- Department of Kinetics and Catalysis, Institute of Problems of Chemical Physics, RAN, Chernogolovka, Russia
The energy, material, and environmental problems of society require clean materials and impose an urgent need to develop effective chemical processes for obtaining and converting energy to ensure further sustainable development. To solve these challenges, it is necessary, first of all, to learn solar energy harvesting through the development of artificial photosynthesis. In our planet, water, carbon dioxide, and methane are such affordable and inexhaustible clean materials. Electro/photocatalytic water splitting, and also CO2 and CH4 transforming into valuable products, requires the search for relevant efficient and selective processes and catalysts. Of great interest is the emerging new generation of bioinspired catalysts—metallocavitins (MCs). MCs are attracting increasing attention of researchers as advanced models of metalloenzymes, whose efficiency and selectivity are well known. The primary field of MC application is fine organic synthesis and enantioselective catalysis. On the other hand, MCs demonstrate high activity for energy challenging reactions involving small gas molecules and high selectivity for converting them into valuable products. This mini-review will highlight some recent advances in the synthesis of organic substances using MCs, but its main focus will be on the rapid development of advanced catalysts for the activation of small molecules, such as H2O, CO2, and CH4, and the prospects for creating related technological processes in the future.
Introduction
In recent decades, we have witnessed the emergence of an innovative generation of bioinspired catalysts—metallocavitins (MCs). Cavitins are a class of nanoporous molecules and supramolecular ensembles interesting for heterogenous metal catalysis, based usually on transition metals. The performance of the transition metal catalyst increases due to its retention in molecular nanocontainers with intrinsic porosity usually used for preparation of metallocavitins. At the same time, microporous compounds, such as charcoal or zeolites, are used long time as carriers for metal ions in industrial heterogenous catalysis because they greatly increase the performance of the encapsulated transition metals. There are two big classes of cavitins: the first one, discrete individual molecules—monocavitins, such as, for example, cyclodextrins, calixarenes, metal-organic cages (MOCs), covalent organic cages (COCs), and, the second, extended ones—polycavitins, such as zeolites, metal-organic frameworks (MOFs), covalent organic frameworks (COFs), and polypeptides, which all together demonstrate a rich library of architectures varying in shape, size, and geometry (Figure 1). MOFs and MOCs are formed by coordination-driven self-assembly. They are composed of polydentate organic linkers and inorganic nodes containing metal ions or clusters known as secondary binding units (SBUs). On the other hand, COFs and COCs are organic molecules of different sizes and complexity, synthesized via covalent binding. M-cavitins demonstrate high activities for energetically challenging reactions with participation of small gas molecules and high selectivity to valuable products. Nowadays, the use of new sources of clean materials and the development of efficient processes for their conversion are a necessary requirement for growing energy needs and environmental protection. In this connection, M-cavitins are promising catalysts for future technology.
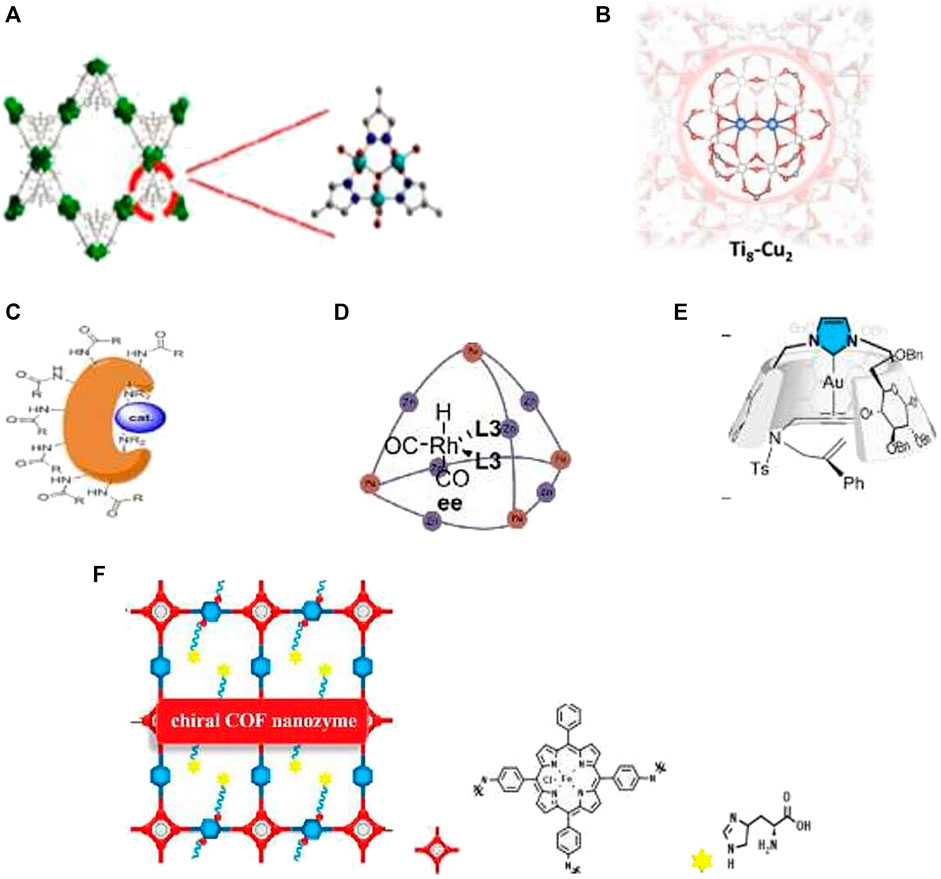
FIGURE 1. IMC as bioinspired catalysts: (A) Artificial catechol oxidase (Li et al, 2020). (B) Artificial binuclear copper monooxygenase based on Ti-MOF (Feng et al, 2021). (C) MC (cat-RuOs) for enantioselective dihydroxylation and epoxydation of styrene derivatives (Leurs et al, 2018). (D) MC for the alkene size-selective hydroformylation (Nurttila et al, 2019). (E) N-heterocyclic carbene-capped CD-Au complex for an enantioselective enyne cycloisomerization (Roland et al., 2018). (F) Chiral COF nanozyme for enantioselective L-DOPA oxidation (Zhou et al, 2020).
Metallocavitins as Bioinspired Catalysts
Metals in the coordination cavitins may serve either only for constructive goals or also as a coordinately unsaturated catalytic center. The general approaches for the incorporation of metal active sites into cavitins include metallolinkers, non-covalent encapsulation of metal complexes and enzymes, templated metal/ligand assemblies, and post-synthetic metalation (Leenders et al., 2015). The complexity of MOCs and COCs has increased dramatically in recent times: heteroleptic, mixed-metal, and low symmetry assemblies and different composites are becoming more commonplace (Pullen et al., 2021). The right balance between flexibility and rigidity of cavitins is favorable for binding substrates and releasing products (Zhang et al., 2017). Shape-persistent organic cages permit precise control of their size and geometry (Holsten et al., 2021). On the other hand, adaptability is a hallmark of enzymes. Flexible cavitins mimic these owing to structural changes that accompany adsorption and desorption steps (Heerden et al., 2021). Cavitins are able to create chiral molecular catalysts via preferential secondary interactions between the substrate and framework that induce enantioselectivities not achievable in homogenous systems. Thus, they catalyze the asymmetric acetylation of aromatic aldehydes and 2-aminobenzamide to generate the products with up to 93% yield and 97% ee (Hou et al., 2021). As was noted, the primary field of MC application is fine organic synthesis and enantioselective catalysis. It is well-known that special channels in enzymes facilitate transport of substrates and products. The mesoporous MOF MnO2@OMUiO-66(Ce), containing artificial substrate channels and MnO2 attached to Ce–O clusters, was designed as a super-active artificial catalase (Yang et al., 2021a). These authors proposed some guides for the rational design of super-active biomimetic systems. MOF-818, containing nodes of trinuclear copper centers that mimic the active sites of catechol oxidase, shows efficient catechol oxidase activity. This artificial enzyme oxidizes o-diphenoles to o-qunones with good substrate specificity (Li et al., 2020, Table 1.1, Figure 1A). The direct selective oxidations of the most difficult C–H bonds with O2 are very challenging reactions and play an important role in fine organic synthesis (Shteinman and Mitra, 2021). Nature has created highly active and selective binuclear metal-containing monooxygenases working with the participation of O2 and a reducing agent and capable of activating the most inert C–H bond of alkanes involving methane. Recently the MOF-based artificial binuclear monooxygenase Ti8–Cu2 (Table 1.2, Figure 1B) was prepared via metalation of the SBU in a Ti-MOF. The SBU provided a precise binding pocket for the installation of binuclear Cu cofactors to cooperatively activate O2. In the presence of co-reductants, Ti8–Cu2 demonstrated excellent catalytic activity and selectivity in monooxygenation processes, including epoxidation, hydroxylation, and sulfoxidation, with TOF, which is much higher than that of mononuclear Ti8–Cu1 (Feng et al., 2021). It would be interesting to check and develop this monooxygenase for alkane hydroxylation involving methane. While polycavitins are used for fabrication of advanced heterogenous catalysts (Bavykina et al., 2020), monocavitins are more suitable for modeling and academic study of the enzyme active sites. However in the recent years, MOC use in catalysis has also increased. For example, the highest activity for the enantioselective dihydroxylation and epoxidation of styrene derivatives was obtained by using Ru or Os complexes linked with bovine serum albumin (BSA, Leurs et al., 2018, Table 1.3, Figure 1C). Also, by coordination of the monocavitin, based on an Fe4(Zn-L)6 cage to rhodium, efficient hydroformylation catalysts Rh@Fe4(Zn-L)6, that shows excellent product selectivity, has been obtained (Nurttila et al, 2019; Table 1.4, Figure 1D). For the case of N-heterocyclic carbene-capped CD gold complexes (Figure 1E). (ICD)Au Cl stereoselectivity in enyne-cycloisomerization depends on the nature of the cyclodextrin: α-ICD and β- ICD give the enantiomer for which the approach is the easiest according to their helical shape, and γ- ICD does not afford enantioselectivity because of its symmetrical shape (Roland et al., 2018; Table 1.5, Figure 1E). The incorporation of iron porphyrin and L- or D-histidines endows chiral COF nanozymes with high activity and selectivity in the peroxidase oxidation of dopa enantiomers (Figure 1F). This artificial peroxidase possesses 21.7 times higher activity than natural HRP (Zhou et al., 2020). “Substrate selectivity is more difficult to rationalize for small molecules, such as H2, O2, CO2, and CH4, which possess too narrow a range of physical characteristics to allow either precise positioning or discrimination between reactants. Nevertheless, metalloenzymes have evolved to metabolize these small-molecule substrates with high selectivity and efficiency due to small-molecule tunnels and gate-effects. It delivers the right substrate to the right location at the right time, for example, for selective oxidation of the strongest aliphatic hydrocarbon bond in methane” (Banerjee and Lipscomb, 2021). “Also for the small molecule discussed here, the spatial and temporal control of delivery of protons and electrons to the active site is crucial to maintain product selectivity in these transformations” (Amanullah et al., 2021). The activation of small molecules, such as CO2, N2, O2, and CH4, has always been a dream in chemistry. The modern challenges of climate change, energy deficit, and quality of the fossil resources used make the transformation of these small molecules more important than ever before. M-cavitins demonstrate high activities for energetically challenging reactions with participation of small gas molecules and high selectivity to valuable products. This explains the great interest in these processes and rapid development of advanced catalysts for the activation of small molecules, such as H2O, CO2 and CH4, in recent years.
H2O and Artificial Photosynthesis
Water in combination with sunlight can serve as a source of cheap and clean energy, provided that artificial photosynthesis is mastered. The challenges of the incumbent energy crisis and environmental problems can be solved via photochemical, electrochemical, and photoelectrochemical water splitting (WS) to produce oxygen and hydrogen green fuels. For the realization of artificial photosynthesis, it is “necessary to develop the design of WS catalysts that can be incorporated into future sunlight-to-chemical fuel assemblies” (Ezhov et al., 2020). WS involves two reactions: oxygen evolution reaction (OER) and hydrogen evolution reaction (HER). Photoinduced WS into oxygen and hydrogen is one of the most interesting pathways for solving the incumbent society problems. A porphyrinic zirconium MOF nanotube HNTM-Ir/Pt possessed a high photocatalytic HER rate of 201.9 mmol g−1h−1 and better recycling stability under visible light irradiation in WS than earlier HNTM-Pt or HNTM-Ir (He et al., 2018). The double-shelled TiO2@ZIF-8 hollow spheres used for HER under illumination show an efficient charge separation by electron injection from ZIF-8 to TiO2, high photocatalytic quantum efficiencies of 50.89% at 380 nm, and high HER rate up to 261.7 mmol g−1h−1, which is 3.5 times higher than that of bare TiO2 (Qiu et al., 2021). Donor–acceptor imine-linked COFs produce hydrogen with a rate 20.7 mmol g−1h−1 under visible light irradiation due to protonation of their imine linkages and improved charge separation efficiency (Yang et al., 2021b). Electrocatalytic WS has been regarded as another promising approach for producing hydrogen and oxygen under mild conditions. An Fe–Cu layered double hydroxide integrated with a Co MOF ZIF-12 to form LDH-ZIF-12 composite shows enhanced OER performance as compared to individual components, that is, Fe–Cu-LDH and ZIF-12. Chronoamperometric studies show that this composite leads to a higher current density and low overpotential, and is an efficient and stable electrocatalyst for commercial use (Hameed et al., 2021). The multi-shelled hollow Mn/Fe-hexaiminobenzene MOF (Mn/Fe-HIB-MOF) (Figure 2A) is an excellent bifunctional electrocatalyst for dioxygen reduction reaction and OER. It has better performance than commercial RuO2, Mn-HIB-MOF, and Fe-HIB-MOF catalysts (Shinde et al., 2019)]. The Fe–Ni-MOF show remarkable electrocatalytic performance with a low overpotential of 266 mV at 100 mA cm−2 and a high TOF value of 0.261 s−1 for water oxidation (Figure 2B, Wang C.-P. et al, 2021). The iridacycle-decorated COF shows 10-fold efficiency enhancement in photocatalytic hydrogen evolution from aqueous formate solution in comparison with its molecular counterpart under mild conditions (Hu et al., 2021). Photoinduced water oxidation by MOFs has been widely studied in the past few years. The active water oxidation catalyst cis-[Ru(bpy) (5,5′-dcbpy) (H2O)2]2+ was incorporated into UIO-67 MOFs using postsynthetic modification of the framework (Figure 2C). XAS, EPR, and Raman spectroscopy confirmed the formation of M-cavitin and the highly active RuV = O key intermediate (Ezhov et al., 2020). A bioinspired trinuclear copper catalyst developed recently for water oxidation displays a TOF value of 20 000 s−1, which is 150 times and 15 times higher than that of the mono- and the bis-Cu complexes, respectively (Figure 2D; Chen Q.-F. et al, 2021). Also, four MOCs based on cobalt ions and imidazolate ligands were studied recently on photo-driven water oxidation for the first time (Chen Z.-Y. et al, 2021). These studies revealed that photoinduced water oxidation starts via e− transfer from the excited [Ru(bpy)3]2+* to Na2S2O8 and then to the bis (μ-oxo) dicobalt active sites which further donate electrons to the oxidized [Ru(bpy)3]3+ to drive water oxidation (Figure 2E). Self-assembled nanospheres with guanidinium binding sites form sulfonate-functionalized preorganized ruthenium catalysts for electrochemical water oxidation, which leads to an increase in the reaction rate by almost 100 times compared to a homogenous system (Figure 2F) (Yu et al., 2018). Hierarchical bifunctional catalysts for WS are undoubtedly the most promising catalysts of the low-carbon energy future. Such a Fe3O4@MnOx binary metal oxide core–shell nano-polyhedron fabricated recently (Figure 2F, Li et al., 2021) involves cathodic 2e− HER and anodic 4 e− OER and has a low HER/OER overpotential of 242/188 mV (10 mA cm−2), a small Tafel slope of 116.4/77.6 mV dec−1, and a long-term cyclability of 5 h. By applying this catalyst as an independent cathode and anode, the overall WS cell supplies a competitive voltage of 1.64 V to achieve 10 mA cm−2 and good work stability of 80 h. Another example is Fe-doped NiCo2O4/Ni3S4 hollow heterostructure nanotubes which implement highly efficient electrocatalytic overall WS with low overpotential of 29.1 mV (10 mA cm−2), a relatively small Tafel slope of 53.3 mV dec−1 for the HER, and 259 mV at a current density of 100 mA cm−2 (33.1 mV dec−1) for the OER (Liu et al., 2021).
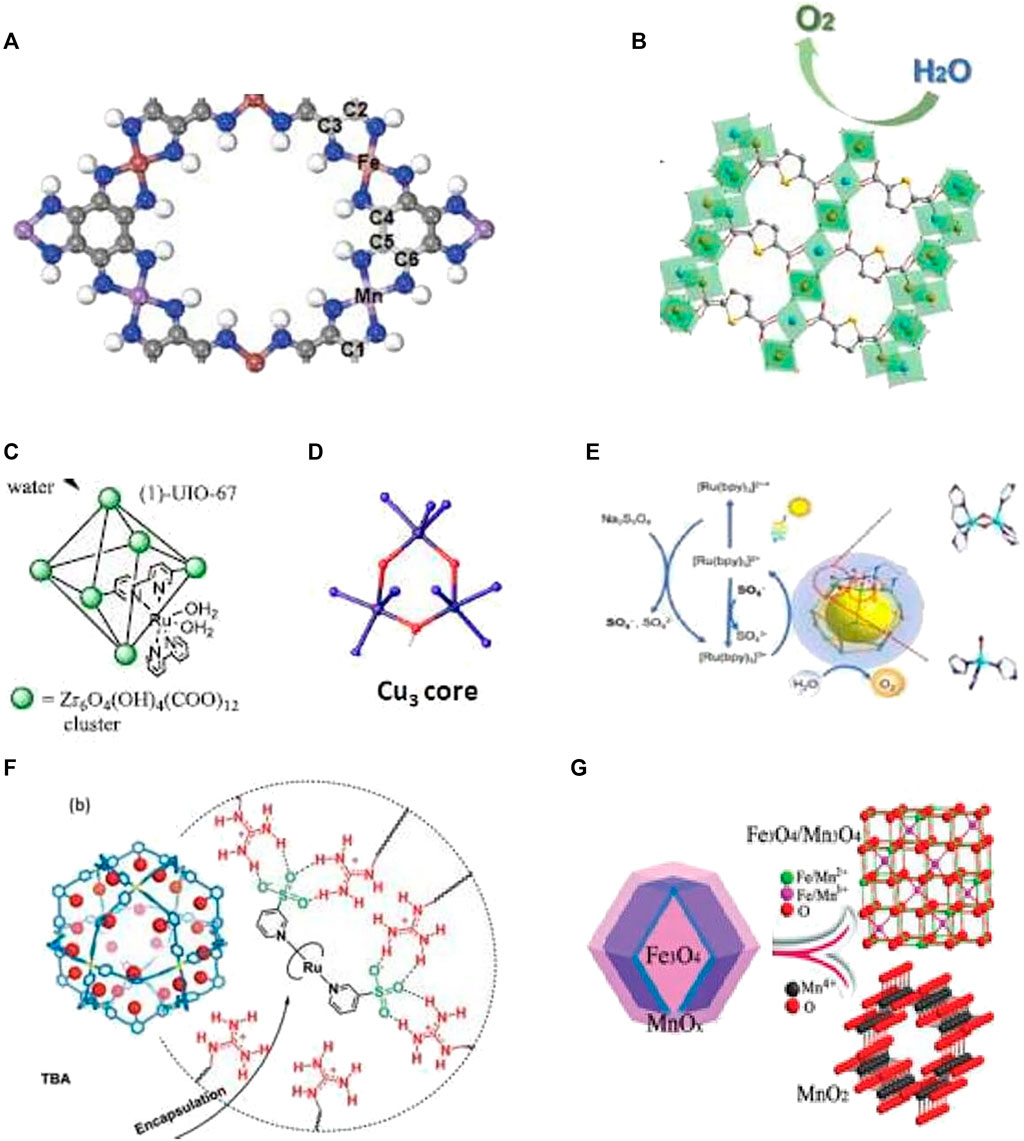
FIGURE 2. MC for WS and O reduction. (A) Mn/Fe-hexaiminobenzene MOF for electrocatalytic oxygen reduction reaction and OER (Shinde et al, 2019). (B) Fe–Ni MOF electrocatalyst for water oxidation (Wang K. et al, 2021). (C) Active water oxidation catalyst cis-[Ru(bpy) (5,5′ dcbpy) (H₂O)2]2+ (1) incorporated into UIO-67 MOF (Ezhov et al, 2020) (D) Cu-core of the bioinspired catalyst for water oxidation (Chen Z. -Y. et al, 2021). (E) Co-based MOC for photo-driven water oxidation (Chen Q. F. et al. 2021). (F) Preorganization of the ruthenium catalyst in self-assembled nanospheres for electrochemical water oxidation (Yu et al, 2018). (G) Hierarchical Fe–Mn binary metal oxide core-shell nano-polyhedron for electrochemical WS (Li et al, 2021).
CO2 and Methane
Mastering artificial photosynthesis includes along with photolytic WS also solar light harvesting and photochemical reduction of carbon dioxide (CO2R). The light harvesting systems based on supramolecular assembly in aqueous media contain light-absorbing chromophores which gather and convert the excitation energy to chemical energy via energy transfers from donors to acceptors. Some cavitins, for example, hydrophobic macrocycles, such as CD and calixarenes, play a prominent role in forming these supramolecular systems (Wang H et al., 2021). The photochemical CO2R was studied for a number of MCs. In cavitins, the transition state of the target reaction can be stabilized more efficiently in comparison with bulk solution. Thus, Ir complex incorporated into Zr-MOC-NH2 was used for CO2 photoreduction. DFT calculations and in situ IR show that the Ir(III) complex is the catalytic center and −NH2 in the cavity plays a synergetic role in the stabilization of the transition state and Ir∙CO2 intermediate (Qi et al., 2021). Under irradiation by visible light, the single IrIII-MOC-NH2 cage can convert CO2 into CO with a selectivity of 99.5% and a TOF of 120 h−1, which is 3.4 times higher that of bulk Ir(III) complex and 100 times higher than that of the classical MOF counterpart, IrIII-Uio-67-NH2 (Figure 3A, Qi et al., 2021). Photocatalytic CO2 R with the MIL-100(Fe)-CsPbBr3 composite having a high specific surface area, an enhanced solar light response, and an improved charge carrier separation resulted in an excellent photocatalytic performance with 20.4 μmol CO produced per gram of the photocatalyst during 1 hour under visible light irradiation (Cheng et al., 2020). Anionic MOFs as a host and a cationic photosensitizer as a guest were self-assembled into a photocatalytic system, Ru@Cu-HHTP, which showed high activity for photocatalytic CO2R under sunlight with CO selectivity of 91.3% (Figure 3B, Huang et al., 2021). An Ni-MOF–derived catalyst for the light-driven methanation of CO2 produces methane with a rate of 488 mmol g_1h_1 under UV–visible–IR irradiation and displayed excellent recyclability without loss of catalytic activity (Khan et al., 2021). Because of the highly stacked layers, some COFs have semiconductive properties and exhibit good catalytic performance in photo-CO2R (Nguyen and Alzamly, 2021). The d-UiO-66/MoS2 composite implements the photocatalytic conversion of CO2 and H2O to CH3COOH under visible light. The evolution rate and selectivity of CH3COOH reached 39.0 mmol g_1h_1 and 94%, respectively, without any C1 products (Figure 3C, Yu F. et al, 2021). Electrochemical CO2R has also been proven to be a promising strategy among various types of energy conversion. A desired electrocatalyst should have a high TON, a high TOF, and low overpotential (Zhang H. et al, 2021). The Zn-based MOF CALF20 demonstrates the highest CO Faradaic efficiency of 94.5% at −0.97 V vs. RHE with a TOF of 1360.8 h−1 and a partial current density of −32.8 mA/cm2 for electrochemical CO2R to CO (Al-Attas et al., 2021). The redox-active In-MOF shows the first example of an Ni-based MOF catalyst in electrocatalytic CO2R, which suggests new prospects for designing novel and efficient non-noble, metal-based, redox-active, biomimetic MOFs (Zhou et al., 2021). Conductive phthalocyanine-based MOF (NiPc-NiO4) nanosheets linked by nickel-catecholate are highly efficient electrocatalysts for the CO2R to CO electroreduction (Figure 3D). The obtained NiPc–NiO4 has good conductivity and exhibited a selectivity of 98.4% toward CO production and a large CO partial current density of 34.5 mA cm−2, outperforming the reported MOF catalysts (Yi et al., 2021). Hydrogenation of CO2 to valuable chemicals may also be of great interest for the implementation of a sustainable carbon cycle. Multiple Cu centers supported on Ti-MOF (Figure 3E) catalyze CO2 hydrogenation to ethylene. Zeng et al., (2021) present a new tandem route for CO2-to-C2H4 conversion via CO2 hydrogenation to ethanol followed by its dehydration. The catalyst exhibits high ethylene selectivity, that is, > 90%. The MOF UiO-66 was used in tandem with its zirconium oxide nodes and an incorporated ruthenium PNP pincer complex to hydrogenate CO2 to HCOOH, then to CH3OH, giving the highest reported TON 19 000 and TOF 9100 h−1. Moreover, the reaction was readily recyclable, leading to a cumulative TON of 100,000 after 10 reaction cycles (Figure 3F, Rayder et al., 2021). The neighboring Zn2+-O-Zr4+ sites obtained by postsynthetic treatment of Zr6(μ3-O)4(μ3-OH)4 nodes of MOF-808 by ZnEt2 gave the MOF-808-Zn catalyst, which exhibits >99% MeOH selectivity in CO2 hydrogenation at 250°C and a high space-time yield of up to 190.7 mg MeOH gZn−1 h−1 and exhibited excellent stability for at least 100 h (Figure 3G, Zhang Y. et al, 2021). Bifunctional MOF containing tripyridyl complexes of Fe and Mn converts styrenes into styrene carbonates via tandem epoxydation using O2 and then CO2 insertion and also effectively transforms alkylaromatic hydrocarbons into cyanohydrins via involvement of a high-spin FeIV (S = 2) center in the challenging oxidation of the sp3 C–H bond. (Figure 3H, Shi et al., 2021). A new porous COF assembled from 12-nuclear [Cu12] nanocages {[Cu2(L4−) (H2O)2]·4DMA·2H2O}n with two types of nanotubular channels and a large specific surface area effectively catalyzes the cycloaddition of CO2 to various epoxides under mild conditions (Figure 3I, Wang W.-M. et al, 2021). Carbonylation reactions are of particular importance for organic synthesis. The use of CO2 in the carbonylation reaction catalyzed by composite (Cu1Pd2)z@PCN-222(Co) allows the photosynthesis of benzophenone with 90% yield and 97% selectivity under mild conditions (Fu et al., 2021). Cavitins are of interest in the development of tandem methylation reactions utilizing CO2 as a one-carbon building block that would enable a more sustainable chemical industry (Rooney et al., 2021). The development of the direct low-temperature selective oxidation of methane to methanol has remained an active area of research over the last 50 years (Shteinman, 2020). Compared to WS and CO2 conversion, the effective and selective chemical routes to valorize methane with participation of cavitins are relatively less elaborated. Direct methane conversion has been carried out in the gas phase over Cu- and Fe-containing zeolites in the stepwise cyclic process that involves activating the transition metal with O2 or N2O at 400–500o C, then methane reaction with active intermediates at 200o C, and finally product extraction with water steam. However, the rate and productivity of these processes are still very low (Shteinman, 2020). Catalytic processes for the hydroxylation of methane on zeolite Fe-Cu-ZSM-5 with a selectivity of 20–80% under aqueous conditions at 50°C using H2O2 were proposed (Hammond et al., 2012; Yu T. et al, 2021). The Cu–Fe (2/0.1)/ZSM-5 catalyst is an efficient catalyst for the direct conversion of methane into methanol in the liquid phase using H2O2, which exhibits an excellent methanol productivity of 431 molMeOH·mol−1Fe·h−1 and methanol selectivity of 80%. The mechanism based on catalytic, spectroscopic, and theoretical results was suggested (Yu T. et al, 2021): the acid sites adjacent to iron Bronsted contribute to the formation of an active Fe (V) = O intermediate via the dehydration of formed Fe−OOH in the aqueous H2O2 solution, enabling the homolytic cleavage of the primary C−H by radical-rebound mechanism to generate •CH3 radicals that are quickly captured by •OH radicals to form CH3OH. Contrary to Cu- and Fe-containing zeolites, the study of MOF-based MMO mimics is still at the early stage and suffers from the same problems which are low productivity and rate and low methanol selectivity due to overoxidation (Shteinman, 2020).
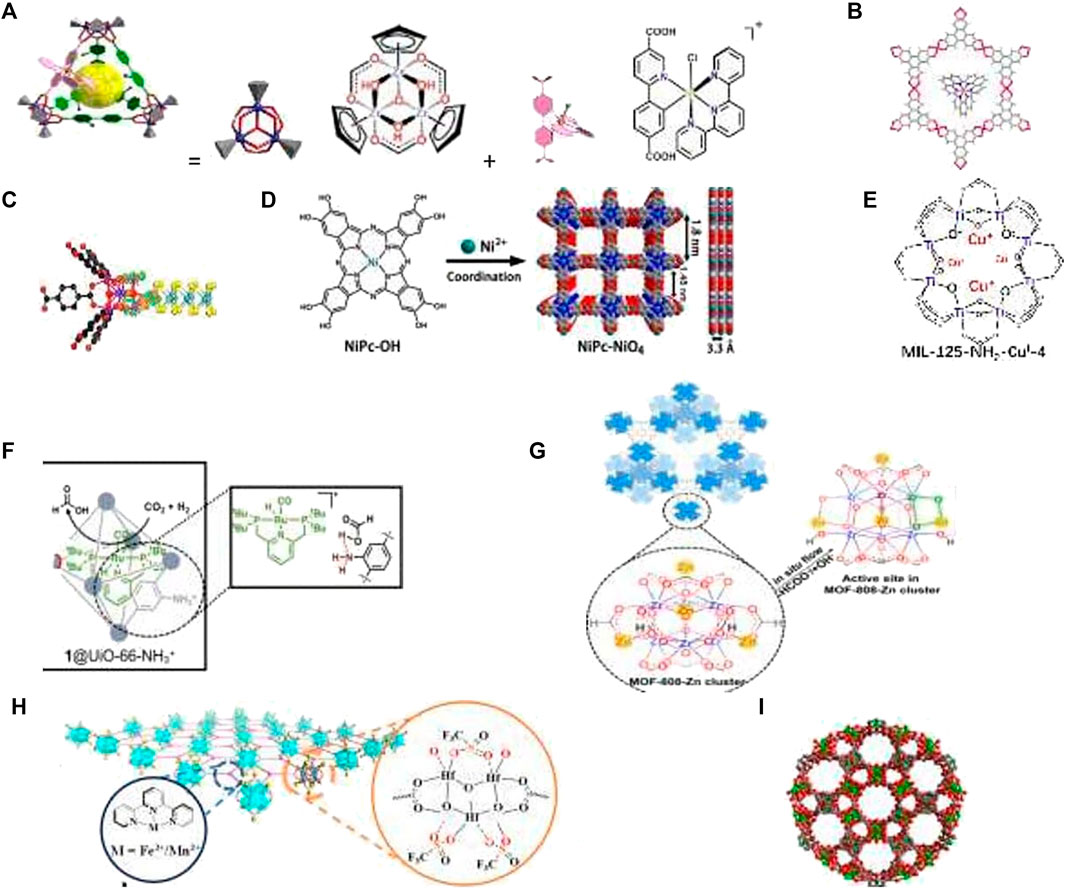
FIGURE 3. MC for CO2 transformations: (A) MOC decorated with an Ir(III) complex for CO2 photoreduction. (B) Electrostatic attraction-driven assembly of MOF with a photosensitizer for photocatalytic CO2 reduction to CO (Huang et al, 2021). (C) Hierarchically porous d-UiO-66/MoS2 for selective conversion of CO2 with 1–120 into CH3COOH (Yu T. et al, 2021). (D) Conductive two-dimensional phthalocyanine-based MOF nanosheets linked by nickel-catecholate for CO2 electroreduction. (E) Multiple cuprous centers supported on Ti-MOF for CO2 hydrogenation to ethylene (Zeng et al, 2021). (F) Multicomponent catalyst system based on MOF UiO-66 for the hydrogenation of carbon dioxide to methanol (Rayder et al, 2021). (G) MOF-808-Zn catalyst for CO2 hydrogenation (Zhang H. et al, 2021). (H) Bifunctional Metal-Organic Layers for tandem catalytic transformations using molecular oxygen and carbon dioxide (Shi et al, 2021). (I) COF assembled from 12-nuclear [Cu12] nanocages with two types nanotubular channels for a catalysis the cycloaddition of CO2 to various epoxides (Wang K. et al, 2021).
Summary, Discussion, and Perspective
Cavities and other holes are ubiquitous in the material world. Enzymes are inherently natural cavitins. Chemical cavitins have emerged due to efforts of synthetic and supramolecular chemists. M-cavitins demonstrate high activities for energetically challenging reactions. Much information has already been accumulated on the photocatalytic and electrochemical conversion of energy for the ecological production of fuels, materials, and chemicals. Still, looking ahead, innovative solutions are still needed to overcome the global energy crisis and improve the environment. The solution of these problems is primarily connected with the development of fundamental scientific research of bioinspired catalysts. These studies will require completely new solutions for the design and synthesis of a more diverse library of M-cavitins with innovative structures, metal ion composition, and functionality. Despite many achievements of electrocatalytic WS, highly active and durable catalysts have to be developed to overcome the kinetic barriers in this process, especially for the OER (Zhang H. et al, 2021). Electrocatalysis of other abundant resources, such as methane, is now increasingly coming into focus. In this area much research remains to be carried out. In particular, efforts to delineate reaction mechanisms and extract the fundamental insights are necessary to develop economically competitive electrosynthetic routes using methane. Though there still remains a considerable gap between academic research and industrial applicability, the fundamental research covered here with atomically precise catalytic systems serves as a promising foundation for the future (Zhang H et al., 2021). Using visible light irradiation to reduce CO2 to C-based products is an environmental and economic method which transforms solar energy in the form of chemical bonds. COF chemistry has been exponentially explored during the last several years, and the photocatalytic CO2 R processes catalyzed by COF materials are in their early stage (Nguyen and Alzamly, 2021). The direct oxidation of methane in a laboratory setup with participation of M-cavitins using mild conditions is still a challenging problem. There is still a huge gap between MMO enzymes and chemical catalysts based on M-cavitins both in activity and selectivity and also, importantly, in the mechanism of direct oxidation of methane to methanol.
Author Contributions
The author confirms being the sole contributor of this study and has approved it for publication.
Funding
This study was supported by the Russian Ministry of Science and Education (Project No. AAAA-A19-119071190045–0).
Conflict of Interest
The author declares that the research was conducted in the absence of any commercial or financial relationships that could be construed as a potential conflict of interest.
Publisher’s Note
All claims expressed in this article are solely those of the authors and do not necessarily represent those of their affiliated organizations, or those of the publisher, the editors, and the reviewers. Any product that may be evaluated in this article, or claim that may be made by its manufacturer, is not guaranteed or endorsed by the publisher.
References
Al-Attas, T. A., Marei, N. N., Yong, X., Yasri, N. G., Thangadurai, V., Shimizu, G., et al. (2021). Ligand-Engineered Metal-Organic Frameworks for Electrochemical Reduction of Carbon Dioxide to Carbon Monoxide. ACS Catal. 11, 7350–7357. doi:10.1021/acscatal.1c01506
Amanullah, S., Saha, P., Nayek, A., Ahmed, M. E., and Dey, A. (2021). Biochemical and Artificial Pathways for the Reduction of Carbon Dioxide, Nitrite and the Competing Proton Reduction: Effect of 2nd Sphere Interactions in Catalysis. Chem. Soc. Rev. 50, 3755–3823. doi:10.1039/d0cs01405b
Banerjee, R., and Lipscomb, J. D. (2021). Small-Molecule Tunnels in Metalloenzymes Viewed as Extensions of the Active Site. Acc. Chem. Res. 54, 2185–2195. doi:10.1021/acs.accounts.1c00058
Bavykina, A., Kolobov, N., Khan, I. S., Bau, J. A., Ramirez, A., and Gascon, J. (2020). Metal-Organic Frameworks in Heterogeneous Catalysis: Recent Progress, New Trends, and Future Perspectives. Chem. Rev. 120 (16), 8468–8535. doi:10.1021/acs.chemrev.9b00685
Chen, Q.-F., Cheng, Z.-Y., Liao, R.-Z., and Zhang, M.-T. (2021). Bioinspired Trinuclear Copper Catalyst for Water Oxidation with a Turnover Frequency up to 20000 S-1. J. Am. Chem. Soc. 143, 19761–19768. doi:10.1021/jacs.1c08078
Chen, Z.-Y., Long, Z.-H., Wang, X.-Z., Zhou, J.-Y., Wang, X.-S., Zhou, X.-P., et al. (2021). Cobalt-Based Metal-Organic Cages for Visible-Light-Driven Water Oxidation. Inorg. Chem. 60, 10380–10386. doi:10.1021/acs.inorgchem.1c00907
Cheng, R., Debroye, E., Hofkens, J., and Roeffaers, M. B. J. (2020). Efficient Photocatalytic CO2 Reduction with MIL-100(Fe)-CsPbBr3 Composites. Catalysts 10, 1352. doi:10.3390/catal10111352
Ezhov, R., Karbakhsh Ravari, A., Page, A., and Pushkar, Y. (2020). Water Oxidation Catalyst Cis-[Ru(bpy)(5,5′-dcbpy)(H2O)2]2+ and Its Stabilization in Metal-Organic Framework. ACS Catal. 10, 5299–5308. doi:10.1021/acscatal.0c00488
Feng, X., Song, Y., Chen, J. S., Xu, Z., Dunn, S. J., and Lin, W. (2021). Rational Construction of an Artificial Binuclear Copper Monooxygenase in a Metal-Organic Framework. J. Am. Chem. Soc. 143, 1107–1118. doi:10.1021/jacs.0c11920
Fu, S., Yao, S., Guo, S., Guo, G.-C., Yuan, W., Lu, T.-B., et al. (2021). Feeding Carbonylation with CO2 via the Synergy of Single-Site/Nanocluster Catalysts in a Photosensitizing MOF. J. Am. Chem. Soc. 143, 20792–20801. doi:10.1021/jacs.1c08908
Hameed, A., Batool, M., Iqbal, W., Abbas, S., Imran, M., Khan, I. A., et al. (2021). ZIF-12/Fe-Cu LDH Composite as a High Performance Electrocatalyst for Water Oxidation. Front. Chem. 9, 686968. doi:10.3389/fchem.2021.686968
Hammond, C., Forde, M. M., Ab Rahim, M. H., Thetford, A., He, Q., Jenkins, R. L., et al. (2012). Direct Catalytic Conversion of Methane to Methanol in an Aqueous Medium by Using Copper-Promoted Fe-ZSM-5. Angew. Chem. Int. Ed. 51, 5129–5133. doi:10.1002/anie.201108706
He, T., Chen, S., Ni, B., Gong, Y., Wu, Z., Song, L., et al. (2018). Zirconium-Porphyrin-Based Metal-Organic Framework Hollow Nanotubes for Immobilization of Noble-Metal Single Atoms. Angew. Chem. Int. Ed. 57, 3493–3498. doi:10.1002/anie.201800817
Heerden, D. P., Smith, V. J., Aggarwal, H., and Barbour, L. J. (2021). High Pressure In Situ Single‐Crystal X‐Ray Diffraction Reveals Turnstile Linker Rotation Upon Room‐Temperature Stepped Uptake of Alkanes. Angew. Chem. Int. Ed. 60, 13430–13435. doi:10.1002/anie.202102327
Holsten, M., Feierabend, S., Elbert, S. M., Rominger, F., Oeser, T., and Mastalerz, M. (2021). Soluble Congeners of Prior Insoluble Shape Persistent Imine Cages. Chem. Eur. J. 27, 9383–9390. doi:10.1002/chem.202100666
Hou, B., Yang, S., Yang, K., Han, X., Tang, X., Liu, Y., et al. (2021). Confinement‐Driven Enantioselectivity in 3D Porous Chiral Covalent Organic Frameworks. Angew. Chem. Int. Ed. 60, 6086–6093. doi:10.1002/anie.202013926
Hu, J., Mehrabi, H., Meng, Y.-S., Taylor, M., Zhan, J.-H., Yan, Q., et al. (2021). Probe Metal Binding Mode of Imine Covalent Organic Frameworks: Cycloiridation for (Photo)catalytic Hydrogen Evolution from Formate. Chem. Sci. 12, 7930–7936. doi:10.1039/d1sc01692j
Huang, N.-Y., He, H., Liu, S., Zhu, H.-L., Li, Y.-J., Xu, J., et al. (2021). Electrostatic Attraction-Driven Assembly of a Metal-Organic Framework with a Photosensitizer Boosts Photocatalytic CO2 Reduction to CO. J. Am. Chem. Soc. 143, 17424–17430. doi:10.1021/jacs.1c05839
Khan, I. S., Mateo, D., Shterk, G., Shoinkhorova, T., Poloneeva, D., Garzón Tovar, L., et al. (2021). An Efficient Metal-Organic Framework Derived Nickel Catalyst for the Light Driven Methanation of CO2. Angew. Chem. Int. Ed. 60, 26476–26482. doi:10.1002/anie.202111854
Leenders, S. H. A. M., Gramage-Doria, R., de Bruin, B., and Reek, J. N. H. (2015). Transition Metal Catalysis in Confined Spaces. Chem. Soc. Rev. 44, 433–448. doi:10.1039/c4cs00192c
Leurs, M., Dorn, B., Wilhelm, S., Manisegaran, M., and Tiller, J. C. (2018). Multicore Artificial Metalloenzymes Derived from Acylated Proteins as Catalysts for the Enantioselective Dihydroxylation and Epoxidation of Styrene Derivatives. Chem. Eur. J. 24, 10859–10867. doi:10.1002/chem.201802185
Li, M., Chen, J., Wu, W., Fang, Y., and Dong, S. (2020). Oxidase-like MOF-818 Nanozyme with High Specificity for Catalysis of Catechol Oxidation. J. Am. Chem. Soc. 142, 15569–15574. doi:10.1021/jacs.0c07273
Li, Y.-W., Su, S.-K., Yue, C.-Z., Shu, J., Zhang, P.-F., Du, F.-H., et al. (2021). Hierarchical Fe-Mn Binary Metal Oxide Core-Shell Nano-Polyhedron as a Bifunctional Electrocatalyst for Efficient Water Splitting. Dalton Trans. 50, 17265–17274. doi:10.1039/d1dt03048e
Liu, Z., Zhao, B., Pan, C., and Zhao, H. (2021). Binder-free Fe-Doped NiCo2O4/Ni3S4 Hollow Heterostructure Nanotubes for Highly Efficient Overall Water Splitting. Dalton Trans. 50, 18155–18163. doi:10.1039/d1dt02904e
Nguyen, H. L., and Alzamly, A. (2021). Covalent Organic Frameworks as Emerging Platforms for CO2 Photoreduction. ACS Catal. 11, 9809–9824. doi:10.1021/acscatal.1c02459
Nurttila, S. S., Brenner, W., Mosquera, J., van Vliet, K. M., Nitschke, J. R., and Reek, J. N. H. (2019). Size-Selective Hydroformylation by a Rhodium Catalyst Confined in a Supramolecular Cage. Chem. Eur. J. 25, 609–620. doi:10.1002/chem.201804333
Pullen, S., Tessarolo, J., and Clever, G. H. (2021). Increasing Structural and Functional Complexity in Self-Assembled Coordination Cages. Chem. Sci. 12, 7269–7293. doi:10.1039/d1sc01226f
Qi, X., Zhong, R., Chen, M., Sun, C., You, S., Gu, J., et al. (2021). Single Metal-Organic Cage Decorated with an Ir(III) Complex for CO2 Photoreduction. ACS Catal. 11, 7241–7248. doi:10.1021/acscatal.1c01974
Qiu, T., Gao, S., Liang, Z., Wang, D. G., Tabassum, H., Zhong, R., et al. (2021). Pristine Hollow Metal-Organic Frameworks: Design, Synthesis and Application. Angew. Chem. Int. Ed. 60, 17314–17336. doi:10.1002/anie.202012699
Rayder, T. M., Bensalah, A. T., Li, B., Byers, J. A., and Tsung, C.-K. (2021). Engineering Second Sphere Interactions in a Host-Guest Multicomponent Catalyst System for the Hydrogenation of Carbon Dioxide to Methanol. J. Am. Chem. Soc. 143, 1630–1640. doi:10.1021/jacs.0c08957
Roland, S., Suarez, J. M., and M. Sollogoub, M. (2018). Confinement of Metal–N-Heterocyclic Carbene Complexes to Control Reactivity in Catalytic Reactions. Chem. Eur. J. 24, 12464–12473. doi:10.1002/chem.201801278
Rooney, C. L., Wu, Y., Tao, Z., and Wang, H. (2021). Electrochemical Reductive N-Methylation with CO2 Enabled by a Molecular Catalyst. J. Am. Chem. Soc. 143, 19983–19991. doi:10.1021/jacs.1c10863
Shi, W., Quan, Y., Lan, G., Ni, K., Song, Y., Jiang, X., et al. (2021). Bifunctional Metal-Organic Layers for Tandem Catalytic Transformations Using Molecular Oxygen and Carbon Dioxide. J. Am. Chem. Soc. 143, 16718–16724. doi:10.1021/jacs.1c07963
Shinde, S. S., Lee, C. H., Jung, J.-Y., Wagh, N. K., Kim, S.-H., Kim, D.-H., et al. (2019). Unveiling Dual-Linkage 3D Hexaiminobenzene Metal-Organic Frameworks towards Long-Lasting Advanced Reversible Zn-Air Batteries. Energy Environ. Sci. 12, 727–738. doi:10.1039/C8EE02679C
Shteinman, A. A. (2020). Bioinspired Oxidation of Methane: from Academic Models of Methane Monooxygenases to Direct Conversion of Methane to Methanol. Kinet Catal. 61, 339–359. doi:10.1134/S0023158420030180
Shteinman, A. A., and Mitra, M. (2021). Nonheme Mono- and Dinuclear Iron Complexes in Bio-Inspired C-H and C-C Bond Hydroxylation Reactions: Mechanistic Insight. Inorg. Chim. Acta 523, 120388. doi:10.1016/j.ica.2021.120388
Wang, C.-P., Feng, Y., Sun, H., Wang, Y., Yin, J., Yao, Z., et al. (2021). Self-Optimized Metal-Organic Framework Electrocatalysts with Structural Stability and High Current Tolerance for Water Oxidation. ACS Catal. 11, 7132–7143. doi:10.1021/acscatal.1c01447
Wang, H., Jin, Y., Sun, N., Zhang, W., and Jiang, J. (2021). Post-synthetic Modification of Porous Organic Cages. Chem. Soc. Rev. 50, 8874–8886. doi:10.1039/d0cs01142h
Wang, K., Velmurugan, K., Li, B., and Hu, X.-Y. (2021). Artificial Light-Harvesting Systems Based on Macrocycle-Assisted Supramolecular Assembly in Aqueous media. Chem. Commun. 57, 13641–13654. doi:10.1039/d1cc06011b
Wang, W.-M., Wang, W.-T., Wang, M.-Y., Gu, A.-L., Hu, T.-D., Zhang, Y.-X., et al. (2021). A Porous Copper-Organic Framework Assembled by [Cu12] Nanocages: Highly Efficient CO2 Capture and Chemical Fixation and Theoretical DFT Calculations. Inorg. Chem. 60, 9122–9131. doi:10.1021/acs.inorgchem.1c01104
Yang, J., Li, K., Li, C., and Gu, J. (2021a). In Situ Coupling of Catalytic Centers into Artificial Substrate Mesochannels as Super‐Active Metalloenzyme Mimics. Small 17 (35), 2101455. doi:10.1002/smll.202101455
Yang, J., Acharjya, A., Ye, M. Y., Rabeah, J., Li, S., Kochovski, Z., et al. (2021b). Protonated Imine‐Linked Covalent Organic Frameworks for Photocatalytic Hydrogen Evolution. Angew. Chem. Int. Ed. 60, 19797–19803. doi:10.1002/anie.202104870
Yi, J. D., Si, D. H., Xie, R., Yin, Q., Zhang, M. D., Wu, Q., et al. (2021). Conductive Two‐Dimensional Phthalocyanine‐based Metal-Organic Framework Nanosheets for Efficient Electroreduction of CO2. Angew. Chem. Int. Ed. 60, 17108–17114. doi:10.1002/anie.202104564
Yu, F., Jing, X., Wang, Y., Sun, M., and Duan, C. (2021). Hierarchically Porous Metal-Organic Framework/MoS2 Interface for Selective Photocatalytic Conversion of CO2 with H2O into CH3COOH. Angew. Chem. Int. Ed. 60, 24849–24853. doi:10.1002/anie.202108892
Yu, F., Poole, D., Mathew, S., Yan, N., Hessels, J., Orth, N., et al. (2018). Control over Electrochemical Water Oxidation Catalysis by Preorganization of Molecular Ruthenium Catalysts in Self-Assembled Nanospheres. Angew. Chem. Int. Ed. 57, 11247–11251. doi:10.1002/anie.201805244
Yu, T., Li, Z., Lin, L., Chu, S., Su, Y., Song, W., et al. (2021). Highly Selective Oxidation of Methane into Methanol over Cu-Promoted Monomeric Fe/ZSM-5. ACS Catal. 11, 6684–6691. doi:10.1021/acscatal.1c00905
Zeng, L., Cao, Y., Li, Z., Dai, Y., Wang, Y., An, B., et al. (2021). Multiple Cuprous Centers Supported on a Titanium-Based Metal-Organic Framework Catalyze CO2 Hydrogenation to Ethylene. ACS Catal. 11, 11696–11705. doi:10.1021/acscatal.1c01939
Zhang, D., Martinez, A., and Dutasta, J.-P. (2017). Emergence of Hemicryptophanes: From Synthesis to Applications for Recognition, Molecular Machines, and Supramolecular Catalysis. Chem. Rev. 117, 4900–4942. doi:10.1021/acs.chemrev.6b00847
Zhang, H., Cheng, W., Luan, D., and Lou, X. W. (2021). Atomically Dispersed Reactive Centers for Electrocatalytic CO2 Reduction and Water Splitting. Angew. Chem. Int. Ed. 60, 13177–13196. doi:10.1002/anie.202014112
Zhang, Y., Li, J., and Kornienko, N. (2021). Towards Atomic Precision in HMF and Methane Oxidation Electrocatalysts. Chem. Commun. 57, 4230–4238. doi:10.1039/d1cc01155c
Zhou, Y., Liu, S., Gu, Y., Wen, G.-H., Ma, J., Zuo, J.-L., et al. (2021). In(III) Metal-Organic Framework Incorporated with Enzyme-Mimicking Nickel Bis(dithiolene) Ligand for Highly Selective CO2 Electroreduction. J. Am. Chem. Soc. 143, 14071–14076. doi:10.1021/jacs.1c06797
Keywords: metallocavitins, sustainable developing, H2O, CO2, methane
Citation: Shteinman AA (2022) Metallocavitins as Promising Industrial Catalysts: Recent Advances. Front. Chem. 9:806800. doi: 10.3389/fchem.2021.806800
Received: 17 November 2021; Accepted: 29 December 2021;
Published: 11 February 2022.
Edited by:
Vipul Sharma, Tampere University, FinlandReviewed by:
Venkata Krishnan, Indian Institute of Technology Mandi, IndiaCopyright © 2022 Shteinman. This is an open-access article distributed under the terms of the Creative Commons Attribution License (CC BY). The use, distribution or reproduction in other forums is permitted, provided the original author(s) and the copyright owner(s) are credited and that the original publication in this journal is cited, in accordance with accepted academic practice. No use, distribution or reproduction is permitted which does not comply with these terms.
*Correspondence: Albert A. Shteinman, c2h0ZWlubWFuMjAwMkBtYWlsLnJ1