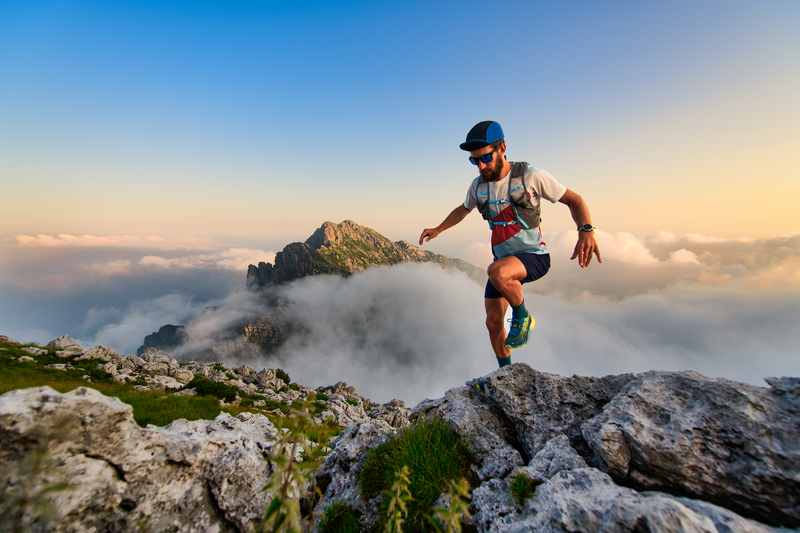
95% of researchers rate our articles as excellent or good
Learn more about the work of our research integrity team to safeguard the quality of each article we publish.
Find out more
ORIGINAL RESEARCH article
Front. Chem. , 16 December 2021
Sec. Organic Chemistry
Volume 9 - 2021 | https://doi.org/10.3389/fchem.2021.797858
This article is part of the Research Topic Bioactive Natural Products from Microbes: Isolation, Characterization, Biosynthesis and Structure Modification View all 15 articles
Three new humulane-type sesquiterpenoids, penirolide A (1), penirolide B (2), and 10-acetyl-phomanoxide (3), together with three known compounds aurasperone A (4), pughiinin A (5), and cyclo(l-Leu-l-Phe) (6) were isolated from the endophytic fungus Penicillium sp. derived from the leaves of Carica papaya L. Their structures including their absolute configurations were determined based on the analysis of NMR and HRESIMS spectra, NMR chemical shifts, and ECD calculations. Compounds 2, 3, 5, and 6 significantly inhibited glucagon-induced hepatic glucose production, with EC50 values of 33.3, 36.1, 18.8, and 32.1 μM, respectively. Further study revealed that compounds 2, 3, 5, and 6 inhibited hepatic glucose production by suppression of glucagon-induced cAMP accumulation.
Endophytic fungi, living in plants but non-pathogenic, have been proven to be promising sources of secondary metabolites with unusual structures as well as intriguing pharmacology activities, and become interesting and important resources for drug discovery (Strobel, 2003; Uzma et al., 2018; Gupta et al., 2020; Zhang et al., 2006). In recent years, increasing attention has been attracted to metabolite profiles of endophytic fungi from medicinal plants (Kaul et al., 2012). Papaya, Carica papaya L. (papaya), an edible and medicinal plant cultivated in tropical and subtropical regions, has been used as topical dressings for ulcer and dermatitis treatment, has gastrointestinal uses such as anti-helminthic and antibacterial activity treatments, has been used as anti-arthritis treatment, and has traditional uses for fertility control (Krishna et al., 2008; Pinnamaneni, 2017).
In our continuing search for structurally novel and biologically active secondary metabolites from endophytic fungi, three new humulane-type sesquiterpenoids, penirolides A (1) and B (2), and 10-acetyl-phomanoxide (3), were isolated from the endophytic fungus Penicillium sp. derived from the leaves of papaya. Humulane-type sesquiterpenoids, an uncommon type of compounds featuring an 11-membered macrocycle, were found in plants, liverworts, and fungi, and exhibited various bioactivities including antibacterial, antifungal, cytotoxic, and immunosuppressive activities (Liao et al., 2013; Luo et al., 2006; Toyota et al., 2004). Due to the flexible 11-membered macrocycle in the molecule, elucidation of the stereochemistry of humulane-type sesquiterpenoids is very challenging. Chiral derivatization and chemical conversions were successfully applied to clarify their configurations (Liao et al., 2013). However, limited amounts of sample available hampers broad use of these methods in the structural elucidation of natural products. Quantum calculations of NMR shifts represents a simple, useful, and fast alternative in address complex stereochemical problems by comparing experimental and computed values using parameters (Lodewyk et al., 2012; Grimblat and Sarotti, 2016), such as correlation coefficient, mean absolute error (MAE), corrected mean absolute error (CMAE), CP3 parameter (Smith and Goodman, 2009), DP4 probability (Smith and Goodman, 2010), or its improved version, DP4+ probability (Grimblat et al., 2015), avoiding chemical derivatization. In our effort to determine the configurations of the new humulane-type sesquiterpenoids (1–3), extensive spectroscopic analysis including 1D and 2D NMR spectra, NMR chemical shift calculations coupled with DP4+ probability analysis, and ECD calculations were utilized. In addition, all the isolates were evaluated for their anti-diabetic activity on a glucagon-induced glucose production model in mouse hepatocytes. Herein, the isolation, structural elucidation, and biological activities of compounds 1–6 Figure 1 were reported.
Compound 1 was isolated as yellow oil. Its molecular formula was determined as C15H26O4 from the positive ion peak at m/z 293.1723 [M + Na]+ (calcd for C15H26NaO4, 293.1723), requiring three indices of hydrogen deficiency. The 1H NMR spectrum (Table 1) showed signals for two olefinic protons (δH 5.47 and 5.43), three methylenes (δH 1.27–2.28), three oxygenated methines (δH 2.93, 3.43, and 3.44), and four methyls (δH 1.06, 1.08, 1.11, and 1.24). The 13C NMR spectrum revealed the presence of 15 carbons, which were classified by HSQC spectrum as one double bond (δC 122.8 and 144.7), three methylenes (δC 38.1, 45.1, and 45.6), three oxygenated methines (δC 75.6, 80.3, and 63.7), three quaternary carbons (δC 36.1, 67.1, and 75.6) including two oxygenated, and four methyls (δC 16.5, 22.3, 26.7, and 28.5). The 1H−1H COSY spectrum of 1 presented three coupling spin systems, H-7α/H-7β−H-8, H-3α/H-3β−H-4−H-5, and H-10−H-11α/H-11β−H-1 (Figure 2). The HMBC correlations (Figure 2) from CH3-13 (δH 1.06, s)/CH3-14 (1.11, s) to C-5 (δC 144.7), C-6 (δC 36.1), and C-7 (δC 45.1), from H-7α (δH 1.91, s)/H-7β (δH 1.27, s) to C-5, from CH3-12 (1.08, s) to C-1 (δC 80.3), C-2 (δC 75.6), and C-3 (δC 45.6), from H-3α (δH 2.20, s)/H-3β (δH 2.28, s) to C-1, and from CH3-15 (1.24, s) to C-8 (δC 63.7), C-9 (δC 67.1), and C-10 (δC 75.6) connected the three coupling spin systems and formed the Humulane-type sesquiterpenoid skeleton. The olefinic bond and the macrocycle accounted for two indices of hydrogen deficiency. The remaining one degree of unsaturation together with the analysis of the chemical shift of C-8 and C-9 suggested the existence of oxirane ring at positions 8 and 9.
The vicinal coupling constant (J = 15.8 Hz) between H-4 and H-5 assigned the E configured Δ4 double bond. In the ROESY spectrum, the key correlation of CH3-15 with H-8 (δH 2.93) indicated that CH3-15 and H-8 were cofacial (Figure 3). However, due to the flexibility of the 11-membered ring and the signals overlap (e.g., H-1 and H-10), the relative configuration of other stereocenters (C-1, C-2, and C-10) on the ring could not be fully determined by ROESY spectrum. Thus, we performed theoretical NMR chemical shifts calculations of eight diastereomers (Figure 4) of 1 at mPW1PW91-SCRF/6-311G(d,p)//B3LYP-D3BJ/6-31G(d) theoretical level in methanol with the GIAO method (Wolinski et al., 1990). The calculated 13C and 1H NMR chemical shifts of (1S*,2S*,8R*,9S*,10R*)-1 showed the best agreement with the experimental values. Furthermore, DP4+ analysis (Grimblat et al., 2015) predicted that (1S*,2S*,8R*,9S*,10R*)-1 was the most likely candidates with 100% probability. The absolute configuration of 1 was assigned by the ECD calculation (Figure 5). The calculated ECD curve of (1S,2S,8R,9S,10R)-1 matched well with the experimental curve, establishing the absolute configuration of 1 as 1S,2S,8R,9S,10R. Collectively, compound 1 was identified as shown in Figure 1 and named penirolide A.
FIGURE 4. Linear regression analysis between experimental and calculated 13C and 1H NMR chemical shifts of isomers of 1.
FIGURE 5. Experimental spectrum of 1 in methanol and calculated ECD spectra of (1S,2S,8R,9S,10R)-1 and (1R,2R,8S,9R,10S)-1.
Compound 2 was isolated as yellow oil. Its molecular formula was determined as C17H28O4 from the positive ion at m/z 319.1882 [M + Na]+ (calcd for C17H28NaO4, 319.1880) in the HRESIMS, corresponding to four degrees of unsaturation. The 1H NMR spectrum (Table 1) showed resonances for one olefinic proton (δH 5.61), three sp3 methines (δH 1.01, 2.93, and 3.04), five methyls (δH 0.87, 0.98, 1.15, 1.81, and 2.01), and three methylenes in which one methylene has exceptionally upfield chemical shifts (δH 0.53 and 0.38), suggesting the existence of a cyclopropane unit. The 13C NMR spectrum (Table 1) revealed the presence of 17 carbons comprising five methyls, three methylenes, five methines (including one olefinic and three oxygenated), and four non-protonated carbons (including one olefinic and one carbonyl). Three coupling spin systems, H-1−H-11α/H-11β, H-3α/H-3β−H-4−H-5, and H-7α/H-7β−H-8, could be deduced from the 1H-1H COSY correlations (Figure 2). In the HMBC spectrum, correlations of CH3-12 (δH 0.87, s) with C-2 (δC 25.8), C-3 (δC 14.6), and C-4 (δC 24.5) indicated the presence of C-2−C-3−C-4 cyclopropane moiety, which connected with C-1 as established by the correlations from H-1 (δH 3.04) to C-2 and C-3. The HMBC correlations (Figure 2) from two methyls, CH3-13 (δH 1.15) and CH3-14 (δH 0.98), to C-5 (δC 72.7), C-6 (δC 39.4), and C-7 (δC 38.9), from H-5 (δH 2.93) to C-7 (δC 38.9), and from CH3-15 (δH 1.81) to C-8 (δC 126.8), C-9 (δC 132.2), and C-10 (δC 68.6) constructed a 10-membered ring with two geminal methyls at C-6 and one methyl at C-9, which fused with the above noted cyclopropane ring through C-2/C-3. Additional HMBC correlations from CH3-17 (δH 2.01) and H-10 (δH 5.61) to carbonyl C-16 (δC 170.3) enable attachment of an acetyl group to the C-10.
The relative configuration of 2 was initially assigned by ROESY correlations. The ROESY correlations (Figure 3) of CH3-15 with H-8 (δH 5.46) and H-10 with H-7α (δH 1.76)/H-7β (δH 2.58) indicated Z geometry for the Δ8 double bond. The sequential ROESY correlations of H-10/H-4/H-1/H-3β placed these protons on the same face the ring. The CH3-12 showed ROESY correlations with H-3α and H-5, suggesting that they were located on the face opposite to H-4. However, relative configuration assignment only based on ROESY correlations is usually not reliable enough in conformationally flexible molecules such as macrocycles. In order to irrefutably determine the relative configuration of the chiral centers (C-1, C-5, and C-10) on the macrocycle of 2, eight possible diastereomers of 2 were applied to theoretical calculations of NMR chemical shifts followed by DP4+ analysis (Figure 6). The calculated chemical shifts of (1R*,2R*,4R*,5S*,10S*)-2 showed best agreement with the experimental values among the possible diastereomers and (1R*,2R*,4R*,5S*,10S*)-2 possessed 100% DP4+ probability (Grimblat et al., 2015), indicating that (1R*,2R*,4R*,5S*,10S*)-2 was the most likely candidate structure. The ECD calculation was further employed to clarify the absolute configuration of 2. The calculated ECD curve of (1R,2R,4R,5S,10S)-2 well fitted with the experimental one (Figure 7), defining the stereochemistry of 2 as 1R,2R,4R,5S,10S. Accordingly, compound 2 was elucidated as shown in Figure 1 and named penirolide B.
FIGURE 6. Linear regression analysis between experimental and calculated 13C and 1H NMR chemical shifts of isomers of 2.
FIGURE 7. Experimental spectrum of 2 in methanol and calculated ECD spectra of (1R,2R,4R,5S,10S)-2 and (1S,2S,4S,5R,10R)-2.
Compound 3 was obtained as yellow oil and its molecular formula of C17H26O4 was assigned by HRESIMS positive ion peak at 317.1723 [M + Na]+ (calcd for C17H26NaO4, 317.1723), indicating five degrees of unsaturation. The 1H NMR spectrum (Table 1) displayed signals for one olefinic proton (δH 5.44), four sp3 methines (δH 2.71, 2.76, 2.33, and 5.86), five methyls (δH 0.77, 1.12, 1.27, 1.71, and 2.05), and three methylenes. The 13C NMR spectrum (Table 1) showed resonances for 17 carbons ascribed by HSQC spectrum as five methyls, three methylenes, five methines (including one olefinic and three oxygenated), and four non-protonated carbons (including one olefinic and one carbonyl). These NMR data were very similar to that of phomanoxide (Zhang et al., 2015) whose structure was confirmed by x-ray crystallography, with the only difference being the presence of an additional acetyl group [δH 2.05 (s, 3H), δC 21.3, CH3-17; δC 170.1, C-16] in 3. The deshielded shift of C-10 (δC 68.7; ΔδC 2.1) in 3 relative to that of phomanoxide indicated that the acetyl attached at C-10, which was supported by the HMBC correlations from CH3-17 and H-10 (δH 5.86) to carbonyl C-16 (Figure 2).
In the ROESY spectrum of 3, the correlations of CH3-12 with H-3α, H-4, and H-11α suggested that these protons resided on the same face of the molecule. Cross peak of H-3β/H-5 indicated that the two protons were cofacial (Figure 3). The large coupling constant JH-10−H-11α = 12.0 Hz demonstrated that H-10 and H-11α were trans oriented. Therefore, the relative configuration was proposed as 1R*,2R*,4S*,5S*,10S*, which was further confirmed by the NMR chemical shift calculation (Figure 8) and its similar NMR data to that of phomanoxide. The 1R,2R,4S,5S,10S configuration for 3 was established based on its well-matched experimental and calculated ECD curves (Figure 9). Thus, the structure of 3 was elucidated as depicted in Figure 1 and named 10-acetyl-phomanoxide.
FIGURE 8. Linear regression analysis between experimental and calculated 13C and 1H NMR chemical shifts of isomers of 3.
FIGURE 9. Experimental spectrum of 3 in methanol and calculated ECD spectra of (1R,2R,4S,5S,10S)-3 and (1S,2S,4R,5R,10R)-3.
In addition, three known compounds, aurasperone A (4) (Campos et al., 2005), pughiinin A (5) (Pittayakhajonwut et al., 2002), and cyclo(l-Leu-l-Phe) (6) (Stark and Hofmann, 2005), were identified by comparison of their NMR data with literature data.
The liver plays a major role in whole body glucose metabolism by maintaining a balance between glucose production and glucose storage. Excessive hepatic glucose production contributes substantially to diabetes, and it is proposed that suppression of hepatic glucose production may provide therapeutic advantages for the control of diabetes (Xiao et al., 2017; Liao et al., 2021). To investigate the anti-diabetic effect of the six compounds, we examined the glucose production in hepatocytes. Compounds 2, 3, 5, and 6 significantly inhibited glucagon-induced hepatic glucose production, with EC50 values of 33.3, 36.1, 18.8, and 32.1 μM, respectively, while it was >200 µM for Compounds 1 and 4, and 2.3 μM for the positive control metformin (Figure 10A). In response to glucagon, cAMP is a second messenger to initiate glucagon signaling cascades in hepatic glucose production. Compounds 2, 3, 5, and 6 treatment suppressed cAMP accumulation (Figure 10B). These results indicated that compounds 2, 3, 5, and 6 inhibited hepatic glucose production by suppression glucagon-induced cAMP accumulation.
FIGURE 10. Effects of compounds 1–6 on glucagon-induced hepatic glucose production (A) and cAMP accumulation (B) in mouse hepatocytes. *p < 0.05 and #p < 0.05 as compared with the blank group and group treated with glucagon, respectively.
Optical rotations were measured with a JASCO P-1020 digital polarimeter. The infrared spectra were recorded on a Shimadzu UV2550 spectrophotometer (Shimadzu, Kyoto, Japan). The mass spectrometric (HRESIMS) data were acquired using an API QSTAR Pulsar mass spectrometer (Bruker, Bremen, Germany). Semipreparative high-performance liquid chromatography (HPLC) equipped with octadecyl silane (ODS) column (Cosmosil ODS-A, 10 × 250 nm, 5 μm, 4 ml/min) was used to isolate compounds. The solvents used to the preparative HPLC, such as methanol, acetonitrile, hexane, and ethanol were of chromatographic grade (Concord Technology Co. Ltd., Tianjin, China). The solvents used to the extraction or isolation of the columns, such as ethyl acetate, methanol, chloroform, and methanol, were of analytical pure (Concord Technology Co. Ltd., Tianjin, China). The NMR spectra were recorded with a Bruker AV-500 spectrometer (Bruker, Bremen, Germany) using TMS as an internal standard. Silica gel (60–80 and 200–300 mesh; Qingdao Haiyang Chemical Co. Ltd., Qingdao, China) and Rp-C18 (20–45 μm; Fuji Silysia Chemical Ltd., Durham, NC, United States) were used for column chromatography.
The fungus Penicillium sp. was isolated from healthy papaya leaves collected in Haikou, Hainan Province, People’s Republic of China, and identified by sequence analysis of the ITS region of rDNA (GenBank No. MT729953). A voucher strain was deposited in the Institute of Tropical Bioscience and Biotechnology.
Plugs of agar with mycelium were cut from solid medium and transferred aseptically to a 1,000-ml Erlenmeyer flask, containing 300 ml of liquid medium (glucose 10 g/L, maltose 20 g/L, monosodium glutamate 10 g/L, yeast extract 3 g/L, corn starch 1 g/L, mannitol 20 g/L, MgSO4 0.3 g/L, and KH2PO4 0.5 g/L). The whole culture broth (45 L) was harvested and filtered to yield the mycelium cake and liquid broth. The mycelium cake and liquid broth were extracted by EtOAc three times. The two EtOAc extracts were evaporated under reduced pressure and combined based on their similar metabolite profiles provided by HPLC analysis, affording a total of 12 g of EtOAc extract. The extract was separated by silica gel column eluted with different ratios of Petroleum ether-EtOAc (8:1, 6:1, 4:1, 2:1, 1:1, and 0:1) to afford six fractions (Fr.1–6). Fr.4 (0.52 g) was purified by a Rp-C18 silica gel column (MeOH-H2O, 70%–30%), followed by semi-preparative HPLC (MeCN-H2O, containing 0.1% Formic acid, 50:50, v/v, 4.0 ml min−1) to obtain compounds 1 (7.0 mg, tR = 9.5 min) and 2 (4.6 mg, tR = 6.5 min). Fr.3 (1.03 g) was applied to a Rp-C18 silica gel column chromatography (MeOH-H2O, 50%–50%) and semi-preparative HPLC (MeCN-H2O, containing 0.1% Formic acid, 20:80, v/v, 4.0 ml min−1) to obtain compound 3 (4.8 mg, tR = 9.6 min). Fr.6 (1.01 g) was subjected to a Rp-C18 silica gel column chromatography (MeOH-H2O, 80‒20%) to obtain three subfractions (Fr.6.1–Fr.6.3), Compounds 4 (18.3 mg, tR = 8.3 min) and 5 (18.2 mg, tR = 7.5 min) were obtained from Fr.6.1 by semi-preparative HPLC (MeCN-H2O, containing 0.1% formic acid, 75:25, v/v, 4.0 ml min−1). Fr.6.3 (0.33 g) was subjected to semi-preparative HPLC (MeCN-H2O, containing 0.1% formic acid, 15:85, v/v, 4.0 ml min−1) to afford 6 (3.2 mg, tR = 10.5 min).
Yellow oily; [ɑ]
Yellow oily; [ɑ]
Yellow oily; [ɑ]
The conformations of the isomers of compounds 1–3 were generated by iMTD-GC method embedded in Crest program (Pracht et al., 2020). Two conformations with the root-mean-square (RMS) distance and energy deviation of 0.5 Å and 0.25 kcal/mol, respectively, were considered as duplicates and one of them was removed. Density functional theory calculations were performed with the Gaussian 16 package (Frisch et al., 2019). The remaining conformers with population over 1% were optimized at the B3LYP-D3BJ/6-31G(d) level in gas phase and the conformers within an energy window of 3 kcal/mol were kept. Then, these conformers were refined by re-optimizations at the B3LYP-D3BJ/6-311G(d,p) level with the IEFPCM solvent model in methanol for 1 and chloroform for 2 and 3, and frequency analysis of all optimized conformations was also performed at the same level of theory to ensure that no imaginary frequencies were present, confirming that the optimized structures were minima on their potential energy surfaces. NMR shielding tensors were calculated with the GIAO method (Wolinski et al., 1990) at the mPW1PW91/6-311G(d,p) level with the IEFPCM solvent model in methanol for 1 and chloroform for 2 and 3. The calculated isotropic magnetic shielding constants (σ) were Boltzmann averaged according to their Gibbs free energies. The shielding constants were converted into chemical shifts by referencing to TMS at 0 ppm according to the formula δcal = σTMS–σcal, where the σTMS (the shielding constant of TMS) was calculated at the same level. For each candidate, the parameters a and b of the linear regression δcal = aδexp + b; the correlation coefficient, R2; the mean absolute error (MAE) defined as Σn |δcal–δexp|/n; and the corrected mean absolute error, CMAE, defined as Σn |δcorr–δexp|/n, where δcorr = (δcal–b)/a, were calculated. DP4+ probability analysis was performed using the calculated NMR shielding tensors with DP4+ excel file (Grimblat et al., 2015). ECD spectra were calculated by the TDDFT methodology at the B3LYP/def2TZVP utilizing IEFPCM in methanol. The final ECD spectra were simulated by averaging the spectra of lowest energy conformers according to the Boltzmann distribution theory and their relative Gibbs free energy (ΔG) using SpecDis 1.71 (Bruhn et al., 2013) with σ = 0.30 eV and uv shift = 5 nm for 1 and 10 nm for 2 and 3, respectively.
Male C57BL/6J mice (6 weeks old) were purchased from Pengyue Laboratory Animal Company (Jinan, China). Animal care and experiments were approved by the Animal Ethics Committee of Shandong Agriculture University. Primary mouse hepatocytes were prepared as previously described (Xiao et al., 2017). Briefly, fasted C57BL/6J male mice were anesthetized and livers were washed with Krebs-HEPES and digested with collagenase IV by perfusion through the inferior vena cava at 3 ml/min. Then, the whole liver was removed 6 min later, and hepatocytes were extracted in DMEM with 10% FBS. After filtering, cells were resuspended and cultured in 96- or 48-well plates. Then, the cells were treated as indicated.
Primary mouse hepatocytes were seeded in a 96-well plate and treated with 100 nM glucagon and various concentrations of test compounds (1–200 μM) for 24 h. After that, cell viability was assessed by the MTT method (Pan et al., 2021). For the determination of glucose production, hepatocytes were incubated in KRB solution containing relevant substrates (10 mM pyruvate, 100 nM glucagon) or indicated compounds (1, 5, 10, 100, and 200 μM) for 6 h. Then, the cell supernatant was collected for glucose analysis using the commercial kit.
Hepatocytes were incubated with the indicated compounds and stimulated with glucagon (100 nM) for 2 h, lysed in cell lysis buffer, and the supernatant was harvested for the assays of cAMP (Xiao et al., 2017). All data were expressed as the mean ± SD from at least three independent experiments.
The original contributions presented in the study are included in the article/Supplementary Material. Further inquiries can be directed to the corresponding authors.
Y-XZ and PC contributed to the conception and design of the study. LY and F-DK determined the planar structures and absolute configurations, and wrote the draft of the manuscript. F-RW and Q-YM undertook the isolation and purification of all compounds. NX contributed to bioactivity assay. Q-YX undertook collection and identification of strain work. Y-GW and H-FD improved the manuscript. All authors contributed to manuscript revision as well as read and approved the submitted version.
This research was supported by the Natural Science Foundation of Hainan Province (2019CXTD411), the Financial Fund of the Ministry of Agriculture and Rural Affairs, P. R. of China (NFZX2021), the Natural Science Foundation of Shandong Province (ZR2019BH080), the National Natural Science Foundation of China (82004014), the China Agriculture Research System (CARS-21), and the Central Public-Interest Scientific Institution Basal Research Fund for Chinese Academy of Tropical Agricultural Sciences (1630052017002 and 1630052021019).
The authors declare that the research was conducted in the absence of any commercial or financial relationships that could be construed as a potential conflict of interest.
All claims expressed in this article are solely those of the authors and do not necessarily represent those of their affiliated organizations, or those of the publisher, the editors, and the reviewers. Any product that may be evaluated in this article, or claim that may be made by its manufacturer, is not guaranteed or endorsed by the publisher.
The Supplementary Material for this article can be found online at: https://www.frontiersin.org/articles/10.3389/fchem.2021.797858/full#supplementary-material
Bruhn, T., Schaumlöffel, A., Hemberger, Y., and Bringmann, G. (2013). SpecDis: Quantifying the Comparison of Calculated and Experimental Electronic Circular Dichroism Spectra. Chirality 25, 243–249. doi:10.1002/chir.22138
Campos, F. R., Barison, A., Daolio, C., Ferreira, A. G., and Rodrigues-Fo, E. (2005). Complete1H and13C NMR Assignments of Aurasperone A and Fonsecinone A, Two Bis-Naphthopyrones Produced by Aspergillus Aculeatus. Magn. Reson. Chem. 43, 962–965. doi:10.1002/mrc.1654
Frisch, M. J., Trucks, G. W., Schlegel, H. B., Scuseria, G. E., Robb, M. A., Cheeseman, J. R., et al. (2019). Gaussian 16, Revision C.01. Wallingford CT: Gaussian, Inc.
Grimblat, N., and Sarotti, A. M. (2016). Computational Chemistry to the Rescue: Modern Toolboxes for the Assignment of Complex Molecules by GIAO NMR Calculations. Chem. Eur. J. 22, 12246–12261. doi:10.1002/chem.201601150
Grimblat, N., Zanardi, M. M., and Sarotti, A. M. (2015). Beyond DP4: An Improved Probability for the Stereochemical Assignment of Isomeric Compounds Using Quantum Chemical Calculations of NMR Shifts. J. Org. Chem. 80, 12526–12534. doi:10.1021/acs.joc.5b02396
Gupta, S., Chaturvedi, P., Kulkarni, M. G., and Van Staden, J. (2020). A Critical Review on Exploiting the Pharmaceutical Potential of Plant Endophytic Fungi. Biotechnol. Adv. 39, 107462. doi:10.1016/j.biotechadv.2019.107462
Kaul, S., Gupta, S., Ahmed, M., and Dhar, M. K. (2012). Endophytic Fungi from Medicinal Plants: A Treasure Hunt for Bioactive Metabolites. Phytochem. Rev. 11, 487–505. doi:10.1007/s11101-012-9260-6
Krishna, K. L., Paridhavi, M., and Patel, J. A. (2008). Review on Nutritional, Medicinal and Pharmacological Properties of Papaya (Carica Papaya Linn.). Nat. Prod. Radiance 7, 364–363.
Liao, C.-S., Tang, C.-P., Yao, S., and Ye, Y. (2013). Humulane-type Sesquiterpenoids from Pilea Cavaleriei Subsp. Crenata. Org. Biomol. Chem. 11, 4840–4846. doi:10.1039/C3OB40872H
Liao, W., Yang, W., Shen, Z., Ai, W., Pan, Q., Sun, Y., et al. (2021). Heme Oxygenase-1 Regulates Ferrous Iron and Foxo1 in Control of Hepatic Gluconeogenesis. Diabetes 70, 696–709. doi:10.2337/db20-0954
Lodewyk, M. W., Siebert, M. R., and Tantillo, D. J. (2012). Computational Prediction of 1H and 13C Chemical Shifts: A Useful Tool for Natural Product, Mechanistic, and Synthetic Organic Chemistry. Chem. Rev. 112, 1839–1862. doi:10.1021/cr200106v
Luo, D.-Q., Gao, Y., Gao, J. M., Wang, F., Yang, X. L., Liu, J. K., et al. (2006). Humulane-type Sesquiterpenoids from the Mushroom Lactarius Mitissimus. J. Nat. Prod. 69, 1354–1357. doi:10.1021/np060153l
Pan, G., Zhao, Y., Ren, S., Liu, F., Xu, Q., Pan, W., et al. (2021). Indole-terpenoids with Anti-inflammatory Activities from Penicillium Sp. HFF16 Associated with the Rhizosphere Soil of Cynanchum Bungei Decne. Front. Microbiol. 12, 710364. doi:10.3389/fmicb.2021.710364
Pinnamaneni, R. (2017). Nutritional and Medicinal Value of Papaya (Carica Papaya Linn.). Wjpps 6, 2559–2578. doi:10.20959/wjpps20178-9947
Pittayakhajonwut, P., Theerasilp, M., Kongsaeree, P., Rungrod, A., Tanticharoen, M., and Thebtaranonth, Y. (2002). Pughiinin A, a Sesquiterpene from the FungusKionochaeta pughiiBCC 3878. Planta Med. 68, 1017–1019. doi:10.1055/s-2002-35653
Pracht, P., Bohle, F., and Grimme, S. (2020). Automated Exploration of the Low-Energy Chemical Space with Fast Quantum Chemical Methods. Phys. Chem. Chem. Phys. 22, 7169–7192. doi:10.1039/C9CP06869D
Smith, S. G., and Goodman, J. M. (2010). Assigning Stereochemistry to Single Diastereoisomers by GIAO NMR Calculation: The DP4 Probability. J. Am. Chem. Soc. 132, 12946–12959. doi:10.1021/ja105035r
Smith, S. G., and Goodman, J. M. (2009). Assigning the Stereochemistry of Pairs of Diastereoisomers Using GIAO NMR Shift Calculation. J. Org. Chem. 74, 4597–4607. doi:10.1021/jo900408d
Stark, T., and Hofmann, T. (2005). Structures, Sensory Activity, and Dose/Response Functions of 2,5-Diketopiperazines in Roasted Cocoa Nibs (Theobroma Cacao). J. Agric. Food Chem. 53, 7222–7231. doi:10.1021/jf051313m
Strobel, G. A. (2003). Endophytes as Sources of Bioactive Products. Microbes Infect. 5, 535–544. doi:10.1016/S1286-4579(03)00073-X
Toyota, M., Omatsu, I., Braggins, J., and Asakawa, Y. (2004). New Humulane-type Sesquiterpenes from the Liverworts Tylimanthus Tenellus and Marchantia Emarginata Subsp. Tosana. Chem. Pharm. Bull. 52, 481–484. doi:10.1248/cpb.52.481
Uzma, F., Mohan, C. D., Hashem, A., Konappa, N. M., Rangappa, S., Kamath, P. V., et al. (2018). Endophytic Fungi-Alternative Sources of Cytotoxic Compounds: A Review. Front. Pharmacol. 9, 309. doi:10.3389/fphar.2018.00309
Wolinski, K., Hinton, J. F., and Pulay, P. (1990). Efficient Implementation of the Gauge-independent Atomic Orbital Method for NMR Chemical Shift Calculations. J. Am. Chem. Soc. 112, 8251–8260. doi:10.1021/ja00179a005
Xiao, N., Lou, M.-D., Lu, Y.-T., Yang, L.-L., Liu, Q., Liu, B., et al. (2017). Ginsenoside Rg5 Attenuates Hepatic Glucagon Response via Suppression of Succinate-Associated HIF-1α Induction in HFD-Fed Mice. Diabetologia 60, 1084–1093. doi:10.1007/s00125-017-4238-y
Zhang, H. W., Song, Y. C., and Tan, R. X. (2006). Biology and Chemistry of Endophytes. Nat. Prod. Rep. 23, 753–771. doi:10.1039/B609472B
Keywords: endophytic fungus, Penicillium sp., humulane-type sesquiterpenoid, anti-diabetic activity, cAMP accumulation
Citation: Wang F-R, Yang L, Kong F-D, Ma Q-Y, Xie Q-Y, Wu Y-G, Dai H-F, Chen P, Xiao N and Zhao Y-X (2021) Humulane-Type Macrocyclic Sesquiterpenoids From the Endophytic Fungus Penicillium sp. of Carica papaya. Front. Chem. 9:797858. doi: 10.3389/fchem.2021.797858
Received: 19 October 2021; Accepted: 15 November 2021;
Published: 16 December 2021.
Edited by:
Xiachang Wang, Nanjing University of Chinese Medicine, ChinaReviewed by:
Weaam Ebrahim, Mansoura University, EgyptCopyright © 2021 Wang, Yang, Kong, Ma, Xie, Wu, Dai, Chen, Xiao and Zhao. This is an open-access article distributed under the terms of the Creative Commons Attribution License (CC BY). The use, distribution or reproduction in other forums is permitted, provided the original author(s) and the copyright owner(s) are credited and that the original publication in this journal is cited, in accordance with accepted academic practice. No use, distribution or reproduction is permitted which does not comply with these terms.
*Correspondence: Ping Chen, Y2hlbnBpbmcwODIxM0AxNjMuY29t; Na Xiao, eGlhb25hMTk4NzA3QDEyNi5jb20=; You-Xing Zhao, emhhb3lvdXhpbmdAaXRiYi5vcmcuY24=
†These authors have contributed equally to this work
Disclaimer: All claims expressed in this article are solely those of the authors and do not necessarily represent those of their affiliated organizations, or those of the publisher, the editors and the reviewers. Any product that may be evaluated in this article or claim that may be made by its manufacturer is not guaranteed or endorsed by the publisher.
Research integrity at Frontiers
Learn more about the work of our research integrity team to safeguard the quality of each article we publish.