- 1College of Information Science and Engineering, Shandong Agricultural University, Taian, China
- 2College of Mechanical and Electronic Engineering, Shandong Agricultural University, Taian, China
- 3College of Physics, University-Industry Joint Center for Ocean Observation and Broadband Communication, Qingdao University, Qingdao, China
Nickel sulfide has been widely studied as an anode material for lithium-ion batteries due to its environmental friendliness, low cost, high conductivity, and high theoretical capacity. A simple hydrothermal method was used to prepare NiS nanospheres materials with the size in the range of 100–500 nm. The NiS nanospheres electrodes exhibited a high reversible capacity of 1402.3 mAh g−1 at 200 mA g−1 after 280 cycles and a strong rate capability of 814.8 mAh g−1 at 0.8 A g−1 and 1130.5 mAh g−1 when back to 0.1 A g−1. Excellent electrochemical properties and the simple preparation method of the NiS nanospheres make it possible to prepare NiS on a large scale as the anode of lithium-ion batteries.
Introduction
With the gradual exhaustion of fossil energy and the resulting emission of carbon oxides, the development of new green and sustainable energy, such as wind energy and solar energy, presents a strong trend, which puts forward higher requirements for energy storage and conversion technology (Li S. et al., 2021). As new energy storage devices, lithium-ion batteries (LIBs) have been widely studied due to their long service life, no memory effect, high charging efficiency, and environmental friendliness (Hong and Song, 2018; Hou et al., 2020; Li W. et al., 2020; Teng et al., 2020; Zheng et al., 2020; Gao et al., 2021; Li H. et al., 2021; Li Z. et al., 2021; Wang L et al., 2021). Since LIBs were commercialized by Sony in 1991, graphite has been the main anode of LIBs for a long period (Park and Lee, 2019). Although graphite has high conductivity and good cycling stability, its poor rate performance and low theoretical capacity will limit its application and development in the future (Dong et al., 2019; Ren et al., 2021; Xia et al., 2021).
Nanomaterials have shown excellent physical and chemical properties in many fields due to their larger specific surface area and higher activity (Wang et al., 2014; Wang et al., 2017; Li H. et al., 2019; Zhou et al., 2019; Gu et al., 2021; Wang X et al., 2021; Zhao et al., 2021; Liang et al., 2022; Wang et al., 2022). Transition metal sulfide (TMS) nanomaterials have been extensively researched in the field of the anode materials of LIBs due to low redox potential, good conductivity, strong cycling stability, and high theoretical capacity (Zhao et al., 2018; Wang et al., 2020; Yang et al., 2021). Among them, NiS is an excellent choice to replace graphite anodes because of its good stability, high theoretical capacity (590 mAh g−1), and high conductivity (Ren et al., 2021). Recently, Lee et al. synthesized hierarchical carbon-coated NiS with a discharge capacity of 606 mAh g−1 after 100 cycles (Park and Lee, 2019). Gao et al. prepared porous NiS@NSC tubules using biological templates, which showed a discharge capacity of 715.9 mAh g−1 at the 200th cycle (Dong et al., 2019). Wang et al. prepared NiS/C nanomaterials with biomass biochar, exhibiting a reversible capacity of 411.6 mAh g−1 at the 100th cycle (Xia et al., 2021). However, most NiS nanomaterials have not shown the satisfying reversible capacity and cycle stability, and complex preparations restricted its mass production.
In this work, NiS nanospheres were prepared by a hydrothermal method and showed outstanding performance as anodes for LIBs. The initial discharge and charge capacities reached 1418.5 mAh g−1 and 778.3 mAh g−1 at 0.2 A g−1, respectively. The reversible capacity was up to 1402.3 mAh g−1 after 280 charging-discharging cycles. The discharge specific capacity of NiS nanospheres reached 814.8 mAh g−1 at 0.8 A g−1, and it increased to 1130.5 mAh g−1 when back to 0.1 A g−1, indicating an enhanced rate capability. The outstanding electrochemical performance indicated that the NiS nanosphere materials are more potential anodes for LIBs.
Methods of Preparation and Characterization
Preparation of NiS Nanospheres
The schematic diagram of the preparation process of NiS nanospheres is shown in Figure 1. There were 244.85 mg (2 mmol) of L-cysteine and 475.38 mg (2 mmol) of NiCl2·6H2O added into ethylene glycol (35 ml) and stirred by a magnetic stirrer for more than 2 h. The mixture was transferred to a Teflon-sealed autoclave and thoroughly reacted for 24 h at 200°C. The black powder samples were acquired after alternately washing 6 times with deionized water and ethanol in a centrifuge (10,000 rpm for 10 min) and drying at 70°C in a vacuum for 12 h.
Characterization of NiS Nanospheres
X-ray diffraction (XRD, SmartLab, Rigku Japan) and scanning electron microscope (SEM, GeminiSEM300, Zeiss, Germany) were used to characterize the composition, structure, and morphology of the black powder. The scan rate of the Cu Кα radiation is 5°/min in the range of 20°–80° for the XRD measurements.
Electrochemical Test of NiS Nanospheres
The anodes were made of carboxymethyl cellulose (CMC), carbon black, and NiS nanospheres powders (mass ratio 7:2:1). After coating the mixed paste on copper foil uniformly, the copper foil was dried for 12 h at 70°C under vacuum and cut into discs (113 mm2). The CR-2032 cells were assembled in argon with Celgard 2250 films used as the diaphragm, 1M LiPF6 solution with dimethyl ethyl carbonate and ethyl carbonate (v/v = 1:1) used as the electrolyte, and lithium disks used as counter-electrodes.
Land-CT3001A battery testing systems were used for the cycle performance test of NiS nanospheres at several different current densities. The electrochemical impedance spectroscopy (EIS, 10−2–105 Hz) and the cyclic voltammetry (CV, 0.1–1.5 mV s−1) curves were determined by a CHI660E electrochemical workstation. The electrochemical tests were realized at room temperature between 0.01 and 3.0 V.
Results and Discussion
Structure and Morphology of NiS Nanospheres
The XRD patterns of the materials shown in Figure 2A have a high degree of matching with the standard card PDF No. 02-1280, which shows that the sample is pure NiS and no other components exist. The sharp diffraction peaks indicate that the samples are crystalline. In addition, the different peaks at 30.167°, 34.742°, 46.034°, 53.546°, and 73.327°, respectively, corresponded to the (100), (101), (102), (110), and (202) crystal planes of NiS. To further investigate the morphology, the NiS material was tested by SEM. The materials consisted of nanospheres with sizes between 100 and 500 nm as shown in Figure 2B.
Electrochemical Performance of NiS Nanospheres
The initial five CV curves were measured at 0.1 mV s−1, as is shown in Figure 3A. In CV curves, three reduction peaks existed near 0.94, 1.32, and 1.58 V during the first cathode sweep (lithiation). The peak at 0.94 V denotes the formation of the solid electrolyte interphase (SEI) layer, which can be seen from the fact that this peak no longer exists in the second circle (Duan et al., 2015; Fan et al., 2017; Jin et al., 2017; Dong et al., 2019). The reduction peaks at 1.58 and 1.32 V represent the reduction process from NiS to Ni, which corresponds to the two reactions of Equations 1 and 2, respectively (Wang et al., 2011; Dong et al., 2019; Xia et al., 2021).
In the second cycle, the two peaks shifted to 1.74 and 1.27 V, while in the third cycle, they shifted to 1.77 and 1.35 V, which was due to the activation of the materials (Ni et al., 2012). The two reduction peaks in subsequent cycles almost completely coincide with that in the third cycle, which indicates the stable reduction reaction process.
Two oxidation peaks can be observed near 1.46 and 2.17 V during the first anode sweep (delithiation). The peak at 1.46 V, which vanishes in following cycles, represents the decomposition of the SEI layer (Vadivazhagan et al., 2021; Xia et al., 2021), and the peak at 2.17 V relates to the reaction of Ni to NiS (Vadivazhagan et al., 2021), which corresponds to Equation 3 (Vadivazhagan et al., 2021).
The oxidation peak at 2.17 V split into three peaks at 1.99, 2.14, and 2.27 V in the second cycle, which also represents the production of NiS (Han et al., 2017; Ren et al., 2021; Xia et al., 2021). In the subsequent cycles, the peak positions of the oxidation processes almost completely coincide with that in the second cycle, which shows the stable oxidation reaction process and cycle stability.
The initial five constant current discharge-charge curves of NiS nanospheres were measured at 100 mA g−1, as shown in Figure 3B. There is a small platform between 1.6 and 1.7 V and a large platform between 1.0 and 1.5 V during the first discharge process. The small platform corresponds to the reduction process of NiS to Ni3S2, while the large platform signifies the formation of the SEI layer as well as the reduction process of Ni3S2 to Ni, which completely corresponds to the CV curves. There is a small platform near 1.4 V and a large platform near 2.0 V in the first charge process, which corresponds very well to the oxidation peaks in the CV curves. As shown in Figure 3B, the discharge and charge capacities of the first cycle are 1949 mAh g−1 and 1257 mAh g−1 respectively. The discharge and charge capacities can be stable around 1200 mAh g−1 in the following four cycles, which are much higher than the theoretical capacity of NiS (Li Q. et al., 2020; Kim et al., 2020). The phenomenon could originate from the reversible formation of polymeric gel-like films around the transition metal particles (Laruelle et al., 2002), the interface lithium storage (Zhukovskii et al., 2006), and the surface conversion of LiOH to Li2O and LiH (Hu et al., 2013). It is worth noting that the discharge-charge curves almost completely coincide after the first circle, which shows the great electrochemical stability and reversibility.
The cycle performance and the rate capabilities of NiS nanospheres were measured, as shown in Figure 4. The initial discharge and charge capacities at 200 mA g−1 are up to 1418.5 mAh g−1 and 778.3 mAh g−1, respectively. The initial Coulomb efficiency is 54.9% and increases rapidly to more than 90% in the second cycle, and then remains near 100% to the 280th cycle. The cycle curve has two upward trends. The first upward trend during the initial 20 cycles could be ascribed to the active process of NiS nanospheres in the first few redox reaction cycles (Li L. et al., 2019; Li S. et al., 2019), and the second upward trend during the 160th to 280th cycle could be due to the increase of active sites caused by the rupture of the NiS nanospheres during cycles (Duan et al., 2015; Wang et al., 2020, Wang L et al., 2021) and/or the reversible growth of a polymeric gel-like film resulting from the kinetically activated electrolyte degradation (Feng et al., 2013; Rui et al., 2014; Duan et al., 2015). After 280 discharge-charge cycles, the high specific capacity of 1402.3 mAh g−1 is obtained, which shows that the NiS nanosphere materials have good reversibility and stability as the anode of LIBs. The comparison of electrochemical properties between this work and other NiS-based electrode materials is shown in Table 1, which shows the outstanding electrochemical performance of the NiS nanospheres materials.
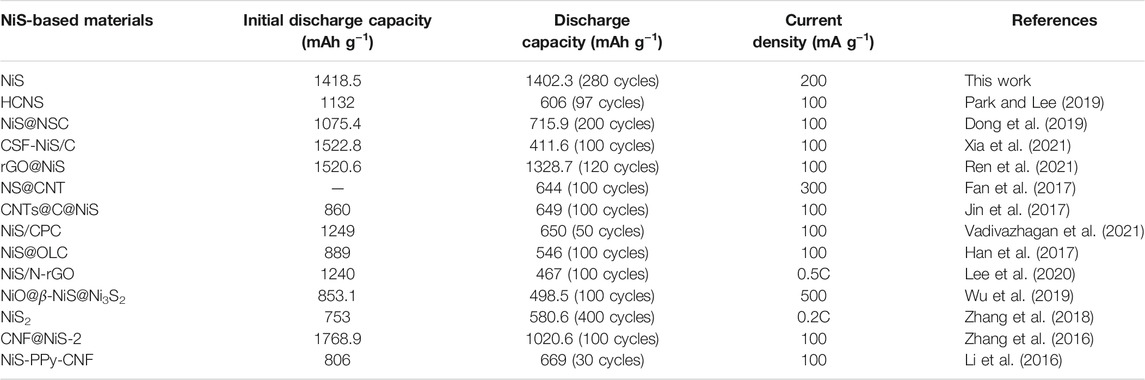
TABLE 1. Comparison of electrochemical properties between NiS2 nanospheres and other reported NiS-based materials.
It can be seen from Figure 4B that the specific capacities are 1224.3 mAh g−1, 1157.1 mAh g−1, 1003.6 mAh g−1, and 814.8 mAh g−1 at 0.1 A g−1, 0.2 A g−1, 0.5 A g−1, and 0.8 A g−1, respectively. The specific capacity returns to 1130.5 mAh g−1 when back to 0.1 A g−1, which shows that the NiS nanosphere electrodes have good reversibility and stability.
Kinetics Characterizations of NiS Nanospheres
To study the kinetic characteristics of NiS nanospheres as anodes of LIBs, the EIS of NiS nanospheres electrodes was measured before and after the cycle test, as shown in Figure 5A. The Nyquist curves are both made up of a straight line and two semicircles. The intercept denotes the resistance of the electrolyte and the electrode (Rs), the diameters of the small and large semicircles denote the resistance of the SEI layer to lithium-ions migration (Rcf) and the charge transfer resistance (Rct), respectively. It is obvious from Figure 5A that both the diameters before the cycle test are much larger than those after the cycle test, which shows that electrons and lithium-ions can move more easily and the NiS nanospheres electrodes have good conductivity during cycles. The insert of Figure 5A shows the equivalent circuit of the EIS curves, and all the parameters can be quantitatively fitted by it. The fitted values of Rs, Rcf, and Rct are shown in Table 2. Rs changes a little before and after the cycle test, but Rcf and Rct decrease significantly after the cycle test, which is more conducive to improving reversible capacity and cycle stability (Teng et al., 2020; Ren et al., 2021).
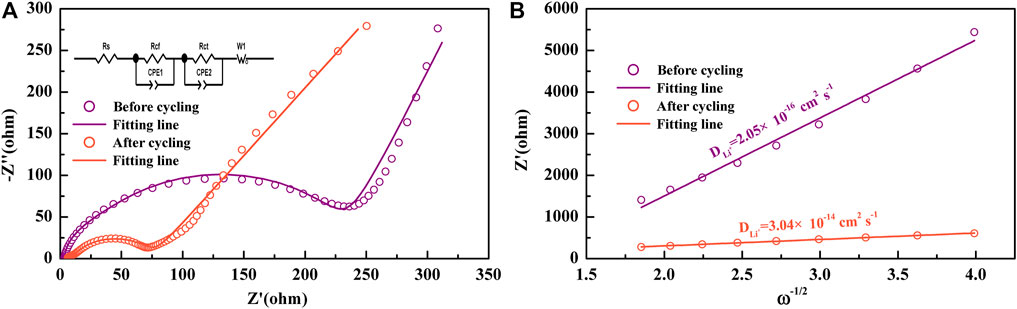
FIGURE 5. (A) EIS of NiS nanosphere electrodes before and after cycles; the inset is the equivalent circuit. (B)
The diffusion coefficient of lithium-ions can be obtained by the following equations (Chen et al., 2018; Teng et al., 2020):
where R represents the gas constant, T represents the thermodynamic temperature, here is the room temperature, A represents the surface area of the electrode, n stands for the number of electrons transferred in the oxidation or reduction reaction per molecule, f represents the Faraday constant, C represents the concentration of lithium-ions, and
To further study the dynamic characteristics of the NiS nanospheres, CV curves at different sweep rates were measured. The shapes of CV curves in Figure 6A are very similar, only the intensities of the peaks increase with the increase of sweep rates, which shows that NiS nanosphere electrodes have good cyclic reversibility in the process of lithium and delithium (Ren et al., 2021). The contribution proportions of ion diffusion and capacitance effect in the cycles at different sweep rates can be roughly estimated by the following two equations (Ge et al., 2018; Li W. et al., 2020).
where i represents the peak current, and v represents the sweep rate. It indicates that the charge-discharge process is dominated by ion diffusion when b approaches 0.5, while when b approaches 1, the charge-discharge process mainly depends on the capacitance effect. The b values corresponding to the three peaks of redox reactions are 0.82, 0.71, and 0.75, respectively, as shown in Figure 6B, which shows that the current is the result of the joint action of ion diffusion and capacitance effect in these three reaction processes.
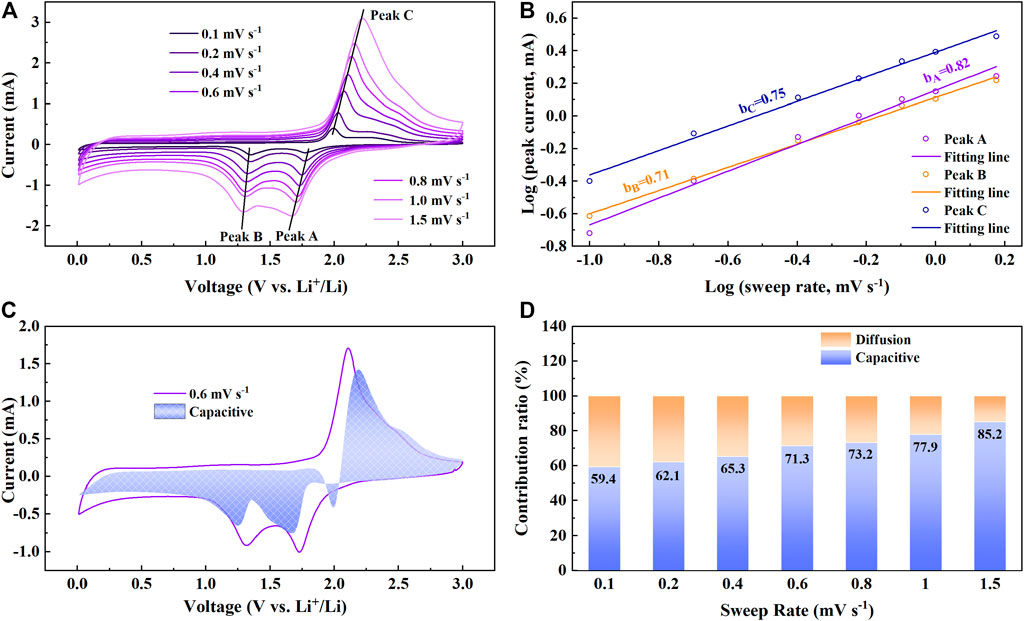
FIGURE 6. (A) CV curves at different sweep rates. (B) The corresponding plots of log(i) vs. log(v) at three redox peaks. (C) The CV curves and the voltage distribution (shaded part) at the sweep rate of 0.6 mV s−1. (D) Contribution ratio of capacitive at various sweep rates.
To further research the role of capacitance effect in NiS nanospheres electrodes, the following equation can be used to quantitatively calculate the current contribution proportion of capacitance effect (Wang et al., 2007; Ge et al., 2018).
where
Conclusion
In this paper, NiS nanospheres were successfully synthesized by a simple hydrothermal method. The NiS nanosphere materials used as the anode of LIBs are not only simple to manufacture but also show outstanding electrochemical performance. After 280 charging-discharging circles, a high reversible specific capacity of 1402.3 mAh g−1 at 200 mA g−1 was obtained. In addition, the NiS nanosphere electrodes display a good rate capability. The reversible capacity still reaches 814.8 mAh g−1 even at 0.8 A g−1. In addition, the reversible capacity can still reach 1130.5 mAh g−1 when back to 0.1 A g−1. Furthermore, the conductivity after the cycle test is higher than that before the cycle test, which may be one of the reasons why the reversible capacity of NiS nanosphere electrodes increases during cycling. The proportion of capacitance contribution reaches 85.2% at 1.5 mV s−1, showing a strong rate capability of NiS nanospheres electrodes. The outstanding cycling stability and rate capability indicated that the NiS nanosphere materials are more promising anodes for LIBs.
Data Availability Statement
The raw data supporting the conclusion of this article will be made available by the authors, without undue reservation.
Author Contributions
All authors listed have made a substantial, direct, and intellectual contribution to the manuscript writing, editing, and reviewing work, and approved it for publication.
Funding
This work was funded by the National Natural Science Foundation of China No. 22179066, and the Project of Shandong Province Higher Educational Science and Technology Program (No. J17KA184).
Conflict of Interest
The authors declare that the research was conducted in the absence of any commercial or financial relationships that could be construed as a potential conflict of interest.
Publisher’s Note
All claims expressed in this article are solely those of the authors and do not necessarily represent those of their affiliated organizations, or those of the publisher, the editors, and the reviewers. Any product that may be evaluated in this article, or claim that may be made by its manufacturer, is not guaranteed or endorsed by the publisher.
References
Chen, H., Zhang, B., Wang, X., Dong, P., Tong, H., Zheng, J.-c., et al. (2018). CNT-decorated Na3V2(PO4)3 Microspheres as a High-Rate and Cycle-Stable Cathode Material for Sodium Ion Batteries. ACS Appl. Mater. Inter. 10, 3590–3595. doi:10.1021/acsami.7b16402
Dong, X., Deng, Z.-P., Huo, L.-H., Zhang, X.-F., and Gao, S. (2019). Large-Scale Synthesis of NiS@N and S Co-doped Carbon Mesoporous Tubule as High Performance Anode for Lithium-Ion Battery. J. Alloys Compounds. 788, 984–992. doi:10.1016/j.jallcom.2019.02.326
Duan, W., Yan, W., Yan, X., Munakata, H., Jin, Y., and Kanamura, K. (2015). Synthesis of Nanostructured Ni3S2 with Different Morphologies as Negative Electrode Materials for Lithium Ion Batteries. J. Power Sourc. 293, 706–711. doi:10.1016/j.jpowsour.2015.05.098
Fan, P., Liu, H., Liao, L., Fu, J., Wang, Z., Lv, G., et al. (2017). Flexible and High Capacity Lithium-Ion Battery Anode Based on a Carbon Nanotube/Electrodeposited Nickel Sulfide Paper-like Composite. RSC Adv. 7, 49739–49744. doi:10.1039/c7ra08239h
Feng, N., Hu, D., Wang, P., Sun, X., Li, X., and He, D. (2013). Growth of Nanostructured Nickel Sulfide Films on Ni Foam as High-Performance Cathodes for Lithium Ion Batteries. Phys. Chem. Chem. Phys. 15, 9924–9930. doi:10.1039/c3cp50615k
Gao, M., Zhou, W.-Y., Mo, Y.-X., Sheng, T., Deng, Y., Chen, L., et al. (2021). Outstanding Long-Cycling Lithium−Sulfur Batteries by Core-Shell Structure of S@Pt Composite with Ultrahigh Sulfur Content. Adv. Powder Mater. doi:10.1016/j.apmate.2021.09.006
Ge, P., Hou, H., Li, S., Huang, L., and Ji, X. (2018). Three-Dimensional Hierarchical Framework Assembled by Cobblestone-Like CoSe2@C Nanospheres for Ultrastable Sodium-Ion Storage. ACS Appl. Mater. Inter. 10, 14716–14726. doi:10.1021/acsami.8b01888
Gu, Z. Y., Guo, J. Z., Zhao, X. X., Wang, X. T., Xie, D., Sun, Z. H., et al. (2021). High‐ionicity Fluorophosphate Lattice via Aliovalent Substitution as Advanced Cathode Materials in Sodium‐ion Batteries. InfoMat. 3, 694–704. doi:10.1002/inf2.12184
Han, D., Xiao, N., Liu, B., Song, G., and Ding, J. (2017). One-pot Synthesis of Core/Shell-Structured NiS@onion-Like Carbon Nanocapsule as a High-Performance Anode Material for Lithium-Ion Batteries. Mater. Lett. 196, 119–122. doi:10.1016/j.matlet.2017.03.042
Hong, S.-H., and Song, M. Y. (2018). Syntheses of Nano-Sized Co-Based Powders by Carbothermal Reduction for Anode Materials of Lithium Ion Batteries. Ceramics Int. 44, 4225–4229. doi:10.1016/j.ceramint.2017.12.002
Hou, X., Li, W., Wang, Y., Li, S., Meng, Y., Yu, H., et al. (2020). Sodium-Based Dual-Ion Batteries via Coupling High-Capacity Selenium/Graphene Anode with High-Voltage Graphite Cathode. Chin. Chem. Lett. 31, 2314–2318. doi:10.1016/j.cclet.2020.04.021
Hu, Y.-Y., Liu, Z., Nam, K.-W., Borkiewicz, O. J., Cheng, J., Hua, X., et al. (2013). Origin of Additional Capacities in Metal Oxide Lithium-Ion Battery Electrodes. Nat. Mater. 12, 1130–1136. doi:10.1038/nmat3784
Jin, R., Jiang, Y., Li, G., and Meng, Y. (2017). Amorphous Carbon Coated Multiwalled Carbon Nanotubes@transition Metal Sulfides Composites as High Performance Anode Materials for Lithium Ion Batteries. Electrochimica Acta. 257, 20–30. doi:10.1016/j.electacta.2017.10.078
Kim, H., Choi, W., Yoon, J., Um, J. H., Lee, W., Kim, J., et al. (2020). Exploring Anomalous Charge Storage in Anode Materials for Next-Generation Li Rechargeable Batteries. Chem. Rev. 120, 6934–6976. doi:10.1021/acs.chemrev.9b00618
Laruelle, S., Grugeon, S., Poizot, P., Dollé, M., Dupont, L., and Tarascon, J.-M. (2002). On the Origin of the Extra Electrochemical Capacity Displayed by MO/Li Cells at Low Potential. J. Electrochem. Soc. 149, A627–A634. doi:10.1149/1.1467947
Lee, Y.-J., Ha, T.-H., Cho, G.-B., Kim, K.-W., Ahn, J.-H., and Cho, K.-K. (2020). Fabrication of Nickel Sulfide/Nitrogen-Doped Reduced Graphene Oxide Nanocomposite as Anode Material for Lithium-Ion Batteries and its Electrochemical Performance. J. Nanosci. Nanotechnol. 20, 6782–6787. doi:10.1166/jnn.2020.18783
Li, H., Guo, S., Shin, K., Wong, M. S., and Henkelman, G. (2019). Design of a Pd-Au Nitrite Reduction Catalyst by Identifying and Optimizing Active Ensembles. ACS Catal. 9, 7957–7966. doi:10.1021/acscatal.9b02182
Li, L., Wang, L., Zhang, M., Huang, Q., Chen, L., and Wu, F. (2019). High-Performance Lithium-Ion Battery Anodes Based on Mn3O4/Nitrogen-Doped Porous Carbon Hybrid Structures. J. Alloys Compounds. 775, 51–58. doi:10.1016/j.jallcom.2018.10.106
Li, S., Li, B., Zhong, Y., Pan, Z., Xu, M., Qiu, Y., et al. (2019). Mn2O3@C Yolk-Shell Nanocubes as Lithium-Storage Anode with Suppressed Surface Electrolyte Decomposition. Mater. Chem. Phys. 222, 256–262. doi:10.1016/j.matchemphys.2018.10.015
Li, H., Hu, Z., Xia, Q., Zhang, H., Li, Z., Wang, H., et al. (2021). Operando Magnetometry Probing the Charge Storage Mechanism of CoO Lithium‐Ion Batteries. Adv. Mater. 33, 2006629. doi:10.1002/adma.202006629
Li, S., Gu, Z.-Y., Guo, J.-Z., Hou, X.-K., Yang, X., Zhao, B., et al. (2021). Enhanced Electrode Kinetics and Electrochemical Properties of Low-Cost NaFe2PO4(SO4)2 via Ca2+ Doping as Cathode Material for Sodium-Ion Batteries. J. Mater. Sci. Technology. 78, 176–182. doi:10.1016/j.jmst.2020.10.047
Li, Z., Zhang, Y., Li, X., Gu, F., Zhang, L., Liu, H., et al. (2021). Reacquainting the Electrochemical Conversion Mechanism of FeS2 Sodium-Ion Batteries by Operando Magnetometry. J. Am. Chem. Soc. 143, 12800–12808. doi:10.1021/jacs.1c06115
Li, Q., Li, H., Xia, Q., Hu, Z., Zhu, Y., Yan, S., et al. (2020). Extra Storage Capacity in Transition Metal Oxide Lithium-Ion Batteries Revealed by In Situ Magnetometry. Nat. Mater. 20, 76–83. doi:10.1038/s41563-020-0756-y
Li, W, Liang, H.-J., Hou, X.-K., Gu, Z.-Y., Zhao, X.-X., Guo, J.-Z., et al. (2020). Feasible Engineering of Cathode Electrolyte Interphase Enables the Profoundly Improved Electrochemical Properties in Dual-Ion Battery. J. Energ. Chem. 50, 416–423. doi:10.1016/j.jechem.2020.03.043
Li, X., Chen, Y., Zou, J., Zeng, X., Zhou, L., and Huang, H. (2016). Stable Freestanding Li-Ion Battery Cathodes by In Situ Conformal Coating of Conducting Polypyrrole on NiS-Carbon Nanofiber Films. J. Power Sourc. 331, 360–365. doi:10.1016/j.jpowsour.2016.09.067
Liang, H., Zhang, H., Zhao, L., Chen, Z., Huang, C., Zhang, C., et al. (2022). Layered Fe2(MoO4)3 Assemblies with Pseudocapacitive Properties as Advanced Materials for High-Performance Sodium-Ion Capacitors. Chem. Eng. J. 427, 131481–131489. doi:10.1016/j.cej.2021.131481
Ni, S., Yang, X., and Li, T. (2012). Fabrication of a Porous NiS/Ni Nanostructured Electrode via a Dry Thermal Sulfuration Method and its Application in a Lithium Ion Battery. J. Mater. Chem. 22, 2395–2397. doi:10.1039/c2jm15394g
Park, J. H., and Lee, J. W. (2019). Visualized Pulverization via Ex Situ Analyses: Nickel Sulfide Anode Caged in a Hierarchical Carbon. J. Electrochem. Soc. 166, A838–A847. doi:10.1149/2.1071904jes
Ren, H., Wang, J., Cao, Y., Luo, W., and Sun, Y. (2021). Nickel Sulfide Nanoparticle Anchored Reduced Graphene Oxide with Improved Lithium Storage Properties. Mater. Res. Bull. 133, 111047–111057. doi:10.1016/j.materresbull.2020.111047
Rui, X., Tan, H., and Yan, Q. (2014). Nanostructured Metal Sulfides for Energy Storage. Nanoscale. 6, 9889–9924. doi:10.1039/c4nr03057e
Teng, X., Zhang, F., Li, Q., Wang, X., Ye, W., Li, H., et al. (2020). Interfacial Engineering of Self-Supported SnO2 Nanorod Arrays as Anode for Flexible Lithium-Ion Batteries. J. Electrochem. Soc. 167, 120515–120524. doi:10.1149/1945-7111/abac86
Vadivazhagan, M., Shakkeel, N. K., and Nallathamby, K. (2021). Demonstration of Biocarbon-Added NiS Porous Nanospheres as a Potential Anode for Lithium-Ion Batteries. Energy Fuels. 35, 8991–9000. doi:10.1021/acs.energyfuels.1c00582
Wang, J., Polleux, J., Lim, J., and Dunn, B. (2007). Pseudocapacitive Contributions to Electrochemical Energy Storage in TiO2 (Anatase) Nanoparticles. J. Phys. Chem. C. 111, 14925–14931. doi:10.1021/jp074464w
Wang, K., Li, L., Zhang, T., and Liu, Z. (2014). Nitrogen-doped Graphene for Supercapacitor with Long-Term Electrochemical Stability. Energy. 70, 612–617. doi:10.1016/j.energy.2014.04.034
Wang, L.-H., Dai, Y.-K., Qin, Y.-F., Chen, J., Zhou, E.-L., Li, Q., et al. (2020). One-Pot Synthesis and High Electrochemical Performance of CuS/Cu1.8S Nanocomposites as Anodes for Lithium-Ion Batteries. Materials. 13, 3797–3808. doi:10.3390/ma13173797
Wang, L, Teng, X.-L., Qin, Y.-F., and Li, Q. (2021). High Electrochemical Performance and Structural Stability of CoO Nanosheets/CoO Film as Self-Supported Anodes for Lithium-Ion Batteries. Ceramics Int. 47, 5739–5746. doi:10.1016/j.ceramint.2020.10.160
Wang, X., Liu, W., Wang, C., Zhang, S., Ding, M., and Xu, X. (2021). Enhanced Formaldehyde Gas Sensing Performance of Ternary CuBi2O4 Oxides through Oxygen Vacancy Manipulation and Surface Platinum Decoration. Sensors Actuators B: Chem. 344, 130190–130199. doi:10.1016/j.snb.2021.130190
Wang, X., Liu, W., Wang, T., Zhao, Y., Zhao, G., Zhang, S., et al. (2022). Synthesis of Multishelled SnOx/Co3O4 Amorphous/Crystalline Heterophase with Galvanic Replacement Reaction for superior HCHO Sensing. Sensors Actuators B: Chem. 350, 130876–130886. doi:10.1016/j.snb.2021.130876
Wang, X., Zhang, S., Shao, M., Huang, J., Deng, X., Hou, P., et al. (2017). Fabrication of ZnO/ZnFe2O4 Hollow Nanocages Through Metal Organic Frameworks Route with Enhanced Gas Sensing Properties. Sensors Actuators B: Chem. 251, 27–33. doi:10.1016/j.snb.2017.04.114
Wang, Y., Zhu, Q., Tao, L., and Su, X. (2011). Controlled-Synthesis of NiS Hierarchical Hollow Microspheres with Different Building Blocks and Their Application in Lithium Batteries. J. Mater. Chem. 21, 9248–9254. doi:10.1039/c1jm10271k
Wu, X., Li, S., Xu, Y., Wang, B., Liu, J., and Yu, M. (2019). Hierarchical Heterostructures of NiO Nanosheet Arrays Grown on pine Twig-like β-NiS@Ni3S2 Frameworks as Free-Standing Integrated Anode for High-Performance Lithium-Ion Batteries. Chem. Eng. J. 356, 245–254. doi:10.1016/j.cej.2018.08.187
Xia, G., Li, X., He, J., Wang, Y., Gu, Y., Liu, L., et al. (2021). A Biomass-Derived Biochar-Supported NiS/C Anode Material for Lithium-Ion Batteries. Ceramics Int. 47, 20948–20955. doi:10.1016/j.ceramint.2021.04.093
Yang, Z. Y., Yuan, Y. F., Zhu, M., Yin, S. M., Cheng, J. P., and Guo, S. Y. (2021). Superior Rate-Capability and Long-Lifespan Carbon Nanotube-In-nanotube@Sb2S3 Anode for Lithium-Ion Storage. J. Mater. Chem. A. 9, 22334–22346. doi:10.1039/d1ta06708g
Zhang, L., Huang, Y., Zhang, Y., Gu, H., Fan, W., and Liu, T. (2016). Flexible Electrospun Carbon Nanofiber@NiS Core/Sheath Hybrid Membranes as Binder-free Anodes for Highly Reversible Lithium Storage. Adv. Mater. Inter. 3, 1500467–1500476. doi:10.1002/admi.201500467
Zhang, Y., Lu, F., Pan, L., Xu, Y., Yang, Y., Bando, Y., et al. (2018). Improved Cycling Stability of NiS2 Cathodes Through Designing a "Kiwano" Hollow Structure. J. Mater. Chem. A. 6, 11978–11984. doi:10.1039/c8ta01551a
Zhao, J., Zhang, Y., Wang, Y., Li, H., and Peng, Y. (2018). The Application of Nanostructured Transition Metal Sulfides as Anodes for Lithium Ion Batteries. J. Energ. Chem. 27, 1536–1554. doi:10.1016/j.jechem.2018.01.009
Zhao, W. C., Yuan, Y. F., Du, P. F., Yin, S. M., and Guo, S. Y. (2021). Intimately Coupled MoP Nanocrystalline@carbon Nanosheets-Assembled Hierarchical Mesoporous Nanospheres for High-Performance Sodium-Ion Storage. Electrochimica Acta. 389, 138712. doi:10.1016/j.electacta.2021.138712
Zheng, Y. Q., Yuan, Y. F., Tong, Z. W., Yin, H., Yin, S. M., and Guo, S. Y. (2020). Watermelon-like TiO2 Nanoparticle (P25)@microporous Amorphous Carbon Sphere with Excellent Rate Capability and Cycling Performance for Lithium-Ion Batteries. Nanotechnology. 31, 215407. doi:10.1088/1361-6528/ab73be
Zhou, Y., Huang, Y., Pang, J., and Wang, K. (2019). Remaining Useful Life Prediction for Supercapacitor Based on Long Short-Term Memory Neural Network. J. Power Sourc. 440, 227149–227157. doi:10.1016/j.jpowsour.2019.227149
Keywords: anodes, lithium-ion batteries, hydrothermal method, NiS nanospheres, electrochemical performance
Citation: Wang L-H, Ren L-L, Qin Y-F and Li Q (2022) Hydrothermal Preparation and High Electrochemical Performance of NiS Nanospheres as Anode for Lithium-Ion Batteries. Front. Chem. 9:812274. doi: 10.3389/fchem.2021.812274
Received: 10 November 2021; Accepted: 16 December 2021;
Published: 03 February 2022.
Edited by:
Jun Zhang, Zhejiang University of Technology, ChinaReviewed by:
Xiao-Dong Zhu, Qingdao University of Science and Technology, ChinaYongfeng Yuan, Zhejiang Sci-Tech University, China
Copyright © 2022 Wang, Ren, Qin and Li. This is an open-access article distributed under the terms of the Creative Commons Attribution License (CC BY). The use, distribution or reproduction in other forums is permitted, provided the original author(s) and the copyright owner(s) are credited and that the original publication in this journal is cited, in accordance with accepted academic practice. No use, distribution or reproduction is permitted which does not comply with these terms.
*Correspondence: Yu-Feng Qin, cWlueXVmZW5nQHNkYXUuZWR1LmNu
†These authors have contributed equally to this work