- 1Beijing Key Laboratory of Environmental Science and Engineering, School of Materials Science and Engineering, Beijing Institute of Technology, Beijing, China
- 2International Center for Quantum Materials, School of Physics, Peking University, Beijing, China
- 3Collaborative Innovation Center of Quantum Matter, Beijing, China
- 4Interdisciplinary Institute of Light-Element Quantum Materials and Research Center for Light-Element Advanced Materials, Peking University, Beijing, China
Interfacial water is closely related to many core scientific and technological issues, covering a broad range of fields, such as material science, geochemistry, electrochemistry and biology. The understanding of the structure and dynamics of interfacial water is the basis of dealing with a series of issues in science and technology. In recent years, atomic force microscopy (AFM) with ultrahigh resolution has become a very powerful option for the understanding of the complex structural and dynamic properties of interfacial water on solid surfaces. In this perspective, we provide an overview of the application of AFM in the study of two dimensional (2D) or three dimensional (3D) interfacial water, and present the prospect and challenges of the AFM-related techniques in experiments and simulations, in order to gain a better understanding of the physicochemical properties of interfacial water.
Introduction
Interfacial water is ubiquitous in nature and closely related to various issues both in fundamental research and technological applications, such as the dissolution of salts (Wandelt and Thurgate, 2002), desalination of seawater (Somorjai and Li, 2010), biological reactions (Tanaka et al., 2002), nanoconfined amorphous water (Manni et al., 2019) and lubricant systems (Urbakh et al., 2004). To gain a deep understanding of these important processes, the investigation of the structure and dynamic process like the rearrangement and growth mechanism of interfacial water on heterogeneous surfaces should be conducted. The symmetry of interfacial water is complex, for it shares boundaries with other substances. Therefore, it is more difficult to investigate interfacial water compared with bulk water which is well known to have 20 kinds of crystalline polymorph (ice Ih, ice Ic, ice II ∼ XIX) (Gasser et al., 2021). Particularly, the investigation of the growth process of interfacial water is extremely challenging, which requires non-invasive high resolution imaging, as the metastable or intermediate edge structures involved are rather fragile. With high sensitivity to the local environment and short range forces, AFM is very useful to detect the structure and dynamics of water molecules on a diversity of substrates, from insulating to metal substrate (Peng et al., 2018a). Until now, AFM imaging, together with ab initio calculation, classical molecular dynamics (MD) calculation and AFM simulation, has been applied to the investigation of the interfacial water on a wide variety of substrates, such as minerals (Putnis, 2014), biomolecules (Maver et al., 2016; Dufrene et al., 2017), and organic films (Verma and Sharma, 2010). Previously, we introduced the development of AFM technique both in experiments and simulations, as well as their applications in the study of interfacial water, with particular emphasis on the detection of the nanoclusters and one-dimensional chains (Cao et al., 2019). As a continuation, this perspective reviews the latest progresses in the utilization of AFM imaging in 2D and 3D interfacial water, including adsorbed water networks and electrolytes, as well as their growth processes. An outlook of future AFM study and a brief summary are presented in the end.
The Ultrahigh-Resolution Imaging of Interfacial Water on Various Substrates
Interfacial water molecules on solid surfaces could form various structures, which depends not only on the lattice spacing and symmetry, but also on the substrate chemical reactivity and water-substrate interaction (Hodgson and Haq, 2009). With the consideration of the lattice matching with bulk water, the close-packed surfaces of Ni, Pt, Ru and Au are ideal substrates for studying water adsorption (Thurmer and Nie, 2013; Shiotari et al., 2019; Ma et al., 2020). Besides, the structures and properties of interfacial water in ionic hydration systems, which are susceptible to the influences of ions in the environment, are of particular importance to tackle many scientific and technical issues (Strmcnik et al., 2009), for instance, the kinetics of hydrogen evolution and oxidation reactions. In the following, we discuss the recent advances in the structural and dynamic properties of interfacial water and electrolytes on various substrates with the application of AFM.
Characterization of 2D Ice on Various Substrates
1) Characterization of 2D Water Networks on Ni(111)
Ni commonly used in alloys and cell electrodes, direct observations of water adsorptions on Ni-based materials are helpful to understand various surface phenomena, such as corrosion, wetting, electrochemical reactions and the water-related heterogeneous catalysis (Michaelides et al., 2004). Recently, with low-temperature AFM, the structures of 2D water networks on the terraces and at the step edges of Ni(111) were observed (Shiotari et al., 2019). It was found that at 78K, water molecules are assembled in disorder, while at 140–145K, they rearrange the structure and form small clusters with a central cyclic (H2O)6, which are possibly the nucleus of the initial growth of H-bonding networks (Nakamura and Ito, 2004). Such small clusters then disappear after annealed at 150K, homogeneous huge islands of the (√28 × √28)R19° superstructure starting to dominate on the terraces, which are composed of pentagonal, hexagonal and heptagonal rings (Figures 1A,B). Meanwhile, at the monoatomic step edge of Ni(111), another water network is formed, containing pentagonal and octagonal rings along the step direction with a periodicity of four times Ni interatomic distance (4a0) (Figures 1C–F). Such a periodic 1D pentagonal-octagonal network closely resembles the domain boundaries of H2O/Ru (0001) (Maier et al., 2016), the water islands on stepped Cu(551) surface (Lin et al., 2018), and the defect rows in the second water layer on SnPt (111) (Gerrard et al., 2019). This indicates that the 1D pentagonal-octagonal network structure is a representative defect existing in the interfacial water layers on metal surfaces. Moreover, 2D water networks on metal surfaces, such as Pt (111) (Nie et al., 2010) and Ru (0001) (Maier et al., 2014), have also been studied by AFM. Variations in water networks on different surfaces have been reported, ranging from close-packed metal surfaces to the insulating surfaces. These studies revealed the adsorption structures of water on different metals, which is important for a deep understanding of the catalytic reactivity and the wettability on terraces and at step edges.
2) Determination of Intrinsic Reconstruction of Ice-I Surfaces on Rh (111) and Pt (111)
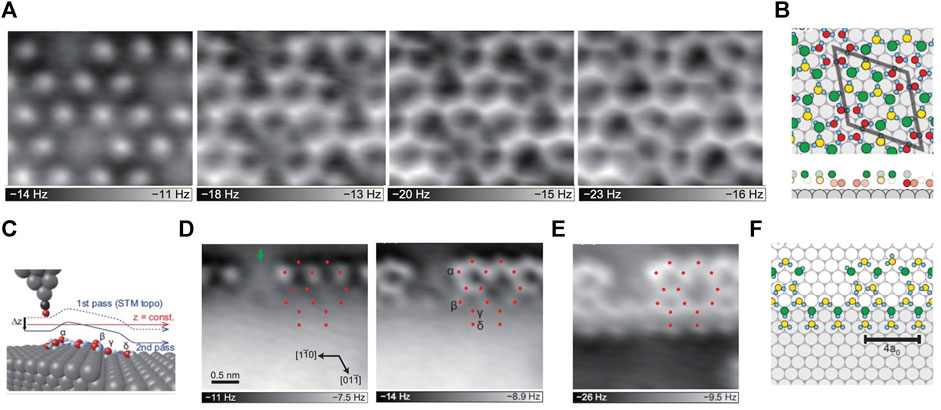
FIGURE 1. 2D water networks on Ni(111) (A) AFM images of the 2D water networks of the same area on the terraces, the tip height decreases from left to right (B) The proposed structure of the water monolayer based on the AFM images. The gray, cyan, green, yellow and red spheres represent Ni, H, topmost O, middle O and bottommost O atoms, respectively; the unit cell of (√28 × √28) R19 is represented by the gray rhombus; the bottom panels are the side-view of the above structures, without H atoms for clarity (C) The schematic diagram of the tip and step. The gray, cyan, black and red spheres represent Ni, H, C and O atoms, respectively (D) Constant-height AFM images of the water network at the step edge, the tip height decreases from left to right (E) AFM image of the same region in (D), but the tip trajectory is consistent with that of the STM image, as the solid blue line in (C). The red dots in (D–E) represent the O-atom positions (F) The water network structure at the step edge proposed based on the AFM images. Adapted with permission from Shiotari, et al., 2019. Physical Review Materials 3(9): 093,001 (Shiotari et al., 2019).
Compared with non-contact AFM using quartz tuning fork sensors (Thurmer and Nie, 2013), AFM with a silicon cantilever and optical interferometer possesses a higher sensitivity in interatomic force detection (Kaiser et al., 2007). Based on this technique, Kawakami et al. studied the atomic structures of ice-I surfaces on Rh (111) and Pt (111), and found that the AFM images of the terraces of ice on both Rh (111) and Pt (111) have rather similar characteristics, e.g., random distribution, number reduction and (2 × 2) order of dangling H atoms, and (2 × 2) order distortion of O lattice (Kawakami et al., 2020). However, only one crystalline phase of ice (ice-Ih) is observed on Rh (111), while the crystalline phase of ice on Pt (111) varies with the thickness of the ice film, e.g., the number of ice layers (Thurmer and Nie, 2013). That is to say, it is ice-Ic at medium thickness (10–50 BLs) and ice-Ih in a dominant state at other thicknesses. Although the crystalline phase of ice on Pt substrate differs at different ice thickness, their densities of the dangling H atoms are the same, and their AFM images are quite similar with a (2 × 2) order. This reveals that the changes in the H-atom orientation and the interface effects of ice Ih and ice Ic have a negligible influence on the surface reconstruction. Moreover, it is found that the (2 × 2) order reconstruction of ice-I surfaces, including the sparse distribution of the residual dangling H atoms and the removal of dangling H atoms, is caused by the electrostatic repulsion between the dangling H atoms. The above findings about the molecular reconstruction are crucial to understand chemical and physical phenomena, such as the growth and melting of ice, and chemical reactions (Kawakami et al., 2020).
3) Atomic Imaging of Edge Structure and Growth of a 2D Hexagonal Ice
Although the structure of 2D water network on surface has been widely studied (Nie et al., 2010; Kiselev et al., 2017), it is rather challenging to capture the atomic details of metastable or intermediate edge structures during ice growth. This is due to the fact that the fragileness and the short life span of intermediate edge structures call for fast and non-invasive detection (Lupi et al., 2014). Recently, Ma et al. achieved a high resolution imaging of the edge structures of a 2D bilayer hexagonal ice on Au (111) by weakly perturbative AFM with a CO-functionalized tip (Ma et al., 2020). Combining experiments with theoretical calculations, the orientation of each water molecule, namely the O-H directionality in the 2D bilayer ice, was revealed. Moreover, a new type of edge, which coexists with the zigzag edge in 2D hexagonal ice and along the armchair direction but is reconstructed into a complex periodical structure consisting of 5756-membered rings, was also found. Ma et al. also achieved the freeze and imaging of various intermediate structures at the two types of edges, and rebuilt distinct ice growth mechanisms (Figures 2A,B). Combined with ab initio calculations and classical MD simulations, the growth at the zigzag edge was found to follow a collective bridging mechanism facilitated by a periodic array of pentagons, in contrast to a local seeding growth mode with a manifestation of the 5756–5656 interconversion at the armchair edge (Figures 2C,D). Furthermore, not only the distance between water molecules but also the commensurability between ice lattice and the substrate was found to have a negligible effect on the relative stability of the two types of edges, demonstrating the generality of observed growth behavior. Such new growth pattern is in sharp contrast to the conventional growth mode of bilayer hexagonal ices and 2D hexagonal material, shedding light on the physical mechanism of ice growth on hydrophobic substrates or under hydrophobic confinement.
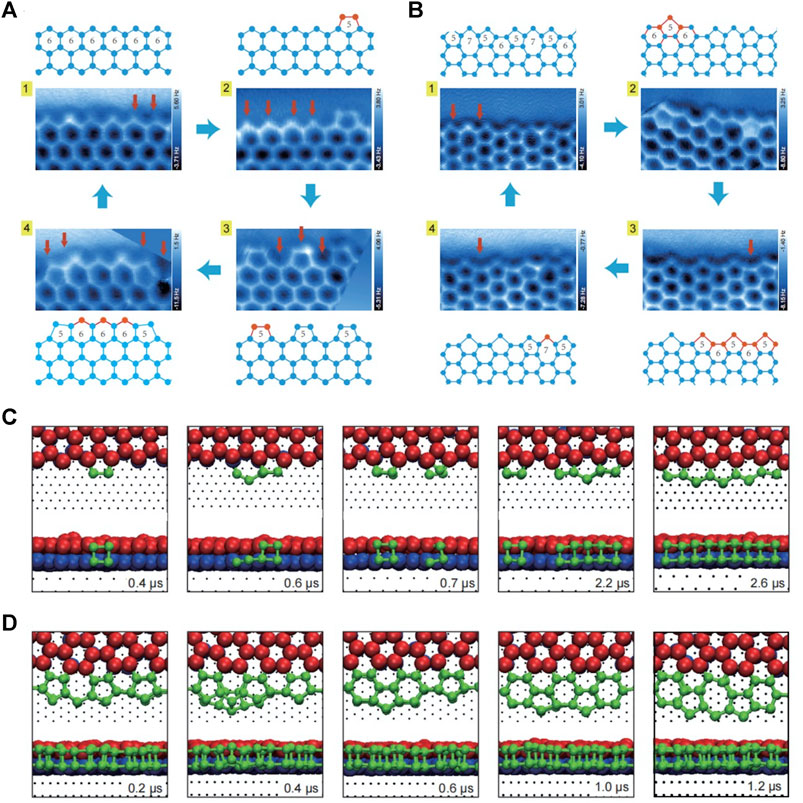
FIGURE 2. The growth process of zigzag and armchair edges of the 2D bilayer hexagonal ice formed on Au(111) (A–B) Constant-height AFM images and corresponding models of the steady state (1) and metastable states (2–4) of the zigzag (A) and armchair edges (B) (C–D) Time-elapsed snapshots during the growth of zigzag (C) and armchair edges (D) obtained by MD simulation. In (A–B), the proposed growing process (from one to 4) is indicated by blue arrows; the addition of one bilayer water pair is labeled by a red arrow in AFM images; the red balls and sticks in ball-stick models represent the newly added bilayer water pairs, while the blue ones represent the existing structures. In (C–D), the simulation times are shown on bottom right of each snapshot, the bottom- and top-layer water molecules of the preexistent bilayer ice are denoted by the blue and red spheres, respectively; the newly deposited water molecules are denoted by the green spheres. Adapted with permission from Ma, et al. 2020. Nature 577(7788): 60–63 (Ma et al., 2020).
Probing the Adsorption of Electrolytes at Liquid/Kaolinite Interfaces
The above mentioned works were all performed in a vacuum at low temperature using non-contact AFM (nc-AFM) to guarantee that the sample would not be disturbed and the tip would not be contaminated. However, in case of structure probing of the solid/liquid interface at room temperature, nonnegligible disturbance and tip contamination becomes unavoidable. As a result, nc-AFM imaging under such conditions becomes very difficult. Therefore, the contact mode AFM, whose tip contacts the measured sample during imaging, is more appropriate here due to its higher stability in such cases. With the contact mode AFM, surface structures in liquids could be characterized.
Adsorption of electrolytes (ions) at liquid/solid interfaces plays a critical role in nanomaterial processing to gain desired structures and morphology, since it can alter physicochemical nature of materials (Nelson and Schwartz, 2013; DeWalt-Kerian et al., 2017). Various electrical double layer (EDL) models have been used to describe the ion adsorption on charged surfaces in liquids, but the model-dependent approaches lead to inaccurate predictions of ion adsorption due to inevitable assumptions, for example, whether the diffusion and thermal motion of ions are taken into account for molecular modeling (Modi and Fuerstenau, 1957; Ardizzone et al., 1982). Without any approximation or assumption, Chang et al. studied the electrolytes adsorption at liquid/kaolinite interfaces using an in situ AFM (Chang et al., 2021). They directly visualized the ions located in the EDL and adsorbed on the anisotropic kaolinite, and detected the changes of surface structure of kaolinite during the adsorption of mono- and divalent metal cations.
As a result, it was found that the adsorption of K+ and Cl− in solution cannot change the lattice structure of kaolinite surfaces. Unlike monovalent ions, the adsorption of calcium ions can lead to the changes of the surface morphology at high pH, presenting in a specific adsorption mode. This suggests the strong and direct binding between cations and substrates. Moreover, it was first found that the specific adsorption caused by the hydrolysis of Ca2+ can weaken the electrostatic interaction and decrease the ionic charge. To conclude, AFM is a very useful imaging tool to disclose ion adsorption at the liquid/kaolinite interface and it can be extended to other interfaces.
Perspective
Identification of Multiple Chemical Species in 2D H-Bonding Networks
Identification of multiple chemical species such as OH− or H3O+ solvated in 2D and 3D H-bonding water networks is significant for deepening our knowledge about electrochemical reactions, such as hydrogen or oxygen evolution reaction. Previous studies of the systems like partially dissociated water networks or hydrated protons solvated in H-bonding networks, have been mainly investigated by vibrational spectroscopy such as X-ray photoelectron spectroscopy, infrared and Raman spectroscopies, which can determine the extent of partial dissociation (Meier et al., 2018), or the molecular structures of hydrated protons in water clusters (Fournier et al., 2015). However, these techniques suffer from the difficulty of spectral assignment and the limited spatial resolution (Shen and Ostroverkhov, 2006). In contrast, nc-AFM with a CO-terminated tip which can be operated in a vacuum at low temperatures, shows the ability to imaging the water molecule with sub-molecular resolution in a nearly non-invasive manner (Peng et al., 2018a; Peng et al., 2018b). In this way, partially dissociated water networks on various substrates, like Fe3O4(001) (Meier et al., 2018) and Cu(110) (Shiotari and Sugimoto, 2017) have been studied. However, the identification of chemical species related to water just based on the AFM images is difficult due to the fact that AFM is not very sensitive to H atom, which is small and interacts weakly with the tip. Fortunately, this difficulty can be overcome by the aid of DFT calculation and AFM image simulations (Hapala et al., 2014). Nevertheless, due to the high similarity of H3O+ to H2O, the imaging and identification of H3O+ in the H-bonding network of water is much more challenging, and the related AFM-based studies have not been reported yet. It is believed that by further improving the resolution and sensitivity of qPlus-AFM, this will soon no longer be an issue.
3D Atomic Force Microscopy
Interfacial aqueous layer plays an intermediate or significant role in many aspects, such as corrosion, nanopatterning and adhesion in material science (Garcia et al., 2014), protein folding, inactivation and molecular identification in molecular biology (Laage et al., 2017), the dissolution and growth of ore in geology (Laanait et al., 2015). To fully understand such phenomena and reveal the detailed interactions of liquid water on different solid interface, the detailed 3D structure of hydration layer needs to be established. While nc-AFM is usually affected by the motion of liquid molecules, 3D-AFM with an authentic 3D depth and a high spatial resolution of 1–3 Å is the newest and most successful attempt to achieve this goal, making an accurate imaging of the atom position. In fact, it has already been applied to uncover the nature of a few liquid/solid interfaces, achieving high resolution imaging of water layers at the flat liquid/mica interface (Fukuma et al., 2010) and on non-flat surfaces, such as lipid membranes (Kobayashi et al., 2013), proteins (Herruzo et al., 2013), or DNA (Kuchuk and Sivan, 2018).
The achievements of 3D-AFM made in the investigation of interfacial water on stiff crystalline surfaces and in soft biomolecules (Fukuma and Garcia, 2018) highlight its widely potential applications in catalysis, electrochemistry, cell and molecular biology. Nevertheless, there are still some scientific and instrumental challenges for the promotion of 3D-AFM. For instance, the 3D-AFM-based z-depth imaging, with a maximum of 10 nm (Martin-Jimenez et al., 2016) and a common value of 1 nm, is ideal for the exploration of the adsorption of ions and solvent molecules on flat substrates (Jariwala et al., 2017), but it is rather small for detecting the hydration structures on non-flat systems, such as isolated proteins, nanostructured surfaces and cells (Fukuma and Garcia, 2018). Thus, it is of great significance to improve the sensitivity, resolution and scanning speed of 3D-AFM for its broader applications, e.g., the study of 3D distribution of flexible surface structures, such as chain polymers or lipid headgroups on a biological membrane surface, and in-situ observation.
Multifunctional AFM Techniques for Aqueous Battery Characterization
Being capable of performing under realistic in-situ conditions, multifunctional AFM is conductive to revealing the detailed mechanisms of scientific phenomena and technological processes, the extremely important evolution of liquid/solid interface in battery, for instance. At present, different kinds of multifunctional AFMs have been used in the research of lithium-ion batteries with organic solvents as electrolytes (Yoon et al., 2016), among which electrochemical AFM (EC-AFM) was more widely utilized than others. It can characterize the morphology evolution on anodes (Jeong et al., 2001) and cathodes (Wu et al., 2017), the formation of Li-dendrite (Shen et al., 2018) and the kinetic process of solid electrolyte interface (Novak et al., 2001). Electro-chemical strain microscopy is a very useful tool to measure the volumetric strain driven by potential pulse, and to detect the microscopic details of electronic or ionic transport processes (Yang et al., 2015). Meanwhile, the ability to detect the surface potential between the tip and the sample measured, allows Kelvin probe force microscopy to investigate the relationship between ion distributions and battery performance, as well as the impact of surface charges on ionic intercalation (Luchkin et al., 2014). Moreover, combined with infrared or Raman spectroscopy and mass spectrometry, AFM can effectively overcome the shortcoming of being unable to distinguish the composition of the detected substances, greatly expanding its application in energy fields.
Aqueous battery with water as the electrolyte solvent is the most potential technology for large-scale energy storage systems of wind and solar photovoltaic power generation (Wang et al., 2018). This is due to its superiority of low cost, environmental friendliness, high safety and theoretical capacity (Glatz et al., 2019), while the application of lithium-ion batteries is hindered by its high cost, low safety and environmental issues. However, studies of aqueous batteries are still at the early stage, and further investigations need to be carried out, including in-depth structures and dynamic properties of aqueous battery, as well as their changes in the electro-chemical processes (Zhou et al., 2020). Despite their powerfulness in nanoscale characterization, all types of multifunctional AFMs have limitations in spatial and temporal resolution. For example, EC-AFM has limited scanning rate, thus some fast interface reactions cannot be monitored in real time. In addition, drift during scanning make it difficult to obtain reliable surface characteristics (Chen et al., 2020). These limitations are expected to be overcome in the near future by developing low-noise cantilever deflection sensor (Fukuma et al., 2005) and drift-compensated data acquisition schemes (Abe et al., 2007). Besides, optimal tip preparation is often a key issue in AFM-based techniques such as tip enhanced Raman spectroscopy to ensure high enhancement and good reproducibility (Toca-Herrera, 2019).
Machine Learning to Enhance AFM Interpretation
The interpretation of AFM signals, especially the 3D features appearing in the submolecular-resolution 3D-AFM images, has been achieved by introducing MD simulations. In 2015, with the comparison between the result of 3D-AFM experiment and MD simulation, it was found that the activities between the uppermost surface atoms and tip apex predominantly affected the tip-sample interaction (Fukuma et al., 2015). This finding provides a qualitative answer to the question on the imaging mechanism: why the intrinsic 3D hydration structure (without the AFM tip) is similar to the sub-nanometer resolution contrast appearing in 3D-AFM images.
However, MD simulation has been mainly applied to study the pure water on uniform and rigid surfaces (Miyazawa et al., 2016; Vilhena et al., 2016). It is impractical to utilize MD to analyze 3D-AFM features in many complex problems, owing to its high computational costs. Currently, there is still a large gap between the AFM simulation and experiments. Many issues under more complicated conditions remain to be tackled theoretically, such as the treatment of ions, the influence of ions on force contrast, and the appropriate construction of tip model. In recent years, with the emergence of more and more powerful, effective and efficient algorithms for object detection, classification, image segmentation and quality enhancement, machine learning (ML) has been gradually employed in many physical (Karniadakis et al., 2021) and chemical (Mater and Coote, 2019) investigations, including the applications in solving related questions about AFM.
Using convolutional neural networks, Gordon et al. discerned several spatially correlated patterns of self-organized nanoparticles based on the mixed, highly varied experimental AFM images (Gordon et al., 2020). What’s more, Alldritt et al. developed a deep learning framework that enables a unique molecular structure descriptor to match a suit of AFM images, allowing direct structure determination of organic molecules based on AFM images (Alldritt et al., 2020). Going even further, applying ML to identify configurations and structural evolution of larger and more complex systems, like liquid and ionic hydration layer, requires a great deal of effective, high quality training data obtained from simulation or experiment. As a result, it will lead to the need for a delicate balance between accuracy increasing and resource consuming. Nevertheless, ML is still a promising tool for the in-depth study of all kinds of complex water-related systems, where traditional interpretation means have been a failure or cannot even be attempted. In other words, it could save researchers from tedious and time-consuming repetitive work, and uncover hidden patterns or properties that are invisible to the human eye. Therefore, the development of ML technique will play a crucial role in prompting AFM at the forefront of the characterization technologies.
Conclusion
This perspective presents recent applications of AFM in the study of 2D and 3D interfacial water on solid interfaces, including structures of water networks, ice reconstructions, edge structures and growth mechanism of 2D hexagonal ice as well as the adsorption of electrolytes. The prospect of AFM is to study the more intricate and subsistent interfacial water-related systems, which needs to employ 3D- and multifunction AFM. Furthermore, the interpretation of AFM images of the intricate liquid/solid interface, especially the evolution of 3D-AFM features in AFM image, is extremely challenging due to the fact that AFM signals can be affected by environmental factors. Thus, it urgently requires a further development of effective interpretation approaches of AFM. ML is the most likely solution for the related questions about AFM imaging in complex systems associated with water, when traditional interpretation methods fail or cannot even be attempted. It is reasonable to predict that, with the improvement of AFM imaging and ML techniques, there will be a rapid increase in the atomic-scale investigations of the structural and dynamic properties of liquid/solid interfaces in the foreseeable future.
Data Availability Statement
The original contributions presented in the study are included in the article/Supplementary Material, further inquiries can be directed to the corresponding authors.
Author Contributions
All authors listed have made a substantial, direct, and intellectual contribution to the work and approved it for publication.
Funding
We are thankful for the financial support from the National Natural Science Foundation of China under Grant No. 11935002, the National Key R&D Program under Grant No. 2016YFA0300901, the National Postdoctoral Program for Innovative Talents under Grant No. BX2021040 and the China Postdoctoral Science Foundation under Grant No. 2021M690408.
Conflict of Interest
The authors declare that the research was conducted in the absence of any commercial or financial relationships that could be construed as a potential conflict of interest.
The reviewer (RM) declared a past co-authorship with the authors (DC, LX) to the handling Editor.
Publisher’s Note
All claims expressed in this article are solely those of the authors and do not necessarily represent those of their affiliated organizations, or those of the publisher, the editors and the reviewers. Any product that may be evaluated in this article, or claim that may be made by its manufacturer, is not guaranteed or endorsed by the publisher.
References
Abe, M., Sugimoto, Y., Namikawa, T., Morita, K., Oyabu, N., and Morita, S. (2007). Drift-compensated Data Acquisition Performed at Room Temperature with Frequency Modulation Atomic Force Microscopy. Appl. Phys. Lett. 90 (20), 203103. doi:10.1063/1.2739410
Alldritt, B., Hapala, P., Oinonen, N., Urtev, F., Krejci, O., Federici Canova, F., et al. (2020). Automated Structure Discovery in Atomic Force Microscopy. Sci. Adv. 6 (9), eaay6913. ARTN. doi:10.1126/sciadv.aay6913
Ardizzone, S., Formaro, L., and Lyklema, J. (1982). Adsorption from Mixtures Containing Mono- and Bivalent Cations on Insoluble Oxides and a Revision of the Interpretation of Points of Zero Charge Obtained by Titration. J. Electroanalytical Chem. Interfacial Electrochemistry 133 (1), 147–156. doi:10.1016/0022-0728(82)87013-7
Cao, D., Song, Y., Peng, J., Ma, R., Guo, J., Chen, J., et al. (2019). Advances in Atomic Force Microscopy: Weakly Perturbative Imaging of the Interfacial Water. Front. Chem. 7, 626. doi:10.3389/fchem.2019.00626
Chang, J., Liu, B., Grundy, J. S., Shao, H., Manica, R., Li, Z., et al. (2021). Probing Specific Adsorption of Electrolytes at Kaolinite-Aqueous Interfaces by Atomic Force Microscopy. J. Phys. Chem. Lett. 12 (9), 2406–2412. doi:10.1021/acs.jpclett.0c03521
Chen, H., Qin, Z., He, M., Liu, Y., and Wu, Z. (2020). Application of Electrochemical Atomic Force Microscopy (EC-AFM) in the Corrosion Study of Metallic Materials. Materials 13 (3), 668. doi:10.3390/ma13030668
DeWalt-Kerian, E. L., Kim, S., Azam, M. S., Zeng, H., Liu, Q., and Gibbs, J. M. (2017). pH-Dependent Inversion of Hofmeister Trends in the Water Structure of the Electrical Double Layer. J. Phys. Chem. Lett. 8 (13), 2855–2861. doi:10.1021/acs.jpclett.7b01005
Dufrêne, Y. F., Ando, T., Garcia, R., Alsteens, D., Martinez-Martin, D., Engel, A., et al. (2017). Imaging Modes of Atomic Force Microscopy for Application in Molecular and Cell Biology. Nat. Nanotech 12 (4), 295–307. doi:10.1038/Nnano.2017.45
Fournier, J. A., Wolke, C. T., Johnson, M. A., Odbadrakh, T. T., Jordan, K. D., Kathmann, S. M., et al. (2015). Snapshots of Proton Accommodation at a Microscopic Water Surface: Understanding the Vibrational Spectral Signatures of the Charge Defect in Cryogenically Cooled H+(H2O)n=2-28 Clusters. J. Phys. Chem. A. 119 (36), 9425–9440. doi:10.1021/acs.jpca.5b04355
Fukuma, T., Ueda, Y., Yoshioka, S., and Asakawa, H. (2010). Atomic-Scale Distribution of Water Molecules at the Mica-Water Interface Visualized by Three-Dimensional Scanning Force Microscopy. Phys. Rev. Lett. 104 (1), 016101. doi:10.1103/PhysRevLett.104.016101
Fukuma, T., and Garcia, R. (2018). Atomic- and Molecular-Resolution Mapping of Solid-Liquid Interfaces by 3D Atomic Force Microscopy. ACS Nano 12 (12), 11785–11797. doi:10.1021/acsnano.8b07216
Fukuma, T., Kimura, M., Kobayashi, K., Matsushige, K., and Yamada, H. (2005). Development of Low Noise Cantilever Deflection Sensor for Multienvironment Frequency-Modulation Atomic Force Microscopy. Rev. Scientific Instr. 76 (5), 053704. doi:10.1063/1.1896938
Fukuma, T., Reischl, B., Kobayashi, N., Spijker, P., Canova, F. F., Miyazawa, K., et al. (2015). Mechanism of Atomic Force Microscopy Imaging of Three-Dimensional Hydration Structures at a Solid-Liquid Interface. Phys. Rev. B 92 (15), 155412. ARTN 15541210. doi:10.1103/physrevb.92.155412
Garcia, R., Knoll, A. W., and Riedo, E. (2014). Advanced Scanning Probe Lithography. Nat. Nanotech 9 (8), 577–587. doi:10.1038/Nnano.2014.157
Gasser, T. M., Thoeny, A. V., Fortes, A. D., and Loerting, T. (2021). Structural Characterization of Ice XIX as the Second Polymorph Related to Ice VI. Nat. Commun. 12 (1), 1128. ARTN. doi:10.1038/s41467-021-21161-z
Gerrard, N., Gattinoni, C., McBride, F., Michaelides, A., and Hodgson, A. (2019). Strain Relief during Ice Growth on a Hexagonal Template. J. Am. Chem. Soc. 141 (21), 8599–8607. doi:10.1021/jacs.9b03311
Glatz, H., Lizundia, E., Pacifico, F., and Kundu, D. (2019). An Organic Cathode Based Dual-Ion Aqueous Zinc Battery Enabled by a Cellulose Membrane. ACS Appl. Energ. Mater. 2 (2), 1288–1294. doi:10.1021/acsaem.8b01851
Gordon, O. M., Hodgkinson, J. E. A., Farley, S. M., Hunsicker, E. L., and Moriarty, P. J. (2020). Automated Searching and Identification of Self-Organized Nanostructures. Nano Lett. 20 (10), 7688–7693. doi:10.1021/acs.nanolett.0c03213
Gross, L., Mohn, F., Moll, N., Liljeroth, P., and Meyer, G. (2009). The Chemical Structure of a Molecule Resolved by Atomic Force Microscopy. Science 325 (5944), 1110–1114. doi:10.1126/science.1176210
Hapala, P., Kichin, G., Wagner, C., Tautz, F. S., Temirov, R., and Jelinek, P. (2014). Mechanism of High-Resolution STM/AFM Imaging with Functionalized Tips. Phys. Rev. B 90 (8), 085421. doi:10.1103/PhysRevB.90.085421
Herruzo, E. T., Asakawa, H., Fukuma, T., and Garcia, R. (2013). Three-dimensional Quantitative Force Maps in Liquid with 10 Piconewton, Angstrom and Sub-minute Resolutions. Nanoscale 5 (7), 2678–2685. doi:10.1039/c2nr33051b
Hodgson, A., and Haq, S. (2009). Water Adsorption and the Wetting of Metal Surfaces. Surf. Sci. Rep. 64 (9), 381–451. doi:10.1016/j.surfrep.2009.07.001
Inami, E., and Sugimoto, Y. (2015). Accurate Extraction of Electrostatic Force by a Voltage-Pulse Force Spectroscopy. Phys. Rev. Lett. 114 (24), 246102. ARTN 24610210. doi:10.1103/physrevlett.114.246102
Jariwala, D., Marks, T. J., and Hersam, M. C. (2017). Mixed-dimensional van der Waals heterostructures. Nat. Mater 16 (2), 170–181. doi:10.1038/Nmat4703
Jeong, S.-K., Inaba, M., Mogi, R., Iriyama, Y., Abe, T., and Ogumi, Z. (2001). Surface Film Formation on a Graphite Negative Electrode in Lithium-Ion Batteries: Atomic Force Microscopy Study on the Effects of Film-Forming Additives in Propylene Carbonate Solutions. Langmuir 17 (26), 8281–8286. doi:10.1021/la015553h
Jesse, S., Balke, N., Eliseev, E., Tselev, A., Dudney, N. J., Morozovska, A. N., et al. (2011). Direct Mapping of Ionic Transport in a Si Anode on the Nanoscale: Time Domain Electrochemical Strain Spectroscopy Study. Acs Nano 5 (12), 9682–9695. doi:10.1021/nn203141g
Kaiser, U., Schwarz, A., and Wiesendanger, R. (2007). Magnetic Exchange Force Microscopy with Atomic Resolution. Nature 446 (7135), 522–525. doi:10.1038/nature05617
Karniadakis, G. E., Kevrekidis, I. G., Lu, L., Perdikaris, P., Wang, S., and Yang, L. (2021). Physics-informed Machine Learning. Nat. Rev. Phys. 3 (6), 422–440. doi:10.1038/s42254-021-00314-5
Kawakami, N., Iwata, K., Shiotari, A., and Sugimoto, Y. (2020). Intrinsic Reconstruction of Ice-I Surfaces. Sci. Adv. 6 (37), eabb7986. doi:10.1126/sciadv.abb7986
Kiselev, A., Bachmann, F., Pedevilla, P., Cox, S. J., Michaelides, A., Gerthsen, D., et al. (2017). Active Sites in Heterogeneous Ice Nucleation-The Example of K-Rich Feldspars. Science 355 (6323), 367–371. doi:10.1126/science.aai8034
Kobayashi, K., Oyabu, N., Kimura, K., Ido, S., Suzuki, K., Imai, T., et al. (2013). Visualization of Hydration Layers on Muscovite Mica in Aqueous Solution by Frequency-Modulation Atomic Force Microscopy. J. Chem. Phys. 138 (18), 184704. doi:10.1063/1.4803742
Kuchuk, K., and Sivan, U. (2018). Hydration Structure of a Single DNA Molecule Revealed by Frequency-Modulation Atomic Force Microscopy. Nano Lett. 18 (4), 2733–2737. doi:10.1021/acs.nanolett.8b00854
Laage, D., Elsaesser, T., and Hynes, J. T. (2017). Water Dynamics in the Hydration Shells of Biomolecules. Chem. Rev. 117 (16), 10694–10725. doi:10.1021/acs.chemrev.6b00765
Laanait, N., Callagon, E. B. R., Zhang, Z., Sturchio, N. C., Lee, S. S., and Fenter, P. (2015). X-ray-driven Reaction Front Dynamics at Calcite-Water Interfaces. Science 349 (6254), 1330–1334. doi:10.1126/science.aab3272
Lin, C., Avidor, N., Corem, G., Godsi, O., Alexandrowicz, G., Darling, G. R., et al. (2018). Two-Dimensional Wetting of a Stepped Copper Surface. Phys. Rev. Lett. 120 (7), 076101. doi:10.1103/PhysRevLett.120.076101
Luchkin, S. Y., Amanieu, H.-Y., Rosato, D., and Kholkin, A. L. (2014). Li Distribution in Graphite Anodes: A Kelvin Probe Force Microscopy Approach. J. Power Sourc. 268, 887–894. doi:10.1016/j.jpowsour.2014.06.143
Lupi, L., Kastelowitz, N., and Molinero, V. (2014). Vapor Deposition of Water on Graphitic Surfaces: Formation of Amorphous Ice, Bilayer Ice, Ice I, and Liquid Water. J. Chem. Phys. 141 (18), 18C508. Artn 18c50810. doi:10.1063/1.4895543
Ma, R., Cao, D., Zhu, C., Tian, Y., Peng, J., Guo, J., et al. (2020). Atomic Imaging of the Edge Structure and Growth of a Two-Dimensional Hexagonal Ice. Nature 577 (7788), 60–63. doi:10.1038/s41586-019-1853-4
Maier, S., Lechner, B. A. J., Somorjai, G. A., and Salmeron, M. (2016). Growth and Structure of the First Layers of Ice on Ru(0001) and Pt(111). J. Am. Chem. Soc. 138 (9), 3145–3151. doi:10.1021/jacs.5b13133
Maier, S., Stass, I., Cerdá, J. I., and Salmeron, M. (2014). Unveiling the Mechanism of Water Partial Dissociation on Ru(0001). Phys. Rev. Lett. 112 (12), 126101. doi:10.1103/physrevlett.112.126101
Martin-Jimenez, D., Chacon, E., Tarazona, P., and Garcia, R. (2016). Atomically Resolved Three-Dimensional Structures of Electrolyte Aqueous Solutions Near a Solid Surface. Nat. Commun. 7, 12164. doi:10.1038/ncomms12164
Mater, A. C., and Coote, M. L. (2019). Deep Learning in Chemistry. J. Chem. Inf. Model. 59 (6), 2545–2559. doi:10.1021/acs.jcim.9b00266
Maver, U., Velnar, T., Gaberšček, M., Planinšek, O., and Finšgar, M. (2016). Recent Progressive Use of Atomic Force Microscopy in Biomedical Applications. Trac Trends Anal. Chem. 80, 96–111. doi:10.1016/j.trac.2016.03.014
Meier, M., Hulva, J., Jakub, Z., Pavelec, J., Setvin, M., Bliem, R., et al. (2018). Water Agglomerates on Fe3O4(001). Proc. Natl. Acad. Sci. USA 115 (25), E5642–E5650. doi:10.1073/pnas.1801661115
Michaelides, A., Alavi, A., and King, D. A. (2004). Insight into H2O-Ice Adsorption and Dissociation on Metal Surfaces from First-Principles Simulations. Phys. Rev. B 69 (11), 113404. doi:10.1103/PhysRevB.69.113404
Miyazawa, K., Kobayashi, N., Watkins, M., Shluger, A. L., Amano, K.-i., and Fukuma, T. (2016). A Relationship between Three-Dimensional Surface Hydration Structures and Force Distribution Measured by Atomic Force Microscopy. Nanoscale 8 (13), 7334–7342. doi:10.1039/c5nr08092d
Modi, H. J., and Fuerstenau, D. W. (1957). Streaming Potential Studies on Corundum in Aqueous Solutions of Inorganic Electrolytes. J. Phys. Chem. 61(5), 640–643. doi:10.1021/j150551a029
Nakamura, M., and Ito, M. (2004). Ring Hexamer like Cluster Molecules of Water Formed on a Ni(111) Surface. Chem. Phys. Lett. 384 (4-6), 256–261. doi:10.1016/j.cplett.2003.11.110
Nelson, N., and Schwartz, D. K. (2013). Specific Ion (Hofmeister) Effects on Adsorption, Desorption, and Diffusion at the Solid-Aqueous Interface. J. Phys. Chem. Lett. 4 (23), 4064–4068. doi:10.1021/jz402265y
Nie, S., Feibelman, P. J., Bartelt, N. C., and Thürmer, K. (2010). Pentagons and Heptagons in the First Water Layer on Pt(111). Phys. Rev. Lett. 105 (2), 026102. doi:10.1103/PhysRevLett.105.026102
Novák, P., Joho, F., Lanz, M., Rykart, B., Panitz, J.-C., Alliata, D., et al. (2001). The Complex Electrochemistry of Graphite Electrodes in Lithium-Ion Batteries. J. Power Sourc. 97-98, 39–46. doi:10.1016/s0378-7753(01)00586-9
Peng, J., Cao, D., He, Z., Guo, J., Hapala, P., Ma, R., et al. (2018a). The Effect of Hydration Number on the Interfacial Transport of Sodium Ions. Nature 557 (7707), 701–705. doi:10.1038/s41586-018-0122-2
Peng, J., Guo, J., Hapala, P., Cao, D., Ma, R., Cheng, B., et al. (2018b). Weakly Perturbative Imaging of Interfacial Water with Submolecular Resolution by Atomic Force Microscopy. Nat. Commun. 9, 122. doi:10.1038/s41467-017-02635-5
Putnis, A. (2014). Why Mineral Interfaces Matter. Science 343 (6178), 1441–1442. doi:10.1126/science.1250884
Salvati Manni, L., Assenza, S., Duss, M., Vallooran, J. J., Juranyi, F., Jurt, S., et al. (2019). Soft Biomimetic Nanoconfinement Promotes Amorphous Water over Ice. Nat. Nanotechnol. 14 (6), 609–615. doi:10.1038/s41565-019-0415-0
Shen, C., Hu, G., Cheong, L. Z., Huang, S., Zhang, J. G., and Wang, D. (2018). Direct Observation of the Growth of Lithium Dendrites on Graphite Anodes by Operando EC‐AFM. Small Methods, 2, 1700298. doi:10.1002/smtd.201700298
Shen, Y. R., and Ostroverkhov, V. (2006). Sum-Frequency Vibrational Spectroscopy on Water Interfaces: Polar Orientation of Water Molecules at Interfaces. Chem. Rev. 106 (4), 1140–1154. doi:10.1021/cr040377d
Shiotari, A., Sugimoto, Y., and Kamio, H. (2019). Characterization of Two- and One-Dimensional Water Networks on Ni(111) via Atomic Force Microscopy. Phys. Rev. Mater. 3 (9), 093001. doi:10.1103/PhysRevMaterials.3.093001
Shiotari, A., and Sugimoto, Y. (2017). Ultrahigh-resolution Imaging of Water Networks by Atomic Force Microscopy. Nat. Commun. 8, 14313. ARTN 1431310. doi:10.1038/ncomms14313
Somorjai, G. A., and Li, Y. (2010). Introduction to Surface Chemistry and Catalysis. Hoboken, NY: John Wiley & Sons.
Strmcnik, D., Kodama, K., van der Vliet, D., Greeley, J., Stamenkovic, V. R., and Marković, N. M. (2009). The Role of Non-covalent Interactions in Electrocatalytic Fuel-Cell Reactions on Platinum. Nat. Chem 1 (6), 466–472. doi:10.1038/Nchem.330
Tanaka, M., Mochizuki, A., Ishii, N., Motomura, T., and Hatakeyama, T. (2002). Study of Blood Compatibility with Poly(2-Methoxyethyl Acrylate). Relationship between Water Structure and Platelet Compatibility in Poly(2-Methoxyethylacrylate-Co-2-Hydroxyethylmethacrylate). Biomacromolecules 3 (1), 36–41. doi:10.1021/bm010072y
Thürmer, K., Nie, S., Feibelman, P. J., and Bartelt, N. C. (2014). Clusters, Molecular Layers, and 3D Crystals of Water on Ni(111). J. Chem. Phys. 141 (18), 18C520. doi:10.1063/1.4896300
Thurmer, K., and Nie, S. (2013). Formation of Hexagonal and Cubic Ice during Low-Temperature Growth. Proc. Natl. Acad. Sci. 110 (29), 11757–11762. doi:10.1073/pnas.1303001110
Urbakh, M., Klafter, J., Gourdon, D., and Israelachvili, J. (2004). The Nonlinear Nature of Friction. Nature 430 (6999), 525–528. doi:10.1038/nature02750
Verma, A., and Sharma, A. (2010). Enhanced Self-Organized Dewetting of Ultrathin Polymer Films under Water-Organic Solutions: Fabrication of Sub-micrometer Spherical Lens Arrays. Adv. Mater. 22 (46), 5306–5309. doi:10.1002/adma.201002768
Vilhena, J. G., Pimentel, C., Pedraz, P., Luo, F., Serena, P. A., Pina, C. M., et al. (2016). Atomic-Scale Sliding Friction on Graphene in Water. Acs Nano 10 (4), 4288–4293. doi:10.1021/acsnano.5b07825
Wandelt, K., and Thurgate, S. (2002). Solid-Liquid Interfaces: Macroscopic Phenomena—Microscopic Understanding. Heidelberg, Berlin: Springer Science & Business Media.
Wang, F., Borodin, O., Gao, T., Fan, X., Sun, W., Han, F., et al. (2018). Highly Reversible Zinc Metal Anode for Aqueous Batteries. Nat. Mater 17 (6), 543–549. doi:10.1038/s41563-018-0063-z
Wu, J., Yang, S., Cai, W., Bi, Z., Shang, G., and Yao, J. (2017). Multi-characterization of LiCoO2 Cathode Films Using Advanced AFM-Based Techniques with High Resolution. Sci. Rep. 7, 11164. ARTN 1116410. doi:10.1038/s41598-017-11623-0
Yang, S., Yan, B., Li, T., Zhu, J., Lu, L., and Zeng, K. (2015). In Situ studies of Lithium-Ion Diffusion in a Lithium-Rich Thin Film Cathode by Scanning Probe Microscopy Techniques. Phys. Chem. Chem. Phys. 17 (34), 22235–22242. doi:10.1039/c5cp01999k
Yoon, I., Abraham, D. P., Lucht, B. L., Bower, A. F., and Guduru, P. R. (2016). In Situ Measurement of Solid Electrolyte Interphase Evolution on Silicon Anodes Using Atomic Force Microscopy. Adv. Energ. Mater. 6 (12), 1600099. ARTN. doi:10.1002/aenm.201600099
Zhou, X., Lu, Y., Zhang, Q., Miao, L., Zhang, K., Yan, Z., et al. (2020). Exploring the Interfacial Chemistry between Zinc Anodes and Aqueous Electrolytes via an In Situ Visualized Characterization System. ACS Appl. Mater. Inter. 12 (49), 55476–55482. doi:10.1021/acsami.0c17023
Keywords: interfacial water, atomic force microscopy, structure and dynamics, liquid/solid interface, machine learning
Citation: Cao D, Song Y, Tang B and Xu L (2021) Advances in Atomic Force Microscopy: Imaging of Two- and Three-Dimensional Interfacial Water. Front. Chem. 9:745446. doi: 10.3389/fchem.2021.745446
Received: 22 July 2021; Accepted: 10 September 2021;
Published: 22 September 2021.
Edited by:
Kenichi Yoshikawa, Doshisha University, JapanReviewed by:
Akitoshi Shiotari, Fritz-Haber-Institut, GermanyRunze Ma, Pacific Northwest National Laboratory (DOE), United States
Copyright © 2021 Cao, Song, Tang and Xu. This is an open-access article distributed under the terms of the Creative Commons Attribution License (CC BY). The use, distribution or reproduction in other forums is permitted, provided the original author(s) and the copyright owner(s) are credited and that the original publication in this journal is cited, in accordance with accepted academic practice. No use, distribution or reproduction is permitted which does not comply with these terms.
*Correspondence: Duanyun Cao, ZHljYW9AYml0LmVkdS5jbg==; Limei Xu, bGltZWkueHVAcGt1LmVkdS5jbg==
†DC and YS have contributed equally to this work and share first authorship