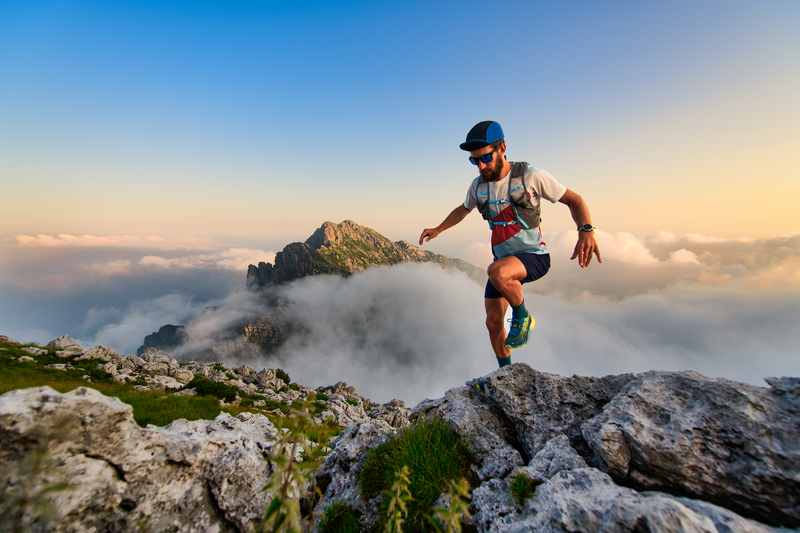
95% of researchers rate our articles as excellent or good
Learn more about the work of our research integrity team to safeguard the quality of each article we publish.
Find out more
MINI REVIEW article
Front. Chem. , 23 September 2022
Sec. Electrochemistry
Volume 10 - 2022 | https://doi.org/10.3389/fchem.2022.1000337
The cyclic stability of the MnOx cathodes for rechargeable zinc ion batteries have substantial obstacles due to Mn3+ disproportionation produces Mn2+ caused by Jahn Teller lattice distortion effect in the process of Zn2+ inter/deintercalation. This mini review summarized bulk-phase and interface stability strategies of manganese oxide cathodes for aqueous Zn-MnOx batteries from the regulation of bulk electronic state of manganese oxide improves its structural stability and the formation of beneficial SEI layer at the interface of electrolyte. It provides theoretical support for the design of manganese oxide cathode materials for aqueous zinc ion batteries with high stability.
Human society has been seeking new energy storage equipment with higher performance (such as higher energy density, power density and long life), low cost and high security to alleviate outstanding environmental problems and severe energy situation (Pomerantseva et al., 2019; Chen et al., 2021; Zheng et al., 2021). At present, lithium-ion batteries are widely used in mobile devices because of their high energy/power density and long life (Manthiram, 2020; Chen et al., 2022). However, with the increasing demand for large-scale energy storage such as electric vehicles and smart grid energy storage systems, as well as the high price of lithium resources and security, other high-performance and low-cost energy storage devices are needed to supplement (Jia et al., 2020; Zhong et al., 2020; Zhou et al., 2020). In recent years, batteries based on other monovalent metal ions (Na+, K+) and multivalent metal ions (Ca2+, Mg2+, Zn2+, Al3+, etc.) have attracted extensive attention due to their rich content and low cost (Li et al., 2020; Pan et al., 2021; Zhou and Guo, 2021). The aqueous zinc ion batteries used zinc metal as the anode material, which has many advantages, such as high theoretical specific capacity (820 mAh g−1), low oxidation-reduction potential of Zn2+/Zn(−0.76 V relative to SHE), which endows the aqueous zinc ion battery with the intrinsic characteristics of environmental friendliness, low cost and high safety (Cui et al., 2022). Especially, zinc ion battery as a kind of multivalent ion batteries, its energy density tends to increase due to the presence of high-capacity multivalent metals as the anode (Selvakumaran et al., 2019). The volumetric energy density of zinc ion battery is as high as 5851 mAh cm−3 higher than Li+ (2061 mAh cm−3), Mg2+ (3834 mAh cm−3), Ca2+ (2072 mAh cm−3) (Chen et al., 2019). This makes the aqueous rechargeable zinc ion battery one of the most promising electrochemical energy storage devices.
Because zinc metal anode has many advantages, the development of aqueous rechargeable zinc ion battery is largely limited by the cathode materials (Xia et al., 2018; Liu et al., 2019). At present, manganese based materials (MnO2, Mn3O4, MnO, etc.) (Tang et al., 2019; Zhu et al., 2020), vanadium based materials (V2O5, VO2, Zn0.25V2O5, etc.) (Wan and Niu, 2019; Boruah et al., 2021; Wang et al., 2022) and Prussian blue (Ming et al., 2019) have been studied as cathode materials for aqueous Zn ion batteries. Among them, manganese oxides (MnOx) have many advantages, such as high operating voltage (∼1.35 V), high theoretical specific capacity (based on single electron reaction ∼308 mAh g−1), multiple valence states of manganese, low cost, etc., making MnOx considered as one of the most potential cathode materials for aqueous rechargeable zinc ion batteries (Wang et al., 2020; Li et al., 2022; Zhang et al., 2022). These outstanding advantages make MnOx, including α-, β-,γ-, λ-, δ- MnO2, MnO, Mn3O4 and other structures are sought after. In 2015, S. H. Oh reported that α-MnO2 (2 × 2 channels) showed a specific capacity of 195 mAh g−1 at 10 mA g−1 and maintained 70% after 30 cycles (Lee et al., 2015). Although MnOx cathode materials show considerable specific capacity, they face the problem of rapid degradation of cycle stability. In order to solve this problem, Y.Y. Xia and Y.G. Wang inserted polyaniline into layered MnO2, which improved the stability of the structure and improved the cycle stability (Huang et al., 2018). J. Zhou and S.Q. Liang grew Mn3O4 in situ on stainless steel mesh, and the structure maintained 500 cycles at 500 mA g−1 (Zhu et al., 2018). These measures can help to improve the battery stability, but there are still substantial obstacles to the application of MnOx based rechargeable zinc ion battery cathode materials (Ming et al., 2019): 1) Mn3+ disproportionation produces Mn2+ caused by Jahn Teller (J-T) lattice distortion effect in the process of Zn2+ inter/deintercalation, which will directly lead to the decline of MnOx cycle stability. 2) The wide band gap of MnOx leads to low conductivity, which results in its unsatisfactory rate capability.
In this mini review, the stability and rate capability improvement strategies of manganese oxide cathode materials are proposed from two perspectives: 1) The electronic state structure of MnOx is regulated through defect engineering (O/Mn vacancy and transition metal doping), which can improve its structural stability, band gap and electrochemical activity, to inhibit Mn dissolution caused by J-T effect, accelerate ion/electron conduction, and improve cycle stability and rate capability. 2) The interfacial reaction kinetics of MnOx cathode and electrolyte are optimized enriching with electrolyte additives, which can be inhibit the dissolution of Mn in the process of MnOx zinc storage and accelerate the diffusion kinetics of ion interface, so as to effectively improve the cycle stability and specific capacity. This review provides theoretical support for the design of manganese oxide cathode materials for aqueous zinc ion batteries with high stability.
By changing the electronic state structure of t2g3egfn1 with high spin of Mn3+ and substituting transition metal elements for Mn3+, the structural stability of MnOx cathode material is improved to suppress the J-T effect, so as to improve its cycle stability, which has been deeply studied in the application of lithium/sodium ion batteries (Li et al., 2009; Xiao et al., 2018). Defect engineering (such as vacancy and doping) can improve the structural stability of MnOx, inhibit Mn3+ disproportionation caused by J-T effect, and improve the cycle stability of MnOx zinc storage process (H Zheng et al., 2022; Ji et al., 2022). At present, part of the work has carried out defect engineering research on manganese-based cathode materials for aqueous zinc ion batteries. In 2019, J. M. Xue pointed out that the Gibbs free energy of Zn2+ adsorption in the vicinity of O vacancy can be reduced to thermoneutral value (≈0.05 eV) by introduction of O vacancy in MnO2 lattice, which suggested that Zn2+ adsorption/desorption process on oxygen-deficient MnO2 is more reversible (Xiong et al., 2019). L. Q. Mai Doped Ti by surface gradient α-MnO2 produces electron compensated O vacancy, which accelerates the migration rate of ions/electrons, thus improving the diffusion coefficient of Zn2+/H+ in Ti-MnO2 (Lian et al., 2019). However, it mainly focuses on improving the electron/ion transfer rate to improve its performance, and less attention is paid to the influence of defect states on the electronic structure of Mn3+ and the improvement of the structural stability of MnOx to inhibit J-T from improving its zinc storage cycle stability. H. Wang and H. Z. Wan introduced O vacancy into Mn-O octahedron of Mn3O4 to improve the electronic structure of Mn3O4, thus improving the cycle stability (Figures 1A–C) (Tan et al., 2020). At the same time, they have effectively improved the structural stability of Mn3O4 and realized high cycle stability by replacing Mn with Co2+ (Figures 1D–F) (Ji et al., 2021). The sulfur doped MnO2 (S-MnO2) nanosheets have been found to be high-performance cathodes for zinc ion batteries (Figures 1G–I). The doped S atoms in O sites with lower electronegativity can improve its bulk conductivity, reduce the electrostatic interaction with Zn2+, and accelerate the reaction kinetics to improve cycle stability (Zhao et al., 2022). The MnOx cathode can be modified by defect engineering (O/Mn vacancy or transition metal element doping, etc.) to improve structural stability, inhibit Mn dissolution, and effectively improve cycle stability. This has important theoretical significance for the commercialization of manganese based zinc ion batteries.
FIGURE 1. (A) Supercell model of Mn3O4. (B) The Mn-O octahedral and pyramidal crystal fields and the d-orbital splitting configurations. (C) The TDOS of the Mn3O4 and Od-Mn3O4 bulk phase. (D) Schematic illustration of Zn//Co-Mn3O4/CNA battery. (E) Cycling performance of the Co-Mn3O4/CNA at 0.2 A g−1. (F) DOS of MnO2 and Co4+-δ-MnO2. (G) The side view of schematic illustration of Zn migration in S-MnO2. (H) The Zn ion diffusion barrier profiles for MnO2 and S-MnO2. (I) Cycling performance of MnO2 and S-MnO2 electrodes at 0.2 A g−1.
The electrode/electrolyte interface reaction process plays a key role in the performance of electrochemical energy storage devices. The different types of electrodes to optimize the electrode/electrolyte interface reaction dynamics using advanced electrolyte and new electrolyte additives can maximize the performance of aqueous zinc ion batteries (Wang et al., 2018; Xu et al., 2019). In 2016, J. Liu added Mn2+ into 2 M ZnSO4 electrolyte to improve the Mn2+ dissolution chemical potential of α-MnO2 electrode material in the process of zinc storage, which can effectively inhibit the J-T effect and improves cycle stability of α-MnO2/Zn battery (Pan et al., 2016). P. Chen etc (Wu et al., 2022) reported that the by-product of Zn4(OH)6SO4∙xH2O (ZHS) from pH fluctuation can react with Mn2+ during charge process at over 1.55 V to form a lowly crystallized (Zn,Mn)2Mn5O12∙4H2O (ZMO), which severely hinders the capacity of MnO2 cathode rapid fading (Figures 2A,B). The introduction of ion exchange resin (IER) can adjust the proton distribution in the electrolyte and eliminate the above adverse ZHS, so as to effectively inhibit the formation of ZMO, to improve cycle stability of MnO2 as cathode of Zn/MnO2 battery. Although some studies have shown that electrolyte optimization can improve the cycle stability of MnOx cathode materials, it is difficult to ensure that the cycle stability is improved without loss of specific capacity and rate performance.
FIGURE 2. (A) A schematic illustration of the irreversible structure change on the β-MnO2 cathode. (B) Schematic illustration of IER releasing and adsorbing protons. (C) The formation mechanism of the unique structure of CaSO4·2H2O (CS) SEI layer-coated Ca2MnO4 (CMO). (D) Schematic illustration of cathode/electrolyte interface in aqueous and waterless electrolyte. (E) The working mechanism of the interfacial reaction of the LiMn2O-cathode in ZnSO4-based aqueous electrolyte. (F) The ex-situ SEM images at fully discharged/charged state after (G) the 500th cycle and (E) the 1000th cycle.
In addition, the adverse effects of zinc hydroxysulfate by-products produced at the electrode/electrolyte interface of Mn based cathode materials in the process of Zn2+/H+ co-insertion have not attracted much attention. The interface of the MnOx electrode/electrolyte form a layer of zinc hydroxysulfate favorable SEI film from adverse factors by adjusting electrolyte environment, which can inhibit the dissolution of Mn2+, and effectively improve the cycle stability and the specific capacity and rate performance at the same time. J. Zhou found in the research of Ca2MnO4/Zn battery containing (Zn2++Mn2+)SO42- electrolyte that CaSO4·2H2O-SEI film formed on the surface of Ca2MnO4 cathode material, which effectively inhibited the dissolution of Mn during charge and discharge, and significantly improved the stability of the battery compared with the battery containing only CH3COO electrolyte (Figure 2C) (Shan et al., 2019). G.Z. Fang and S.Q. Liang put forward the relationship between the desolvation of Zn2+ from [Zn(OH2)6]2+-solvation shell and the electrolyte/electrode interfacial reaction to form Zn4SO4(OH)6·4H2O phase (Figures 2D–G). The Zn//MnO2 battery based on electrolyte optimization displayed the cycling stability of over 2000 cycles (Zhang et al., 2020). Thus, the process dynamics of the interface between cathode and electrolyte can be optimized by adjusting the electrolyte additives to match the manganese removal cathode materials, and the cycle stability can be effectively improved.
This work summarizes the bulk-phase and interface stability strategies of manganese oxide cathodes for aqueous Zn-MnO2 batteries. On the one hand, defect engineering regulated MnOx cathode can improve its structural stability and conductivity to improve cycle stability and rate capability. On the other hand, the interfacial reaction kinetics of MnOx cathode and electrolyte are optimized enriching with electrolyte additives can inhibit the dissolution of Mn and accelerate the diffusion kinetics of ion interface to effectively improve the cycle stability and specific capacity.
Although a lot of research work has been done on the optimization and modification of the cycle stability of MnOx cathode materials, the research on the regulation of bulk electronic states and the mechanism of interface adaptation is not deep and systematic, and the cycle stability that meets the commercial application has not been completely solved. To further improve the overall performance of MnOx cathode in aqueous solution system, we put forward the following anticipations: 1) To explore the best synthetic means and defect types of bulk electronic state regulation of manganese oxide, and systematically study the internal mechanism of bulk electronic state regulation; 2) The Zn2+ solvation structure was optimized by adding polar molecules or other additives into the electrolyte to improve the interface structure of manganese oxide and electrolyte. 3) Seeking an electrolyte adaptation system for in-situ construction of favorable SEI film on the surface of MnOx cathode, and establish a theoretical system of interface optimization; 4) Study the influence of parameters such as cathode and anode matching, electrolyte proportion and adaptation on device performance, and carry out a comprehensive evaluation of key performance such as energy density, power density and cycle stability of aqueous MnOx/Zn battery.
GY was responsible for text editing and article composing. HW were responsible for article review and revision.
This work was supported by the National Natural Science Foundation of China (No. 52002122), and the Project funded by China Postdoctoral Science Foundation (No. 2021M690947).
The authors declare that the research was conducted in the absence of any commercial or financial relationships that could be construed as a potential conflict of interest.
All claims expressed in this article are solely those of the authors and do not necessarily represent those of their affiliated organizations, or those of the publisher, the editors and the reviewers. Any product that may be evaluated in this article, or claim that may be made by its manufacturer, is not guaranteed or endorsed by the publisher.
Boruah, B. D., Mathieson, A., Park, S. K., Zhang, X., Wen, B., Tan, L. F., et al. (2021). Vanadium dioxide cathodes for high-rate photo-rechargeable zinc-ion batteries. Adv. Energy Mat. 11, 2100115. doi:10.1002/aenm.202100115
Chen, L. N., An, Q. Y., and Mai, L. Q. (2019). Recent advances and prospects of cathode materials for rechargeable aqueous zinc-ion batteries. Adv. Mat. Interfaces 6, 1900387. doi:10.1002/admi.201900387
Chen, M. Z., Liu, Y. F., Zhang, Y. Y., Xing, G. C., and Tang, Y. X. (2022). Lithium-rich sulfide/selenide cathodes for next-generation lithium-ion batteries: Challenges and perspectives. Chem. Commun. 58, 3591–3600. doi:10.1039/d2cc00477a
Chen, M. Z., Zhang, Y. Y., Xing, G. C., Chou, S. L., and Tang, Y. X. (2021). Electrochemical energy storage devices working in extreme conditions. Energy Environ. Sci. 14, 3323–3351. doi:10.1039/d1ee00271f
Cui, H. L., Ma, L. T., Huang, Z. D., Chen, Z., and Zhi, C. Y. (2022). Organic materials-based cathode for zinc ion battery. SmartMat 3, 1. doi:10.1002/smm2.1110
H Zheng, Z., Yang, G. C., Yao, J., Li, J. Y., Zheng, J. J., Wu, Z. A., et al. (2022). High-valence molybdenum promoted proton migration and inhibited dissolution for long-life aqueous Zn-MnO2 batteries. Appl. Surf. Sci. 592, 153335. doi:10.1016/j.apsusc.2022.153335
Huang, J. H., Wang, Z., Hou, M. Y., Dong, X. L., Liu, Y., Wang, Y. G., et al. (2018). Polyaniline-intercalated manganese dioxide nanolayers as a high-performance cathode material for an aqueous zinc-ion battery. Nat. Commun. 9, 2906. doi:10.1038/s41467-018-04949-4
Ji, J., Wan, H. Z., Zhang, B., Wang, C., Gan, Y., Tan, Q. Y., et al. (2021). Co2+/3+/4+-Regulated electron state of Mn-O for superb aqueous zinc-manganese oxide batteries. Adv. Energy Mat. 11 (6), 2003203. doi:10.1002/aenm.202003203
Ji, J., Yao, J., Xu, Y. C., Wan, H. Z., Zhang, B., Lv, L., et al. (2022). Promoting proton migration kinetics by Ni2+ regulating enables improved aqueous Zn-MnO2 batteries. Zhengzhou, China: Energy & Environmental Materials. doi:10.1002/eem2.12340
Jia, X. X., Liu, C. F., Neale, Z. G., Yang, J. H., and Cao, G. Z. (2020). Active materials for aqueous zinc ion batteries: Synthesis, crystal structure, morphology, and electrochemistry. Chem. Rev. 120 (15), 7795–7866. doi:10.1021/acs.chemrev.9b00628
Lee, B., Lee, H. R., Kim, H., Chung, K. Y., Cho, B. W., and Oh, S. H. (2015). Elucidating the intercalation mechanism of zinc ions into α-MnO2 for rechargeable zinc batteries. Chem. Commun. 51, 9265–9268. doi:10.1039/c5cc02585k
Li, M., Lu, J., Ji, X. L., Li, Y. G., Shao, Y. Y., Chen, Z. W., et al. (2020). Design strategies for nonaqueous multivalent-ion and monovalent-ion battery anodes. Nat. Rev. Mat. 5, 276–294. doi:10.1038/s41578-019-0166-4
Li, S. Y., Yu, D. X., Liu, L. N., Yao, S. Y., Wang, X. Q., Jin, X., et al. (2022). In-situ electrochemical induced artificial solid electrolyte interphase for MnO@C nanocomposite enabling long-lived aqueous zinc-ion batteries. Chem. Eng. J. 430, 132673. doi:10.1016/j.cej.2021.132673
Li, X. F., Xu, Y. L., and Wang, C. L. (2009). Suppression of Jahn–Teller distortion of spinel LiMn2O4 cathode. J. Alloys Compd. 479, 310–313. doi:10.1016/j.jallcom.2008.12.081
Lian, S. T., Sun, C. L., Xu, W. N., Huo, W. C., Luo, Y. Z., Zhao, K. N., et al. (2019). Built-in oriented electric field facilitating durable Zn-MnO2 battery. Nano Energy 62, 79–84. doi:10.1016/j.nanoen.2019.04.038
Liu, F., Chen, Z. X., Fang, G. Z., Wang, Z. Q., Cai, Y. S., Tang, B. Y., et al. (2019). V2O5 nanospheres with mixed vanadium valences as high electrochemically active aqueous zinc-ion battery cathode. Nanomicro. Lett. 11, 25. doi:10.1007/s40820-019-0256-2
Manthiram, A. (2020). A reflection on lithium-ion battery cathode chemistry. Nat. Commun. 11, 1550. doi:10.1038/s41467-020-15355-0
Ming, J., Guo, J., Xia, C., Wang, W. X., and Alshareef, H. N. (2019). Zinc-ion batteries: Materials, mechanisms, and applications. Mater. Sci. Eng. R Rep. 135, 58–84. doi:10.1016/j.mser.2018.10.002
Pan, H. L., Shao, Y. Y., Yan, P. F., Cheng, Y. W., Han, K. S., Nie, Z. M., et al. (2016). Reversible aqueous zinc/manganese oxide energy storage from conversion reactions. Nat. Energy 1, 16039. doi:10.1038/nenergy.2016.39
Pan, Z. H., Liu, X. M., Yang, J., Li, X., Liu, Z. L., Loh, X. J., et al. (2021). Aqueous rechargeable multivalent metal-ion batteries: Advances and challenges. Adv. Energy Mat. 11, 2100608. doi:10.1002/aenm.202100608
Pomerantseva, E., Bonaccorso, F., Feng, X. L., Cui, Y., and Gogotsi, Y. (2019). Energy storage: The future enabled by nanomaterials. Science 366, eaan8285. doi:10.1126/science.aan8285
Selvakumaran, D., Pan, A. Q., Liang, S. Q., and Cao, G. Z. (2019). A review on recent developments and challenges of cathode materials for rechargeable aqueous Zn-ion batteries. J. Mat. Chem. A 7, 18209–18236. doi:10.1039/c9ta05053a
Shan, G., Quan, L. S., Shan, Z. B., Zhao, F. G., Dan, M., and Jiang, Z. (2019). Cathode interfacial layer formation via in situ electrochemically charging in aqueous zinc-ion battery. ACS Nano 13, 13456–13464. doi:10.1021/acsnano.9b07042
Tan, Q. Y., Li, X. T., Zhang, B., Chen, X., Tian, Y. W., Wan, H. Z., et al. (2020). Valence engineering via in situ carbon reduction on octahedron sites Mn3O4 for ultra-long cycle life aqueous Zn-ion battery. Adv. Energy Mat. 10 (38), 2001050. doi:10.1002/aenm.202001050
Tang, B. Y., Shan, L. T., Liang, S. Q., and Zhou, J. (2019). Issues and opportunities facing aqueous zinc-ion batteries. Energy Environ. Sci. 12, 3288–3304. doi:10.1039/c9ee02526j
Wan, F., and Niu, Z. Q. (2019). Design strategies for vanadium-based aqueous zinc-ion batteries. Angew. Chem. Int. Ed. Engl. 58, 16508–16517. doi:10.1002/ange.201903941
Wang, F., Borodin, O., Gao, T., Fan, X. L., Sun, W., Han, F. D., et al. (2018). Highly reversible zinc metal anode for aqueous batteries. Nat. Mat. 17, 543–549. doi:10.1038/s41563-018-0063-z
Wang, N. Z., Yang, G. C., Gan, Y., Wan, H. Z., Chen, X., Wang, C., et al. (2020). Contribution of cation addition to MnO2 nanosheets on stable Co3O4 nanowires for aqueous zinc-ion battery. Front. Chem. 8, 793. doi:10.3389/fchem.2020.00793
Wang, X., Zhang, Z. C., Huang, M., Feng, J. K., Xiong, S. L., and Xi, B. J. (2022). In situ electrochemically activated vanadium oxide cathode for advanced aqueous Zn-ion batteries. Nano Lett. 22, 119–127. doi:10.1021/acs.nanolett.1c03409
Wu, Y., Zhi, J., Han, M., Liu, Z. Y., Shi, Q. Y., Liu, Y., et al. (2022). Regulating proton distribution by ion exchange resin to achieve long lifespan aqueous Zn-MnO2 battery. Energy Storage Mater. 51, 599–609. doi:10.1016/j.ensm.2022.07.009
Xia, C., Guo, J., Lei, Y. J., Liang, H. F., Zhao, C., and Alshareef, H. N. (2018). Rechargeable aqueous zinc-ion battery based on porous framework zinc pyrovanadate intercalation cathode. Adv. Mat. 30, 1705580. doi:10.1002/adma.201705580
Xiao, Y., Wang, P. F., Yin, Y. X., Zhu, Y. F., Yang, X., Zhang, X. D., et al. (2018). A layered-tunnel intergrowth structure for high-performance sodium-ion oxide cathode. Adv. Energy Mat. 8, 1800492. doi:10.1002/aenm.201800492
Xiong, T., Yu, Z. G., Wu, H. J., Du, Y. H., Xie, Q. D., Chen, J. S., et al. (2019). Defect engineering of oxygen‐deficient manganese oxide to achieve high‐performing aqueous zinc ion battery. Adv. Energy Mat. 9, 1803815. doi:10.1002/aenm.201803815
Xu, W. N., Zhao, K. N., Huo, W. C., Wang, Y. Z., Yao, G., Gu, X., et al. (2019). Diethyl ether as self-healing electrolyte additive enabled long-life rechargeable aqueous zinc ion batteries. Nano Energy 62, 275–281. doi:10.1016/j.nanoen.2019.05.042
Zhang, L., Yang, S. H., Fu, W. Q., Cui, Y. W., Wang, J. Q., Zhao, D. G., et al. (2022). Plasma-induced ε-MnO2 based aqueous zinc-ion batteries and their dissolution-deposition mechanism. J. Mater. Sci. Technol. 127, 206–213. doi:10.1016/j.jmst.2022.03.028
Zhang, T. S., Tang, Y., Fang, G. Z., Zhang, C. Y., Zhang, H. L., Guo, X., et al. (2020). Electrochemical activation of manganese-based cathode in aqueous zinc-ion electrolyte. Adv. Funct. Mater 30, 2002711.
Zhao, Y. J., Zhang, P. J., Liang, J. R., Xia, X. Y., Ren, L. T., Song, L., et al. (2022). Uncovering sulfur doping effect in MnO2 nanosheets as an efficient cathode for aqueous zinc ion battery. Energy Storage Mater. 47, 424–433. doi:10.1016/j.ensm.2022.02.030
Zheng, J., Bock, D. C., Tang, T., Zhao, Q., Yin, J., Tallman, K. R., et al. (2021). Regulating electrodeposition morphology in high-capacity aluminium and zinc battery anodes using interfacial metal-substrate bonding. Nat. Energy 6, 398–406. doi:10.1038/s41560-021-00797-7
Zhong, C., Liu, B., Ding, J., Liu, X. R., Zhong, Y. W., Li, Y., et al. (2020). Decoupling electrolytes towards stable and high-energy rechargeable aqueous zinc–manganese dioxide batteries. Nat. Energy 5, 440–449. doi:10.1038/s41560-020-0584-y
Zhou, J. H., and Guo, S. J. (2021). Carbon-based anode materials for potassium-ion batteries: From material, mechanism to performance. SmartMat 2, 176–201. doi:10.1002/smm2.1042
Zhou, W. H., Zhu, D., He, J., Li, J. C., Chen, H., Chen, Y. G., et al. (2020). A scalable top-down strategy toward practical metrics of Ni-Zn aqueous batteries with total energy densities of 165 Wh kg-1 and 506 Wh L-1. Energy Environ. Sci. 13 (11), 4157–4167. doi:10.1039/d0ee01221a
Zhu, C. Y., Fang, G. Z., Liang, S. Q., Chen, Z. X., Wang, Z. Q., Ma, J. Y., et al. (2020). Electrochemically induced cationic defect in MnO intercalation cathode for aqueous zinc-ion battery. Energy Storage Mater. 24, 394–401. doi:10.1016/j.ensm.2019.07.030
Keywords: MnOx, stability, cathode, interface, zinc ion battery
Citation: Yang G and Wan H (2022) Bulk-phase and interface stability strategies of manganese oxide cathodes for aqueous Zn-MnOx batteries. Front. Chem. 10:1000337. doi: 10.3389/fchem.2022.1000337
Received: 22 July 2022; Accepted: 31 August 2022;
Published: 23 September 2022.
Edited by:
Ge Li, University of Alberta, CanadaReviewed by:
Mingzhe Chen, Nanjing University of Science and Technology, ChinaCopyright © 2022 Yang and Wan. This is an open-access article distributed under the terms of the Creative Commons Attribution License (CC BY). The use, distribution or reproduction in other forums is permitted, provided the original author(s) and the copyright owner(s) are credited and that the original publication in this journal is cited, in accordance with accepted academic practice. No use, distribution or reproduction is permitted which does not comply with these terms.
*Correspondence: Houzhao Wan, aG91emhhb3dAaHVidS5lZHUuY24=
Disclaimer: All claims expressed in this article are solely those of the authors and do not necessarily represent those of their affiliated organizations, or those of the publisher, the editors and the reviewers. Any product that may be evaluated in this article or claim that may be made by its manufacturer is not guaranteed or endorsed by the publisher.
Research integrity at Frontiers
Learn more about the work of our research integrity team to safeguard the quality of each article we publish.