- 1School of Physical Science and Technology, ShanghaiTech University, Shanghai, China
- 2State Key Laboratory of Coordination Chemistry, School of Chemistry and Chemical Engineering, Nanjing National Laboratory of Microstructures, Nanjing University, Nanjing, China
- 3College of Chemistry and Materials Engineering, Wenzhou University, Wenzhou, China
- 4Institut de Chimie de Strasbourg, CNRS-UMR 7177 Université de Strasbourg, Strasbourg, France
Two polymorphic FeII coordination polymers [FeIIL (TPPE)0.5] 1) and [(FeII3L3 (TPPE)1.5)] 2), were obtained from a redox-active tetrathiafulvalene (TTF) functionalized ligand [H2L = 2,2’-(((2-(4,5-bis-(methylthio)-1,3-dithiol-2-ylidene)benzo(d) (1,3) dithiole-5,6-diyl)bis-(azanediyl))bis-(meth anylylidene)) (2E,2E')-bis(3-oxobutanoate)] and a highly luminescent connector {TPPE = 1,1,2,2-tetrakis[4-(pyridine-4-yl)phenyl]-ethene}. Complex 1 has a layered structure where the TPPE uses its four diverging pyridines from the TPPE ligand are coordinated by the trans positions to the flat TTF Schiff-base ligand, and complex 2 has an unprecedented catenation of layers within two interpenetrated frameworks. These coordination polymers reserved the redox activity of the TTF unit. Complex 1 shows gradual spin transition behavior without hysteresis. And the fluorescence intensity of TPPE in 1 changes in tandem with the spin crossover (SCO) transition indicating a possible interplay between fluorescence and SCO behavior.
Introduction
Among numerous multifunctional materials, spin crossover (SCO) complexes exhibiting switching between low-spin (LS) and high-spin (HS) states, are one of the most fashionable examples of molecular bistability (Smith et al., 2003; Weber, 2009; Tao et al., 2012; Harding et al., 2016; Ni et al., 2017). The SCO behavior can be effected by different external incentives such as temperature, pressure, or light radiation (Hoshino et al., 2012; Zheng et al., 2018). The change in the spin-state brings about the attractive shift in structural, optical, and electrical properties making SCO systems absorbing for applications in physics, chemistry and materials science (Gütlich et al., 2000; Halcrow, 2011; Kepp, 2016). Recently, SCO systems displaying multifunctionality [such as electrical conductivity (Wang et al., 2018a; De la Barrera et al., 2018) or optical behaviors (Delgado et al., 2018; Lochenie et al., 2018)] have been focused, while the challenges still persist in developing fluorescent SCO complexes.
In fact, it is a wise choice to employ coordination connectors with distinct configuration and performance in order to incorporate spin transition and fluorescence. The wonderful 1,1,2,2-tetrakis[4-(pyridine-4-yl)phenyl]-ethene (TPPE) ligand, which includes four pyridine rings around a central ethylene with a diverting “propeller” configuration, acts as a bridging ligand to form polymeric networks (Kapadia et al., 2011a; Huang et al., 2012; Icli et al., 2012; Pigge et al., 2013). Additionally, TPPE and its derivatives have extended π-conjugation which may give rise to aggregation-induced emission (AIE); consequently, an increasing number of corresponding reports has emerged (Kapadia et al., 2011b; Gong et al., 2014). Recently, our laboratory constructed a two-dimensional (2D) FeII coordination polymer {[Fe(L)](TPPE)0.5·3CH3OH}n (L is a N2O22− coordinating Schiff-base) showing the hysteretic SCO behavior of 25 K width, of which the correlation of SCO behavior and fluorescent properties were achieved (Ge et al., 2019).
Besides to the luminescent connector, it is anoter excellent strategy to introduce functionalized Schiff-base ligand into the SCO and emission properties. Tetrathiafulvalene (TTF) is a sulphur rich, planar organic model with fourteen highly delocalized π electrons, which has a canonical redox-active core. TTF and its derivatives are readily functionalized to coordinate to a diverse range of magnetic centres and have been widely explored as a means of incorporating redox activity into a material (Su et al., 2017; Wang et al., 2017a; Schönfeld et al., 2020; Zappe et al., 2020). Up to now, a number of redox-active materials based on TTF have been studied (Su et al., 2017; Wang et al., 2017a; Wang et al., 2017b; Qiu et al., 2020; Schönfeld et al., 2021). Inspired by these results, we sought to introduce the luminescent ligand, TPPE, into FeII coordination polymers based on a TTF Schiff-base ligand (H2L = 2,2'-(((2-(4,5-bis (methylthio)-1,3-dithiol-2-ylidene)-benzo[d][1,3]di-thiole-5,6-diyl)-bis(azanediyl))-bis(methanylylidene)) (2E,2E')-bis (3-oxobutanoate)) (Scheme 1). The syntheses, crystal structures, electrochemistry, UV-vis-NIR spectroelectrochemistry, fluorescence and magnetic properties are described. This work demonstrates the possible interplay between SCO behaviour and fluorescence.
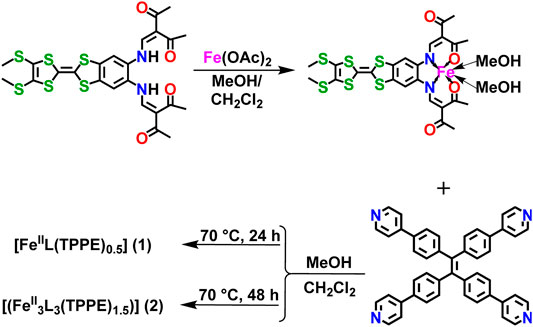
SCHEME 1. The synthetic method for TTF Schiff-base ligand (H2L), precursor [FeⅡL (CH3OH)2], [FeIIL (TPPE)0.5] (1) and [(FeII3L3 (TPPE)1.5)] (2).
Experimental Sections
Synthesis
Synthesis of [FeIIL(TPPE)0.5] (1) We added 5 ml CH2Cl2 + 5 ml MeOH into the [FeIIL(MeOH)2] (20 mg, 0.03 mmol) and TPPE (10 mg, 0.015 mmol) mixture in the gloves box. After heating for 24 h at 70°C in an oven, the mixture was left undisturbed at room temperature. After one week, black rod-like crystals of 1 were gained. Yield: 12.7 mg (42%, grounded on TPPE). Anal. Calcd for C47H38FeN4O4S6: C 58.13, H 3.94, N 5.77%; found: C 58.00, H 3.80, N 5.61%.
Synthesis of [FeII3L3(TPPE)1.5] (2) Compound 2 was synthesized by the same reactants as 1, while after heating for 48 h at 70°C in an oven. It was left in an undisturbed place at room temperature. After one week, black block crystals of 2 were isolated. Yield: 18.8 mg (22%, grounded on TPPE). Anal. Calcd for C141H113Fe3N12O12S18: C 58.15, H 3.91, N 5.77%; found: C 57.98, H 3.75, N 5.59%.
Result and Disscussion
Structural Description
The crystals of 1 appropriate for X-ray structure characterization were obtained by hydrothermal method from precursor [FeIIL (MeOH)2] and bridging ligand TPPE at 70°C for 24 h. However, we could obtain 2 through lengthen the reaction time to 48 h.
The dark red needles of 1 [FeIIL (TPPE)0.5], crystallized in the monoclinic space group C 2/c. The unit cell involves one crystallographically independent [FeIIL] subunit, half a TPPE ligand (Supplementary Figure 1A). The FeII coordination is a slightly distorted [FeN4O2] octahedral coordination configuration in which two nitrogen atoms (N1 and N2) and two oxygen atoms (O1 and O2) from the TTF Schiff-base ligand constitute the basal plane, and two nitrogen atoms (N3 and N4) from tetradentate bridging TPPE (Supplementary Figure 1B), resulted in the expected square-grid [FeIIL (TPPE)0.5]. All of these donors (four nitrogen and two oxygen atoms) lie in cis-locations. The sum of angles among the basal atoms is close to 360°, indicating that Fe1, N1, N2, O1 and O2 share the same plane. The designed square-grid is the key feature of 1, and the four diverging pyridines from the TPPE ligand are coordinated by the trans locations to the flat TTF Schiff-base ligand (Figure 1A). The formation of flat layer is caused by the rigid complanation of the TPPE ligand; and this phenomenon can avoid the penetration of layers, though there is vacant region between the squares. TTF Schiff-base ligand protrudes out of the layer such that adjacent layers are displaced, which resulted in a reduplicative part constituting of two layers per monoclinic C 2/c unit cell (Figure 1B). Typically, for SCO materials, crystallography methods could identify LS and HS states because of differences in bond lengths of the FeII centres between these two states (Δ = 0.14–0.24 Å) (Kahn, 1993). In the present case, the average coordination bond lengths are Fe-Neq (1.882 Å), Fe-Oeq (1.930 Å) and Fe-Nax (2.004 Å). Additionally, the angle for Fe1 [Oeq-Fe1-Oeq] is 89.1 (2)°. All these values for Fe1 are in agreement with those reported for FeII analogues with LS configurations (Kahn, 1993; Rodríguez-Jiménez et al., 2016; Wang et al., 2018b; Rosario-Amorin et al., 2018; Yuan et al., 2018; Schönfeld et al., 2020; Zappe et al., 2020)
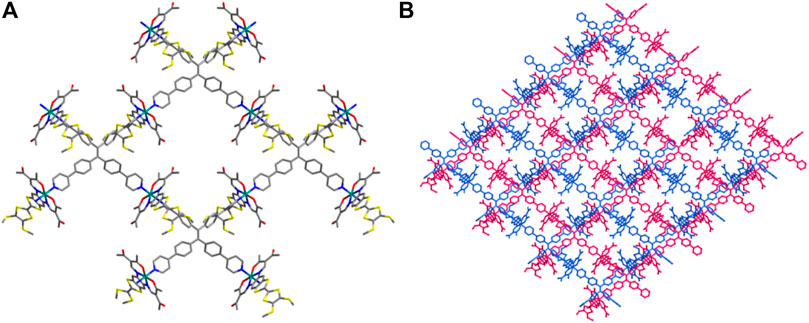
FIGURE 1. 2D network (A) and adjacent interdigitated layers (two layers are shown with two different colours) along the a axis (B) for 1. All of the hydrogen atoms are omitted for clarity, carbon - grey, nitrogen -blue, oxygen - red, sulfur - yellow, iron - teal.
With longer reaction time, a more compacted compound [(FeII3L3 (TPPE)1.5)] (2), was obtained. It crystallizes in the monoclinic space group C 2/c. Three independent [FeIIL] and one and a half TPPE make up the unsymmetric part (Supplementary Figure 2A; Supplementary Table S1); each central FeII is six-coordinated in distorted octahedra where the two nitrogen atoms (N3 and N4A) from the bridging TPPE occupy the axial positions [angle [N3-Fe1-N4A 176.9 (3)°] and the other four atoms (N1, N2, O1 and O2) from the TTF Schiff-base ligand occupy the equatorial positions (sum of the trigonal angles is 358.3°) (Supplementary Figure 2B). The average angle of Oeq-Fe-Oeq is 88.7° [Oeq-Fe1-Oeq 88.5 (3)°, Oeq-Fe2-Oeq 88.6 (2)° and Oeq-Fe3-Oeq 88.9 (2)°] is similar to those found for LS FeII complexes (Kahn, 1993; Rodríguez-Jiménez et al., 2016; Wang et al., 2018b; Rosario-Amorin et al., 2018; Yuan et al., 2018; Schönfeld et al., 2020; Zappe et al., 2020). Additionally, the average bond lengths of Fe-Neq is 1.891 Å [Fe1-Neq 1.870 Å, Fe2-Neq 1.890 Å and Fe3-Neq 1.913 Å], Fe-Oeq is 1.930 Å [Fe1-Oeq 1.935 Å, Fe2-Oeq 1.932 Å and Fe3-Oeq 1.923 Å], and Fe-Nax is 2.006 Å [Fe1-Nax 1.993 Å, Fe2-Nax 2.005 Å and Fe3-Nax 2.021 Å], which is typical for LS FeII complexes (Kahn, 1993; Rodríguez-Jiménez et al., 2016; Wang et al., 2018b; Rosario-Amorin et al., 2018; Yuan et al., 2018; Schönfeld et al., 2020; Zappe et al., 2020). The TPPE and Fe1 ions form 2D layers stack in an ABAB fashion along the c axis (Figures 2A,C). The three-dimensional (3D) frameworks, containing TPPE, Fe2 and Fe3 ions, is 2-fold interpenetrated (Figures 2D–F). By virtue of the axial coordination and size of the TPPE, an unprecedented catenation of layers through two interpenetrated frameworks is formed (Figure 2G).
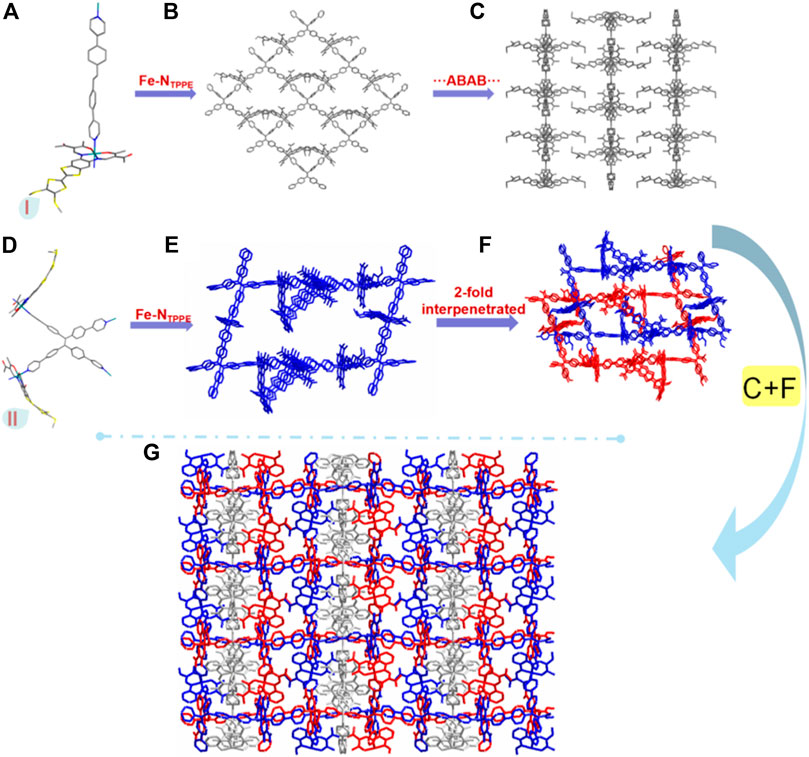
FIGURE 2. Monomer I (A), a 2D network of monomer I (B), the pacing structure of a 2D network of monomer I (C), monomer II (D), 3D frame structure of monomer II (E), the 2-fold interpenetrated of 3D frame structure of monomer II (F) and the 3D interpenetrated framework including 2D network and 3D crystal structure along the a axis (G) for 2. All of the hydrogen atoms are omitted for clarity, carbon - grey, nitrogen -blue, oxygen - red, sulfur - yellow, iron - teal.
Electrochemical Properties
The cyclic voltammogram of H2L (Supplementary Figure 4) displayed two highly invertible oxidation waves at E1/2 = 0.11 and 0.47 V vs Fc/Fc+ (compared to TTF0/TTF·+ = −0.06 and TTF·+/TTF2+ = 0.38 V of TTF itself) (Canevet et al., 2009; Narayan et al., 2012; Su et al., 2017), indicating the construction of the TTF·+ and TTF2+, respectively. Very little change is seen in the CV collected over multiple sweeps (Figure 3A) which demonstrates the stability of the Schiff-base-like ligand to redox change. At faster scan rates (Supplementary Figure 5), a third quasi-reversible oxidation wave was found at 0.99 V, which may tentatively be ascribed to oxidation of the macrocycle i.e. proton assisted oxidation of the ketone groups.
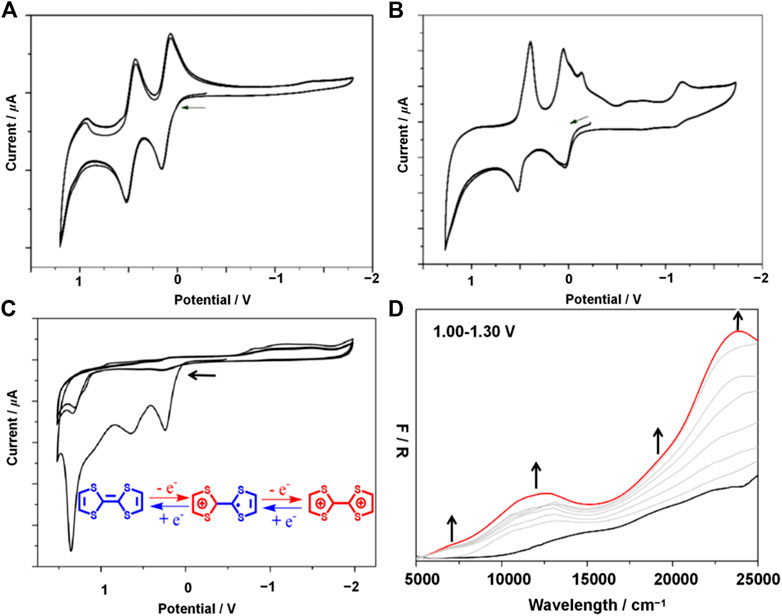
FIGURE 3. Solution state CVs of H2L measured over 3 consecutive sweeps (A), solution state CVs of [FeⅡL(CH3OH)2] measured over 3 consecutive sweeps (B), solid state CV for 1 obtained at 100 mVs−1 over 3 consecutive scans (C), solid-state UV-vis-NIR spectroelectrochemistry of 1 at 0 V (black) and 1.30 V (red) (D). Gray spectra correspond to the spectral transition over the applied potential range of 1.00–1.30 V. Experiment performed in 0.1 mol L−1 TBAPF6 in CH2Cl2. Arrows denote the direction of forward scan.
Upon complexation of H2L with Fe2+ ([FeⅡL(CH3OH)2]) additional redox features were observed (Supplementary Figure 4). Two overlapping invertible oxidation waves were observed at −0.05 and 0.08 V which may be due to the Fe2+/3+ and TTF0/TTF·+ redox couples, respectively. A third, reversible oxidation peak found at 0.46 V corresponds to the oxidation of the TTF·+ cation to TTF2+. The redox potentials of the latter two oxidation waves coincide well with the free base which suggests that coordination of the macrocycle to Fe2+ does not significantly affect the electronic distribution at the TTF site; thus minimal electron delocalization is expected to occur between the TTF and amine functionalities across the aryl spacer. Over multiple potential sweeps, the CVs obtained overlapped well, which confirms the stability of [FeⅡL(CH3OH)2] with redox manipulation (Figure 3B).
The electrochemical data for 1 was quite different to that of the free base H2L and discrete complex [FeⅡL(CH3OH)2] likely to be a result of the crystalline packed nature of the coordination polymer. The CV (Supplementary Figure 7) reveals four irreversible oxidation processes at Eonset = 0.17, 0.65, 1.01 and 1.29 V which may be assigned to the construction of a TTF0/TTF·+, (TTF·+)2, TTF·+/TTF2+ and (TTF2+)2, respectively. Thus, stacking interactions of TTF moieties between interpenetrated nets may stabilise the formation of mixed-valence species. The irreversible nature of these redox processes, however, suggests either that the framework material is unstable to these manipulations or rather that an irreversible structural change occurs in response to the change in oxidation state. This was confirmed with cycling experiments where, upon the second sweep, the current associated with each aforementioned process significantly decreases (Figure 3C). Multiple overlapping reduction processes were observed between −0.86 and −1.50 V which were also apparent in the CV of [FeⅡL(CH3OH)2]. These features may thus be assigned to the discrete complex unit, however, the origin of these processes remains elusive.
We have took the high complexity of CV and square wave voltammetry into consideration and performed the UV-vis-NIR spectroelectrochemistry (SEC) electrochemical processes in order to examine the electrochemical processes electrochemical processes (Figure 3D; Supplementary Figures 10-11). The SEC data revealed a marked spectral change at 1.00 V; new low energy features were observed at ca. 7,000 and 12,000 cm−1 as well as an intensification of the bands at 19,000 and 24,000 cm−1 which are owing to the construction of the TTF•+ (Canevet et al., 2009). The observation of these bands in the as-synthesized material suggests the presence of TTF•+ may be as result of the relatively low oxidation potential associated with the TTF0/•+ redox couple (0.17 vs Fc/Fc+ determined by CV).
Magnetic Properties
We investigated the magnetic properties for 1 and 2 from 2 to 350 K in 1,000 Oe (Figure 4; Supplementary Figure 12). The χMT product at 350 K of 1 was 2.28 cm3 K mol−1 (Figure 4), which indicates that there were about 70% transformation from [LS-LS] to [HS-HS] (Kahn, 1993; Rodríguez-Jiménez et al., 2016; Wang et al., 2018b; Rosario-Amorin et al., 2018; Yuan et al., 2018; Schönfeld et al., 2020; Zappe et al., 2020). As the temperature reduced, the χMT value gradually decreased and achieved a plateau product of 1.13 cm3 K mol−1 at 90 K, indicating the spin transition from HS state of FeII center to its LS state (Kahn, 1993; Rodríguez-Jiménez et al., 2016; Rosario-Amorin et al., 2018; Wang et al., 2018b; Yuan et al., 2018; Schönfeld et al., 2020; Zappe et al., 2020). Below 28 K, the χMT product sharply decreases, attaining 0.71 cm3 K mol−1 at 2 K, which can be owing to the presence of zero-field splitting of the residual HS FeII ions or antiferromagnetic interaction among FeII centres (Suleimanov et al., 2015). However, at 350 K, the χMT product for 2 is 2.16 cm3 K mol−1, which is much lower than the expected product for three isolated HS FeII centres (Supplementary Figure 12), indicating that the LS FeII centres are dominant in 2 (Garcia et al., 2011; Wang et al., 2015; Schönfeld et al., 2020; Zappe et al., 2020).
Fluorescence Properties
The fluorescence emission of 1 at room temperature is compared to that of the pure TPPE (Supplementary Figure 13). TPPE itself displays a strong emission at 472 nm when excited at 360 nm (Supplementary Figure 13). Under the identical excitation wavelength, the emission bands for 1 and 2 occur at 442 and 464 nm, and the fluorescence intensities for 1 and 2 were reduced by a factor of three, respectively (Supplementary Figure 13). The prominent hypochromic effect occured in fluorescence spectra, which may be impacted by the π-π* conversion in the centre of ligand. And these results may be attributed to the coordination between Schiff-base ligand and the metal centre as well as the introduction of TPPE to the FeII complex (Ge et al., 2019).
In search for the connection between SCO and fluorescence properties in 1, a study of its fluorescence emission was investigated while varying the temperature from 90 to 300 K (Figure 5A; Supplementary Figure 14). Upon warming, the fluorescence intensity of 1 increased gradually, reaching a maximum at 140 K and the fluorescence intensity decreased until 160 K. However, when the temperature increased to 160 K, the fluorescence intensity began to increase suddenly until 180 K, followed by a decrease in intensity upon further warming. These drastic temperature-dependent variations in fluorescence intensity occur in the range 90–300 K which agrees moderately well with the thermally induced SCO behavior of 1. We speculate that the changes in coordination geometry and bond lengths between the ligand and FeII ions associated with the spin transition could affect the fluorescence properties mentioned before (Garcia et al., 2011; Wang et al., 2015).
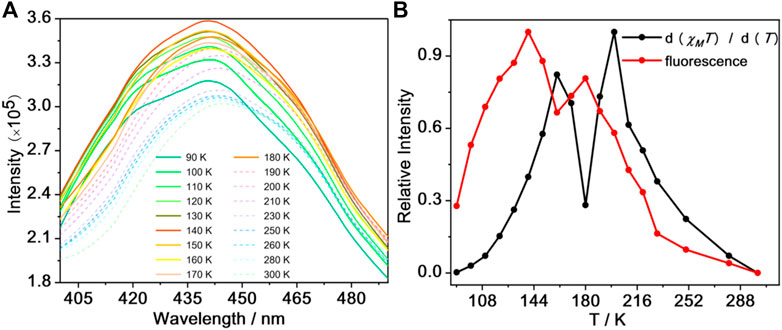
FIGURE 5. The fluorescence spectra for 1 over the temperature range 90–300 K (A) and the d (χMT)/d(T) curve (black line) and fluorescence normalized curve (red line) for 1(B).
To further check on this hypothesis, we have made normalized data of d (χMT)/dT and fluorescence intensity of 1 in the 90–300 K temperature region (warming mode) (Figure 5B). From this plot, the SCO transition profile of compound 1 is consistent with the change in fluorescence intensity, suggesting that the fluorescence change is related to the SCO transition; the slight difference between the peaks in Figure 5B is likely due to differences in the thermal sweep rates of the two experiments. We speculate that the main reason is the invertible electron transport between the antibonding orbitals of FeII ions and the lowest unoccupied molecular orbital of TPPE (Wang et al., 2015; Ge et al., 2019). For comparison, the temperature dependence fluorescence spectra of the precursor [FeIIL(MeOH)2] was measured. It exhibits weaker fluorescence signal under excitation at 360 nm from 90 to 280 K (Supplementary Figure 15). The emission intensity gradually increased from 90 to 260 K and it decreased from 260 to 280 K at about 435 nm, which may be attributed to the vibration of molecular geometry. In conclusions, the drastic temperature-dependent variations in emission intensity maybe assigned to the coordination of the N atom from bridging ligand and the central FeII ion from precursor [FeIIL(MeOH)2]. It can be evidenced that the almost monotone decreasing in the fluorescence intensity of TPPE can make clear the electron transport mechanism demonstrated above.
Conclusion
In summary, two FeII coordination polymers [FeIIL (TPPE)0.5] 1) and [(FeII3L3 (TPPE)1.5)] 2) have been successfully prepared by introducing the redox-active TTF unit as well as the fluorescent TPPE ligand. Magnetic investigations reveal that 1 exhibits SCO behaviour, while 2 remains in the LS state. Because of the synergetic effect between SCO and fluorescence, the changes of the spin state of complex 1 could regulate the luminescence intensity of the TPPE ligand. Moreover, the electrochemical studies show that these coordination polymers reserved the redox activity of the TTF unit. Further efforts aimed towards the preparation of diverse multifunctional SCO materials exhibiting higher transition temperature show great promise and are currently being undertaken in our laboratory.
Data Availability Statement
All datasets generated for this study are included in the article/Supplementary Material.
Author Contributions
Original idea was conceived by YQ, LC, JG, and JS; experiments and data analysis were performed by YQ, LC, JG, GM, and MK; structure characterization was performed by YQ, LC, and JS, manuscript was drafted YQ, JG, JS, GM, and MK All authors have given approval to the manuscript.
Funding
This work was supported by the National Natural Science Foundation (No. 21801054), the Natural Science Foundation of Zhejiang Province (No. LY20B010003), the China Postdoctoral Science Foundation (No. 2019M661788).
Conflict of Interest
The authors declare that the research was conducted in the absence of any commercial or financial relationships that could be construed as a potential conflict of interest.
Acknowledgments
Deanna M. D’Alessandro and Chanel Leong in School of Chemistry, the University of Sydney are acknowledged for their assistance in diffuse reflectance UV–Vis–NIR spectra, solid state electrochemical, and spectroelectrochemical measurements.
Publisher’s Note
All claims expressed in this article are solely those of the authors and do not necessarily represent those of their affiliated organizations, or those of the publisher, the editors and the reviewers. Any product that may be evaluated in this article, or claim that may be made by its manufacturer, is not guaranteed or endorsed by the publisher.
Supplementary Material
The Supplementary Material for this article can be found online at: https://www.frontiersin.org/articles/10.3389/fchem.2021.692939/full#supplementary-material.
References
Canevet, D., Sallé, M., Zhang, G., Zhang, D., and Zhu, D. (2009). Tetrathiafulvalene (TTF) Derivatives: Key Building-Blocks for Switchable Processes. Chem. Commun., 2245–2269. doi:10.1039/b818607n
De la Barrera, S. C., Sinko, M. R., Gopalan, D. P., Sivadas, N., Seyler, K. L., Watanabe, K., et al. (2018). Tuning Ising Superconductivity with Layer and Spin-Orbit Coupling in Two-Dimensional Transition-Metal Dichalcogenides. Nat. Commun. 9, 1427. doi:10.1038/s41467-018-03888-4
Delgado, T., Tissot, A., Guénée, L., Hauser, A., Valverde-Muñoz, F. J., Seredyuk, M., et al. (2018). Very Long-Lived Photogenerated High-Spin Phase of a Multistable Spin-Crossover Molecular Material. J. Am. Chem. Soc. 140, 12870–12876. doi:10.1021/jacs.8b06042
Garcia, Y., Robert, F., Naik, A. D., Zhou, G., Tinant, B., Robeyns, K., et al. (2011). Spin Transition Charted in a Fluorophore-Tagged Thermochromic Dinuclear Iron(II) Complex. J. Am. Chem. Soc. 133, 15850–15853. doi:10.1021/ja205974q
Ge, J. Y., Chen, Z., Zhang, L., Liang, X., Su, J., Kurmoo, M., et al. (2019). A Two‐Dimensional Iron(II) Coordination Polymer with Synergetic Spin‐Crossover and Luminescent Properties. Angew. Chem. Int. Ed. 58, 8789–8793. doi:10.1002/anie.201903281
Gong, Q., Hu, Z., Deibert, B. J., Emge, T. J., Teat, S. J., Banerjee, D., et al. (2014). Solution Processable MOF Yellow Phosphor with Exceptionally High Quantum Efficiency. J. Am. Chem. Soc. 136, 16724–16727. doi:10.1021/ja509446h
Gütlich, P., Garcia, Y., and Goodwin, H. A. (2000). Spin Crossover Phenomena in Fe(II) Complexes. Chem. Soc. Rev. 29, 419–427. doi:10.1039/b003504l
Halcrow, M. A. (2011). Structure:function Relationships in Molecular Spin-Crossover Complexes. Chem. Soc. Rev. 40, 4119–4142. doi:10.1039/c1cs15046d
Harding, D. J., Harding, P., and Phonsri, W. (2016). Spin Crossover in Iron(III) Complexes. Coord. Chem. Rev. 313, 38–61. doi:10.1016/j.ccr.2016.01.006
Hoshino, N., Iijima, F., Newton, G. N., Yoshida, N., Shiga, T., Nojiri, H., et al. (2012). Three-way Switching in a Cyanide-Bridged [CoFe] Chain. Nat. Chem. 4, 921–926. doi:10.1038/nchem.1455
Huang, G., Zhang, G., and Zhang, D. (2012). Turn-on of the Fluorescence of Tetra(4-Pyridylphenyl)ethylene by the Synergistic Interactions of Mercury(II) Cation and Hydrogen Sulfate Anion. Chem. Commun. 48, 7504–7506. doi:10.1039/c2cc32504g
Icli, B., Solari, E., Kilbas, B., Scopelliti, R., and Severin, K. (2012). Multicomponent Assembly of Macrocycles and Polymers by Coordination of Pyridyl Ligands to 1,4-bis(benzodioxaborole)benzene. Chem. Eur. J. 18, 14867–14874. doi:10.1002/chem.201202313
Kapadia, P. P., Magnus, M. A., Swenson, D. C., and Pigge, F. C. (2011a). Generation and Solid State Characterization of Tetrapyridinium Perchlorate Salts Derived from Tetrapyridyl Tetraphenylethylenes. J. Mol. Struct. 1003, 82–86. doi:10.1016/j.molstruc.2011.07.030
Kapadia, P. P., Widen, J. C., Magnus, M. A., Swenson, D. C., and Pigge, F. C. (2011b). Tetrapyridyl Tetraphenylethylenes: Supramolecular Building Blocks with Aggregation-Induced Emission Properties. Tetrahedron Lett. 52, 2519–2522. doi:10.1016/j.tetlet.2011.03.029
Kepp, K. P. (2016). Theoretical Study of Spin Crossover in 30 Iron Complexes. Inorg. Chem. 55, 2717–2727. doi:10.1021/acs.inorgchem.5b02371
Lochenie, C., Schötz, K., Panzer, F., Kurz, H., Maier, B., Puchtler, F., et al. (2018). Spin-crossover Iron(II) Coordination Polymer with Fluorescent Properties: Correlation between Emission Properties and Spin State. J. Am. Chem. Soc. 140, 700–709. doi:10.1021/jacs.7b10571
Narayan, T. C., Miyakai, T., Seki, S., and Dincă, M. (2012). High Charge Mobility in a Tetrathiafulvalene-Based Microporous Metal-Organic Framework. J. Am. Chem. Soc. 134, 12932–12935. doi:10.1021/ja3059827
Ni, Z.-P., Liu, J.-L., Hoque, M. N., Liu, W., Li, J.-Y., Chen, Y.-C., et al. (2017). Recent Advances in Guest Effects on Spin-Crossover Behavior in Hofmann-type Metal-Organic Frameworks. Coord. Chem. Rev. 335, 28–43. doi:10.1016/j.ccr.2016.12.002
Pigge, F. C., Kapadia, P. P., and Swenson, D. C. (2013). Halogen Bonded Networks from Pyridyl-Substituted Tetraarylethylenes and Diiodotetrafluorobenzenes. CrystEngComm. 15, 4386–4391. doi:10.1039/c3ce26732f
Qiu, Y.-R., Cui, L., Cai, P.-Y., Yu, F., Kurmoo, M., Leong, C. F., et al. (2020). Enhanced Dielectricity Coupled to Spin-Crossover in a One-Dimensional Polymer Iron(II) Incorporating Tetrathiafulvalene. Chem. Sci. 11, 6229–6235. doi:10.1039/d0sc02388d
Rodríguez-Jiménez, S., Feltham, H. L. C., and Brooker, S. (2016). Non-porous Iron(II)-based Sensor: Crystallographic Insights into a Cycle of Colorful Guest-Induced Topotactic Transformations. Angew. Chem. Int. Ed. 55, 15067–15071. doi:10.1002/anie.201608813
Rosario-Amorin, D., Dechambenoit, P., Bentaleb, A., Rouzières, M., Mathonière, C., and Clérac, R. (2018). Multistability at Room Temperature in a Bent-Shaped Spin-Crossover Complex Decorated with Long Alkyl Chains. J. Am. Chem. Soc. 140, 98–101. doi:10.1021/jacs.7b11042
Schönfeld, S., Dankhoff, K., Baabe, D., Zaretzke, M. K., Bröring, M., Schötz, K., et al. (2020). Iron(II) Spin Crossover Complexes Based on a Redox Active Equatorial Schiff-base-like Ligand. Inorg. Chem. 59, 8320–8333. doi:10.1021/acs.inorgchem.0c00725
Schönfeld, S., Hörner, G., Heinemann, F. W., Hofmann, A., Marschall, R., and Weber, B. (2021). Spin States of 1D Iron(II) Coordination Polymers with Redox Active TTF(py) 2 as Bridging Ligand. Z. Anorg. Allg. Chem. 647, 295–305. doi:10.1002/zaac.202000286
Smith, D. M. A., Dupuis, M., Vorpagel, E. R., and Straatsma, T. P. (2003). Characterization of Electronic Structure and Properties of a Bis(histidine) Heme Model Complex. J. Am. Chem. Soc. 125, 2711–2717. doi:10.1021/ja0280473
Su, J., Yuan, S., Wang, H.-Y., Huang, L., Ge, J.-Y., Joseph, E., et al. (2017). Redox-switchable Breathing Behavior in Tetrathiafulvalene-Based Metal-Organic Frameworks. Nat. Commun. 8, 2008. doi:10.1038/s41467-017-02256-y
Suleimanov, I., Kraieva, O., Sánchez Costa, J., Fritsky, I. O., Molnár, G., Salmon, L., et al. (2015). Electronic Communication between Fluorescent Pyrene Excimers and Spin Crossover Complexes in Nanocomposite Particles. J. Mater. Chem. C 3, 5026–5032. doi:10.1039/c5tc00667h
Tao, J., Wei, R.-J., Huang, R.-B., and Zheng, L.-S. (2012). Polymorphism in Spin-Crossover Systems. Chem. Soc. Rev. 41, 703–737. doi:10.1039/c1cs15136c
Wang, C.-F., Li, R.-F., Chen, X.-Y., Wei, R.-J., Zheng, L.-S., and Tao, J. (2015). Synergetic Spin Crossover and Fluorescence in One-Dimensional Hybrid Complexes. Angew. Chem. Int. Ed. 54, 1574–1577. doi:10.1002/anie.201410454
Wang, H.-Y., Cui, L., Xie, J.-Z., Leong, C. F., D’Alessandro, D. M., and Zuo, J.-L. (2017b). Functional Coordination Polymers Based on Redox-Active Tetrathiafulvalene and its Derivatives. Coord. Chem. Rev. 345, 342–361. doi:10.1016/j.ccr.2016.10.011
Wang, H. Y., Ge, J. Y., Hua, C., Jiao, C. Q., Wu, Y., Leong, C. F., et al. (2017a). Photo‐ and Electronically Switchable Spin‐Crossover Iron(II) Metal-Organic Frameworks Based on a Tetrathiafulvalene Ligand. Angew. Chem. Int. Ed. 56, 5465–5470. doi:10.1002/anie.201611824
Wang, J.-L., Liu, Q., Meng, Y.-S., Liu, X., Zheng, H., Shi, Q., et al. (2018b). Fluorescence Modulation via Photoinduced Spin Crossover Switched Energy Transfer from Fluorophores to FeII Ions. Chem. Sci. 9, 2892–2897. doi:10.1039/c7sc05221a
Wang, Y., Ying, J., Zhou, Z., Sun, J., Wen, T., Zhou, Y., et al. (2018a). Emergent Superconductivity in an Iron-Based Honeycomb Lattice Initiated by Pressure-Driven Spin-Crossover. Nat. Commun. 9, 1914. doi:10.1038/s41467-018-04326-1
Weber, B. (2009). Spin Crossover Complexes with N4O2 Coordination Sphere-The Influence of Covalent Linkers on Cooperative Interactions. Coord. Chem. Rev. 253, 2432–2449. doi:10.1016/j.ccr.2008.10.002
Yuan, J., Wu, S.-Q., Liu, M.-J., Sato, O., and Kou, H.-Z. (2018). Rhodamine 6G-Labeled Pyridyl Aroylhydrazone Fe(II) Complex Exhibiting Synergetic Spin Crossover and Fluorescence. J. Am. Chem. Soc. 140, 9426–9433. doi:10.1021/jacs.8b00103
Zappe, L., Schönfeld, S., Hörner, G., Zenere, K. A., Leong, C. F., Kepert, C. J., et al. (2020). Spin Crossover Modulation in a Coordination Polymer with the Redox-Active Bis-Pyridyltetrathiafulvalene (py2TTF) Ligand. Chem. Commun. 56, 10469–10472. doi:10.1039/d0cc03788e
Keywords: coordination polymers, tetrathiafulvalene, schiff-base ligand, spin-crossover, fluorescence
Citation: Qiu Y-R, Cui L, Ge J-Y, Kurmoo M, Ma G and Su J (2021) Iron(II) Spin Crossover Coordination Polymers Derived From a Redox Active Equatorial Tetrathiafulvalene Schiff-Base Ligand. Front. Chem. 9:692939. doi: 10.3389/fchem.2021.692939
Received: 09 April 2021; Accepted: 19 July 2021;
Published: 02 August 2021.
Edited by:
Alessandro Pratesi, University of Pisa, ItalyReviewed by:
Birgit Weber, University of Bayreuth, GermanyMiguel Clemente-León, University of Valencia, Spain
Copyright © 2021 Qiu, Cui, Ge, Kurmoo, Ma and Su. This is an open-access article distributed under the terms of the Creative Commons Attribution License (CC BY). The use, distribution or reproduction in other forums is permitted, provided the original author(s) and the copyright owner(s) are credited and that the original publication in this journal is cited, in accordance with accepted academic practice. No use, distribution or reproduction is permitted which does not comply with these terms.
*Correspondence: Jian Su, c3VqaWFuQG5qdS5lZHUuY24=; Jing-Yuan Ge, Z2VqaW5neXVhbjkwQDEyNi5jb20=; Mohamedally Kurmoo, a3VybW9vQG5qdS5lZHUuY24=; Guijun Ma, bWFnakBzaGFuZ2hhaXRlY2guZWR1LmNu