- 1Centro de Química Médica, Instituto de Ciencias e Innovación en Medicina, Clínica Alemana Universidad del Desarrollo, Santiago, Chile
- 2Facultad de Química y de Farmacia, Pontificia Universidad Católica de Chile, Santiago, Chile
The reactions between 2-chloro-5-nitro pyrimidine with a serie of α-nucleophile derivatives were kinetically evaluated. The kinetic study was carried out in aqueous media and the data shown an unusual split on the Brønsted type-plot, opening a controversial discussion based on reactivities and possible reaction pathways. These split Brønsted type-plots are discussed over the hypothetical transition state (TS) structures associated to concerted or stepwise mechanisms with emphasis on hydrogen bond interactions between electrophile/nucleophile pair able to determine the reactivities and the plausible reaction routes.
Introduction
The alpha effect accounts for the increased nucleophilic strength due to the presence of an adjacent atom to the nucleophilic center with a lone pair of electrons (Jencks and Carriuolo, 1960a, 1960b; Edwards and Pearson, 1962; Dixon and Bruice, 1972; Buncel and Um, 2004; Kirby et al., 2005; Ren and Yamataka, 2007; 2009; Ormazábal-Toledo et al., 2013b; Kool et al., 2014; Kölmel and Kool, 2017) The nucleophilic strength has been frequently related with the basicity of the nucleophile. However, sometimes the nucleophilicity is greater than the basicity (Anderson and Jencks, 1960) The nucleophilicity concept is associated to electron-rich species (nucleophiles), at the same way, the electrophilicity to electron-deficient species (electrophile) (Ingold, 1929, 1933, 1934) Both concepts are based on electron theory of Lewis (Lewis, 1923) and the general acid-base theory of Brönsted and Lowry (Brönsted, 1923; Lowry, 1923) Then, nucleophilicity/electrophilicity have been used as quantitative scales in order to rationalize the chemical reactivity (Contreras et al., 2003)
The term “α-effect” was used by Edwards and Pearson in order to describe an additional factor relative to the polarizability that influences the nucleophilicity (Edwards and Pearson, 1962) Currently, there are different hypotheses about this effect, such as: 1) increased polarization of the nucleophiles; 2) stabilization of the Transition State (TS) structures along the of the Potential Energy Surface (PES) by the lone pair at α position; 3) relative stability of the reaction products and 4) ground state destabilization due to electron-electron repulsion (Anderson and Jencks, 1960; Edwards and Pearson, 1962; Dixon and Bruice, 1972; Bell et al., 1974; Fountain et al., 2003; Um et al., 2006; Gallardo-Fuentes et al., 2014) Hudson et al showed that the magnitude of the α-effect will increase with larger
Therefore, it is possible that the α-effect could be related with several factors, and more studies are needed to provide a detailed description about how this significant effect operates. For better understanding the α-effect, in the present work we studied the magnitude of the α-effect of the reacting pair (electrophile/nucleophile) evaluating the nucleophilic rate coefficients of a nucleophilic aromatic substitution (SNAr) reaction in aqueous media (Cho et al., 2014) The postulated mechanism for a SNAr reaction involves a nucleophilic addition followed by elimination of a leaving group (LG) and it requires the presence of at least one strong electron-withdrawing (Bunnett and Morath, 1955; Liebman et al., 1996) substituent in the ring of the electrophile to stabilize the intermediate, called Meisenheimer Complex (MC) and good LG (Bunnett and Zahler, 1951; Banjoko and Babatunde, 2004; Crampton et al., 2004, 2007; Um et al., 2007; Terrier, 2013; Ormazábal-Toledo et al., 2013b; Gallardo-Fuentes et al., 2014; Gazitúa et al., 2014; Contreras et al., 2015; Calfumán et al., 2017; 2018; Sánchez et al., 2018b) The first step of the reaction mechanism corresponds to the formation of a MC. In a second step, the LG detaches after an intramolecular proton transfer (RLPT) from the nucleophile(Bernasconi and De Rossi, 1976; Ma̧kosza, 1993; Bernasconi et al., 2004; Nudelman, 2009; Um et al., 2012; Ormazabal-Toledo et al., 2013; Ormazábal-Toledo et al., 2013a; Swager and Wang, 2017) Scheme 1 shows the general reaction mechanism for a SNAr reaction. However, more recently, a concerted mechanism has been postulated for this type of reactions. In many cases, the nucleophilic attack on the ipso carbon at the aromatic ring occurs concertedly with the LG departure within a single stepwise pathway without a MC formation (Terrier, 2013; Neumann et al., 2016; Calfumán et al., 2017; Neumann and Ritter, 2017; Stenlid and Brinck, 2017; Kwan et al., 2018; Campodónico et al., 2020) The literature summarizes the mechanistic trends based on the chemical nature of substrates and nucleophiles(Ormazábal-Toledo et al., 2013b; Gazitúa et al., 2014; Alarcón-Espósito et al., 2015, 2016, 2017; 2018; Sánchez et al., 2018a; Campodónico et al., 2020) However, few articles highlight the stabilization of the species along the PES based on hydrogen bond (HB) interactions of the reacting pair (Newington et al., 2007; Ormazábal-Toledo et al., 2013a, 2013b; Gallardo-Fuentes et al., 2014; Calfumán et al., 2017; Sánchez et al., 2018b)

SCHEME 1. General reaction mechanism for a SNAr with a hypothetical protonated nucleophile. LG corresponds to the Leaving Group and EWG corresponds to electron withdrawing groups.
In this work, we studied the reaction of 2-chloro-5-nitro pyrimidine (electrophile) with the family of α-nucleophiles depicted in Table 1 (see bottom in Results and Discussion) in aqueous media. Scheme 2 describes the SNAr reaction between 2-chloro-5-nitro pyrimidine and a hypothetical alpha-nucleophile. The main goal was to determine the α-effect on the studied reaction considering the kinetic results and the analysis of the Brønsted type-plot in addition to chemical structures analysis of the reacting pairs.
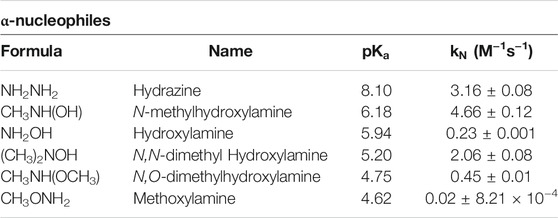
TABLE 1. Summary of nucleophiles and their

SCHEME 2. General reaction mechanism for a SNAr between 2-chloro-5-nitro pyrimidine with a hypothetical protonated nucleophile.
A Brønsted plot corresponds to a free energy relationship that correlates the logarithm of the nucleophilic rate coefficients (
where
Materials and Methods
Reactants
2-Chloro-5-nitro pyrimidine and all the nucleophiles were of the highest quality available commercial products by Sigma Aldrich and Merck. The certificate of analysis guarantees purity ≥99%.
Kinetic Measurements
The kinetics were carried out spectrophotometrically by means of a diode array spectrophotometer in aqueous media, monitoring the appearance of 2,4-dinitrophenoxide anion at 360 nm. The experimental conditions were 25.0 ± 0.1°C, ionic strength 0.2 M (KCl), at three different pH values maintained by partial protonation of the nucleophiles. All the reactions were studied under excess of the nucleophile at least 10 times greater than the substrate concentration (Um et al., 2007, 2012) in order to achieve pseudo-first-order kinetic conditions. The reactions were started by injection of a substrate stock solution 0.1 M in acetonitrile (10 μl) into the amine solution (2.5 ml in the spectrophotometric cell) reaching a concentration of 0.0004 M in the cell. The formation of colored amino-substituted nitropyrimidine compounds were monitored by UV–vis spectroscopy. In all runs, the pseudo-first-order rate constant (
Product Analysis
In the studied reactions, the increase of a band centred in the range of 330—550 nm was observed; attributed to the corresponding reaction products for all nucleophile series studied.
Synthesis of Products
5-Nitro-N-phenylpyrimidin-2-amine
To a solution of 2-chloro-5-nitropyrimidine (40 mg, 0.25 mmol) in CH3CN (1.0 ml), was added aniline (23.3 mg, 0.25 mmol). The reaction mixture was stirred for 4 h at room temperature, the solvent was removed under vacuum to give a yellow solid which was recrystallized from ethanol (35 mg, 65%), mp 201.5–202.5°C (Lit (Von Bebenburg and Thiele, 1970) 202–203°C). 1H-NMR (400 MHz, DMSO-d6) d: 7.13 (t, J = 7.5 Hz, 1H), 7.36 (t, J = 8.0 Hz, 2H), 7.76 (d, J = 8.0 Hz, 2H), 9.22 (s, 2H), 10.84 (s, 1H); 13C-NMR (100 MHz, DMSO-d6) d: 126.0, 129.2, 133.9, 140.3, 143.6, 160.3, 166.0.
2-Hydrazinyl-5-Nitropyrimidine
Using the above procedure, from 2-chloro-5-nitropyrimidine (40 mg, 0.25 mmol) and hydrazine (8.0 mg, 0.25 mmol), was obtained a yellow solid (30 mg, 77%), mp 170–172°C (Lit (Caton and McOmie, 1968) 168–169°C). 1H-NMR (400 MHz, DMSO-d6) d: 9.13 (s, 1 H), 9.20 (s, 1 H), 10.84 (s, 1 H); 13C-NMR (100 MHz, DMSO-d6) d: 136.3, 155.9, 164.3.
Results and Discussion
In the experimental conditions used only one product formation was spectrophotometrically observed for all the reactions studied. Therefore, the possibility of nucleophilic attack at the unsubstituted ring positions is discarded (Um et al., 2007) This fact was confirmed by synthesis and study of the reaction product (see Experimental Section and SM), discarding the possibility of nucleophilic attack at the unsubstituted positions on the aromatic ring (4 and 6, positions).
The values of
The
Linear plots of
The values of
A preliminary inspection of Table 1 reveals that the general trend in reactivity is: N-methyl hydroxylamine > hydrazine > N,N-dimethyl hydroxylamine > N,O-dimethyl hydroxylamine > hydroxylamine > methoxylamine. Note that, this trend is not in agreement with the
Figure 1 shows the statistically corrected Brønsted-type plot for the studied reactions, and the nucleophile serie do not follow the same trend. Unusually, the Brønsted-type plot is split in two trends, but three points in each one is not enough to establish a correlation and to establish the rate-determining step (RDS) of the reaction mechanism. However, in a first approach a split Brønsted-type plot would suggest that: 1) the studied nucleophile serie have TS structurally different and they should be associated to RDS of the reaction mechanism and 2) the reactivity of the nucleophiles is associated to its chemical structure and steric hindrance close to the nucleophilic center.
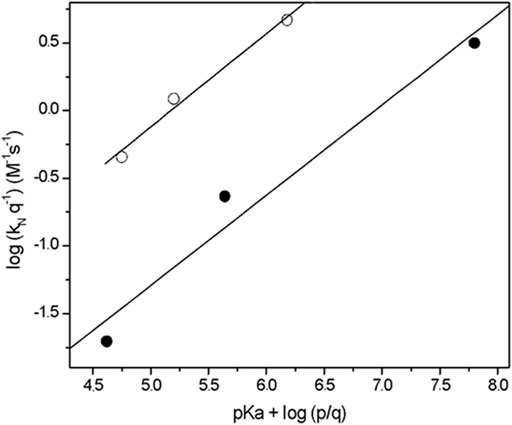
FIGURE 1. Brønsted -type plots (statistically corrected) obtained for the reactions of 2-choro-5-nitro pyrimidine with alpha nucleophile series in aqueous solution, at 25.0°C and ionic strength of 0.2 M in KCl. In increasing order of
Then, from Figure 1 is observed an increased order in reactivity for the nucleophiles in both trends in agreement with theirs
1) The first trend in nucleophilicity is denoted by full circles in Figure 1 that shows the reactivities of hydrazine > hydroxylamine > methoxylamine which agrees with their
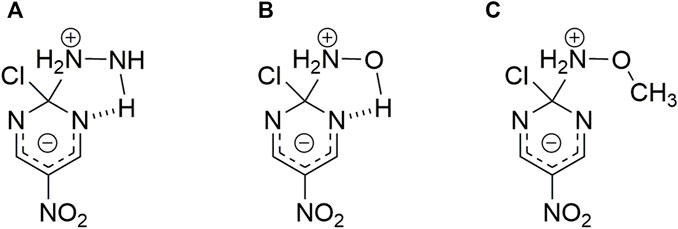
SCHEME 3. Possible HB interaction between the reacting pair. Structures correspond to hydrazine (A), hydroxylamine (B) and methoxylamine (C) nucleophiles toward 2-chloro-5-nitro pyrimidine, respectively.(Gallardo-Fuentes et al., 2014)
2) The second trend (empty circles in Figure 1) shows the following order of reactivity: N-methyl hydroxylamine > N,N-dimethyl hydroxylamine > N,O-dimethyl hydroxylamine. This trend shows the decreasing effect of methyl groups on the nucleophilic reactivity; N-methylhydroxylamine is 2.3 times more reactive than N,N-dimethylhydroxylamine, which in turns is 4.6 times more reactive than N,O-dimethylhydroxylamine.
The comparison between both trends shown an increase in reactivity for the second trend (see Figure 1). For instance, N,N-dimethyl hydroxylamine and hydroxylamine have similar
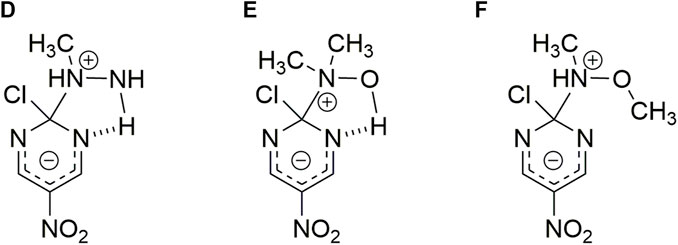
SCHEME 4. Possible HB interaction between the reacting pair. Structures correspond to N-methyl hydroxylamine (D), N,N-dimethyl hydroxylamine (E) and N,O-dimethyl hydroxylamine (F) nucleophiles toward 2-chloro-5-nitro pyrimidine, respectively. (In analogy to Scheme 2) (Gallardo-Fuentes et al., 2014)
In order to reinforce the hypothesis that stereo-electronic effects on TS stabilization, may activate the electrophile and to improve the nucleophilicity of the nucleophile, a kinetic study of a serie of anilines using the same substrate was performed. With this purpose, the stereo-electronic effect of electron-donors (-NH2, -OMe, -Me) and one electron-acceptor (Me-C=O) groups in the nucleophile, was studied. Table 2 summarize the values of
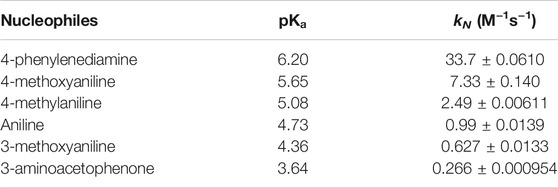
TABLE 2. Aniline serie and their
Figure 2 shows a Brønsted type-plot with a
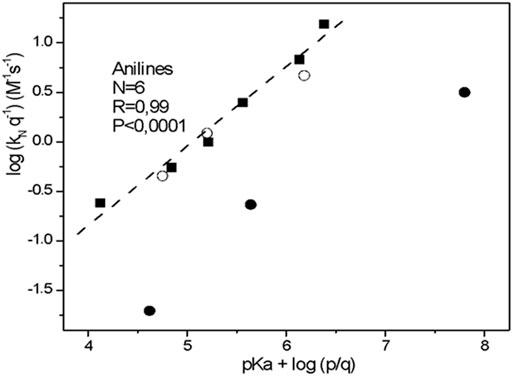
FIGURE 2. Brønsted -type plot (statistically corrected) obtained for the reactions of 2-choro-5-nitro pyrimidine with aniline series in aqueous solution, at 25.0°C and ionic strength of 0.2 M in KCl (full square). The empty circles correspond to: N,O-dimethyl hydroxylamine, N,N-dimethyl hydroxylamine and N-methyl hydroxylamine compounds and full circles correspond to: methoxylamine, hydroxylamine and hydrazine compounds, respectively (see Figure 1).
Focusing our analyses over the chemical structure of the aniline serie; the rate coefficients are notably sensitive to the inductive effects of the substituents. Thus, electron-donating p-substituent has a strong effect on the nucleophilicity and the reactivity order for the nucleophiles agrees with theirs
Accordingly, heterocyclic substrates that contains nitrogen atoms in its chemical structure assist a favorable nucleophilic attack by high nucleophilic amines, but slow LG departures. On this way, the nature of the reacting pair and the reaction media drastically affects the nucleophilic reaction rates and the RDS on the reaction mechanism (Klopman and Frierson, 1984; Garver et al., 2011; Gazitúa et al., 2018; Um et al., 2018)
Finally, in order to determine the HB effect, it was carried out the kinetic study of phenyl hydrazine (see Supplementary Tables S37-S39 and Supplementary Figure S13 in SM) with the same substrate. Note that, this nucleophile with a potential alpha-effect showed a similar behaviour than aniline derivatives (
Concluding Remarks
A complete experimental study on an SNAr reaction has been presented. The experimental results shown an unusual broken on the Brönsted type-plot for the alpha nucleophiles studied, suggesting TS structures structurally different given by the reactivities associated to the chemical structure of them: First, an HB interaction is suggested between the α-hydrogen atom of the nucleophile which is oriented toward the nitrogen atom of the pyrimidine moiety. This HB will promote the reactivity of this serie. Then, a second HB oriented towards the LG, added to the chemical features of the reacting pairs, suggest a concerted route. The second family of alpha-nucleophiles showed a key role of the methyl group inductive effect, stabilizing the ammonium cation in the TS structures, and increasing the reactivity of the nucleophiles. Then, a complete kinetic study based on aniline derivatives toward the same electrophile in order to analyze the Brönsted type-plot, observing a high
Data Availability Statement
The original contributions presented in the study are included in the article/Supplementary Material, further inquiries can be directed to the corresponding author.
Author Contributions
PC design the experiments, performed the kinetic data, analysed results, wrote, discussed and revised the manuscript. RT performed the synthesis and characterization of the reaction products. He discussed and revised the manuscript. CS performed some kinetic data and worked in the manuscript. All the authors have approved the final revised manuscript. PC and behalf of Collaborative Working Group.
Funding
This work was supported by Fondecyt Grant 1150759.
Conflict of Interest
The authors declare that the research was conducted in the absence of any commercial or financial relationships that could be construed as a potential conflict of interest.
Publisher’s Note
All claims expressed in this article are solely those of the authors and do not necessarily represent those of their affiliated organizations, or those of the publisher, the editors, and the reviewers. Any product that may be evaluated in this article, or claim that may be made by its manufacturer, is not guaranteed or endorsed by the publisher.
Supplementary Material
The Supplementary Material for this article can be found online at: https://www.frontiersin.org/articles/10.3389/fchem.2021.740161/full#supplementary-material
References
Alarcón-Espósito, J., Contreras, R., and Campodónico, P. R. (2017). Iso-solvation Effects in Mixtures of Ionic Liquids on the Kinetics of a Model SNAr Reaction. New J. Chem. 41, 13435–13441. doi:10.1039/C7NJ03246C
Alarcón-Espósito, J., Contreras, R., Tapia, R. A., and Campodónico, P. R. (2016). Gutmann's Donor Numbers Correctly Assess the Effect of the Solvent on the Kinetics of SNAr Reactions in Ionic Liquids. Chem. Eur. J. 22, 13347–13351. doi:10.1002/chem.201602237
Alarcón-Espósito, J., Tapia, R. A., Contreras, R., and Campodónico, P. R. (2015). Changes in the SNAr Reaction Mechanism Brought about by Preferential Solvation. RSC Adv. 5, 99322–99328. doi:10.1039/C5RA20779G
Alvaro, C. E. S., Ayala, A. D., and Nudelman, N. S. (2011). Hydrogen-bonded Nucleophile Effects in ANS: the Reactions of 1-chloro and 1-Fluoro-2,4-Dinitrobenzene with 2-guanidinobenzimidazole, 1-(2-aminoethyl)piperidine andN-(3-Aminopropyl)morpholine in Aprotic Solvents. J. Phys. Org. Chem. 24, 101–109. doi:10.1002/poc.1712
Anderson, B. M., and Jencks, W. P. (1960). The Effect of Structure on Reactivity in Semicarbazone Formation1. J. Am. Chem. Soc. 82, 1773–1777. doi:10.1021/ja01492a057
Banjoko, O., and Babatunde, I. A. (2004). Rationalization of the Conflicting Effects of Hydrogen Bond Donor Solvent on Nucleophilic Aromatic Substitution Reactions in Non-polar Aprotic Solvent: Reactions of Phenyl 2,4,6-trinitrophenyl Ether with Primary and Secondary Amines in Benzene-Methanol Mixtures. Tetrahedron 60, 4645–4654. doi:10.1016/j.tet.2004.03.079
Bell, R. P., Critchlow, J. E., and Page, M. I. (1974). ChemInform Abstract: Ground State and Transition State Effects in the Acylation of Alpha-Chymotrypsin in Organic Solvent-Water Mixtures. Chemischer Informationsdienst 5, no. doi:10.1002/chin.197412124
Bernasconi, C. F., Ali, M., Nguyen, K., Ruddat, V., and Rappoport, Z. (2004). Reactions of Substituted (Methylthio)benzylidene Meldrum's Acids with Secondary Alicyclic Amines in Aqueous DMSO. Evidence for Rate-Limiting Proton Transfer. J. Org. Chem. 69, 9248–9254. doi:10.1021/jo040244s
Bernasconi, C. F., and De Rossi, R. H. (1976). Influence of the O-nitro Group on Base Catalysis in Nucleophilic Aromatic Substitution. Reactions in Benzene Solution. J. Org. Chem. 41, 44–49. doi:10.1021/jo00863a010
Brighente, I. M. C., and Yunes, R. A. (1997). The General Mechanisms of Attack of Nitrogen Nucleophiles on Carbonyl Compounds: Facts that Determine the Change of the Rate-pH Profiles. J. Braz. Chem. Soc. 8, 549–553. doi:10.1590/S0103-50531997000500018
Brönsted, J. N. (1923). Einige Bemerkungen über den Begriff der Säuren und Basen. Recl. Trav. Chim. Pays-bas 42, 718–728. doi:10.1002/recl.19230420815
Brönsted, J. N., and Pedersen, K. (1924). Die katalytische Zersetzung des Nitramids und ihre physikalisch-chemische Bedeutung. Z. für Phys. Chem. 108U, 185–235. doi:10.1515/zpch-1924-10814
Buncel, E., Tarkka, R., and Hoz, S. (1993). The Phenomenology of Differently Constructed Brønsted-type Plots. J. Chem. Soc. Chem. Commun., 1993 109–110. doi:10.1039/C39930000109
Buncel, E., and Um, I.-H. (2004). The α-effect and its Modulation by Solvent. Tetrahedron 60, 7801–7825. doi:10.1016/j.tet.2004.05.006
Bunnett, J. F., and Morath, R. J. (1955). The Rates of Condensation of Piperidine with 1-Chloro-2,4-Dinitrobenzene in Various Solvents. J. Am. Chem. Soc. 77, 5165. doi:10.1021/ja01624a063
Bunnett, J. F., and Zahler, R. E. (1951). Aromatic Nucleophilic Substitution Reactions. Chem. Rev. 49, 273–412. doi:10.1021/cr60153a002
Calfumán, K., Gallardo-Fuentes, S., Contreras, R., Tapia, R. A., and Campodónico, P. R. (2017). Mechanism for the SNAr Reaction of Atrazine with Endogenous Thiols: Experimental and Theoretical Study. New J. Chem. 41, 12671–12677. doi:10.1039/C7NJ02708G
Campodónico, P. R., Olivares, B., and Tapia, R. A. (2020). Experimental Analyses Emphasize the Stability of the Meisenheimer Complex in a SNAr Reaction toward Trends in Reaction Pathways. Front. Chem. 8, 583. doi:10.3389/fchem.2020.00583
Castro, E. A., Cañete, A., Campodónico, P. R., Cepeda, M., Pavez, P., Contreras, R., et al. (2013). Kinetic and Theoretical Study on Nucleofugality in the Phenolysis of 3-nitrophenyl and 4-nitrophenyl 4-cyanophenyl Thionocarbonates. Chem. Phys. Lett. 572, 130–135. doi:10.1016/j.cplett.2013.04.002
Castro, E. A. (2007). Kinetics and Mechanisms of Reactions of Thiol, Thiono and Dithio Analogues of Carboxylic Esters with Nucleophiles. An Update. J. Sulfur Chem. 28, 401–429. doi:10.1080/17415990701415718
Castro, E. A., Leandro, L., Millán, P., and Santos, J. G. (1999). Kinetics and Mechanism of the Reactions of Anilines with Ethyl S-Aryl Thiocarbonates. J. Org. Chem. 64, 1953–1957. doi:10.1021/jo982063u
Caton, M. P. L., and McOmie, J. F. W. (1968). Pyrimidines. Part XVII. Nitration of 5-Acetamido-2-Phenylpyrimidine and the Synthesis of Some 5-nitropyrimidines. J. Chem. Soc. C, 836–838. doi:10.1039/J39680000836
Cho, H.-J., Kim, M.-Y., and Um, I.-H. (2014). The α-Effect in SNAr Reaction of Y-Substituted-Phenoxy-2,4-Dinitrobenzenes with Amines: Reaction Mechanism and Origin of the α-Effect. Bull. Korean Chem. Soc. 35, 2448–2452. doi:10.5012/BKCS.2014.35.8.2448
Contreras, R., Andres, J., Safont, V. S., Campodonico, P., and Santos, J. G. (2003). A Theoretical Study on the Relationship between Nucleophilicity and Ionization Potentials in Solution Phase. J. Phys. Chem. A. 107, 5588–5593. doi:10.1021/jp0302865
Contreras, R., Campodónico, P. R., and Ormazábal-Toledo, R. (2015). “Theoretical and Experimental Methods for the Analysis of Reaction Mechanisms in SNAr Processes,” in Arene Chemistry: Reaction Mechanisms and Methods for Aromatic Compounds. Editor J. Mortier (Wiley & Sons), 175–193. doi:10.1002/9781118754887.ch7
Crampton, M. R., Emokpae, T. A., Howard, J. A. K., Isanbor, C., and Mondal, R. (2004). Leaving Group Effects on the Mechanism of Aromatic Nucleophilic Substitution (SNAr) Reactions of Some Phenyl 2,4,6-trinitrophenyl Ethers with Aniline in Acetonitrile. J. Phys. Org. Chem. 17, 65–70. doi:10.1002/poc.690
Crampton, M. R., Emokpae, T. A., and Isanbor, C. (20072007). The Effects of Ring Substituents and Leaving Groups on the Kinetics of SNAr Reactions of 1-Halogeno- and 1-Phenoxy-Nitrobenzenes with Aliphatic Amines in Acetonitrile. Eur. J. Org. Chem. 2007, 1378–1383. doi:10.1002/ejoc.200600968
Dixon, J. E., and Bruice, T. C. (1972). .alpha. Effect. V. Kinetic and Thermodynamic Nature of the .Alpha. Effect for Amine Nucleophiles. J. Am. Chem. Soc. 94, 2052–2056. doi:10.1021/ja00761a043
Edwards, J. O., and Pearson, R. G. (1962). The Factors Determining Nucleophilic Reactivities. J. Am. Chem. Soc. 84, 16–24. doi:10.1021/ja00860a005
Evanseck, J. D., Blake, J. F., and Jorgensen, W. L. (1987). Ab Initio study of the SN2 Reactions of Hydroxide and Hydroperoxide with Chloromethane. J. Am. Chem. Soc. 109, 2349–2353. doi:10.1021/ja00242a018
Filippini, F., and Hudson, R. F. (1972). A General Treatment of Enhanced Nucleophilic Reactivity. J. Chem. Soc. Chem. Commun., 522–523. doi:10.1039/C39720000522
Fountain, K. R., Felkerson, C. J., Driskell, J. D., and Lamp, B. D. (2003). The α-Effect in Methyl Transfers from S-Methyldibenzothiophenium Fluoroborate to Substituted N-Methylbenzohydroxamates. J. Org. Chem. 68, 1810–1814. doi:10.1021/jo0206263
Gallardo-Fuentes, S., Tapia, R. A., Contreras, R., and Campodónico, P. R. (2014). Site Activation Effects Promoted by Intramolecular Hydrogen Bond Interactions in SNAr Reactions. RSC Adv. 4, 30638–30643. doi:10.1039/C4RA04725G
Garver, J. M., Gronert, S., and Bierbaum, V. M. (2011). Experimental Validation of the α-Effect in the Gas Phase. J. Am. Chem. Soc. 133, 13894–13897. doi:10.1021/ja205741m
Gazitúa, M., Tapia, R. A., Contreras, R., and Campodónico, P. R. (2018). Effect of the Nature of the Nucleophile and Solvent on an SNAr Reaction. New J. Chem. 42, 260–264. doi:10.1039/C7NJ03212A
Gazitúa, M., Tapia, R. A., Contreras, R., and Campodónico, P. R. (2014). Mechanistic Pathways of Aromatic Nucleophilic Substitution in Conventional Solvents and Ionic Liquids. New J. Chem. 38, 2611–2618. doi:10.1039/C4NJ00130C
Gordillo, R., Dudding, T., Anderson, C. D., and Houk, K. N. (2007). Hydrogen Bonding Catalysis Operates by Charge Stabilization in Highly Polar Diels−Alder Reactions. Org. Lett. 9, 501–503. doi:10.1021/ol0629925
Ingold, C. K. (1933). 266. Significance of Tautomerism and of the Reactions of Aromatic Compounds in the Electronic Theory of Organic Reactions. J. Chem. Soc., 1120–1127. doi:10.1039/JR9330001120
Ingold, C. K. (1934). Principles of an Electronic Theory of Organic Reactions. Chem. Rev. 15, 225–274. doi:10.1021/cr60051a003
Ingold, C. K. (1929). The Principles of Aromatic Substitution, from the Standpoint of the Electronic Theory of Valency. Recl. Trav. Chim. Pays-bas 48, 797–812. doi:10.1002/recl.19290480808
Jencks, W. P., and Carriuolo, J. (1960a). General Base Catalysis of the Aminolysis of Phenyl Acetate1. J. Am. Chem. Soc. 82, 675–681. doi:10.1021/ja01488a044
Jencks, W. P., and Carriuolo, J. (1960b). Reactivity of Nucleophilic Reagents toward Esters. J. Am. Chem. Soc. 82, 1778–1786. doi:10.1021/ja01492a058
Kamlet, M. J., Abboud, J. L. M., Abraham, M. H., and Taft, R. W. (1983). Linear Solvation Energy Relationships. 23. A Comprehensive Collection of the Solvatochromic Parameters, .pi.*, .alpha., and .beta., and Some Methods for Simplifying the Generalized Solvatochromic Equation. J. Org. Chem. 48, 2877–2887. doi:10.1021/jo00165a018
Kirby, A. J., Dutta-Roy, N., da Silva, D., Goodman, J. M., Lima, M. F., Roussev, C. D., et al. (2005). Intramolecular General Acid Catalysis of Phosphate Transfer. Nucleophilic Attack by Oxyanions on the PO32- Group. J. Am. Chem. Soc. 127, 7033–7040. doi:10.1021/ja0502876
Kirby, A. J., Manfredi, A. M., Souza, B. S. d., Medeiros, M., Priebe, J. P., Brandão, T. A. S., et al. (2008). Reactions of Alpha-Nucleophiles with a Model Phosphate Diester. Arkivoc 2009, 28–38. doi:10.3998/ark.5550190.0010.305
Kirby, A. J., Tondo, D. W., Medeiros, M., Souza, B. S., Priebe, J. P., Lima, M. F., et al. (2009). Efficient Intramolecular General-Acid Catalysis of the Reactions of α-Effect Nucleophiles and Ammonia Oxide with a Phosphate Triester. J. Am. Chem. Soc. 131, 2023–2028. doi:10.1021/ja808746f
Klopman, G., and Frierson, M. R. (1984). The Alpha-Effect. A Theoretical Study Incorporating Solvent Effects. Croat. Chem. Acta 57, 1411–1415.
Kölmel, D. K., and Kool, E. T. (2017). Oximes and Hydrazones in Bioconjugation: Mechanism and Catalysis. Chem. Rev. 117, 10358–10376. doi:10.1021/acs.chemrev.7b00090
Kool, E. T., Crisalli, P., and Chan, K. M. (2014). Fast Alpha Nucleophiles: Structures that Undergo Rapid Hydrazone/Oxime Formation at Neutral pH. Org. Lett. 16, 1454–1457. doi:10.1021/ol500262y
Kwan, E. E., Zeng, Y., Besser, H. A., and Jacobsen, E. N. (2018). Concerted Nucleophilic Aromatic Substitutions. Nat. Chem 10, 917–923. doi:10.1038/s41557-018-0079-7
Lewis, G. N. (1923). Valence and the Structure of Atoms and Molecules. Am. Chem. Soc., Monograph Series. California: The Chemical Catalog Co., Inc.
Liebman, J. F., Campbell, M. S., and Slayden, S. W. (1996). Thermochemistry of Amines, Nitroso Compounds, Nitro Compounds and Related Species. Chem. Amin. Nitroso, Nitro Relat. Groups, 337–378. doi:10.1002/047085720X.ch8
Lowry, T. M. (1923). The Uniqueness of Hydrogen. J. Chem. Technol. Biotechnol. 42, 43–47. doi:10.1002/jctb.5000420302
Makosza, M. (1993). Book Review: Nucleophilic Aromatic Displacement. The Influence of the Nitro Group.(Series: Organic Nitro Chemistry Series). By F. Terrier, Angew. Chem. Int. Ed. Engl. 32, 302–303. doi:10.1002/anie.199303022
Neumann, C. N., Hooker, J. M., and Ritter, T. (2016). Concerted Nucleophilic Aromatic Substitution with 19F− and 18F−. Nature 534, 369–373. doi:10.1038/nature17667
Neumann, C. N., and Ritter, T. (2017). Facile C-F Bond Formation through a Concerted Nucleophilic Aromatic Substitution Mediated by the PhenoFluor Reagent. Acc. Chem. Res. 50, 2822–2833. doi:10.1021/acs.accounts.7b00413
Newington, I., Perez-Arlandis, J. M., and Welton, T. (2007). Ionic Liquids as Designer Solvents for Nucleophilic Aromatic Substitutions. Org. Lett. 9, 5247–5250. doi:10.1021/ol702435f
Nigst, T. A., Antipova, A., and Mayr, H. (2012). Nucleophilic Reactivities of Hydrazines and Amines: The Futile Search for the α-Effect in Hydrazine Reactivities. J. Org. Chem. 77, 8142–8155. doi:10.1021/jo301497g
Nudelman, N. S. (2009). “SNAr Reactions of Amines in Aprotic Solvents,” in Patai’s Chemistry of Functional Groups, 2–5. doi:10.1002/9780470682531.pat0096
Ormazábal-Toledo, R., Contreras, R., and Campodónico, P. R. (2013a). Reactivity Indices Profile: A Companion Tool of the Potential Energy Surface for the Analysis of Reaction Mechanisms. Nucleophilic Aromatic Substitution Reactions as Test Case. J. Org. Chem. 78, 1091–1097. doi:10.1021/jo3025048
Ormazábal-Toledo, R., Contreras, R., Tapia, R. A., and Campodónico, P. R. (2013b). Specific Nucleophile-Electrophile Interactions in Nucleophilic Aromatic Substitutions. Org. Biomol. Chem. 11, 2302–2309. doi:10.1039/C3OB27450K
Ormazabal-Toledo, R., Santos, J. G., Ríos, P., Castro, E. A., Campodónico, P. R., and Contreras, R. (2013). Hydrogen Bond Contribution to Preferential Solvation in SNAr Reactions. J. Phys. Chem. B 117, 5908–5915. doi:10.1021/jp4005295
Ren, Y., and Yamataka, H. (2006). The α-Effect in Gas-phase SN2 Reactions Revisited. Org. Lett. 8, 119–121. doi:10.1021/ol0526930
Ren, Y., and Yamataka, H. (2007). The α-Effect in Gas-phase SN2 Reactions: Existence and the Origin of the Effect. J. Org. Chem. 72, 5660–5667. doi:10.1021/jo070650m
Rohrbach, S., Murphy, J. A., and Tuttle, T. (2020). Computational Study on the Boundary between the Concerted and Stepwise Mechanism of Bimolecular SNAr Reactions. J. Am. Chem. Soc. 142, 14871–14876. doi:10.1021/jacs.0c01975
Sánchez, B., Calderón, C., Garrido, C., Contreras, R., and Campodónico, P. R. (2018a). Solvent Effect on a Model SNAr Reaction in Ionic Liquid/water Mixtures at Different Compositions. New J. Chem. 42, 9645–9650. doi:10.1039/C7NJ04820C
Sánchez, B., Calderón, C., Tapia, R. A., Contreras, R., and Campodónico, P. R. (2018b). Activation of Electrophile/Nucleophile Pair by a Nucleophilic and Electrophilic Solvation in a SNAr Reaction. Front. Chem. 6, 509. doi:10.3389/fchem.2018.00509
Stenlid, J. H., and Brinck, T. (2017). Nucleophilic Aromatic Substitution Reactions Described by the Local Electron Attachment Energy. J. Org. Chem. 82, 3072–3083. doi:10.1021/acs.joc.7b00059
Swager, T. M., and Wang, P. (2017). A Negotiation between Different Nucleophiles in SNAr Reactions. Synfacts 13, 0148. doi:10.1055/s-0036-1589929
Terrier, F. (2013). The SNAr Reactions: Mechanistic Aspects. Mod. Nucleophilic Aromat. Substit., 231–361. doi:10.1002/9783527656141.ch1
Um, I.-H., Chung, E.-K., and Lee, S.-M. (1998). An Unusual Ground-State Stabilization Effect and Origins of the Alpha-Effect in Aminolyses of Y-Substituted Phenyl X-Substituted Benzoates. Can. J. Chem. 76, 729–737. doi:10.1139/v98-043
Um, I.-H., Hwang, S.-J., and Buncel, E. (2006). Solvent Effect on the α-Effect: Ground-State versus Transition-State Effects; a Combined Calorimetric and Kinetic Investigation. J. Org. Chem. 71, 915–920. doi:10.1021/jo051823f
Um, I.-H., Im, L.-R., Kang, J.-S., Bursey, S. S., and Dust, J. M. (2012). Mechanistic Assessment of SNAr Displacement of Halides from 1-Halo-2,4-Dinitrobenzenes by Selected Primary and Secondary Amines: Brønsted and Mayr Analyses. J. Org. Chem. 77, 9738–9746. doi:10.1021/jo301862b
Um, I.-H., Min, S.-W., and Dust, J. M. (2007). Choice of Solvent (MeCN vs H2O) Decides Rate-Limiting Step in SNAr Aminolysis of 1-Fluoro-2,4-Dinitrobenzene with Secondary Amines: Importance of Brønsted-type Analysis in Acetonitrile. J. Org. Chem. 72, 8797–8803. doi:10.1021/jo701549h
Um, I.-H., Moon, H.-J., Shin, Y.-H., and Dust, J. M. (2018). Medium Effect on the α-effect for Nucleophilic Substitution Reactions of P-Nitrophenyl Acetate with Benzohydroxamates and M-Chlorophenoxide in DMSO-H2o Mixtures as Contrasts with MeCN-H2o Mixtures: Comparing Two Very Different Polar Aprotic Solvent Components. Can. J. Chem. 96, 922–928. doi:10.1139/cjc-2018-0103
Von Bebenburg, W., and Thiele, K. (1970). Antipyretic 2-(phenylamino)- and 2-(pyridylamino)pyrimidines with an Amino or Amido Group in the 5-position. US3499898A. United States: United States Patent Office.
Keywords: SNAr reactions, mechanisms, hydrogen bond interaction, reactivity, brønsted type-plots
Citation: Campodónico PR, Tapia RA and Suárez-Rozas C (2022) How the Nature of an Alpha-Nucleophile Determines a Brønsted Type-Plot and Its Reaction Pathways. An Experimental Study. Front. Chem. 9:740161. doi: 10.3389/fchem.2021.740161
Received: 12 July 2021; Accepted: 30 December 2021;
Published: 02 February 2022.
Edited by:
Doo Soo Chung, Seoul National University, South KoreaReviewed by:
Jose Ramón Mora, Universidad San Francisco de Quito, EcuadorJing Ma, Henan University, China
Copyright © 2022 Campodónico, Tapia and Suárez-Rozas. This is an open-access article distributed under the terms of the Creative Commons Attribution License (CC BY). The use, distribution or reproduction in other forums is permitted, provided the original author(s) and the copyright owner(s) are credited and that the original publication in this journal is cited, in accordance with accepted academic practice. No use, distribution or reproduction is permitted which does not comply with these terms.
*Correspondence: Paola R. Campodónico, cGNhbXBvZG9uaWNvQHVkZC5jbA==