- 1Shenzhen Key Laboratory of Flexible Printed Electronics Technology Center, Harbin Institute of Technology, Shenzhen, China
- 2School of Materials Science and Engineering, Harbin Institute of Technology, Shenzhen, China
- 3School of Materials Science and Engineering, Dongguan University of Technology, Dongguan, China
- 4Department of Civil and Environmental Engineering, Harbin Institute of Technology, Shenzhen, China
In recent years, thanks to the investigation of the in-depth mechanism, novel cathode material exploitation, and electrolyte optimization, the electrochemical performance of rechargeable Zn-based batteries (RZBs) has been significantly improved. Nevertheless, there are still some persistent challenges locating the instability of the Zn anodes that hinder the commercialization and industrialization of RZBs, especially the obstinate dendrites and hydrogen evolution reaction (HER) on Zn anodes, which will dramatically compromise the cycle stability and Coulombic efficiency. Therefore, various strategies with fundamental design principles focusing on the suppression of dendrite and the HER have been carefully summarized and categorized in this review, which are critically dissected according to the intrinsic mechanisms. Finally, pertinent insights into the challenges and perspectives on the future development of Zn anodes are also emphasized, expecting to supply potential research directions to promote the practical applications of RZBs.
Introduction
Rechargeable Zn-based batteries (RZBs) that possess synergistic advantages of intrinsic safety, environmental benignity, and satisfactory energy density have raised particular attention and been considered as a promising candidate for next-generation energy storage. Typically, RZBs consist of a metallic Zn anode, electrolyte, and an (in)organic cathode. Tremendous progress in recent years has been made for RZBs, which mainly focused on developing high-performance cathode materials (e.g., manganese-based oxides (Pan et al., 2016), vanadium-based oxides (Liu et al., 2020), Prussian blue analog-based compounds (Hurlbutt et al., 2018), and organic materials (Wan et al., 2018)) based on diverse energy storage mechanisms including chemical conversion, Zn2+ intercalation, co-insertion, and hybrid chemistry mechanism (Li et al., 2019). Additionally, electrolytes also play a key role in rechargeable Zn-based batteries, which can be divided into aqueous and non-aqueous electrolytes (e.g., organic electrolytes and ion liquid). The recent mainstream is the aqueous electrolytes, which are generally divided into neutral/mildly acid or alkaline based on pH. Through a series of systematic research for cathode materials exploration and electrolyte optimization, the electrochemical performances of RZBs have been significantly enhanced.
The Zn anode features the advantages of resource availability, low electrochemical potential (−0.76 V), and high theoretical capacity (820 mAh g−1), and its reaction can be generalized to be a zinc-plating/striping process (Chao et al., 2019; Jia et al., 2020). Nevertheless, the instability of the Zn anode and insufficient understanding of relative mechanisms have impeded RZBs from being deployed in their proposed commercialization and large-scale production. The main issues, from which the Zn anodes are suffering, can be categorized into two parts: dendrites and hydrogen evolution reaction (HER)/corrosion. Similar to many other metal anodes, the formation of Zn dendrites during the cyclic plating/stripping process is an intractable problem that results in capacity fading and eventual battery failure (Zhao J. et al., 2019; Yang Q. et al., 2020). On the other side, the HER and corrosion in aqueous electrolytes are also persistent challenges, which normally leads to the increased impedance, capacity deterioration, and electrolyte leakage (Ma et al., 2020). Worse of all, these issues are mutually reinforced that the byproducts originated from the HER and corrosion will be detrimental to the uniform ion transmission, thus inducing more dendrites. Meanwhile, the accumulated dendrites will increase specific areas on the Zn anode surface, exacerbating the HER and corrosion (Li et al., 2020a; Yi et al., 2021). If appropriate coping strategies can be developed to alleviate or even eliminate these problems, it will greatly enhance the battery performance of RZBs.
In this article, we first briefly summarize the fundamental mechanisms and effects of Zn dendrites and the HER in aqueous electrolytes. Second, with the aim of an integrated improvement, the corresponding stabilization strategies to suppress Zn dendrites, the HER, and corrosion are comprehensively summarized and discussed from perspectives of thermodynamics and kinetics. Finally, challenges and constructive perspectives of Zn anode optimization for the commercialization and industrialization of RZBs are proposed, with a hope to promote their practical applications.
Fundamentals of Dendrite Formation and the Hydrogen Evolution Reaction
So far, Zn metal has been widely applied as anode materials for RZBs due to its intrinsic eco-friendliness and electrochemical stability. Many research studies based on experiments and simulations have been performed on the Zn anodes to achieve a systematic understanding of the electrochemical properties and associated influences on RZBs. However, it still suffers from some detrimental issues which are plaguing the scale-up implementation of RZBs. During the Zn plating/stripping process, the Zn dendrites would form on the surface of the Zn anode and increase its surface area. Additionally, HER/corrosion reactions would also occur on the metal Zn surface, causing a continuous consumption of active Zn and the increase of OH− concentration. The evolved H2 on the anode surface would hinder Zn nucleation, leading to the increased overpotential and an uneven deposition. Meanwhile, the insoluble byproducts would form and adhere to the anode surface, resulting in the surface passivation of fresh Zn. This process would adversely affect the interface impedance between the electrolyte and the Zn anode, resulting in the increased electrode polarization. These irreversible side reactions of the HER, corrosion, and surface passivation, as well as Zn dendrite formation, generate a vicious circle and seriously affect the stability of the Zn anode (Tang et al., 2019). In this review, the persistent challenges of dendrite formation and the HER are comprehensively discussed.
Fundamentals of Dendrite Formation
Under the electric field, the plating/stripping process of Zn ions is mainly commanded by the microenvironments of the anode and electrolyte including the surface polarization and 2D diffusion, as well as the mass transfer near the interface, resulting in the existence of original protrusions on the anode surface (Trócoli and La Mantia, 2015). These protrusions can be subsequently consolidated by the “tip effect” and grow perpendicularly to the surface to eventually formed dendrites (Zhang et al., 2020). Concentration polarization, which is referred to the electrochemical potential shift of the cell by comparing with its equilibrium value originated from the ion concentration difference between the electrolyte and the electrode surface, will further exacerbate dendrite issues at high current density (Yang et al., 2019). The growth of the Zn dendrite will ultimately make it pierce through the separator and cause short circuit.
Fundamentals of the Hydrogen Evolution Reaction
The hydrogen evolution during Zn plating/stripping is originated from both H2O hydrolysis and zinc corrosion, which also normally occurs on the Zn anode surface. The fundamental reason of the hydrogen evolution is the thermodynamic instability of the Zn anode while working in an aqueous solution (Amiinu et al., 2017). In the mildly acidic/neutral electrolytes, the fundamental reaction of Zn anodes can be simply explained as the equation Zn=Zn2++2e−. The reaction chemistry of the interfacial parasitic HER of Zn anodes can be depicted as 2H2O+2e−→H2↑+2OH−, and the formation of insoluble byproducts can be summarized as Zn2++2e−→Zn(OH)2 and Zn(OH)2→ZnO + H2O or other byproducts (Bayaguud et al., 2022). In addition, while in alkaline electrolytes, the working mechanism of Zn anodes can be explained as Zn+4OH− = Zn(OH)4-+2e−. The reaction chemistry of the parasitic HER can also be depicted as 2H2O+2e−→H2↑+2OH−, which occurred on the Zn anode surface accompanied by many other complex parasitic reactions (Tang et al., 2019).
In recent research of Zn-based batteries, RZBs in mild electrolytes are the main steam. The Zn-based batteries in an alkaline electrolyte that has not been fully investigated are briefly introduced in this review for future reference. Moreover, the HER and dendrite formation are both susceptible to the interface between the electrolyte and anode, as well as the surface condition of the Zn anode. The hydrogen evolution normally occurs from chemical/electrochemical reactions and causes the increased internal pressure of the sealed electric cells, leading to the failure of the sealing.
Dendrite Inhibition Strategies
Exploring the suppression strategies of the Zn dendrite for uniform Zn deposition is beneficial to the cyclic stability and high Coulombic efficiency of rechargeable Zn-based batteries. Figure 1A illustrated the formation process of the Zn dendrite (Lu et al., 2018), and the surface micromorphology of deposited Zn reveals a flake distribution (Figure 1B) (Yang et al., 2019). During the charging–discharging cycles, the formation of the Zn dendrite is dominated by the mass transfer process mainly affected by the concentration gradient and the electric field distribution (Li et al., 2018). In addition, the dominant crystallographic orientation and the initial surface texture of the Zn anode also have great influence on the electrochemical performance, especially the rate and cycle capabilities. With the efforts of all parts concerned, a series of strategies have been proposed and categorized into four types: mechanical shielding, crystallographic orientation manipulation, electric field control, and ion transmission regulation.
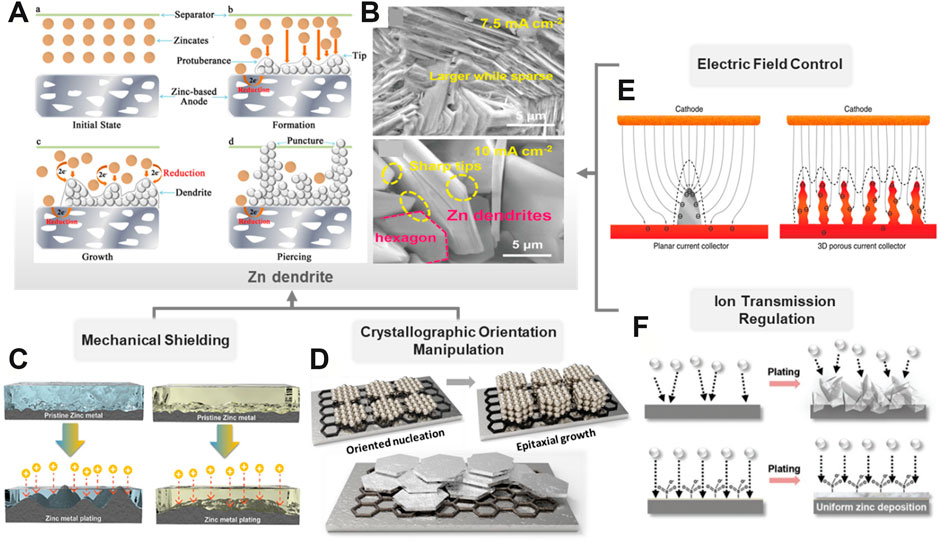
FIGURE 1. Schematic diagram of strategies for Zn dendrite suppression. (A) Fundamentals of Zn dendrite formation. (Lu et al., 2018) with permission from Wiley-VCH. (B) Scanning electron microscopy (SEM) images of the Zn dendrite. (Yang et al., 2019) with permission from Wiley-VCH. (C) Comparison of the morphology evolution of Zn foils with the liquid electrolyte and self-healable hydrogel electrolyte. (Ling et al., 2021) with permission from ELSEVIER. (D) Schematic illustration of the epitaxial metal electrodeposition of the Zn crystal. (E) Working mechanism and effect of the 3D current collector. (Yang et al., 2015) with permission from Springer. (F) Comparison of Zn deposition behavior while using the liquid electrolyte and a zwitterionic hydrogel electrolyte. (Mo et al., 2020) with permission from Wiley-VCH.
Mechanical Shielding
The Zn dendrites normally grow perpendicularly to the substrate and lead to the trend of a short circuit process. A shielding layer with high Young’s modulus will contribute to alleviating the dendrite growth from vertical evolution and lead to a better uniform deposition surface (Figure 1C) (Ling et al., 2021). From the mechanical perspective, physically mechanical shielding undoubtedly influences the dendrite growth by improving the interface strength to prevent battery failure. For instance, the solid-state electrolyte (SSE) can not only provide mechanical shielding with high strength but also avoid many parasitic reactions which often occur in aqueous counterparts (Wu et al., 2020). In general, SSEs include inorganic solid electrolytes (ISEs) and solid polymer electrolytes (SPEs). Research practices in the field of RZBs usually focus on the SPEs with moderate strength for the mechanical shielding layer. For instance, poly(ethylene oxide) (PEO) has attracted wide attention due to its good solubility to salts and relatively high ionic conductivity (Ma et al., 2020). In addition, the performance of ionic conductivity and mechanical stability of SPEs can be optimized by a combination of the PEO skeleton and functional fillers such as TiO2 nanoparticles, in which Zn2+ ions are transferred by the segmental motion of PEO chains, and the TiO2 nanoparticles contribute to the mechanical strength (Hiralal et al., 2010).
For RZBs, higher mechanical strength will further stabilize the Zn anodes theoretically. However, there are still no deep research and systematic simulation to quantify the threshold in the previous SSE research. Moreover, the poor interfacial contact and low ionic conductivity accompanied by high polarization are also becoming the burning issues. The physical shielding method is generally executed in coordination with other strategies in the strengthened solid-state electrolyte, separator, surface coating, and so on, and the most vital problem in developing strong mechanical shielding is how to balance the mechanical properties and electrochemical properties such as ion conductivity.
Crystallographic Orientation Manipulation
At the beginning of electrodeposition, the deposited Zn nucleates randomly along various crystallographic orientations. The Zn crystals are subsequently formed and grow through the preferred crystallographic (001) facet due to their hexagonally close-packed orientated texture, resulting in hexagonal crystal plates in diverse directions (Han et al., 2020). Typically, the crystals which grow vertically to the substrate will form large dendrites and eventually pierce the separator, leading to battery failure. If the Zn crystal plates grow along the (002) direction, which are parallel to the substrate during electrodeposition, the resultant Zn anode with a dense and parallel-orientated texture would deliver better electrochemical/chemical stability (Zheng et al., 2019).
A lattice-fitting substrate for Zn deposition is critical for crystallographic orientations. During the epitaxial electrodeposition process, if the coherent or semicoherent interface layer between the deposited Zn layers and the substrate is formed and tends to grow parallelly to the substrate, a maximum interfacial compatibility and a minimal interfacial energy between the Zn-deposited layer and the substrate will be realized (Figure 1D) (Du et al., 2020). For instance, Archer et al. employed graphene, which possessed a low lattice mismatch with Zn, as an epitaxial deposition substrate to lock the crystallographic orientation of Zn plating (Zheng et al., 2019). The minimal lattice strain of the epilayer growth could facilitate the formation of a compact and flat interface for uniform Zn deposition.
The composition of the electrolyte that involves the salts, solvents, and additives is another important factor for the crystallographic orientations of Zn deposition. For example, sodium dodecyl sulfate (SDS) as the organic additive could effectively facilitate the Zn crystal growth along the (002) plane, thus resulting in a dense and orientated electroplating texture and thus extending the battery lifespan to over 1,000 cycles (Sun et al., 2017).
Apart from the substrate and electrolyte, the composition of a separator also influences the Zn crystal growth in a uniform manner. For example, a composite Nafion membrane was investigated and reported as an effective separator to regulate the Zn deposition behavior (Yuan et al., 2019). The abundant sulfate groups on the Nafion-based membrane could reshape the coordination of Zn2+ in an aqueous medium and contribute to the formation of a solid electrolyte interface (SEI) that is parallel to the substrate surface. This SEI was beneficial to the epitaxial Zn deposition and the oriented crystal growth, leading to a prolonged battery lifespan.
Electric Field Control
Electric field is the driving force of ion transmission for initiating electrochemical reactions. With the elaborate structural design of electrodes or current collectors, the local areal current density will be decreased, leading to decreased polarization and uniform electric field distribution. This will alter the dendrite growth into a lateral trend, instead of vertical accumulation (Zou et al., 2018). For a conventional planar current collector, abundant small protrusions will be formed on the planar structure under the electric field due to 2D ion diffusion and nucleation and act as the favorable stripping points amplifying the subsequent deposition. When employing a 3D current collector such as the porous foam (Chamoun et al., 2015), sponge (Parker et al., 2017), micro-nanosheet (Zhao T. et al., 2018), and multi-channel metal lattices (Zhang et al., 2021), it was found that a uniform electric field could be initiated by the enlarged electroactive surface. The ion would nucleate and deposit in a homogenous way and gradually fill in the micropores within the 3D current collector, eventually eliminating the dendrite issues (Figure 1E) (Yang et al., 2015). Lu et al. developed a designed 3D Zn@CNTs with good affinity between the Zn layer and substrate, which displayed a stable stripping/plating cycling with over 200 h in a symmetric cell due to the homogenous precipitation of Zn (Zeng Y. et al., 2019). However, compared with the planar structure, the side reaction issues of the 3D structures are more severe due to their larger electroactive sites.
Employing charge/discharge protocols is an effective and cost-saving strategy to influence the dendrite growth by introducing preset in situ charging/discharging steps. Currently, an electrohealing strategy was developed to eliminate the existing dendrite by applying a cyclic stripping/plating process under the relatively low current density (Yang et al., 2019). Through this treatment, the concentration polarization surrounding the dendrite tips formed during current density cycling will be eased, and the sharp tips will, thus, be removed during stripping, resulting in a flat dendritic morphology and smooth surface. This strategy enabled the Zn symmetric cell a 516% prolonged lifespan even at 10 mA cm−2. This strategy is accessible without any special treatment, which provides a new perspective for battery maintenance.
Ion Transmission Regulation
The ion transmission makes a significant difference in its nucleation barrier. In general, through regulating electrolyte components and concentration, the faster and more uniform ion migration can display a smaller nucleation polarization, which is conducive to the dendrite suppression (Zhang et al., 2016). Interfacial modification can stabilize the anode by alleviating parasitic reactions and providing an ion filter for uniform deposition in the meantime. Some functionalized separators will also regulate the ion flux motion for facilitating homogenous plating (Liang et al., 2020). Therefore, the strategies for ion transmission regulation can be developed from the following aspects: electrolytes, surface coatings, and separators.
The strategy of electrolyte optimization includes ion transfer facilitation and ion flux regulation. Normally, the salts with large anions in an electrolyte, such as Zn(CF3SO3)2 and zinc bis(trifluoromethanesulfonyl)imide (Zn(TFSI)2), contribute to a smaller potential hysteresis during Zn2+ plating/stripping than ZnSO4 or ZnCl2. This is because the large anions will attenuate the solvated sheath around Zn2+, leading to the lower ion-flux resistance (Sun et al., 2017). Hydrogel electrolyte, which possesses the homogenous polymeric matrix, can guide a uniform Zn ion flux and deposition (Figure 1F) (Mo et al., 2020). Similarly, some organic electrolyte additives such as polyethylene glycol (PEG) can be adsorbed on the anode surface and restrict the 2D Zn2+ diffusion, leading to uniform and dense Zn deposition (Mitha et al., 2018). Some surfactants such as sodium dodecyl sulfate and cetyltrimethylammonium bromide are also conducive to the ion transmission via selective adsorption, thus affecting the preferential orientation of Zn dendrites (Zeng X. et al., 2019; Liu et al., 2019).
Surface coating is a direct way to stabilize anodes prepared by spin coating, doctor blading, and atomic layer deposition, which can guide the Zn ion flux and facilitate a uniform Zn nucleation, thus realizing a fat Zn deposition layer (Li et al., 2020b; Deng et al., 2021). An ideal coating layer to prevent the dendrite growth should possess high ionic conductivity, long-term stability, and good compatibility with electrolytes. Normally, it can be clarified into inorganic and organic coatings. So far, CaCO3- (Kang et al., 2018), TiO2- (Zhao K. et al., 2018), ZnO- (Xie X. et al., 2020), and hafnium oxide (HfO2) (Li B. et al., 2021)-based inorganic coatings have been investigated and developed to guide the ion flux for uniform deposition. Nevertheless, their large weight will lower the power density (Li Q. et al., 2020). Organic coatings, such as PAM, polyvinyl butyral (PVB), 502 glue, and polyacrylonitrile (PAN), have been widely investigated and utilized as artificial SEIs for Zn anode stabilization, which exhibit abundant polar groups that can interact with solvated metallic ions (Li et al., 2022). These polymeric coatings could not only provide homogenous ion pathways and restrict the 2D diffusion of Zn2+ on the surface but also function like a shelter to block the detrimental side reactions. However, the mechanical stability of these polymeric coatings for dendrite suppression needs improvement (Rui et al., 2020).
The intrinsic properties of a separator such as elastic modulus, composition, and void uniformity will significantly influence the ion flux, resistance of ion transmission, and ion distribution. Through comparative study of the most commonly used separators, such as the filter paper, glass fiber, and polypropylene membrane, researchers found that these materials were difficult to achieve high elastic modulus, uniform ion distribution, low ion flux resistance, and good wettability at the same time (Xie C. et al., 2020). Hence, developing advanced separators is essential to prevent the dendrite growth and improve battery performance. Ghosh et al. reported a Zn2+-integrated Nafion-based ionomer membrane with superior mechanical strength and wettability, which can effectively guide the uniform ion distribution on the surface of the electrode (Ghosh et al., 2019).
Hydrogen Evolution Reaction Suppression Strategies
The HER is mainly related with the chemical activity of Zn metal and water, which is harmful to the battery performance of RZBs by causing corrosion and cell inflation (Figure 2A) (Bayaguud et al., 2022). Excessive hydrogen evolution may cause capacity deterioration and electrolyte leakage (Zhao J. et al., 2019). Substantial evidences have indicated that the HER can normally be mitigated from the thermodynamic and dynamic points such as manipulating the chemical activity and constructing a physical barrier (Yi et al., 2021).
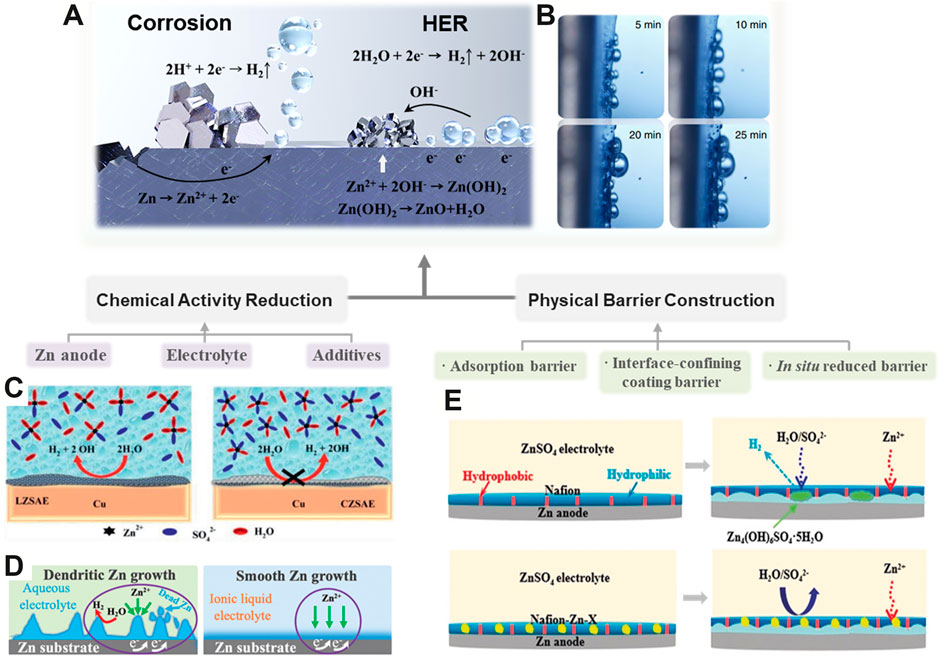
FIGURE 2. Schematic diagram of strategies for HER suppression. (A) Fundamentals of the HER and corrosion. (B) In situ optical micrograph of the HER. (Qiu et al., 2019) with permission from Springer. (C) High concentrated ZnSO4 electrolyte for HER suppression. (Olbasa et al., 2020) with permission from American Chemical Society. (D) Comparison of the HER and corrosion while using the aqueous electrolyte and ionic liquid electrolyte. (Ma et al., 2020) with permission from Wiley-VCH. (E) HER and corrosion inhibition effect of polyamide composite coating in the ZnSO4 electrolyte. (Cui et al., 2020) with permission from Wiley-VCH.
Chemical Activity Reduction
The activity of the Zn anode plays a vital role in the appearance of spontaneous corrosion and the HER catalytic activity during Zn stripping/plating (Figure 2B) (Qiu et al., 2019). To this end, optimizing the composition of the Zn anode can increase the corrosion resistance by enlarging the overpotential threshold of the HER (Shivkumar et al., 1998). In Zn–air batteries, some heavy metals such as Pb, Hg, and their oxides have been widely utilized as additives to suppress Zn corrosion, but the heavy metal pollution issues they brought cannot be ignored (Lee et al., 2006; Huang & Yang, 2015). Hence, some eco-friendly metallic compounds containing Al, Ni, In, and their oxides/hydroxides have been developed. For example, a Zn–Al–In hydrotalcite-like metallic compound which possesses a high overpotential of the HER was fabricated as a Zn anode (Wang et al., 2014). This composite anode enables the overpotential of the HER shift positively, thus inhibiting the corrosion of the anode. In addition, a series of metal oxides (e.g., Al2O3, Bi2O3, In2O3, etc.) have been introduced into Zn anodes as additives to suppress the HER and corrosion issues (Lee et al., 2013). Considering the Zn/Al2O3 composites as an example, it was found that the Zn/Al2O3 anode exhibited the lowest HER rate, whereas the pure Zn anode displayed the highest one. This is ascribed to the higher HER overpotential induced by the incorporation of Al2O3.
Apart from modifying the Zn anode, engineering the electrolyte could also significantly influence the HER process. Typically, tuning the concentration of salts, solvent, and the additives in the electrolyte is a fundamental approach to regulate the kinetics and thermodynamics of the HER. Increasing the salt concentration can not only decrease the equilibrium potential of H2O/H2 but also alter the coordination of Zn2+ ions and reshape the solvation sheath, which are favorable for HER suppression (Figure 2C) (Olbasa et al., 2020). For instance, the concentrated ZnCl2 with 30 mol L−1 could facilitate the coordination between Zn2+ ions and water molecules to form [Zn(OH2)Cl4]2− and [ZnCl4]2− instead of the [Zn(OH2)6]2+, thus ameliorating the HER and anode corrosion (Zhang et al., 2018). Similarly, a “water-in-salt” electrolyte containing 1 M Zn(CF3SO3)2 and 21 M LiN(CF3SO2)2 was reported. This electrolyte could enable the corrosion potential to shift positively from −0.974 to −0.768 V based on Tafel plots, which was due to the constrained activity of water. As a result, the corrosion and HER are restrained (Wan et al., 2019). In addition, several organic solvents such as acetonitrile and ethyl methyl carbonate have been widely used to dissolve a variety of Zn salts (e.g., Zn(ClO4)2, Zn(TFSI)2, and zinc trifluoromethanesulfonate (Zn(TfO)2).) for preparing potential non-aqueous electrolytes, which displayed a wide electrochemical stability window in RZBs (Wang et al., 2020; Pan et al., 2018). Ionic liquid is another important category of non-aqueous electrolytes to eliminate the HER, which features high-temperature stability and good compatibility with Zn salts. Recently, the 1-ethyl-3-methylimidazolium tetrafluoroborate (ILZE) electrolyte dissolved with 2 mol L−1 Zn(BF4)2 was developed, which showed excellent HER and Zn dendrite suppression properties during prolonged cycling (Figure 2D) (Ma et al., 2020).
Adding functional additives into an aqueous electrolyte can also effectively stabilize the H2O molecules for HER suppression. Some inorganic oxides (e.g., fumed) such as silica have been employed as an electrolyte additive to suppress the HER because they can form strong interaction with water molecules through hydrogen bonds (Huang et al., 2019). Moreover, some organic additives such as polyacrylamide (PAM) could decrease the Zn nucleation barrier, thereby leading to a compact and dense Zn deposition, which is beneficial to the HER and corrosion suppression (Zhang et al., 2019).
Increasing the salt concentration not only can suppress the HER but also can increase the ionic conductivity in a certain content. However, high salt concentration may bring some undesirable parasitic reactions, an increased cost, and the salting out effect. In addition, by utilizing the organic solvents, the property of HER inhibition will be very effective due to its water-free characteristic. However, the ionic conductivity of most organic solvents is always unsatisfactory, and the safety issues such as flammability and toxicity still need to be solved. For the additive employment, the ionic conductivity of the electrolyte and cost would not be seriously affected due to the low content of additives in the electrolyte, but the long-term stability of HER inhibition of utilizing additives may not be as good as the aforementioned two strategies.
Physical Barrier Construction
Constructing a physical barrier between the electrode and electrolyte is a direct and effective way to ameliorate the HER and corrosion in an aqueous electrolyte through physically isolating the H2O molecules from the anode. Based on the working mechanisms, the reported barriers can be divided into three categories as discussed in the following section.
The first type is the adsorption barrier, which is operated by the interaction between the functional groups in the barrier and substrate surface. For instance, a small amount of 10 mmol of tricine(N-(tri(hydroxymethyl)methyl)glycine) was employed as the corrosion inhibitor to protect the Zn anode, which showed over 90% inhibition efficiency (Nady, 2017). This was ascribed to the fact that the inhibitor molecules physically interacted on the Zn anode could alleviate the formation of the corrosion product of Zn5(OH)8Cl2·H2O. In addition, some organic molecules and surfactants can also inhibit the HER and Zn corrosion through surface adsorption as discussed in the “Chemical Activity Reduction” section (Sun et al., 2017).
Similar to the SEI layer in a lithium ion battery, an in situ reduced barrier which contains anions with variable valence can be applied in RZBs to construct a protective layer for HER suppression. For example, the electrolytes containing ClO4− could form an insoluble chloride layer onto the surface of the Zn anode due to its reduction during the repeated cycling process, which significantly prolonged the cycle lifespan of Zn anodes without any HER and corrosion by-products that could be detected by XPS characterization (Wang et al., 2019).
Furthermore, a suitable interface-confining coating barrier can also configure a chemically stable electrolyte/electrode interface to restrain the contact between free H2O molecules and the Zn anode surface, thus eliminating the parasitic reactions (Li C. et al., 2021; Shan et al., 2021). Recently, a MOF-based coating with abundant ion-transmission tunnels was developed for the Zn anode, which could spontaneously regulate the solvation structure to obtain a super-saturated electrolyte before Zn2+ reduction (Yang H. et al., 2020). The Zn2+·OSO32− ion could constrain water molecules after a partial desolvation process and subsequently transferred to the Zn anode through MOF channels with proper configuration, resulting in high electrochemical stability. In addition, some organic coatings such as polyamide (PA) (Zhao Z. et al., 2019), polymer glue (Jiao et al., 2021), and Nafion film (Cui et al., 2020), which normally show high adhesion to the substrate and low permeability of water molecules, can also serve as a HER barrier to protect the Zn anode from direct contact of water molecules, thus alleviating the undesirable gas evolution and Zn corrosion (Figure 2E).
Conclusion and Outlook
In conclusion, although tremendous research has been made for developing high-performance RZBs, there are still persistent challenges needed to be conquered, especially the Zn anode issues involving dendrites and corrosion/HER issues. In this review, the fundamentals of the Zn dendrites and HER on Zn anodes have been briefly summarized accompanied with the associated reaction mechanisms. To address these issues, a series of strategies and the fundamental design principles for the dendrite and HER suppression are categorized and analyzed in-depth. To facilitate the deployment in practical applications of RZBs, the following suggestions and anticipations were proposed:
1. In general, the issues faced in practical applications are mutually exacerbated, and only employing a single optimization strategy cannot thoroughly eliminate all the problems. Hence, synergistic strategies with integrated functions combining the aforementioned methods to tackle multiple problems are highly desired. For instance, the economic hydrogel electrolyte can not only mediate the ion transmission but also serve as a thin surface coating to provide physical shielding for dendrite and corrosion prevention, thus improving the long-term stability.
2. Addressing one issue should not repose on deteriorating other properties. For example, applying 3D electrodes for dendrite suppression may expose a more specific surface for causing passivation and the HER, especially under harsh operating conditions.
3. The major advantages of RZBs compared to lithium batteries are cost-effectiveness, extremely intrinsic safety, and environment-friendly. Therefore, while exploring the organic electrolyte of RZBs for HER suppression, the aspects of toxicity and flammability should be carefully considered. Otherwise, the research for developing RZBs would be of limited significance.
4. While operating at a high temperature, the Zn corrosion and HER in RZBs would generally be aggravated due to its increased corrosion current and decreased HER overpotential. It is worth conducting the research regarding the adaptability and reliability of RZBs under extreme conditions.
5. In practical use, Zn corrosion and hydrogen evolution will also occur slowly in a standing stage on the Zn anodes. To reflect their practicability, apart from repeated charge–discharge test, the self-discharge test and storage test should also be performed on RZBs to evaluate the long-term stability of the aqueous RZBs precisely.
Author Contributions
FM and NH wrote the manuscript. LC, ML, JZ, SY, and WW collected articles and contributed to the manuscript design and refine. JZ contributed to the manuscript revision. JW acquired the funding and revised the manuscript. All the authors collected and read the manuscript and contributed to manuscript writing.
Funding
The authors gratefully acknowledge the financial support by Shenzhen Municipality under the Project of Scientific Research Foundation for Returned Scholars (Grant No. DD11409018). This research was also sponsored by the Shenzhen Science and Technology Program (Grant No. KQTD20200820113045083) in Guangdong Province.
Conflict of Interest
The authors declare that the research was conducted in the absence of any commercial or financial relationships that could be construed as a potential conflict of interest.
Publisher’s Note
All claims expressed in this article are solely those of the authors and do not necessarily represent those of their affiliated organizations, or those of the publisher, the editors, and the reviewers. Any product that may be evaluated in this article, or claim that may be made by its manufacturer, is not guaranteed or endorsed by the publisher.
References
Amiinu, I. S., Pu, Z., Liu, X., Owusu, K. A., Monestel, H. G. R., Boakye, F. O., et al. (2017). Multifunctional Mo-N/C@MoS2 Electrocatalysts for HER, OER, ORR, and Zn-Air Batteries. Adv. Funct. Mater. 27, 1702300. doi:10.1002/adfm.201702300
Bayaguud, A., Fu, Y., and Zhu, C. (2022). Interfacial Parasitic Reactions of Zinc Anodes in Zinc Ion Batteries: Underestimated Corrosion and Hydrogen Evolution Reactions and Their Suppression Strategies. J. Energ. Chem. 64, 246–262. doi:10.1016/j.jechem.2021.04.016
Chamoun, M., Hertzberg, B. J., Gupta, T., Davies, D., Bhadra, S., Van Tassell, B., et al. (2015). Hyper-dendritic Nanoporous Zinc Foam Anodes. NPG Asia Mater. 7, e178. doi:10.1038/am.2015.32
Chao, D., Zhou, W., Ye, C., Zhang, Q., Chen, Y., Gu, L., et al. (2019). An Electrolytic Zn-MnO 2 Battery for High‐Voltage and Scalable Energy Storage. Angew. Chem. 131, 7905–7910. doi:10.1002/ange.201904174
Cui, Y., Zhao, Q., Wu, X., Chen, X., Yang, J., Wang, Y., et al. (2020). An Interface-Bridged Organic-Inorganic Layer that Suppresses Dendrite Formation and Side Reactions for Ultra-long-life Aqueous Zinc Metal Anodes. Angew. Chem. Int. Ed. Engl. 59, 16594–16601. doi:10.1002/anie.202005472
Deng, C., Xie, X., Han, J., Lu, B., Liang, S., and Zhou, J. (2021). Stabilization of Zn Metal Anode through Surface Reconstruction of a Cerium‐Based Conversion Film. Adv. Funct. Mater. 31, 2103227. doi:10.1002/adfm.202103227
Du, W., Ang, E. H., Yang, Y., Zhang, Y., Ye, M., and Li, C. C. (2020). Challenges in the Material and Structural Design of Zinc Anode towards High-Performance Aqueous Zinc-Ion Batteries. Energy Environ. Sci. 13, 3330–3360. doi:10.1039/D0EE02079F
Ghosh, M., Vijayakumar, V., and Kurungot, S. (2019). Dendrite Growth Suppression by Zn 2+ ‐Integrated Nafion Ionomer Membranes: Beyond Porous Separators toward Aqueous Zn/V 2 O 5 Batteries with Extended Cycle Life. Energy Technol. 7, 1900442. doi:10.1002/ente.201900442
Han, C., Zhang, T., Li, J., Li, B., and Lin, Z. (2020). Enabling Flexible Solid-State Zn Batteries via Tailoring Sulfur Deficiency in Bimetallic Sulfide Nanotube Arrays. Nano Energy 77, 105165. doi:10.1016/j.nanoen.2020.105165
Hiralal, P., Imaizumi, S., Unalan, H. E., Matsumoto, H., Minagawa, M., Rouvala, M., et al. (2010). Nanomaterial-Enhanced All-Solid Flexible Zinc−Carbon Batteries. ACS nano 4, 2730–2734. doi:10.1021/nn901391q
Huang, J., Chi, X., Han, Q., Liu, Y., Du, Y., Yang, J., et al. (2019). Thickening and Homogenizing Aqueous Electrolyte towards Highly Efficient and Stable Zn Metal Batteries. J. Electrochem. Soc. 166, A1211–A1216. doi:10.1149/2.1031906jes
Huang, J., and Yang, Z. (2015). A One-Pot Method to Prepare a ZnO/Ag/polypyrrole Composite for Zinc Alkaline Secondary Batteries. RSC Adv. 5, 33814–33817. doi:10.1039/C5RA01452B
Hurlbutt, K., Wheeler, S., Capone, I., and Pasta, M. (2018). Prussian Blue Analogs as Battery Materials. Joule 2, 1950–1960. doi:10.1016/j.joule.2018.07.017
Jia, H., Wang, Z., Tawiah, B., Wang, Y., Chan, C.-Y., Fei, B., et al. (2020). Recent Advances in Zinc Anodes for High-Performance Aqueous Zn-Ion Batteries. Nano Energy 70, 104523. doi:10.1016/j.nanoen.2020.104523
Jiao, Y., Li, F., Jin, X., Lei, Q., Li, L., Wang, L., et al. (2021). Engineering Polymer Glue towards 90% Zinc Utilization for 1000 hours to Make High‐Performance Zn‐Ion Batteries. Adv. Funct. Mater. 31, 2107652. doi:10.1002/adfm.202107652
Kang, L., Cui, M., Jiang, F., Gao, Y., Luo, H., Liu, J., et al. (2018). Nanoporous CaCO3 Coatings Enabled Uniform Zn Stripping/Plating for Long-Life Zinc Rechargeable Aqueous Batteries. Adv. Energ. Mater. 8, 1801090. doi:10.1002/aenm.201801090
Lee, C. W., Sathiyanarayanan, K., Eom, S. W., Kim, H. S., and Yun, M. S. (2006). Effect of Additives on the Electrochemical Behaviour of Zinc Anodes for Zinc/air Fuel Cells. J. Power Sourc. 160, 161–164. doi:10.1016/j.jpowsour.2006.01.070
Lee, S.-M., Kim, Y.-J., Eom, S.-W., Choi, N.-S., Kim, K.-W., and Cho, S.-B. (2013). Improvement in Self-Discharge of Zn Anode by Applying Surface Modification for Zn-Air Batteries with High Energy Density. J. Power Sourc. 227, 177–184. doi:10.1016/j.jpowsour.2012.11.046
Li, B., Xue, J., Han, C., Liu, N., Ma, K., Zhang, R., et al. (2021a). A Hafnium Oxide-Coated Dendrite-free Zinc Anode for Rechargeable Aqueous Zinc-Ion Batteries. J. Colloid Interf. Sci. 599, 467–475. doi:10.1016/j.jcis.2021.04.113
Li, B., Zhang, X., Wang, T., He, Z., Lu, B., Liang, S., et al. (2022). Interfacial Engineering Strategy for High-Performance Zn Metal Anodes. Nano-micro Lett. 14, 1–31. doi:10.1007/s40820-021-00764-7
Li, C., Shi, X., Liang, S., Ma, X., Han, M., Wu, X., et al. (2020b). Spatially Homogeneous Copper Foam as Surface Dendrite-free Host for Zinc Metal Anode. Chem. Eng. J. 379, 122248. doi:10.1016/j.cej.2019.122248
Li, C., Xie, X., Liang, S., and Zhou, J. (2020a). Issues and Future Perspective on Zinc Metal Anode for Rechargeable Aqueous Zinc‐ion Batteries. Energy Environ. Mater. 3, 146–159. doi:10.1002/eem2.12067
Li, C., Xie, X., Liu, H., Wang, P., Deng, C., Lu, B., et al. (2021b). Integrated ‘all-In-One’strategy to Stabilize Zinc Anodes for High-Performance Zinc-Ion Batteries. Natl. Sci. Rev., nwab177. doi:10.1093/nsr/nwab177
Li, H., Ma, L., Han, C., Wang, Z., Liu, Z., Tang, Z., et al. (2019). Advanced Rechargeable Zinc-Based Batteries: Recent Progress and Future Perspectives. Nano Energy 62, 550–587. doi:10.1016/j.nanoen.2019.05.059
Li, Q., Pan, H., Li, W., Wang, Y., Wang, J., Zheng, J., et al. (2018). Homogeneous Interface Conductivity for Lithium Dendrite-free Anode. ACS Energ. Lett. 3, 2259–2266. doi:10.1021/acsenergylett.8b01244
Li, Q., Zhao, Y., Mo, F., Wang, D., Yang, Q., Huang, Z., et al. (2020c). Dendrites Issues and Advances in Zn Anode for Aqueous Rechargeable Zn‐based Batteries. EcoMat 2, e12035. doi:10.1002/eom2.12035
Liang, J., Chen, Q., Liao, X., Yao, P., Zhu, B., Lv, G., et al. (2020). A Nano‐shield Design for Separators to Resist Dendrite Formation in Lithium‐Metal Batteries. Angew. Chem. Int. Ed. 59, 6561–6566. doi:10.1002/anie.201915440
Ling, W., Mo, F., Wang, J., Liu, Q., Liu, Y., Yang, Q., et al. (2021). Self-Healable Hydrogel Electrolyte for Dendrite-free and Self-Healable Zinc-Based Aqueous Batteries. Mater. Today Phys. 20, 100458. doi:10.1016/j.mtphys.2021.100458
Liu, S., Kang, L., Kim, J. M., Chun, Y. T., Zhang, J., and Jun, S. C. (2020). Recent Advances in Vanadium‐Based Aqueous Rechargeable Zinc‐Ion Batteries. Adv. Energ. Mater. 10, 2000477. doi:10.1002/aenm.202000477
Liu, Z., Wang, D., Tang, Z., Liang, G., Yang, Q., Li, H., et al. (2019). A Mechanically Durable and Device-Level Tough Zn-MnO2 Battery with High Flexibility. Energ. Storage Mater. 23, 636–645. doi:10.1016/j.ensm.2019.03.007
Lu, W., Xie, C., Zhang, H., and Li, X. (2018). Inhibition of Zinc Dendrite Growth in Zinc‐Based Batteries. ChemSusChem 11, 3996–4006. doi:10.1002/cssc.201801657
Ma, L., Chen, S., Li, N., Liu, Z., Tang, Z., Zapien, J. A., et al. (2020). Hydrogen‐Free and Dendrite‐Free All‐Solid‐State Zn‐Ion Batteries. Adv. Mater. 32, 1908121. doi:10.1002/adma.201908121
Mitha, A., Yazdi, A. Z., Ahmed, M., and Chen, P. (2018). Surface Adsorption of Polyethylene Glycol to Suppress Dendrite Formation on Zinc Anodes in Rechargeable Aqueous Batteries. ChemElectroChem 5, 2409–2418. doi:10.1002/celc.201800572
Mo, F., Chen, Z., Liang, G., Wang, D., Zhao, Y., Li, H., et al. (2020). Zwitterionic Sulfobetaine Hydrogel Electrolyte Building Separated Positive/Negative Ion Migration Channels for Aqueous Zn‐MnO 2 Batteries with Superior Rate Capabilities. Adv. Energ. Mater. 10, 2000035. doi:10.1002/aenm.202000035
Nady, H. (2017). Tricine [ N- ( Tri ( Hydroxymethyl ) Methyl ) glycine ] - A Novel green Inhibitor for the Corrosion Inhibition of Zinc in Neutral Aerated Sodium Chloride Solution. Egypt. J. Pet. 26, 905–913. doi:10.1016/j.ejpe.2016.02.004
Olbasa, B. W., Fenta, F. W., Chiu, S.-F., Tsai, M.-C., Huang, C.-J., Jote, B. A., et al. (2020). High-Rate and Long-Cycle Stability with a Dendrite-free Zinc Anode in an Aqueous Zn-Ion Battery Using Concentrated Electrolytes. ACS Appl. Energ. Mater. 3, 4499–4508. doi:10.1021/acsaem.0c00183
Pan, C., Zhang, R., Nuzzo, R. G., and Gewirth, A. A. (2018). ZnNi X Mn X Co 2-2 X O 4 Spinel as a High‐Voltage and High‐Capacity Cathode Material for Nonaqueous Zn‐Ion Batteries. Adv. Energ. Mater. 8, 1800589. doi:10.1002/aenm.201800589
Pan, H., Shao, Y., Yan, P., Cheng, Y., Han, K. S., Nie, Z., et al. (2016). Reversible Aqueous Zinc/manganese Oxide Energy Storage from Conversion Reactions. Nat. Energ. 1, 1–7. doi:10.1038/nenergy.2016.39
Parker, J. F., Chervin, C. N., Pala, I. R., Machler, M., Burz, M. F., Long, J. W., et al. (2017). Rechargeable nickel-3D Zinc Batteries: An Energy-Dense, Safer Alternative to Lithium-Ion. Science 356, 415–418. doi:10.1126/science.aak9991
Qiu, H., Du, X., Zhao, J., Wang, Y., Ju, J., Chen, Z., et al. (2019). Zinc Anode-Compatible Iin-Ssitu Solid Electrolyte Interphase via Cation Solvation Modulation. Nat. Commun. 10, 5374. doi:10.1038/s41467-019-13436-3
Rui, M., Jiang, Y., and Zhu, A. (2020). Sub-micron Calcium Carbonate as a Template for the Preparation of Dendrite-like PANI/CNT Nanocomposites and its Corrosion protection Properties. Chem. Eng. J. 385, 123396. doi:10.1016/j.cej.2019.123396
Shan, L., Wang, Y., Liang, S., Tang, B., Yang, Y., Wang, Z., et al. (2021). Interfacial Adsorption-Insertion Mechanism Induced by Phase Boundary toward Better Aqueous Zn‐ion Battery. InfoMat 3, 1028–1036. doi:10.1002/inf2.12223
Shivkumar, R., Kalaignan, G. P., and Vasudevan, T. (1998). Studies with Porous Zinc Electrodes with Additives for Secondary Alkaline Batteries. J. Power Sourc. 75, 90–100. doi:10.1016/S0378-7753(98)00096-2
Sun, K. E. K., Hoang, T. K. A., Doan, T. N. L., Yu, Y., Zhu, X., Tian, Y., et al. (2017). Suppression of Dendrite Formation and Corrosion on Zinc Anode of Secondary Aqueous Batteries. ACS Appl. Mater. Inter. 9, 9681–9687. doi:10.1021/acsami.6b16560
Tang, B., Shan, L., Liang, S., and Zhou, J. (2019). Issues and Opportunities Facing Aqueous Zinc-Ion Batteries. Energ. Environ. Sci. 12, 3288–3304. doi:10.1039/C9EE02526J
Trócoli, R., and La Mantia, F. (2015). An Aqueous Zinc-Ion Battery Based on Copper Hexacyanoferrate. ChemSusChem 8, 481–485. doi:10.1002/cssc.201403143
Wan, F., Zhang, L., Wang, X., Bi, S., Niu, Z., and Chen, J. (2018). An Aqueous Rechargeable Zinc-Organic Battery with Hybrid Mechanism. Adv. Funct. Mater. 28, 1804975. doi:10.1002/adfm.201804975
Wan, F., Zhang, Y., Zhang, L., Liu, D., Wang, C., Song, L., et al. (2019). Reversible Oxygen Redox Chemistry in Aqueous Zinc‐Ion Batteries. Angew. Chem. Int. Ed. 58, 7062–7067. doi:10.1002/anie.201902679
Wang, G., Kohn, B., Scheler, U., Wang, F., Oswald, S., Löffler, M., et al. (2020). A High‐Voltage, Dendrite‐Free, and Durable Zn-Graphite Battery. Adv. Mater. 32, 1905681. doi:10.1002/adma.201905681
Wang, L., Zhang, Y., Hu, H., Shi, H.-Y., Song, Y., Guo, D., et al. (2019). A Zn(ClO4)2 Electrolyte Enabling Long-Life Zinc Metal Electrodes for Rechargeable Aqueous Zinc Batteries. ACS Appl. Mater. Inter. 11, 42000–42005. doi:10.1021/acsami.9b10905
Wang, R., Yang, Z., Yang, B., Wang, T., and Chu, Z. (2014). Superior Cycle Stability and High Rate Capability of Zn-Al-In-Hydrotalcite as Negative Electrode Materials for Ni-Zn Secondary Batteries. J. Power Sourc. 251, 344–350. doi:10.1016/j.jpowsour.2013.11.071
Wu, K., Huang, J., Yi, J., Liu, X., Liu, Y., Wang, Y., et al. (2020). Recent Advances in Polymer Electrolytes for Zinc Ion Batteries: Mechanisms, Properties, and Perspectives. Adv. Energ. Mater. 10, 1903977. doi:10.1002/aenm.201903977
Xie, C., Li, Y., Wang, Q., Sun, D., Tang, Y., and Wang, H. (2020b). Issues and Solutions toward Zinc Anode in Aqueous Zinc‐ion Batteries: A Mini Review. Carbon Energy 2, 540–560. doi:10.1002/cey2.67
Xie, X., Liang, S., Gao, J., Guo, S., Guo, J., Wang, C., et al. (2020a). Manipulating the Ion-Transfer Kinetics and Interface Stability for High-Performance Zinc Metal Anodes. Energ. Environ. Sci. 13, 503–510. doi:10.1039/C9EE03545A
Yang, C. P., Yin, Y. X., Zhang, S. F., Li, N. W., and Guo, Y. G. (2015). Accommodating Lithium into 3D Current Collectors with a Submicron Skeleton towards Long-Life Lithium Metal Anodes. Nat. Commun. 6, 8058–8059. doi:10.1038/ncomms9058
Yang, H., Chang, Z., Qiao, Y., Deng, H., Mu, X., He, P., et al. (2020b). Constructing a Super‐Saturated Electrolyte Front Surface for Stable Rechargeable Aqueous Zinc Batteries. Angew. Chem. Int. Ed. 59, 9377–9381. doi:10.1002/anie.202001844
Yang, Q., Li, Q., Liu, Z., Wang, D., Guo, Y., Li, X., et al. (2020a). Dendrites in Zn‐Based Batteries. Adv. Mater. 32, 2001854. doi:10.1002/adma.202001854
Yang, Q., Liang, G., Guo, Y., Liu, Z., Yan, B., Wang, D., et al. (2019). Do Zinc Dendrites Exist in Neutral Zinc Batteries: A Developed Electrohealing Strategy to In Situ Rescue In‐Service Batteries. Adv. Mater. 31, 1903778. doi:10.1002/adma.201903778
Yi, Z., Chen, G., Hou, F., Wang, L., and Liang, J. (2021). Strategies for the Stabilization of Zn Metal Anodes for Zn‐Ion Batteries. Adv. Energ. Mater. 11, 2003065. doi:10.1002/aenm.202003065
Yuan, D., Manalastas, W., Zhang, L., Chan, J. J., Meng, S., Chen, Y., et al. (2019). Lignin@Nafion Membranes Forming Zn Solid-Electrolyte Interfaces Enhance the Cycle Life for Rechargeable Zinc‐Ion Batteries. ChemSusChem 12, 4889–4900. doi:10.1002/cssc.201901409
Zeng, X., Hao, J., Wang, Z., Mao, J., and Guo, Z. (2019b). Recent Progress and Perspectives on Aqueous Zn-Based Rechargeable Batteries with Mild Aqueous Electrolytes. Energ. Storage Mater. 20, 410–437. doi:10.1016/j.ensm.2019.04.022
Zeng, Y., Zhang, X., Qin, R., Liu, X., Fang, P., Zheng, D., et al. (2019a). Dendrite‐Free Zinc Deposition Induced by Multifunctional CNT Frameworks for Stable Flexible Zn‐Ion Batteries. Adv. Mater. 31, 1903675. doi:10.1002/adma.201903675
Zhang, C., Holoubek, J., Wu, X., Daniyar, A., Zhu, L., Chen, C., et al. (2018). A ZnCl2 Water-In-Salt Electrolyte for a Reversible Zn Metal Anode. Chem. Commun. 54, 14097–14099. doi:10.1039/C8CC07730D
Zhang, G., Zhang, X., Liu, H., Li, J., Chen, Y., and Duan, H. (2021). 3D‐Printed Multi‐Channel Metal Lattices Enabling Localized Electric‐Field Redistribution for Dendrite‐Free Aqueous Zn Ion Batteries. Adv. Energ. Mater. 11, 2003927. doi:10.1002/aenm.202003927
Zhang, N., Cheng, F., Liu, Y., Zhao, Q., Lei, K., Chen, C., et al. (2016). Cation-Deficient Spinel ZnMn2O4 Cathode in Zn(CF3SO3)2 Electrolyte for Rechargeable Aqueous Zn-Ion Battery. J. Am. Chem. Soc. 138, 12894–12901. doi:10.1021/jacs.6b05958
Zhang, Q., Luan, J., Fu, L., Wu, S., Tang, Y., Ji, X., et al. (2019). The Three‐Dimensional Dendrite‐Free Zinc Anode on a Copper Mesh with a Zinc‐Oriented Polyacrylamide Electrolyte Additive. Angew. Chem. 131, 15988–15994. doi:10.1002/ange.201907830
Zhang, Q., Luan, J., Huang, X., Zhu, L., Tang, Y., Ji, X., et al. (2020). Simultaneously Regulating the Ion Distribution and Electric Field to Achieve Dendrite‐Free Zn Anode. Small 16, 2000929. doi:10.1002/smll.202000929
Zhao, J., Zhang, J., Yang, W., Chen, B., Zhao, Z., Qiu, H., et al. (2019a). "Water-in-deep Eutectic Solvent" Electrolytes Enable Zinc Metal Anodes for Rechargeable Aqueous Batteries. Nano Energy 57, 625–634. doi:10.1016/j.nanoen.2018.12.086
Zhao, K., Wang, C., Yu, Y., Yan, M., Wei, Q., He, P., et al. (2018b). Ultrathin Surface Coating Enables Stabilized Zinc Metal Anode. Adv. Mater. Inter. 5, 1800848. doi:10.1002/admi.201800848
Zhao, T., Zhang, G., Zhou, F., Zhang, S., and Deng, C. (2018a). Toward Tailorable Zn-Ion Textile Batteries with High Energy Density and Ultrafast Capability: Building High-Performance Textile Electrode in 3D Hierarchical Branched Design. Small 14, 1802320. doi:10.1002/smll.201802320
Zhao, Z., Zhao, J., Hu, Z., Li, J., Li, J., Zhang, Y., et al. (2019b). Long-life and Deeply Rechargeable Aqueous Zn Anodes Enabled by a Multifunctional Brightener-Inspired Interphase. Energ. Environ. Sci. 12, 1938–1949. doi:10.1039/C9EE00596J
Zheng, J., Zhao, Q., Tang, T., Yin, J., Quilty, C. D., Renderos, G. D., et al. (2019). Reversible Epitaxial Electrodeposition of Metals in Battery Anodes. Science 366, 645–648. doi:10.1126/science.aax6873
Keywords: rechargeable Zn-based batteries, Zn anode, stabilization, dendrites, hydrogen evolution
Citation: Mo F, He N, Chen L, Li M, Yu S, Zhang J, Wang W and Wei J (2022) Strategies for Stabilization of Zn Anodes for Aqueous Zn-Based Batteries: A Mini Review. Front. Chem. 9:822624. doi: 10.3389/fchem.2021.822624
Received: 26 November 2021; Accepted: 28 December 2021;
Published: 09 February 2022.
Edited by:
Xiaolei Wang, University of Alberta, CanadaReviewed by:
Chuanxin He, Shenzhen University, ChinaJiang Zhou, Central South University, China
Xiao Liang, Hunan University, China
Copyright © 2022 Mo, He, Chen, Li, Yu, Zhang, Wang and Wei. This is an open-access article distributed under the terms of the Creative Commons Attribution License (CC BY). The use, distribution or reproduction in other forums is permitted, provided the original author(s) and the copyright owner(s) are credited and that the original publication in this journal is cited, in accordance with accepted academic practice. No use, distribution or reproduction is permitted which does not comply with these terms.
*Correspondence: Jiaolong Zhang, amlhb2xvbmdfemhhbmdAMTYzLmNvbQ==; Jun Wei, anVud2VpQGhpdC5lZHUuY24=
†These authors have contributed equally to this work