- 1School of Material Science & Engineering, Beijing Institute of Technology, Beijing, China
- 2School of Mechatronical Engineering, Beijing Institute of Technology, Beijing, China
As an important component of energetic materials, high-energy oxidant is one of the key materials to improve their energy. The oxidizability of oxidant directly determines the intensity of combustion or explosion reaction. It is generally believed that when the nature of reductant is certain, the stronger the oxidizability, the more intense the reaction. Dioxygenyl cation (O2+) and pentazenium cation (N5+) are two kinds of super oxidizing ions, which oxidizability are comparable to that of fluorine. A series of high energetic ionic salts with O2+, N5+ and various anions as active components are designed, and the results show that: 1) Most ionic salts have appropriate thermodynamic stability, high density (up to 2.201 g/cm3), high enthalpy of formation (up to 1863.234 kJ/mol) and excellent detonation properties (up to 10.83 km/s, 45.9 GPa); 2) The detonation velocity value of O2 (nitrotetrazole-N-oxides) and O2B(N3)4 exceed 10.0 km/s, and the detonation pressure exceed 45.0 GPa because of the O2+ salts have higher crystal density (g/cm3) and oxygen balance than that of N5+salts; 3) With a higher nitrogen content than O2+, the N5+ salts have higher enthalpy of formation, which exceed 330 kJ/mol than that of O2+ salts; 4) The linear spatial structure of N5+ leads the salts to reduce their density. Encouragingly, this study proves that these super oxidizing ions have the potential to become high-energy oxidants, which could be a theoretical reference for the design of new high energetic materials.
1 Introduction
An oxidant is a reactant that oxidizes or removes electrons from other reactants during a redox reaction, which is widely used in aerospace propellants, explosives, and pyrotechnics (Connelly and Geiger, 1996) . The oxidants, which directly participate in combustion or explosion reactions, is one of the indispensable components for such chemical reactions. For example, dinitramide salts (Venkatachalam et al., 2004) and perchlorates (Zeng and Bernstein, 2019) are added to energetic formulas to increase the oxygen balance and density of the whole system.
In energetic materials, the functions of oxidants are: 1) Speeding up burning more intensely; 2) Causing materials that are normally not readily combustible in air to burn more readily; 3) Causing combustible materials to burn spontaneously without a source of ignition. It is generally agreed that the oxidant with strong oxidizability can significantly improve the energetic properties of mixed explosive when the nature of reductant is certain.
However, traditional oxidants (such as ammonium perchlorate, hydrazine perchlorate, nitroyl perchlorate, hydroxylamine perchlorate, etc.) often contain halogen elements, which are easy to cause harm to human body and the environment; The energy level of existing high-energy oxidants such as 1,3,5-triamino-2,4,6-trinitrobenzene (TATB), 1,1-diamino-2,2-dinitroethylene (FOX-7), dihydroxylammonium 5,5′-bistetrazole-1,1′-diolate (TKX-50), octahydro-1,3,5,7-tetranitro-1,3,5,7-tetrazocine (HMX), hexahydro-1,3,5-trinitro-1,3,5-triazine (RDX), and hexanitrohexaazaisowurtzitane (CL-20) has reached the limit of energetic oxidants. The design and development of energetic ionic salts with super oxidation and gas products are expected to promote the innovative development of traditional energetic materials. Excitingly, the O2+ and N5+ are two super oxidizing cation systems, and their oxidizing power are comparable to that of fluorine gas. These two cations consist only of oxygen and nitrogen elements, respectively, which ensures that their reaction products are likely to be nitrogen and various gaseous non-metallic oxides.
Dioxygenyl hexafluoroplatinate containing O2+ was first synthesized by Bartlett et al. (Bartlett and Lohmann, 1962) in 1962, which started the wave of research of dioxygenyl cation. Subsequently, various O2+ salts containing fluorine anions [such as O2+MF6- (M = P, As, Sb, Bi, Pt, Ru, Rh, Pd, or Au), O2+M2F11− (M = Sb, Bi, Nb, or Ta), Or O2+MF4- (M = B)] were successively characterized (Grill et al., 1970; Nikitin and Rosolovskii, 1971; Goetschel and Loos, 1972; McKee and Bartlett, 1973; Christe et al., 1974; Edwards et al., 1974; Gillespie and Schrobilgen, 1974; DiSalvo et al., 1975; Griffiths et al., 1975; Holloway and Schrobilgen, 1975; Rigny and Falconer, 1975). In 1973, Stein et al. (1973) used O2SbF6 to remove xenon and radon from the atmosphere, and oxygen was released after the reaction. It directly proved that O2+ has strong oxidizability, so that it can directly oxidize the inert gas and release oxygen. In 1976, Falconer et al. (1976) synthesized O2+PdF6−, that is, Pd (V) can be stabilized to hexafluorides by forming a complex salt with dioxygenyl cation. In 1976, Christie et al. (1976) synthesized and characterized another new O2+ salt: O2+GeF5−. This substance was prepared by UV photolysis of GeF4-F2-O2 mixture in quartz at −78°C. In 1991, Fisher et al. (1991) studied the reaction between O2+ and CF4/C2F6, and found that FCO+ and F2CO+ would be generated after the reaction, which shows the super oxidizability of O2+. However, the above studies focus on the stability and oxidizability of dioxygenyl ions, and did not involve too much research on energetic materials. Holfter studied the reaction between O2+BF4− and activated sodium azide in the presence of metallic aluminum in 1997 (Holfter et al., 1997). The reaction produces NaBF4, N2 and Al2O3, and releases 434 kcal/mol of heat, which fully shows that O2+BF4− is a high energy density material.
In addition, the all-nitrogen cation N5+ is another attractive super oxidizing ion compared to the O2+. Christe et al. (1999) first reported the synthesis and characterization of N5+AsF6− in 1999, which has a chain structure and could be a high energy density material. This study shows that N5+AsF6− is a white solid slightly soluble in anhydrous HF, which is relatively stable at 22
Based on the previous researches, the super oxidation and potential energetic properties of N5+ and O2+ greatly encourage us to further study the application potential of these substances in the field of energetic materials. In this paper, N5+ and O2+ are used as the cationic components of energetic ionic salts, while the polyazole rings, BF4−, B(N3)4-, NO3−, C(NO2)3-, and N3−are used as anions to design two new types of energetic ionic salts (see Scheme 1). By means of quantum chemical calculation, we studied the physical properties (density, enthalpy of formation), stability and detonation properties of these two energetic ionic salts. We hope that this research work can deepen people’s understanding of super oxidizing ions, and provide some reference and theoretical support for the development of new energetic oxidants.
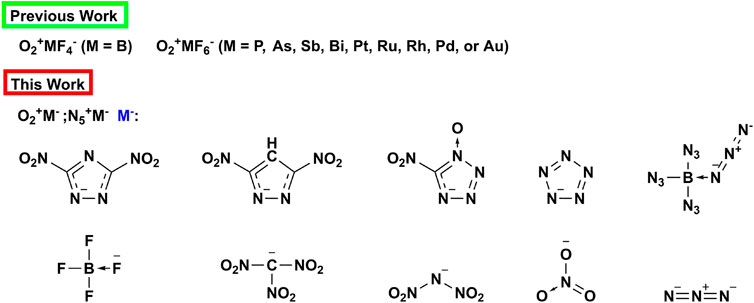
SCHEME 1. Fluorinated anions for stabilizing O2+ in previous works and energetic anions used in this paper to stabilize O2+ and N5+.
2 Calculation method
The geometries of each structure corresponding to the stationary point on the potential energy surface (PES) of the specie studied were fully optimized using density functional theory at the M06-2X/6-311+G (d, p) level with Gaussian 09 (Wong et al., 2002; Zhao and Truhlar, 2007; Frisch et al., 2016). Zero-point energies and thermal corrections to enthalpy (the correction factor is 0.97) and Gibbs free energies are computed at the same DFT level. Single-point electronic energies are afterward refined using PWPB95 (Goerigk and Grimme, 2011) functional in conjunction with a def2-QZVP (Weigend and Ahlrichs, 2005) by ORCA (Neese, 2011). The molecular van der Waals surface electrostatic potential distribution of anions and cations were obtained with Multiwfn (Lu and Chen, 2012a; b).
The crystal density (ρ) was estimated using the improved equation (Rice and Byrd, 2013) shown as follows Eq. 1.
where M is the molecular mass of the compound. Vm is the volume of the isolated gas molecule. The
The volume (V) of ionic compounds (MpXq) (Jenkins et al., 1999; Rice et al., 2007) is estimated using the sum of the respective volumes of cations and anions by Eq. 2.
where
As shown in Scheme 2, based on the Born-Haber energy cycle, the enthalpy of formation (Gao et al., 2007) of ionic compounds can be predicted by Eq. 3:
where
where
where
The detonation velocity and pressure were predicted by empirical Kamlet−Jacobs in EXPLO5 (v6.05) program (Sućeska, 2018) by Eqs 6, 7:
where D is the detonation velocity (km/s), P is the detonation pressure (GPa), N is the moles of detonation gases per gram explosive, M̅ is the average molecular weight of these gases, Q is the heat of detonation (cal/g), and ρ is the loaded density of explosives (g/cm3) and is replaced by the theoretical density here.
For ionic compounds (MpXq), the Gibbs free energy change of formation (
3 Results and discussion
3.1 Configuration
Figure 1 shows two types of energetic ionic salt compounds in this paper. For N5+ salts (A to J), all the anions are located inside the V-shape of the N5+. These spatial relative configurations are consistent with previous studies (Wang et al., 2011; Lian et al., 2012; Yu et al., 2015). The reason for this spatial distribution may be due to the electrostatic potential distribution on the surface of the N5+. Similarly, the structures of the O2+ salts are A1 to J1. The intrinsic cause of this spatial structure distribution remains the distribution of electrostatic potential on the surface of anions and cations. As can be seen from Figure 2, the high electrostatic potential portions of N5+ and O2+ are mainly concentrated in the V-shaped inner region of the N5+ (red region) and in the direction vertical to the bond axis of the O-O bond in O2+ (red region). This high positive electrostatic potential region tends to interact with the low negative electrostatic potential in the anion, thus affecting the molecular conformations of the ionic salts in Figure 1.
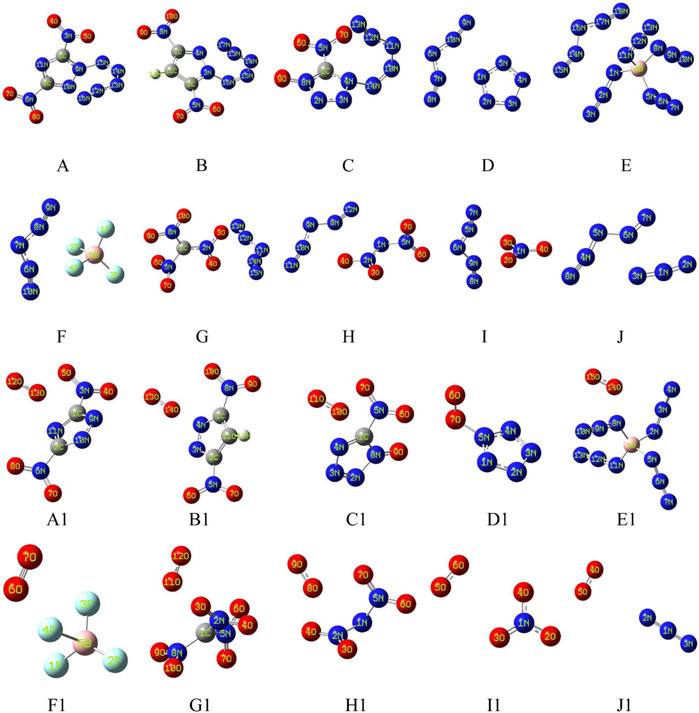
FIGURE 1. Theoretically designed energetic compounds containing N5+ (A–J) and O2+ (A1–J1) ions at M06-2X/6-311+G (d, p) theory.
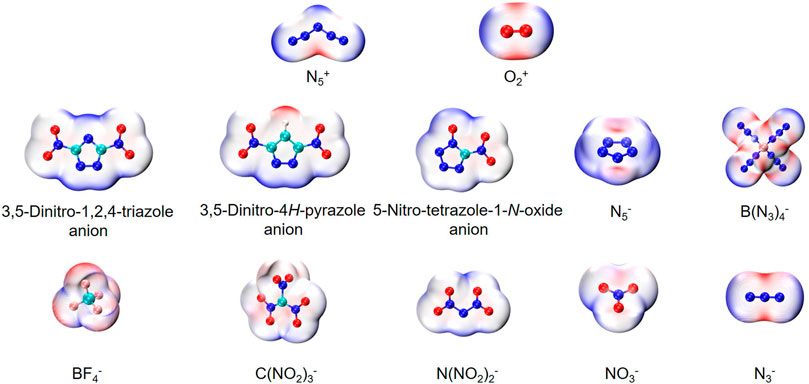
FIGURE 2. The molecular van der Waals surface electrostatic potential distribution of anions and cations. The red region represents the higher electrostatic potential in the molecular and blue region represents the lower electrostatic potential in it.
3.2 Crystal density
Crystal density is a critical consideration for energetic materials. In this section, the effects of different anions on the density of O2+ and N5+ salts are investigated separately. Table 1 lists the volumes, relevant parameters and densities of the individual ionic salt. It is easy to find that the densities of these two classes of ionic salts are designed to be between 1.490 and 2.263 g cm−3.
On the whole, the density of salts formed by anion and O2+ is higher than that of ionic salts formed by anion and N5+. Compared with O2+, the volume of N5+ with V-shaped structure is larger than that of O2+, which is not conducive to the formation of dense accumulation, resulting in the density of this kind of ionic salts are generally lower than these of O2+. Among all ionic salts, A, C, F, G, H for N5+ salts and A1 to I1 for O2+ salts with a density higher than 1.800 g cm−3, which indicate that these compounds maybe have high detonation velocity. The densities of most O2+ salts are higher than those of HMX (1.90 g cm−3) (Wang et al., 2018) and RDX (1.80 g cm−3) (Wang et al., 2018), while the densities of A1, C1, D1, F1, G1 and H1 are close to that of CL-20 (2.04 g cm−3) (Fischer et al., 2015). In addition, the densities of H and I calculated in literature are 1.88 and 1.81 g cm−3, respectively, which higher than those are predicted in this paper (1.806 g cm−3 for H and 1.700 g cm−3 for I). This difference may be caused by the different calculation methods and the fact that the corrected volume is not used in this paper.
In addition, the effect of different anions on the density of these two types of ionic salts is reflected in Figure 3, respectively. In Figure 3, the blue closed line wraps the red one, indicating that the density of the O2+ salts is greater than that of the N5+ salts. Among all ionic salts, the salts formed by BF4− anion have the highest density, reaching 1.944 g cm−3 (1.99 g cm−3 in the literature (Wang et al., 2011)) for N5+ salts and 2.263 g cm−3 for O2+ salts. However, salts formed from N3− have the lowest densities, 1.490 g cm−3 for N5+ salts and 1.610 g cm−3 for O2+ salts, respectively. The radar plot shows that the order of the effect of different anions on the ionic salts density are BF4− > C(NO2)3- > 3,5-Dinitro-1,2,4-triazole anion > 5-Nitro-tetrazole-1-N-oxide anion > N(NO2)2- > 3,5-Dinitro-4H-pyrazole anion > NO3− > B(N3)4- > N5− > N3− for N5+ salts and BF4− > C(NO2)3- > 3,5-Dinitro-1,2,4-triazole anion > N5− = N(NO2)2- > 5-Nitro-tetrazole-1-N-oxide anion >3,5-Dinitro-4H-pyrazole anion > NO3− > B(N3)4- > N3− for O2+ salt.
3.3 Enthalpy of formation
A high and positive enthalpy of formation is a typical feature of energetic compounds. In this section we study the enthalpy of formation of the designed ionic salts. Table 2 contains the formation enthalpies of each anion (
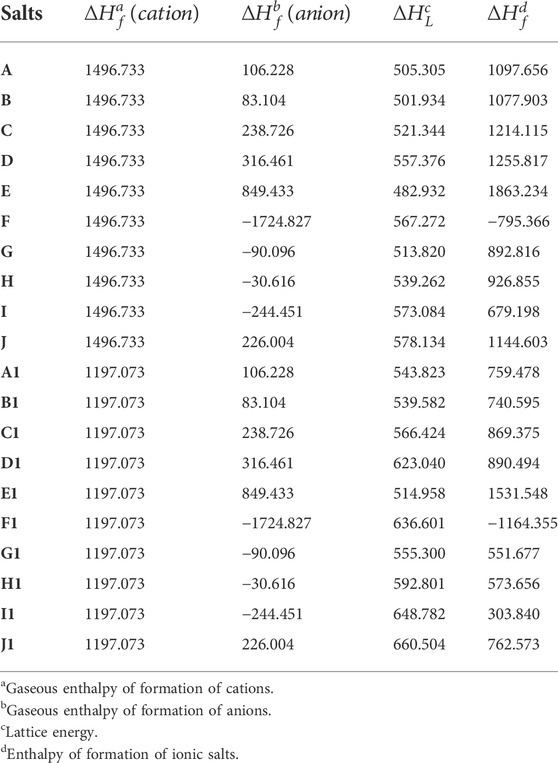
TABLE 2. The enthalpy of formation of O2+ and N5+, various anions and their corresponding salts (∆Hf) (A to J for N5+ salts and A1 to J1 for O2+ salts), as well as lattice energies (∆HL) of these salts. (unit, kJ/mol).
It is easy to find from Table 2 that the enthalpy of formation of salts formed by anion and N5+ is higher than that of ionic salt formed by anion and O2+. The O2+ and N5+ have very high enthalpy of formation (both >1000 kJ/mol), which maybe determine the ultra-high enthalpy of formation for these ionic salts. As deduced earlier, the enthalpy of formation of all the remaining ionic salts are positive except for F and F1. Among them, the highest enthalpy of formation is E (up to 1863.23 kJ/mol) and the lowest is I1 (up to 303.84 kJ/mol). Except for F, the enthalpy of formation of the remaining N5+ salts are all much higher than that of CL-20 (365.4 kJ/mol) (Wang et al., 2018). Although the enthalpy of formation of O2+ salts is not as high as that of N5+ salts, most of the salts also have a fairly high enthalpy of formation (1531.55 kJ/mol for E1).
In addition, the effect of different anions on the enthalpy of formation of these two types of ionic salts is reflected in Figure 4, respectively. In Figure 4, the red closed line wraps the blue one, indicating that the enthalpy of formation of the N5+ salts is greater than that of the O2+ salts. Among all ionic salts, the salts formed by B(N3)4- have the highest enthalpy of formation, reaching 1863.23 kJ/mol for N5+ salts and 1531.55 kJ/mol for O2+ salts, which implies that the B(N3)4- can significantly increase the enthalpy of formation of the compound. However, salts formed from BF4− anions have the lowest enthalpy of formation, −795.37 kJ/mol for N5+ salts and −1164.36 kJ/mol for O2+ salts, respectively. The introduction of O2+ and N5+ with ultra-high enthalpy of formation in compounds is an effective way to increase the enthalpy of formation of compounds. However, fluorine sharply reduces the enthalpy of formation of the compound, so it causes F (−795.37 kJ/mol) and F1 (−1164.355 kJ/mol) to have ultra-low enthalpies of formation. The radar plot shows that the order of the effect of different anions on enthalpy of formation of the ionic salts is B(N3)4- > N5− > 5-Nitro-tetrazole-1-N-oxide anion > N3− > 3,5-Dinitro-1,2,4-triazole anion > 3,5-Dinitro-4H-pyrazole anion > N(NO2)2- > C(NO2)3- > NO3− > BF4−, regardless of whether it is an O2+ salt or an N5+ salt.
3.4 Energetic properties
The explosive properties of energetic materials are to evaluate the energy performance of the characteristic parameters, mainly including the detonation velocity (D), detonation pressure (P), detonation heat (Q), etc. Table 3 contains the D, P, Q and specific impulse (Isp) for all designed ionic salts.
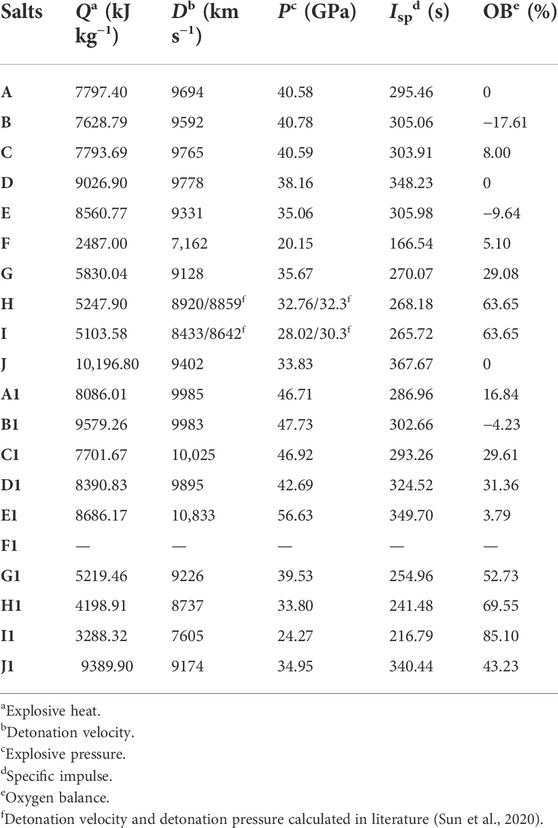
TABLE 3. Predicted explosive properties, specific impulse (Isp) and oxygen balance of N5+ and O2+ salts.
It is easy to find that most of the ionic salts have D in excess of 8,000 m/s. Among the N5+ salts, all except F (7,162 m/s), H (8,920 m/s) and I (8,433 m/s) have D exceeding 9000 m/s, owing to their high density. Similarly, among the O2+ salts, the D exceeded 9000 m/s for all salts except H1 (8,737 m/s), I1 (7,605 m/s) and F1. Excitingly, the D of C1 (10,025 m/s) and E1 (10,833 m/s) exceeded 10,000 m/s, and the D of A1 (9985 m/s), B1 (9983 m/s) and D1 (9895 m/s) were close to 10,000 m/s, which fully demonstrates the potential of O2+ as active ingredients of ultra-high energy oxidants. The introduction of O2+ into the system significantly increases the crystal density and oxygen balance, which fundamentally determines the high detonation velocity properties of this category of salts.
The P is another important index of energetic materials. Compared with the P of CL-20, the P of A1, B1, C1, and E1 are all higher than that of CL-20 in O2+ salts, reaching 46.71, 47.73, 46.92, 56.63 GPa, respectively. In the case of N5+ salts, although the highest p value is not as high as E1 in O2+ salts, the P of some N5+ salts (A, B, C) also reaches more than 40 GPa.
Oxygen balance is an important reference indicator for screening energetic materials. As can be seen in Table 3, the oxygen balance of the O2+ salts are higher than these of the N5+ salts for salts composed of the same anion, which directly indicates that the way to improve the oxygen balance is to introduce O2+ into the system. Energetic ionic salts can be used not only as energetic materials, but also as propellants. The value of Isp can be used to compare the performance of different rocket propellants. Among the N5+ salts, the Isp is higher than 260 s for all ionic salts except F (166.54 s). Five N5+ salts have Isp value exceeding 300 s, with the highest value of J reaching 367.67 s. In contrast, among the O2+ salts, there are four ionic salts with Isp exceeding 300 s, namely B1 (302.66 s), D1 (324.52 s), E1 (349.70 s), and J1 (340.44 s). The ultra-high Isp value further illustrate the promise of these super-oxidizing ionic salts to drive further development of conventional propellants.
In addition, Figure 5 shows the effect of different anions on the detonation velocity A, detonation pressure B, detonation heat C and specific impulse D. Based on the degree of overlap of the two curves (the blue and the red) in the radar plots, we can clearly find the effect of different anions on the detonation parameters of the ionic salts.
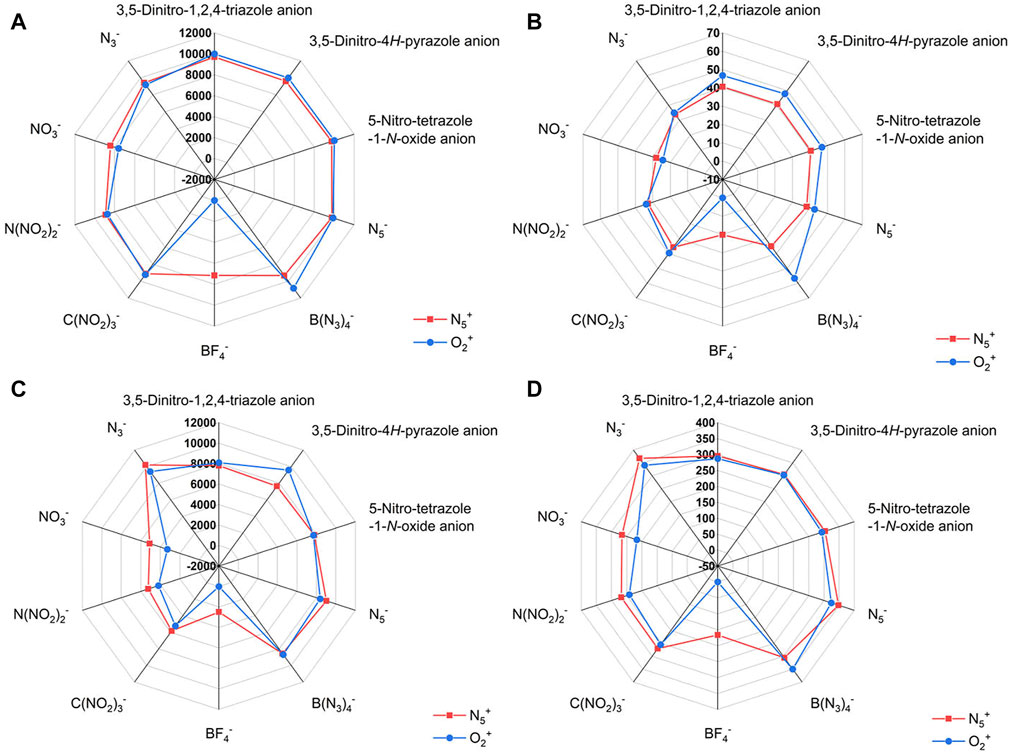
FIGURE 5. The radar plots of the effect of different anions on the detonation parameters of the N5+ and O2+ salts. The (A) is for D, (B) is for P, (C) is for Q and (D) is for Isp, respectively.
Among these ten anion structures, it is not possible to calculate the detonation parameters of the O2+ salts composed of BF4− by the Kamlet−Jacobs formula. The reason for this phenomenon may be due to the ultra-low enthalpy of formation of this ionic salts (−1164.355 kJ/mol for F1). As a comparison, the ionic salt formed by BF4− and N5+ has a calculated detonation parameter, which indicates that the N5+ enhances the energy of this system more than the O2+ (enthalpy of formation of F is −795.37 kJ/mol). It is obvious from the radar plot (Figures 5C,D) that the N3− has a greater influence on the heat of explosion and specific impulse than other anions. The effects of 3,5-Dinitro-1,2,4-triazole anion, 3,5-Dinitro-4H-pyrazole anion, 5-Nitro-tetrazole-1-N-oxide anion and N5− on the detonation velocity are similar in both N5+ and O2+ salts (Figure 5A). B(N3)4- has great influence on the detonation velocity, detonation pressure and specific impulse of O2+ salt (Figures 5A,B,D). Among the N5+ salts, the detonation performance of the ionic salt formed by the BF4− is lower than that of the other anions (Figures 5A–D). This illustrates that the element fluorine is not conducive to the detonation performance of a single-compound energetic material. In ionic salts consisting of C(NO2)3-, N(NO2)2- and NO3−, the detonation properties of the ionic salts gradually increase with the increase in the number of NO2 groups.
3.5 Impact sensitivity
Impact sensitivity (H50) is used to describe the degree of difficulty of explosion of energetic materials under impact stimulation, which indicates the stability of compounds to a certain extent. The higher the value of H50, the more stable the compound is to shock stimulation. Table 4 lists the positive variance (
3.6 Stability
The newly designed high-energy oxidizing ionic salts need to have not only excellent detonation performance, but also sufficient stability. As an allotrope of N5+, the N5− was successfully synthesized by researchers in 2017 (Xu et al., 2017; Zhang et al., 2017). It is believed that the N5− is stable at atmospheric pressure because of its ability to form coordination interactions with metal cations and to form hydrogen bonds with water molecules. Subsequently, a series of ionic salts formed by N5− and organic nitrogen-rich cations (Yang et al., 2018; Xu et al., 2019) were designed and synthesized, which further broadened the study of N5− ions.
Based on this stabilization mechanism, we hypothesize that the N5+ is also capable of stabilizing with certain nonmetallic anions through electrostatic interactions or other van der Waals interactions. Therefore, we first analyzed the various interactions (see Figure 6) existing within the ionic salts by interaction region indicator (IRI) (Lu and Chen, 2021) analyses, and then calculated the Gibbs free energies change of formation of the ionic salts as ways of which to illustrate the stability of the designed energetic ionic salts.
In Figure 6, for N5+ salts, the presence of dark blue portions of N9-N15 in A, N3-N6 in B and N4-N14 in C, respectively, can be found in the IRI isosurface map, implying that all these bonds are relatively strong covalent chemical bonds. In D, E, and H, the presence of dark blue regions at the space outside the ionic fragment, which implies the presence of strong interactions in these parts. However, there is no dark blue region in F, G and J, which is similar to that in D, E and H, suggesting that the binding degree of cation and anion in these three compounds is relatively weak. However, for O2+ salts in Supplementary Figure S1), except D1, there is no obvious covalent interaction between anions and cations in other salts. The results show that in F1, BF4− seems to dissociate, forming F− and BF3 groups, and there is a strong interaction between O2+ and F− in F1. In other ionic compounds, O2+ seems to form a strong van der Waals interaction with anions (green IRI isosurface rather than dark blue isosurface), and a strong interaction (blue IRI isosurface) appears to be formed between O2+ and N5+ in D1. In short, multiple interactions within ionic compounds contribute to the stability of their own structures.
In addition, Table 5 lists the Gibbs free energy change of the formation of each ionic salt. It is easy to find that except for a few ionic salts (D, E, and J in N5+ salts and D1, E1 in O2+ salts), the Gibbs free energy changes of the formation of other ionic salts are positive, indicating that these ionic salt structures can exist stably in thermodynamics. Figure 7 represents the effect of different anions on the Gibbs free energy of ionic salt generation. Relative to the other anions, the BF4− has the highest Gibbs free energy change of the formation of each ionic salt (345.36 kJ/mol for F and 452.44 kJ/mol for F1). In contrast, ionic salts containing N5− have the lowest Gibbs free energy change of the formation (-156.27 kJ/mol for D and-52.861 kJ/mol for D1). The negative Gibbs free energy change of the formation indicate that both D and D1 cannot exist stably, which is consistent with the conclusions of Christe’s study (Dixon et al., 2004). Also, our findings indicate that the free energy change of the ionic salt J formed from the N3− and N5+ is also negative, implying that this ionic salt also cannot exist stably, which is once again consistent with the conclusion of Christe’s study. However, the Gibbs free energy change of the formation of J1, an ionic salt formed from N3− and O2+, becomes positive, implying that this compound can be thermodynamically stable. Finally, the order of the influence of other anions on the Gibbs free energy change of ionic salt are: BF4− > 3,5-Dinitro-1,2,4-triazole anion >3,5-Dinitro-4H-pyrazole anion > C(NO2)3- > N(NO2)2- > NO3− > 5-Nitro-tetrazole-1-N-oxide anion > N3− > B(N3)4- > N5− for N5+ ionic salts or BF4− > 3,5-Dinitro-1,2,4-triazole anion >3,5-Dinitro-4H-pyrazole anion > NO3− > N(NO2)2- > C(NO2)3- > 5-Nitro-tetrazole-1-N-oxide anion > N3− > B(N3)4- > N5− for O2+ ionic salts.
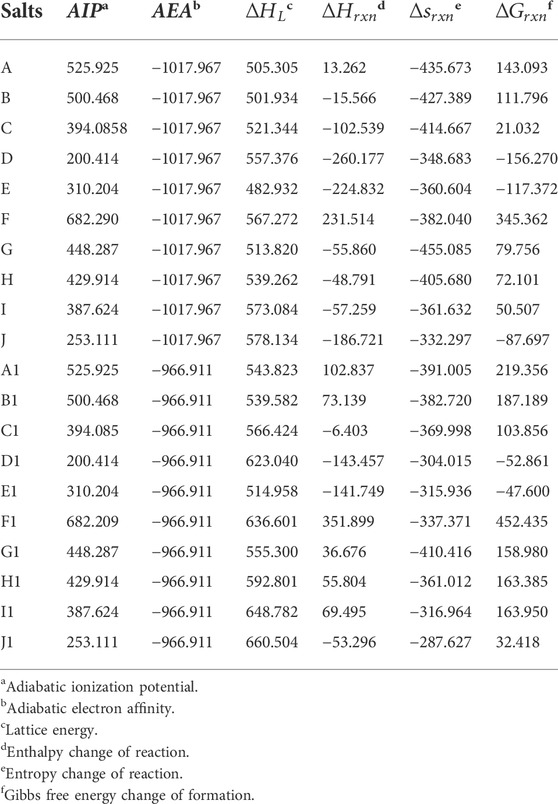
TABLE 5. Calculated adiabatic ionization potential (AIP), adiabatic electron affinity (AEA), lattice energy (
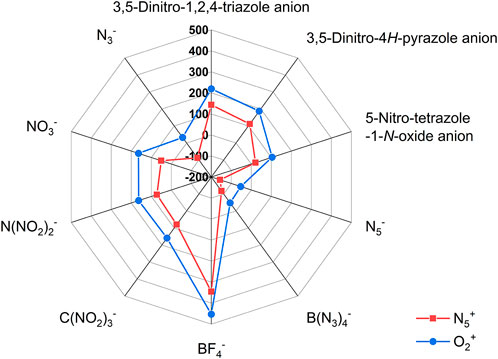
FIGURE 7. The radar plots of the effect of different anions on the Gibbs free energy change of the formation of the N5+ and O2+ salts.
4 Conclusion
The combination of high-energy oxidants N5+ and O2+ with high nitrogen organic anions is a new design idea proposed with the help of quantum chemical calculations. This idea can not only ensure the overall high energy level of ionic salt, but also consider the thermodynamic stability. Most ionic salts have good detonation velocity and pressure because of their high density and enthalpy of formation, suggesting these ionic salts have potential as candidates for high-energy oxidants. The introduction of O2+ into the system is an important means to improve the ionic salt oxygen balance. Considering the energy properties (enthalpy of formation, detonation velocity, detonation pressure, detonation heat, specific impulse) and stability (formation of Gibbs free energy) of these designed ionic salts, we believe that A, B, C, G, H for N5+ salts and A1, B1, C1, G1, H1 for O2+ salts are expected to be further studied and experimentally developed.
Data availability statement
The raw data supporting the conclusion of this article will be made available by the authors, without undue reservation.
Author contributions
XY: Investigation, data curation, visualization, theoretical calculation, writing-original draft. NL, YL, and SP: Writing-review and editing, funding acquisition, conceptualization.
Funding
This work was supported by the National Natural Science Foundation of China (22135003 and 21975023).
Conflict of interest
The authors declare that the research was conducted in the absence of any commercial or financial relationships that could be construed as a potential conflict of interest.
Publisher’s note
All claims expressed in this article are solely those of the authors and do not necessarily represent those of their affiliated organizations, or those of the publisher, the editors and the reviewers. Any product that may be evaluated in this article, or claim that may be made by its manufacturer, is not guaranteed or endorsed by the publisher.
Supplementary material
The Supplementary Material for this article can be found online at: https://www.frontiersin.org/articles/10.3389/fchem.2022.1005816/full#supplementary-material
References
Bartlett, N., and Lohmann, D. H. (1962). 1005. Fluorides of the noble metals. Part II. Dioxygenyl hexafluoroplatinate(V), O2+[PtF6]-. J. Chem. Soc., 5253. doi:10.1039/jr9620005253
Christe, K. O., Schack, C. J., Wilson, R. D., and Pilipovich, D. (1974). Reactions of the (CF3)2NO radical with strong oxidizers. J. Fluor. Chem. 4 (4), 423–431. doi:10.1016/S0022-1139(00)85292-6
Christe, K. O., Wilson, R. D., and Goldberg, I. B. (1976). Dioxygenyl pentafluorogermanate(IV), O2+GeF5-. Inorg. Chem. 15 (6), 1271–1274. doi:10.1021/ic50160a005
Christe, K. O., Wilson, W. W., Sheehy, J. A., and Boatz, J. A. (1999). N5+: A novel homoleptic polynitrogen ion as a high energy density material. Angew. Chem. Int. Ed. 38 (13-14), 2004–2009. doi:10.1002/(sici)1521-3773(19990712)38:13/14<2004::aid-anie2004>3.0.co;2-7
Connelly, N. G., and Geiger, W. E. (1996). Chemical redox agents for organometallic chemistry. Chem. Rev. 96 (2), 877–910. doi:10.1021/cr940053x
DiSalvo, F., Falconer, W., Hutton, R., Rodriguez, A., and Waszczak, J. (1975). A study of the magnetic state of O2+ in fluoride salts. J. Chem. Phys. 62 (7), 2575–2580. doi:10.1063/1.430838
Dixon, D. A., Feller, D., Christe, K. O., Wilson, W. W., Vij, A., Vij, V., et al. (2004). Enthalpies of formation of gas-phase N3, N3-, N5+, and N5- from ab initio molecular orbital theory, stability predictions for N5+N3- and N5+N5-, and experimental evidence for the instability of N5+N3-. J. Am. Chem. Soc. 126 (3), 834–843. doi:10.1021/ja0303182
Edwards, A. J., Falconer, W. E., Griffiths, J. E., Sunder, W. A., and Vasile, M. J. (1974). Syntheses and some properties of dioxygenyl fluorometallate salts. J. Chem. Soc. Dalton Trans. (11), 1129–1133. doi:10.1039/dt9740001129
Falconer, W. E., DiSalvo, F. J., Edwards, A. J., Griffiths, J. E., Sunder, W. A., and Vasile, M. J. (1976). Dioxygenyl hexafluoropalladate(V) O2+PdF6−: A quinquevalent compound of palladium. J. Inorg. Nucl. Chem. 28, 59–60. doi:10.1016/0022-1902(76)80595-7
Fischer, D., Klapötke, T. M., and Stierstorfer, J. (2015). 1, 5-di nitramino tetrazole: High sensitivity and superior explosive performance. Angew. Chem. Int. Ed. 54 (35), 10299–10302. doi:10.1002/anie.201502919
Fisher, E. R., and Armentrout, P. B. (1991). Kinetic energy dependence of the reactions of atomic oxygen(1+) and dioxygenyl ion with tetrafluoromethane and hexafluoroethane. J. Phys. Chem. 95 (16), 6118–6124. doi:10.1021/j100169a015
Frisch, M. J., Trucks, G. W., Schlegel, H. B., Scuseria, G. E., Robb, M. A., Cheeseman, J. R., et al. (2016). Gaussian 09, Revision D. 01. Wallingford CT: Gaussian, Inc. Available at http://www.gaussian.com
Gao, H., Ye, C., Piekarski, C. M., and Shreeve, J. n. M. (2007). Computational characterization of energetic salts. J. Phys. Chem. C 111 (28), 10718–10731. doi:10.1021/jp070702b
Gillespie, R. J., and Schrobilgen, G. J. (1974). Hexafluorobromine(VII) cation, BrF6+. Preparation of BrF6+AsF6- and BrF6+Sb2F11- and characterization by fluorine-19 nuclear magnetic resonance and Raman spectroscopy. Inorg. Chem. 13 (5), 1230–1235. doi:10.1021/ic50135a043
Glasser, L., and Jenkins, H. D. B. (2004). Standard absolute entropies, S°298, from volume or density. Thermochim. Acta 414 (2), 125–130. doi:10.1016/j.tca.2003.12.006
Goerigk, L., and Grimme, S. (2011). Efficient and accurate double-hybrid-meta-GGA density Functionals evaluation with the extended GMTKN30 database for general main group thermochemistry, kinetics, and noncovalent interactions. J. Chem. Theory Comput. 7 (2), 291–309. doi:10.1021/ct100466k
Goetschel, C. T., and Loos, K. R. (1972). Reaction of xenon with dioxygenyl tetrafluoroborate. Preparation of FXe-BF2. J. Am. Chem. Soc. 94 (9), 3018–3021. doi:10.1021/ja00764a022
Griffiths, J., Sunder, W., and Falconer, W. (1975). Raman spectra of O2+MF6−, O2+M2F11 and NO+MF6− salts: M= as, Sb, Bi, Nb, Ta, Ru, Rh, Pd, Pt, Au. Spectrochim. Acta Part A Mol. Spectrosc. 31 (9-10), 1207–1216. doi:10.1016/0584-8539(75)80175-9
Grill, A., Schieber, M., and Shamir, J. (1970). Paramagnetic susceptibility of a 2p unpaired electron in a crystalline field. Phys. Rev. Lett. 25 (11), 747–749. doi:10.1103/PhysRevLett.25.747
Haiges, R., Schneider, S., Schroer, T., and Christe, K. O. (2004). High-energy-density materials: Synthesis and characterization of N5+[P(N3)6]-, N5+[B(N3)4]-, N5+[HF2]- nHF, N5+[BF4]-, N5+[PF6]-, and N5+[SO3F]-. Angew. Chem. Int. Ed. 43 (37), 4919–4924. doi:10.1002/anie.200454242
Holfter, H., Klapötke, T. M., and Schulz, A. (1997). High energetic materials: Reaction of azides with dioxygenyl salts. Propellants Explos. Pyrotech. 22 (1), 51–54. doi:10.1002/prep.19970220111
Holloway, J. H., and Schrobilgen, G. J. (1975). Krypton fluoride chemistry; a route to AuF5, KrF+AuF6–, Xe2F3+AuF6–, and NO+AuF6–: The KrF+–XeOF4 system. J. Chem. Soc. Chem. Commun. (15), 623–624. doi:10.1039/C39750000623
Jenkins, H. D., Roobottom, H. K., Passmore, J., and Glasser, L. (1999). Relationships among ionic lattice energies, molecular (formula unit) volumes, and thermochemical radii. Inorg. Chem. 38 (16), 3609–3620. doi:10.1021/ic9812961
Jenkins, H. D., Tudela, D., and Glasser, L. (2002). Lattice potential energy estimation for complex ionic salts from density measurements. Inorg. Chem. 41 (9), 2364–2367. doi:10.1021/ic011216k
Lian, P., Lai, W., Chang, H., Li, Y., Li, H., Yang, W., et al. (2012). Density functional theoretical study of polynitrogen compounds N5+Y− (Y=B(CF3)4, BF4, PF6 and B(N3)4). Chin. J. Chem. 30 (3), 639–643. doi:10.1002/cjoc.201280011
Lu, T., and Chen, F. (2012a). Multiwfn: A multifunctional wavefunction analyzer. J. Comput. Chem. 33 (5), 580–592. doi:10.1002/jcc.22885
Lu, T., and Chen, F. (2012b). Quantitative analysis of molecular surface based on improved Marching Tetrahedra algorithm. J. Mol. Graph. Model. 38, 314–323. doi:10.1016/j.jmgm.2012.07.004
Lu, T., and Chen, Q. (2021). Interaction region indicator: A simple real space function clearly revealing both chemical bonds and weak interactions. Chem. Methods. 1 (5), 231–239. doi:10.1002/cmtd.202100007
McKee, D., and Bartlett, N. (1973). Dioxygenyl salts dioxygenylhexafluoroantimonate and dioxygenylundecafluorodiantimonate and their convenient laboratory syntheses. Inorg. Chem. 12 (11), 2738–2740. doi:10.1021/ic50129a050
Neese, F. (2011). The ORCA program system. WIREs Comput. Mol. Sci. 2 (1), 73–78. doi:10.1002/wcms.81
Nikitin, I. V., and Rosolovskii, V. Y. (1971). Oxygen fluorides and dioxygenyl compounds. Russ. Chem. Rev. 40 (11), 889–900. doi:10.1070/rc1971v040n11abeh001981
Rice, B. M., and Byrd, E. F. (2013). Evaluation of electrostatic descriptors for predicting crystalline density. J. Comput. Chem. 34 (25), 2146–2151. doi:10.1002/jcc.23369
Rice, B. M., Hare, J. J., and Byrd, E. F. (2007). Accurate predictions of crystal densities using quantum mechanical molecular volumes. J. Phys. Chem. A 111 (42), 10874–10879. doi:10.1021/jp073117j
Rigny, P., and Falconer, W. (1975). 19F relaxation in paramagnetic O2AsF6. J. Chem. Phys. 62 (7), 2581–2583. doi:10.1063/1.430839
Stein, L. (1973). Removal of xenon and radon from contaminated atmospheres with dioxygenyl hexafluoroantimonate, O2SbF6. Nature 243 (5401), 30–32. doi:10.1038/243030a0
Sun, Q., Wang, P., Lin, Q., and Lu, M. (2020). All-nitrogen ion-based compounds as energetic oxidizers: A theoretical study on [N5+] [NO3−], [N5+] [N(NO2)2−], [NO2+] [N5−] and NO2–N3. New J. Chem. 44 (26), 11188–11195. doi:10.1039/d0nj01441a
Venkatachalam, S., Santhosh, G., and Ninan Ninan, K. (2004). An overview on the synthetic routes and properties of ammonium dinitramide (ADN) and other dinitramide salts. Propellants Explos. Pyrotech. 29 (3), 178–187. doi:10.1002/prep.200400043
Vij, A., Wilson, W. W., Vij, V., Tham, F. S., Sheehy, J. A., and Christe, K. O. (2001). Polynitrogen chemistry. Synthesis, characterization, and crystal structure of surprisingly stable fluoroantimonate salts of N5+. J. Am. Chem. Soc. 123 (26), 6308–6313. doi:10.1021/ja010141g
Wang, F., Du, H., Zhang, J., and Gong, X. (2011). DFT studies on the structures and stabilities of N5+-containing salts. Struct. Chem. 22 (5), 1067–1073. doi:10.1007/s11224-011-9802-3
Wang, Y., Liu, Y., Song, S., Yang, Z., Qi, X., Wang, K., et al. (2018). Accelerating the discovery of insensitive high-energy-density materials by a materials genome approach. Nat. Commun. 9 (1), 2444. doi:10.1038/s41467-018-04897-z
Weigend, F., and Ahlrichs, R. (2005). Balanced basis sets of split valence, triple zeta valence and quadruple zeta valence quality for H to Rn: Design and assessment of accuracy. Phys. Chem. Chem. Phys. 7 (18), 3297–3305. doi:10.1039/b508541a
Wilson, W. W., Vij, A., Vij, V., Bernhardt, E., and Christe, K. O. (2003). Polynitrogen chemistry: Preparation and characterization of (N5)2SnF6, N5SnF5, and N5B(CF3)4. Chem. Eur. J. 9 (12), 2840–2844. doi:10.1002/chem.200304973
Wong, M. W., Gill, P. M. W., Nobes, R. H., and Radom, L. (2002). A second-row analogue of the 6-311G(d, basis set: calculated heats of formation for second-row hydrides. J. Phys. Chem. 92 (17), 4875–4880. doi:10.1021/j100328a015
Xu, Y., Tian, L., Li, D., Wang, P., and Lu, M. (2019). A series of energetic cyclo-pentazolate salts: Rapid synthesis, characterization, and promising performance. J. Mat. Chem. A Mat. 7 (20), 12468–12479. doi:10.1039/c9ta01077g
Xu, Y., Wang, Q., Shen, C., Lin, Q., Wang, P., and Lu, M. (2017). A series of energetic metal pentazolate hydrates. Nature 549 (7670), 78–81. doi:10.1038/nature23662
Yang, C., Zhang, C., Zheng, Z., Jiang, C., Luo, J., Du, Y., et al. (2018). Synthesis and characterization of cyclo-pentazolate salts of NH4+, NH3OH+, N2H5+, C(NH2)3+, and N(CH3)4+. J. Am. Chem. Soc. 140 (48), 16488–16494. doi:10.1021/jacs.8b05106
Yu, Y., Li, Y. C., Chen, J. F., Sun, C. H., Li, J. S., Fan, G. J., et al. (2015). Towards understanding the stability of the N5+-containing salts: The role of counterions. RSC Adv. 5 (127), 104841–104845. doi:10.1039/c5ra16304h
Zeng, Z., and Bernstein, E. R. (2019). Ammonium perchlorate and ammonium dihydrogen phosphate as energetic materials: Comparison to ammonium nitrate. J. Phys. Chem. C 123 (19), 12149–12153. doi:10.1021/acs.jpcc.9b02410
Zhang, C., Sun, C., Hu, B., Yu, C., and Lu, M. (2017). Synthesis and characterization of the pentazolate anion cyclo-N5 in (N5)6(H3O)3(NH4)4Cl. Science 355 (6323), 374–376. doi:10.1126/science.aah3840
Zhao, Y., and Truhlar, D. G. (2007). The M06 suite of density functionals for main group thermochemistry, thermochemical kinetics, noncovalent interactions, excited states, and transition elements: Two new functionals and systematic testing of four M06-class functionals and 12 other functionals. Theor. Chem. Acc. 120 (1-3), 215–241. doi:10.1007/s00214-007-0310-x
Keywords: super energetic oxidizer, O2+ and N5+ ionic salts, detonation performance, quantum chemical calculation, energetic materials
Citation: Yang X, Li N, Li Y and Pang S (2022) The ionic salts with super oxidizing ions O2+ and N5+: Potential candidates for high-energy oxidants. Front. Chem. 10:1005816. doi: 10.3389/fchem.2022.1005816
Received: 28 July 2022; Accepted: 29 August 2022;
Published: 21 September 2022.
Edited by:
Michael Gozin, Tel Aviv University, IsraelReviewed by:
Humberto Saint-Martin, Universidad Nacional Autonoma de México, MexicoGen Zhang, Nanjing University of Science and Technology, China
Copyright © 2022 Yang, Li, Li and Pang. This is an open-access article distributed under the terms of the Creative Commons Attribution License (CC BY). The use, distribution or reproduction in other forums is permitted, provided the original author(s) and the copyright owner(s) are credited and that the original publication in this journal is cited, in accordance with accepted academic practice. No use, distribution or reproduction is permitted which does not comply with these terms.
*Correspondence: Yuchuan Li, liyuchuan@bit.edu.cn; Siping Pang, pangsp@bit.edu.cn