, which significantly enhances the Zn anode’s interface stability and cycle lifetime (A. Wang et al., 2020). Previous reported results state that a variety of materials, including carbon-based materials, metal materials, inorganic non-metals, polymers, and composite materials, have been employed as interfacial layers to produce high-performance Zn anodes (Li et al., 2018). As a widely used one-dimensional material, CNTs are lightweight and have excellent chemical properties. Yang et al. (Dong et al., 2020) developed self-supporting, extremely flexible, and conductive CNT/paper scaffolds to stabilize Zn metal anodes because unaltered Zn forms dendrites that lead to unfavorable interactions at the Zn anode/electrolyte interface, ultimately resulting in the failure of RAZIBs (Figure 8A). The porous scaffold’s skeleton mechanically controlled where Zn2+ would deposit on the Zn electrode’s surface, while the conductive CNT network kept the electric field uniform (Zuo et al., 2021). A more stable charge/discharge behavior was also shown by the built Zn@CNT symmetrical cells (Figure 8B). After cycling, the modified ZF didn’t exhibit any notable modifications (Figures 8C,D). Zn anodes were modified by Chen et al. (A. Wang et al., 2020) with nanofibrillar cellulose adhesives and C black coatings. The dendrite formation and side reactions on the anode are minimized by altering zinc foil with a C black coating and a nanofibrillating cellulose (NFC) binder, resulting in excellent interfacial stability between the anode and electrolyte. After 100 cycles, the ZF’s surface was covered in many dendrites (Figure 8F). Before and after cycling, the anode made of ZF modified with a C black coating and NFC binder (ZF@CB-NFC) maintained a consistent surface (Figure 8G). The redesigned anode-based cell exhibited improved cycle stability and CE (Figures 8H,I). The redesigned Zn anode-based symmetrical cell demonstrated improved cycle performance and a lower polarization voltage (Figures 8J,K). After circulation, metrical cells had the same appearance (Figure 8E).
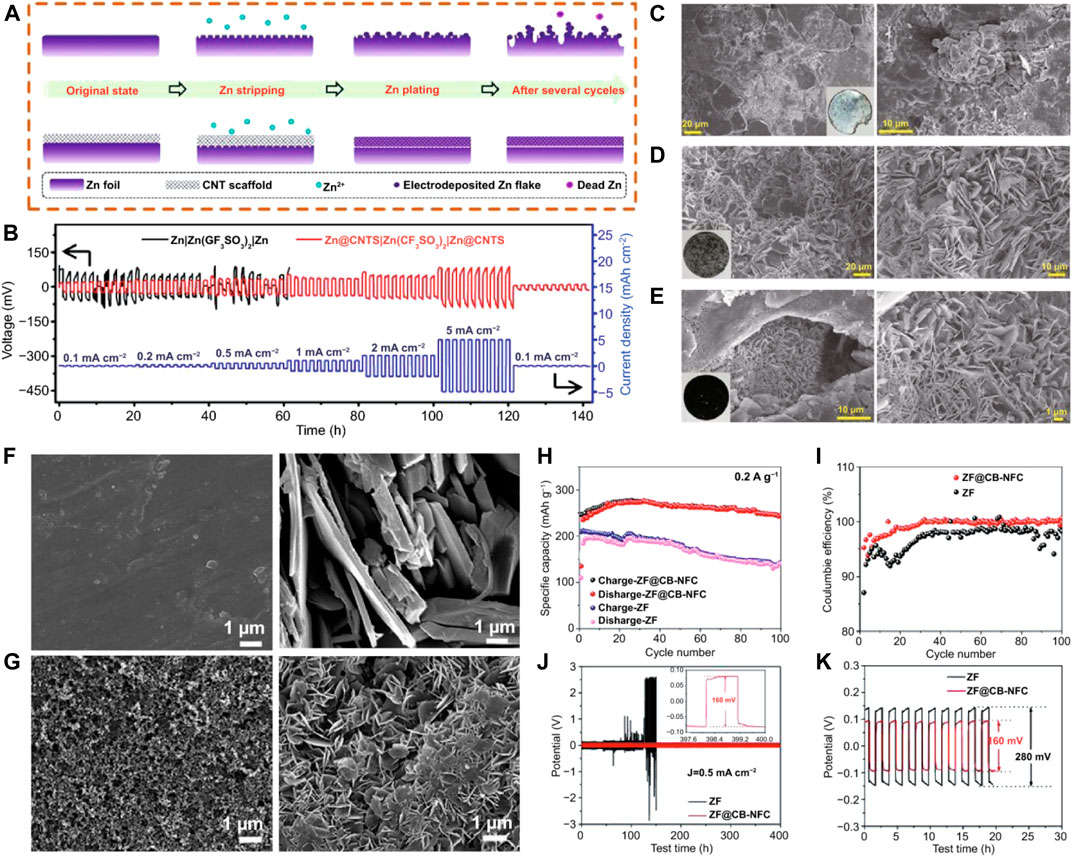
FIGURE 8. (A) Diagrams illustrating the stripping/plating behaviors of Zn anodes stabilized by CNT scaffolds and bare ZF anodes. (B) Rate performance over a 1-h period at varying current densities of 0.1–5 mA·cm−2. (C) Symmetrical Zn||Zn cells’ Zn electrodes as seen in a SEM picture. (D) SEM picture of symmetrical Zn@CNTs cells with Zn electrodes. (E) SEM picture of symmetrical Zn@CNT cells with CNT scaffolds (after cycling tests). SEM pictures of a ZF anode and a ZF@CB-NFC anode are shown in (F,G), respectively. (H) Cycling accomplishments at 0.2 A·g−1. (I) The relationship between Coulombic efficiency and cycle number. (J) Galvanostatic charge/discharge (GCD) curves for Zn symmetrical cells with ZF and ZF@CB-NFC electrodes, 200 cycles at 0.5 mA·cm−2. (K) Zn symmetrical cells with ZF and ZF@CB-NFC electrodes: voltage profiles of the 1st–10th cycles at 0.5 mA cm−2. Creative Commons Attribution 4.0 International license was used to permit this reproduction (Li et al., 2022). Copyright (2021), Springer.
4.5.3 3D porous substrate
High current density, as was already established, is detrimental to zinc’s uniform deposition. The substrate’s specific surface area can be effectively increased by the 3D porous structure, which lowers the local current density (Zhang et al., 2021). As a result, the anode and electrolyte have a larger surface area in contact with each other and the current density is distributed uniformly. Additionally, it is crucial for controlling ion transfer, preventing dendritic development, and preserving the battery’s dimensional stability. As a result, there is less chance that dendrites will form (Bayaguud, Fu, and Zhu 2022). The inhibition of zinc dendrite and the homogeneous distribution of zinc are both made possible by the aforementioned benefits. Therefore, structural modification is a useful strategy for reducing dendritic development (Higashi et al., 2016). These recently discovered methods for preventing zinc dendrites are demonstrated, together with the accompanying electrochemical performance (Table 1).
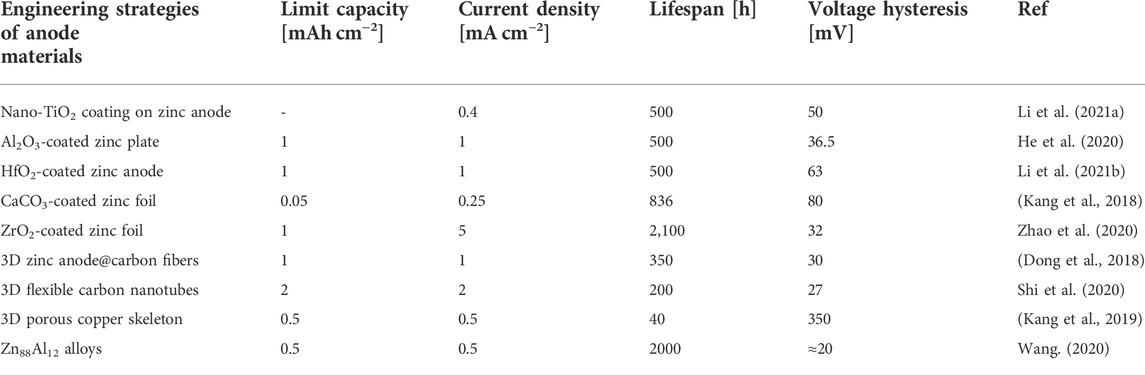
TABLE 1. An overview of recently reported dendrites free engineering strategies of zinc-based anode materials.
5 Summary and perspective
In conclusion, it is critical to recognize that the RZBs are the most viable candidate to construct a low-cost, secure system with adequate capacity, particularly for wearable devices. The main issues with the Zn anode, including as dendritic growth, hydrogen evolution, and passivation, are succinctly summarized in this paper. The most serious issue that might cause battery failure and safety problems is the dendrite problem. As a result of the previous discussion, there are four categories for dendritic suppression strategies: Electric field control involves designing 3D electrodes for low local current density and removing dendrite tips according to predetermined protocols; ion flux regulation involves introducing electrostatic interaction, adsorption, and changing the solvent shear structure; mechanical shield involves creating a strong interface by the use of designed protocols for the initial Zn anode. While each of those approaches can be used independently to improve performance, many approaches can be used simultaneously. An illustration of this is how a metal oxide surface coating will result in a consistent ion flux distribution and act as a physical barrier to stop dendrite formation. The increased electrochemical performance and extended anode lifespan are wonderful developments. Theoretical research and fundamental understanding, however, are still in the early stages and require more study. As a result, difficulties persist and a number of issues need to be taken into account.
Moreover, the problem of zinc dendrites to the ground is typically not resolved by using additions for electrolytes and electrodes, especially in alkaline mediums. In this situation, it is important to use efficient techniques to reduce or even eliminate zinc dendrites’ negative impacts, specifically by eliminating battery shorts as much as possible. Separators need to have a high level of mechanical stability to prevent impalement, as well as a high level of chemical stability to prevent degradation.
These elements will need to be taken into account in the future effort, and the following guidelines are strongly advised:
• A combination of theoretical direction and real-world requirements should be used to design the test conditions and standards. There is enough information to conclude that the current density is influenced by application situations and manufacturing capability, which both determine the mass loading range. The test environment should make an effort to mimic commercial requirements and machine capabilities.
• Future optimization should address several issues simultaneously using a variety of methodologies. For mass manufacturing to be feasible under the conditions of acceptable cost, the strategies should be properly integrated. For instance, the long-term stability is ensured by using affordable electrolyte additives to moderate the ion flux and a thin surface layer for physical shielding and corrosion prevention. The additives are placed in a hydrogel electrolyte with great mechanical strength and homogenous ion channels for dendrite suppression.
• Cutting-edge technologies, such as in-situ XRD for detecting the prevailing crystallographic orientation and in-situ optical/X-ray microscopy for morphological variation, enable the fundamental theory to monitor the interfacial process of the Zn anode in situ.
• Theoretical investigation showed that, different methods and strategies are used to stop uneven deposition. The creation of a stable Zn anode will be substantially accelerated by having a holistic awareness of how to recognize the crucial elements under various conditions.
• The most widely used techniques to completely and permanently inhibit the formation of zinc dendrites up until now have included designing a 3D porous structure with high zinc-based electrode surface areas, using acidic or neutral mediums, ionic liquids along with other novel mediums as electrolytes, and a combination of these techniques. The successful development of a dendrite-free zinc deposition procedure when using these techniques in zinc-based batteries would improve their performance and advance their business model.
Author contributions
All authors listed have made a substantial, direct, and intellectual contribution to the work and approved it for publication.
Acknowledgments
The Bahir Dar University, Bahir Dar Institute of Technology, Bahir Dar Energy Center, Ethiopia is gratefully acknowledged by the authors for its financial support.
Conflict of interest
The authors declare that the research was conducted in the absence of any commercial or financial relationships that could be construed as a potential conflict of interest.
Publisher’s note
All claims expressed in this article are solely those of the authors and do not necessarily represent those of their affiliated organizations, or those of the publisher, the editors and the reviewers. Any product that may be evaluated in this article, or claim that may be made by its manufacturer, is not guaranteed or endorsed by the publisher.
References
Abbasi, Ali, Hosseini, Soraya, Somwangthanaroj, Anongnat, AhmadMohamad, Azmin, and Kheawhom, Soorathep. 2019. “Hydroxide exchange separator membranes for zinc – air battery 1: 1–17. doi:10.3390/ijms20153678
Ayele, D. W., Habtu, N. G., Teshager, M. A., and Workineh, Z. G. (2021b). Recent progress in MnO2-based oxygen electrocatalysts for rechargeable zinc-air batteries. Mater. Today Sustain. 13, 100072. doi:10.1016/j.mtsust.2021.100072
Bayaguud, Aruuhan, Fu, Yanpeng, and Zhu, Changbao (2022). Interfacial parasitic reactions of zinc anodes in zinc ion batteries: Underestimated corrosion and hydrogen evolution reactions and their suppression strategies. J. Energy Chem. 64, 246–262. doi:10.1016/j.jechem.2021.04.016
Cao, Ziyi, Zhuang, Peiyuan, Zhang, Xiang, Ye, Mingxin, Shen, Jianfeng, and PulickelAjayan, M. (2020). Strategies for dendrite-free anode in aqueous rechargeable zinc ion batteries. Adv. Energy Mat. 10 (30), 2001599. doi:10.1002/aenm.202001599
Chen, Jie, Cheng, Zexiao, Liao, Yaqi, Yuan, Lixia, Li, Zhen, and Huang, Yunhui. 2022. “Selection of redox mediators for reactivating dead Li in lithium metal batteries.” Adv. Energy Mater. (n/a): 2201800. doi:10.1002/aenm.202201800
Chu, Yuzhu, Ren, Lingxiao, Hu, Zhenglin, Huang, Chengde, and Luo, Jiayan (2022). An in-depth understanding of improvement strategies and corresponding characterizations towards Zn anode in aqueous Zn-ions batteries. Green Energy Environ., 218–245. doi:10.1016/j.gee.2022.04.008
Cui, Bing-Feng, Han, Xiao-Peng, and Hu, Wen-Bin (2021). Micronanostructured design of dendrite‐free zinc anodes and their applications in aqueous zinc‐based rechargeable batteries. Small Struct. 2 (6), 2000128. doi:10.1002/sstr.202000128
Dong, Liubing, Wu, Yang, Wang, Yang, Tian, Hao, Huang, Yongfeng, Wang, Xianli, et al. (2020). Flexible and conductive scaffold-stabilized zinc metal anodes for ultralong-life zinc-ion batteries and zinc-ion hybrid capacitors. Chem. Eng. J. 384, 123355. doi:10.1016/j.cej.2019.123355
Dong, Wei, Shi, Ji Lei, Wang, Tai Shan, Yin, Ya Xia, Wang, Chun Ru, and Guo, Yu Guo (2018). 3D Zinc@carbon fiber composite framework anode for aqueous Zn-MnO2 batteries. RSC Adv. 8 (34), 19157–19163. doi:10.1039/c8ra03226b
Fu, Jing, Zhang, Jing, Song, Xueping, Zarrin, Hadis, Tian, Xiaofei, Qiao, Jinli, et al. (2016). A flexible solid-state electrolyte for wide-scale integration of rechargeable zinc-air batteries. Energy Environ. Sci. 9 (2), 663–670. doi:10.1039/c5ee03404c
Goh, F. W. Thomas, Liu, Zhaolin, Ge, Xiaoming, Yun, Zong, Du, Guojun, and Andy Hor, T. S. (2013). Ag nanoparticle-modified MnO2 nanorods catalyst for use as an air electrode in zinc-air battery. Electrochimica Acta 114, 598–604. doi:10.1016/j.electacta.2013.10.116
Guo, Leibin, Guo, Hui, Huang, Haili, Tao, Shuo, and Cheng, Yuanhui (2020). Inhibition of zinc dendrites in zinc-based flow batteries. Front. Chem. 8, 557–558. doi:10.3389/fchem.2020.00557
HabtuGabbiye, Nigus, Worku, Ababay Ketema, Ayele, Delele Worku, Teshager, Minbale Admas, and Workineh, Zerihun Getahun (2022). Facile preparation and electrochemical investigations of copper-ion doped α-MnO2 nanoparticles. Lect. Notes Inst. Comput. Sci. Social-Informatics Telecommun. Eng. 412, 543. doi:10.1007/978-3-030-93712-6_36
Han, Chao, Li, Weijie, Liu, Hua Kun, Dou, Shixue, and Wang, Jiazhao (2020). Principals and strategies for constructing a highly reversible zinc metal anode in aqueous batteries. Nano Energy 74, 104880. doi:10.1016/j.nanoen.2020.104880
He, Huibing, Tong, Huan, Song, Xueyang, Song, Xiping, and Liu, Jian (2020). Highly stable Zn metal anodes enabled by atomic layer deposited Al2O3 coating for aqueous zinc-ion batteries. J. Mat. Chem. A Mat. 8 (16), 7836–7846. doi:10.1039/d0ta00748j
Higashi, Shougo, Lee, Seok Woo, JangLee, Soo, Takechi, Kensuke, and Cui, Yi (2016). Avoiding short circuits from zinc metal dendrites in anode by backside-plating configuration. Nat. Commun. 7, 11801–11806. doi:10.1038/ncomms11801
Hosseini, Soraya, Lao-atiman, Woranunt, Han, Siow Jing, Arpornwichanop, Amornchai, Yonezawa, Tetsu, and Kheawhom, Soorathep (2018). Discharge performance of zinc-air flow batteries under the effects of sodium dodecyl sulfate and pluronic F-127. Sci. Rep. 8 (1), 14909–14913. doi:10.1038/s41598-018-32806-3
Huy, Vo Pham Hoang, Hieu, Luong Trung, and Hur, Jaehyun (2021). Zn metal anodes for Zn-ion batteries in mild aqueous electrolytes: Challenges and strategies. Nanomaterials 11 (10), 2746. doi:10.3390/nano11102746
Jia, Hao, Wang, Ziqi, Tawiah, Benjamin, Wang, Yidi, Chan, Cheuk Ying, Fei, Bin, et al. (2020). Recent advances in zinc anodes for high-performance aqueous Zn-ion batteries. Nano Energy 70, 104523. doi:10.1016/j.nanoen.2020.104523
Kang, Litao, Cui, Mangwei, Jiang, Fuyi, Gao, Yanfeng, Luo, Hongjie, Liu, Jianjun, et al. (2018). Nanoporous CaCO3 coatings enabled uniform Zn stripping/plating for long-life zinc rechargeable aqueous batteries. Adv. Energy Mat. 8 (25), 1801090–1801098. doi:10.1002/aenm.201801090
Kang, Zhuang, Wu, Changle, Dong, Liubing, Liu, Wenbao, Mou, Jian, Zhang, Jingwen, et al. (2019). 3D porous copper skeleton supported zinc anode toward high capacity and long cycle life zinc ion batteries. ACS Sustain. Chem. Eng. 7 (3), 3364–3371. doi:10.1021/acssuschemeng.8b05568
Khamsanga, Sonti, Pornprasertsuk, Rojana, Yonezawa, Tetsu, AhmadMohamad, Azmin, and Kheawhom, Soorathep (2019). δ-Mno2 nanoflower/graphite cathode for rechargeable aqueous zinc ion batteries. Sci. Rep. 9 (1), 8441–8449. doi:10.1038/s41598-019-44915-8
Lee, Dong Un, Xu, Pan, Cano, Zachary P., Gyu Park, Moon, and Chen, Zhongweii (2016). Recent progress and perspectives on Bi-functional oxygen electrocatalysts for advanced rechargeable metal--air batteries. J. Mat. Chem. A Mat. 4 (19), 7107–7134. doi:10.1039/c6ta00173d
Li, Bin, Xue, Jing, Han, Chao, Liu, Na, Ma, Kaixuan, Zhang, Ruochen, et al. (2021a). A hafnium oxide-coated dendrite-free zinc anode for rechargeable aqueous zinc-ion batteries. J. Colloid Interface Sci. 599, 467–475. doi:10.1016/j.jcis.2021.04.113
Li, Bin, Xue, Jing, Lv, Xin, Zhang, Ruochen, Ma, Kaixuan, Wu, Xianwen, et al. (2021b). A facile coating strategy for high stability aqueous zinc ion batteries: Porous rutile nano-TiO2 coating on zinc anode. Surf. Coatings Technol. 421 , 127367. doi:10.1016/j.surfcoat.2021.127367
Li, Bin, Zhang, Xiaotan, Wang, Tingting, He, Zhangxing, Lu, Bingan, Liang, Shuquan, et al. (2022). Interfacial engineering strategy for high-performance Zn metal anodes. Nano-Micro Lett. 14. doi:10.1007/s40820-021-00764-7
Li, Canpeng, Xie, Xuesong, Liang, Shuquan, and Jiang, Zhou (2020b). Issues and future perspective on zinc metal anode for rechargeable aqueous zinc-ion batteries. Energy Environ. Mat. 3 (2), 146–159. doi:10.1002/eem2.12067
Li, G., Mezaal, M. A., Zhang, R., Zhang, K., and Lei, L. (2016). Electrochemical performance of MnO2-based air cathodes for zinc-air batteries. Fuel Cells 16 (3), 395–400. doi:10.1002/fuce.201500077
Li, Qing, Zhao, Yuwei, Mo, Funian, Wang, Donghong, Qi, Yang, Huang, Zhaodong, et al. (2020a). Dendrites issues and advances in Zn anode for aqueous rechargeable Zn‐based batteries. EcoMat 2 (3), 1–14. doi:10.1002/eom2.12035
Li, Wei, Wang, Kangli, Zhou, Min, Zhan, Houchao, Cheng, Shijie, and Jiang, Kai (2018). Advanced low-cost, high-voltage, long-life aqueous hybrid sodium/zinc batteries enabled by a dendrite-free zinc anode and concentrated electrolyte. ACS Appl. Mat. Interfaces 10 (26), 22059–22066. doi:10.1021/acsami.8b04085
Li, Yanguang, Gong, Ming, Liang, Yongye, Ju, Feng, Kim, Ji-Eun, Wang, Hailiang, et al. (2013). Advanced zinc-air batteries based on high-performance hybrid electrocatalysts. Nat. Commun. 4 (1), 1805. doi:10.1038/ncomms2812
Ling, Wei, Mo, Funian, Wang, Jiaqi, Liu, Qingjiang, Liu, Yao, Yang, Qixin, et al. (2021). Self-healable hydrogel electrolyte for dendrite-free and self-healable zinc-based aqueous batteries. Mater. Today Phys. 20, 100458. doi:10.1016/j.mtphys.2021.100458
Liu, Tingting, Cheng, Xing, Yu, Haoxiang, Zhu, Haojie, Peng, Na, Zheng, Runtian, et al. (2019a). An overview and future perspectives of aqueous rechargeable polyvalent ion batteries. Energy Storage Mater. 18, 68–91. doi:10.1016/j.ensm.2018.09.027
Liu, Xiaorui, Yuan, Yifei, Liu, Jie, Liu, Bin, Chen, Xu, Jia, Ding, et al. (2019b). Utilizing solar energy to improve the oxygen evolution reaction kinetics in zinc–air battery. Nat. Commun. 10 (1), 4767. doi:10.1038/s41467-019-12627-2
Lu, Wenjing, Xie, Congxin, Zhang, Huamin, and Li, Xianfeng (2018). Inhibition of zinc dendrite growth in zinc-based batteries. ChemSusChem 11 (23), 3996–4006. doi:10.1002/cssc.201801657
Mainar, Aroa R., Blázquez, J. Alberto, and Urdampilleta, Idoia (2017). A brief overview of secondary zinc anode development : The key of improving zinc ‐ based energy storage systems. Int. J. Energy Res. 42, 903–918. doi:10.1002/er.3822
Mo, Funian, Ning, He, Chen, Lina, Li, Mengrui, Yu, Suzhu, Zhang, Jiaolong, et al. (2022). Strategies for stabilization of Zn anodes for aqueous Zn-based batteries: A mini review. Front. Chem. 9, 822624–822629. doi:10.3389/fchem.2021.822624
Pan, Huilin, Shao, Yuyan, Yan, Pengfei, Cheng, Yingwen, Han, Kee Sung, Nie, Zimin, et al. (2016). Storage from conversion reactions” 1, 1. doi:10.1038/NENERGY.2016.39
Pan, Jing, YangXu, Yang, Yang, Huan, Dong, Zehua, Liu, Hongfang, and Xia, Bao Yu (2018). Advanced architectures and relatives of air electrodes in Zn–air batteries. Adv. Sci. (Weinh). 5 (4), 1700691. doi:10.1002/advs.201700691
Qin, Jieqiong, Shi, Haodong, Huang, Kai, Lu, Pengfei, Wen, Pengchao, Xing, Feifei, et al. (2021). Achieving stable Na metal cycling via polydopamine/multilayer graphene coating of a polypropylene separator. Nat. Commun. 12 (1), 5786. doi:10.1038/s41467-021-26032-1
Shi, Minjie, Xiao, Peng, Cheng, Yang, Sheng, Yi, Wang, Bei, Jiang, Jintian, et al. (2020). Scalable gas-phase synthesis of 3D microflowers confining MnO2 nanowires for highly-durable aqueous zinc-ion batteries. J. Power Sources 463, 228209. doi:10.1016/j.jpowsour.2020.228209
Shin, Jaeho, Lee, Jimin, Park, Youngbin, and JangChoi, Wook (2020). Aqueous zinc ion batteries: Focus on zinc metal anodes. Chem. Sci. 11 (8), 2028–2044. doi:10.1039/d0sc00022a
Sumboja, Afriyanti, Ge, Xiaoming, Goh, F. W. Thomas, Li, Bing, Geng, Dongsheng, Andy Hor, T. S., et al. (2015). Manganese oxide catalyst grown on carbon paper as an air cathode for high-performance rechargeable zinc-air batteries. ChemPlusChem 80 (8), 1341–1346. doi:10.1002/cplu.201500183
Tian, Huajun, Zhao, Li, Feng, Guangxia, Yang, Zhenzhong, Fox, David, Wang, Maoyu, et al. (2021). Stable, high-performance, dendrite-free, seawater-based aqueous batteries. Nat. Commun. 12 (1), 1–12. doi:10.1038/s41467-020-20334-6
Wang, Anran, Zhou, Weijun, Huang, Aixiang, Chen, Minfeng, Chen, Jizhang, Tian, Qinghua, et al. (2020a). Modifying the Zn anode with carbon black coating and nanofibrillated cellulose binder: A strategy to realize dendrite-free Zn-MnO2 batteries. J. Colloid Interface Sci. 577, 256–264. doi:10.1016/j.jcis.2020.05.102
Wang, Fei, Borodin, Oleg, Gao, Tao, Fan, Xiulin, Sun, Wei, Han, F., et al. (2018). Fudong han, antonio faraone, joseph A. Dura, kang xu, and chunsheng WangHighly reversible zinc metal anode for aqueous batteries. Nat. Mat. 17 (6), 543–549. doi:10.1038/s41563-018-0063-z
Wang, Jiawei, Yang, Yan, Zhang, Yuxian, Li, Yanmei, Sun, Rong, Wang, Zhongchang, et al. (2021). Strategies towards the challenges of zinc metal anode in rechargeable aqueous zinc ion batteries. Energy Storage Mater. 35, 19–46. doi:10.1016/j.ensm.2020.10.027
Wang, Keliang, Pei, Pucheng, Ma, Ze, Chen, Huicui, Xu, Huachi, Chen, Dongfang, et al. (2015). Dendrite growth in the recharging process of zinc-air batteries. J. Mat. Chem. A Mat. 3, 22648–22655. doi:10.1039/c5ta06366c
Wang, Keliang (2020). Solutions for dendrite growth of electrodeposited zinc. ACS Omega 5 (18), 10225–10227. doi:10.1021/acsomega.0c01485
Wang, Lulu, Huang, Kuo Wei, Chen, Jitao, and Zheng, Junrong (2019). Ultralong cycle stability of aqueous zinc-ion batteries with zinc vanadium oxide cathodes. Sci. Adv. 5 (10), eaax4279–11. doi:10.1126/sciadv.aax4279
Wang, Mingming, Meng, Yahan, Li, Ke, Ahmad, Touqeer, Chen, Na, Xu, Yan, et al. (2022). Toward dendrite-free and anti-corrosion Zn anodes by regulating a bismuth-based energizer. eScience. doi:10.1016/j.esci.2022.04.003
Wang, Sheng Bo, Ran, Qing, Yao, Rui Qi, Shi, Hang, Wen, Zi, Zhao, Ming, et al. (2020b). Lamella-nanostructured eutectic zinc–aluminum alloys as reversible and dendrite-free anodes for aqueous rechargeable batteries. Nat. Commun. 11 (1), 1634–1639. doi:10.1038/s41467-020-15478-4
Worku, A. K., Ayele, D. W., and Habtu, N. G. 2021a. “Recent advances and future perspectives in engineering of bifunctional electrocatalysts for rechargeable zinc–air batteries.” Mater. Today Adv. 9: 100116. doi:10.1016/j.mtadv.2020.100116
Worku, Ababay Ketema, Ayele, Delele Worku, Habtu, Nigus Gabbiye, Admasu, Bimrew Tamrat, Alemayehu, Getu, and Taye, Biniyam Zemene (2022). “Energy storage technologies; recent advances, challenges, and prospectives,” in Planning of hybrid renewable energy systems, electric vehicles and microgrid: Modeling, control and OptimizationAashish kumar bohre, pradyumn chaturvedi, mohan lal kolhe, and sri niwas singh, 125–50 (Singapore: Springer Nature Singapore). doi:10.1007/978-981-19-0979-5_7
Worku, Ababay Ketema, Ayele, Delele Worku, and Habtu, Nigus Gabbiye (2021b). Influence of nickel doping on MnO2 nanoflowers as electrocatalyst for oxygen reduction reaction. SN Appl. Sci. 3 (9), 764. doi:10.1007/s42452-021-04746-7
Worku, Ababay Ketema, Ayele, Delele Worku, Habtu, Nigus Gabbiye, Teshager, Minbale Admas, and Workineh, Zerihun Getahun (2021c). Enhancing oxygen reduction reaction activity of ε-MnO2 nanoparticles via iron doping. J. Phys. Chem. Solids 157, 110207. doi:10.1016/j.jpcs.2021.110207
Worku, Ababay Ketema, Ayele, Delele Worku, Habtu, Nigus Gabbiye, and Yemata, Temesgen Atnafu. 2021d. “Engineering Co3O4/MnO2 nanocomposite materials for oxygen reduction electrocatalysis.” Heliyon 7 (9): e08076. doi:10.1016/j.heliyon.2021.e08076
Xie, Chunlin, Li, Yihu, Wang, Qi, Sun, Dan, Tang, Yougen, and Wang, Haiyan (2020). Issues and solutions toward zinc anode in aqueous zinc-ion batteries: A mini review. Carbon Energy 2 (4), 540–560. doi:10.1002/cey2.67
Yang, Jingjing, Zhao, Ran, Wang, Yahui, Bai, Ying, and Chuan Wu., n. d. Regulating uniform Zn deposition via hybrid artificial layer for stable Aqueous Zn-ion batteries” 2: 1–20.
Yang, Qi, Li, Qing, Liu, Zhuoxin, Wang, Donghong, Guo, Ying, Li, Xinliang, et al. (2020). Dendrites in Zn-based batteries. Adv. Mat. 32 (48), 2004477. doi:10.1002/adma.202001854
Yu, Yanxia, Xu, Wei, Liu, Xiaoqing, and Lu, Xihong (2020). Challenges and strategies for constructing highly reversible zinc anodes in aqueous zinc-ion batteries: Recent progress and future perspectives. Adv. Sustain. Syst. 4 (9), 2000082. doi:10.1002/adsu.202000082
Zeng, Xiaohui, Junnan, Hao, Wang, Zhijie, Mao, Jianfeng, and Guo, Zaiping (2019). Recent progress and perspectives on aqueous Zn-based rechargeable batteries with mild aqueous electrolytes. Energy Storage Mater. 20, 410–437. doi:10.1016/j.ensm.2019.04.022
Zhang, Guanhua, Zhang, Xianan, Liu, Huaizhi, Li, Jinhao, Chen, Yiqin, and Duan, Huigao (2021). 3D-Printed multi-channel metal lattices enabling localized electric-field redistribution for dendrite-free aqueous Zn ion batteries. Adv. Energy Mat. 11 (19), 2003927. doi:10.1002/aenm.202003927
Zhang, Jingsong, Cunningham, Jessica J., Brown, Joel S., and Gatenby, Robert A. (2017). Integrating evolutionary dynamics into treatment of metastatic castrate-resistant prostate cancer. Nat. Commun. 8 (1), 1816–1819. doi:10.1038/s41467-017-01968-5
Zhang, Ning, Cheng, Fangyi, Liu, Yongchang, Zhao, Qing, Lei, Kaixiang, Chen, Chengcheng, et al. (2016). Cation-deficient spinel ZnMn2O4 cathode in Zn(CF3SO3)2 electrolyte for rechargeable aqueous Zn-ion battery. J. Am. Chem. Soc. 138 (39), 12894–12901. doi:10.1021/jacs.6b05958
Zhang, Qi, Luan, Jingyi, Tang, Yougen, Ji, Xiaobo, and Wang, Haiyan (2020). Interfacial design of dendrite-free zinc anodes for aqueous zinc-ion batteries. Angew. Chem. Int. Ed. 59 (32), 13180–13191. doi:10.1002/anie.202000162
Zhang, Yuejuan, Bi, Songshan, Niu, Zhiqiang, Zhou, Weiya, and Xie, Sishen (2022). Design of Zn anode protection materials for mild aqueous Zn-ion batteries. Energy Mat. 2 (2), 200012. doi:10.20517/energymater.2022.08
Zhao, Weiyi, Liu, Ziqiang, Zhong, Chenglin, Shen, Zihan, Jin, Xin, and Zhang, Huigang (2020). ZIF-derived ZnO/Sb composite scaffolded on carbon framework for Ni-Zn batteries. J. Colloid Interface Sci. 579, 823–831. doi:10.1016/j.jcis.2020.06.120
Zhong, Yuwei, Liu, Bin, Zhao, Zequan, Shen, Yuanhao, Liu, Xiaorui, and Cheng, Zhong (2021). Influencing factors of performance degradation of zinc–air batteries exposed to air. Energies 14 (9), 2607. doi:10.3390/en14092607
Zhu, Qiao Nan, Wang, Zhen Ya, JiaWang, Wei, Liu, Xiao Yu, Yang, Dan, Cheng, Li Wei, et al. (2021). Challenges and strategies for ultrafast aqueous zinc-ion batteries. Rare Met. 40 (2), 309–328. doi:10.1007/s12598-020-01588-x
Keywords: rechargeable battery, Zn dendrites, Zinc-based batteries, dendritic morphology, Zinc anode, energy storage and conversion
Citation: Worku AK (2022) Engineering techniques to dendrite free Zinc-based rechargeable batteries. Front. Chem. 10:1018461. doi: 10.3389/fchem.2022.1018461
Received: 13 August 2022; Accepted: 12 September 2022;
Published: 29 September 2022.
Edited by:
Keliang Wang, Beijing Institute of Technology, ChinaCopyright © 2022 Worku. This is an open-access article distributed under the terms of the Creative Commons Attribution License (CC BY). The use, distribution or reproduction in other forums is permitted, provided the original author(s) and the copyright owner(s) are credited and that the original publication in this journal is cited, in accordance with accepted academic practice. No use, distribution or reproduction is permitted which does not comply with these terms.
*Correspondence: Ababay Ketema Worku, d29ya3VrZXRlbWE5MUBnbWFpbC5jb20=