- Department of Chemistry, Indian Institute of Technology, Guwahati, India
Rapid rise of antimicrobial resistance against conventional antimicrobials, resurgence of multidrug resistant microbes and the slowdown in the development of new classes of antimicrobials, necessitates the urgent development of alternate classes of therapeutic molecules. Antimicrobial peptides (AMPs) are small proteins present in different lifeforms in nature that provide defense against microbial infections. They have been effective components of the host defense system for a very long time. The fact that the development of resistance by the microbes against the AMPs is relatively slower or delayed compared to that against the conventional antibiotics, makes them prospective alternative therapeutics of the future. Several thousands of AMPs have been isolated from various natural sources like microorganisms, plants, insects, crustaceans, animals, humans, etc. to date. However, only a few of them have been translated commercially to the market so far. This is because of some inherent drawbacks of the naturally obtained AMPs like 1) short half-life owing to the susceptibility to protease degradation, 2) inactivity at physiological salt concentrations, 3) cytotoxicity to host cells, 4) lack of appropriate strategies for sustained and targeted delivery of the AMPs. This has led to a surge of interest in the development of synthetic AMPs which would retain or improve the antimicrobial potency along with circumventing the disadvantages of the natural analogs. The development of synthetic AMPs is inspired by natural designs and sequences and strengthened by the fusion with various synthetic elements. Generation of the synthetic designs are based on various strategies like sequence truncation, mutation, cyclization and introduction of unnatural amino acids and synthons. In this review, we have described some of the AMPs isolated from the vast repertoire of natural sources, and subsequently described the various synthetic designs that have been developed based on the templates of natural AMPs or from de novo design to make commercially viable therapeutics of the future. This review entails the journey of the AMPs from their natural sources to the laboratory.
Introduction
In the wake of the post-pandemic resurgence, the greatest challenge faced by the civilized world are the antimicrobial infections. Rapidly growing antibiotic resistance (Mathur and Singh, 2005) accompanied by the slowdown of the development of the newer antibiotics (Ventola, 2015) in the recent past, is a reason for major concern. Scientists have been trying to look for alternate strategies or new classes of molecules to combat against the microbes. Antimicrobial peptides (AMPs) are small proteins present in a various plant and animal species that act as the first line of defense against the microbes. Their existence in nature for over millions of years and sustained capability to combat antimicrobial infections is a testimony of complete absence of or very slow development of resistance against them (Yu et al., 2018). This makes them specifically desirable in comparison to the antibiotics, which develop resistance pretty fast. The absence/slow development of resistance against microbes may be attributed to the presence of various modes/mechanisms of action of the AMPs against the bacteria in comparison to the fixed targets used by the antibiotics (Papo and Shai, 2003; Brogden, 2005; Nicolas, 2009). Additionally, AMPs breakdown into amino acids unlike other therapeutics, which might generate potentially harmful metabolites.
AMPs are produced as secondary metabolites and contain upto about 50 amino acid residues. They greatly vary in their sequence, secondary structural preferences, and in their modes of action. While a large variety of AMPs are cationic in nature (CAMPs) containing about +2 to +9 charge in them (Cascales et al., 2018), there can be anionic AMPs (AAMPs) (Harris et al., 2009; Lai et al., 2002; Steffen et al., 2006) and completely hydrophobic AMPs (Epand and Vogel, 1999) as well. Secondary structures of AMPs vary from α-helices [magainin (Zasloff, 1987)], protegrin (Fahrner et al., 1996), LL-37 (Scott et al., 2002), β-sheets [α, β-defensins (Selsted et al., 1985a; Selsted et al., 1985b)], drosomycin (Fehlbaum et al., 1994), thionins (Stec, 2006), plectasin (Mygind et al., 2005), mixed αβ structures [RNase 2, RNase 3 (Hamann et al., 1990)] to extended helices and loop structures (Hancock, 1997; Jenssen et al., 2006) (Figure 1). While membranolysis (Sitaram and Nagaraj, 1999) or disruption in the synthesis of the cell membrane/cell wall is considered as one of the major modes of action of the AMPs, other AMPs have intracellular targets and interfere with the vital intracellular processes (Scocchi et al., 2016). Some AMPs are known to have an immunomodulatory effect on the innate immune system of the host (Hancock and Sahl, 2006). AMPs can belong to various chemical classes like cationic (Findlay et al., 2010), hydrophobic (Schmidtche et al., 2014), amphipathic, disulfide bonded (Krause et al., 2000), cyclic (Dartois et al., 2005) and lipidated (Hu et al., 2012).
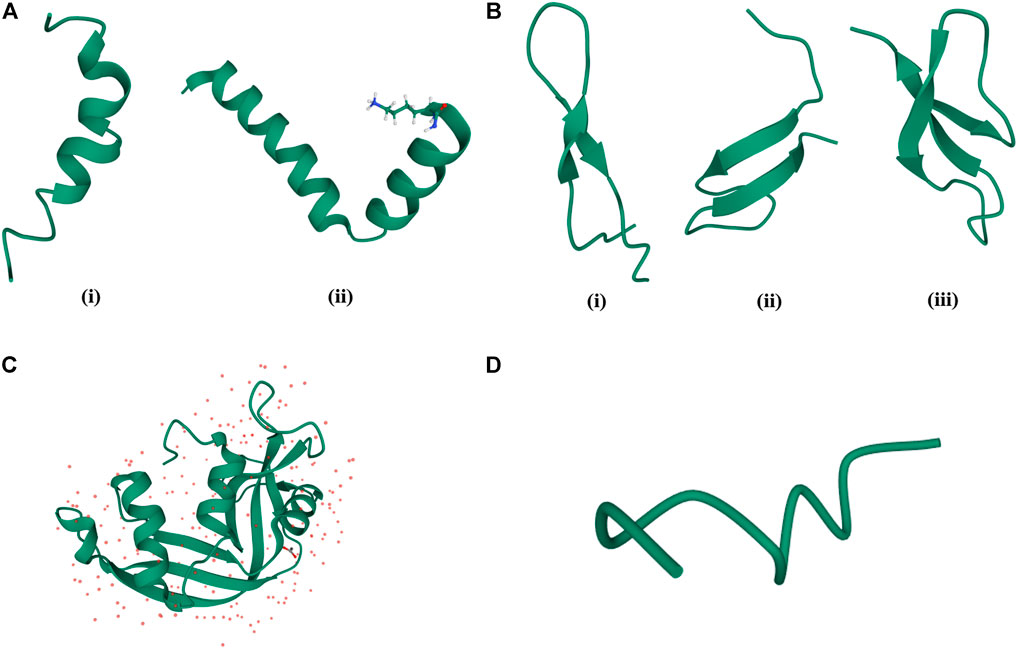
FIGURE 1. Classification of AMPs based on their structures. (A) α-helical AMPs: (i) Magainin (PDB code—2LSA), (ii) Cecropin (PDB code—2LA2). (B) β-sheet AMPs: (i) Bovine Lactoferrin (PDB code—1LFC), (ii) Hepcidin (PDB code—2KEF), (iii) Rabbit Kidney Defensins (PDB code—1EWS). (C) α-helix, β-sheet mixed AMPs: RNase 2 (PDB code—1GQV). (D) Random coil structure of AMPs: Cys deleted protein (PDB code—2MQ5).
Over the last couple of decades, thousands of AMPs have been isolated from different kingdoms of life (Mishra et al., 2018). There are several databases like APD3 (Wang et al., 2016), CAMP (Waghu and Thomas, 2020), DrAMP2.0 (Kang et al., 2019) etc. which catalog the various AMPs that are known to date. AMPs are produced by microorganisms to protect them from other microbes. Some of the AMPs are shared by different classes of eukaryotes like plants and animals (defensins and cyclotides), others are only specific to plants (heveins), while others are specific to certain plant families (snakins). When compared to the other organisms, plants tend to have a higher amount of AMPs due to gene duplications or polyploidy. This may also be attributed to their immobility, which disables them to avoid the biotic and environmental conditions. Thionins, plant defensins, heveins, snakins are some plant-derived AMPs (Nawrot et al., 2014; Tam et al., 2015). Several AMPs like japonicin 2, nigrocin 2, temporin, dermaseptin, magainin, buforin II (Conlon and Mechkarska, 2014; Patocka et al., 2019; Casciaro et al., 2020) have been isolated from the amphibians (Wang et al., 2016), which have broad-spectrum antimicrobial activities against bacteria, fungi, yeast, protozoa and virus along with some potential in cancer therapy (Rabanal and Cajal, 2016). Cepropin, thanatin, defensin, drosomycin, apidaecin, abaecin, melittin are some of the insect derived AMPs (Mylonakis et al., 2016). Crustaceans produce several AMPs like callinectin, homarin, penaeidin, hyastatin, arasin (Zanjani et al., 2018). Mammals produce AMPs like defensins, histatins, LL-37, indolicidin, protegrins and lactoferrins (Wang, 2014; Dutta and Das, 2016). In animals, AMPs are isolated mostly from tissues and organs that are exposed to airborne pathogens like skin, eyes, ears, mouth, airways, lungs, intestines, urinary tract etc. and acts as the first line of innate immune defense (Schauber and Gallo, 2008; Hilchie et al., 2013).
In spite of being a very promising alternative class of therapeutics, only a very few of the AMPs have been commercialized so far. This is owing to several inherent disadvantages of natural AMPs. These include limited quantities of AMPs isolated from the natural sources, uneconomic synthesis of longer AMP sequences, folding hurdles related to the natural AMPs, short half-life of the natural AMPs owing to protease degradability systemic toxicity and delivery issues to the target site. Inspired by nature and to address the shortcomings of the natural AMPs, research on synthetic AMPs has been explored extensively over the last few decades. In this review, we briefly recounted the journey of the natural AMPs from different kingdoms of life to the mainstream of synthetic research. As the field of AMP research is huge, and there are several elaborate reviews on the subtopics discussed here, this review does not claim to be exhaustive in nature. Instead, we have tried to represent and discuss 1) the various chemical classes of AMPs from different kingdoms of life which act as their natural reservoirs, 2) major shortcomings of the natural AMPs which limit their commercial applications, 3) how natural AMPs have influenced and inspired the synthetic AMP research and 4) how the synthetic AMP research addresses the shortcomings of the natural AMPs to make effective therapeutic antimicrobials of the future. In short, this review very briefly depicts the current scenario in the pursuit of the development of AMPs as future commercial therapeutic agents.
Natural Antimicrobial Peptides
There are different ways in which AMPs can be classified. These rely on their physicochemical properties, secondary structure, sources, modes of action etc. In this review, we have broadly classified the AMPs into 1) cationic natural AMPs (Table 1), 2) Cyclic AMPs (Table 1) and 3) AMPs rich in specific amino acid residues like tryptophan (Trp), proline (Pro), histidine (His) and glycine (Gly) (Table 1).
Cationic Antimicrobial Peptides
A large class of natural AMPs have positive charge (+2 to +9) due to the abundance of Lysine (Lys) and Arginine (Arg) residues (Hancock, 1997; Hancock and Rozek, 2002; Powers and Hancock, 2003; Brown and Hancock, 2006; Fernández-Vidal et al., 2007; Mangoni et al., 2007; Mookherjee et al., 2020) in them (Figure 2 and Table 1). This gives an electrostatic advantage to the AMPs in binding to the negatively charged microbial membranes. Often these peptides have amphipathic structures where the positive part of the AMP is separated from the hydrophobic part of the AMP in the tertiary structure. Most of the cationic AMPs have membrane disruptive/lytic mode of action.
Thionins are a class of small cationic plant AMPs rich in Lys, Arg and Cysteine (Cys) residues and are present in all the crucial plant tissues from endosperms to leaves. They are toxic against bacteria, fungi and yeasts. To date about 100 individual thionins have been isolated from about 15 plant species (Stec, 2006; Hussain et al., 2014; Kul'ko et al., 2016). Thionins have two antiparallel α helices and an antiparallel double stranded β-sheet with three or four disulfide bridges. Puroindolins (PINs) are small basic proteins isolated from wheat endosperms that have a unique Trp rich domain and five disulfide bridges. PINs have a conserved tertiary structure that is formed from four helices connected by variable loops, the tertiary structure being held together by five disulfide bridges. Antimicrobial activity of PINs is related to the interactions with the microbial membranes. Defensins are another class of small, cationic AMPs which are rich in Arg and Cys residues. They are present in various domains of life like plants, insects, animals and humans, and are highly active against a large range of microorganisms. Defensins have β-hairpin structures stabilized by three/four disulfide bonds. Defensins bind to membranes to form pore like membrane defects that lead to efflux of intracellular materials and eventual cell death. Plant defensins have antibacterial (Sitaram, 2006), antifungal (Gao et al., 2000), α-amylase and trypsin inhibitory activities (Wijaya et al., 2000). They are also active against yeast, oomycetes and necrotrophic pathogens (Segura et al., 1998; Van der Weerden et al., 2010). Additionally, they manifest anticancer and antiviral activities (Ngai and Ng, 2005; Wong and Ng, 2005). Plant defensins have a conserved structure with three stranded β-sheet and a helix stabilized by four disulfide bridges. Human defensins are of three types-α, β and θ. There are six subclasses of α defensins containing three conserved disulfide connectivities in between Cys I−VI, Cys II−IV and Cys III−V (Ganz, 2001). β-defensins are longer in sequence than the α defensins and have CysI−V, CysII−IV, CysIII−VI disulfide bond connectivities. There are various subclasses of human β-defensins (hBD). While hBD1 and hBD2 are rendered inactive in the presence of physiological salt concentrations (Goldman et al., 1997; Bals et al., 1998), hBD3 retains its activity against Staphylococcus aureus (S. aureus) and vancomycin resistant Enterococcus faecium at physiological salt concentrations (Harder and Bartels, 2001). Bioinformatics approaches have led to the identification of 23 additional human β-defensins from the disulfide bond connectivities, many of which have antimicrobial activities. Insect defensins are about 29–34 amino acids long with activity against both gram-positive and gram-negative bacteria (Hoffmann and Hetru, 1992), though the virulence against the gram-positive bacteria (S. aureus) (Hetru et al., 2003) is more than the gram-negative family (Gomes and Fernandes, 2010).
Cecropins are a family (seven subclasses) of small, cationic AMPs isolated from the hemolymph of giant silk moth Hyalophora cecropia (Steiner et al., 1981). Cecropins are active against gram-positive, gram-negative bacteria and fungi. Cecropins form pores in the bacterial membranes, cause membrane depolarization and lysis (Moore et al., 1996; Bechinger and Lohner, 2006). Bactericidin, lepidopteran and sarcotoxins are structurally related to cecropins (Ouyang et al., 2015). Melittin, a 26 amino acid residue long positively charged peptide hormone present in bee venom, is effective against several strains of bacteria (Jamasbi et al., 2018; Chen et al., 2016). Melittin is also reported to have anti-inflammatory properties (Lee and Bae, 2016) and activity against xanthomonas oryzae, a pathogenic bacteria causing destructive bacterial disease in rice (Shi et al., 2016). Melittin interacts with negative microbial membranes, leads to pore formation, efflux of intracellular material and eventual cell death (Rady et al., 2017). Melittin forms an amphipathic helical structure in the presence of microbial membrane mimics. The activity of melittin is highly dependent on a Pro residue in its primary sequence (Jamasbi et al., 2016). Melittin causes apoptosis in C. albicans (Candida Albicans) through reactive oxygen species (ROS) mediated mitochondria and caspase pathway (Lee and Lee, 2014). Melittin exhibits strong synergistic effects with the conventional antibiotics against MDR isolates of Acenatibactor baumanni and Pseudomonas aeruginosa (P. aeruginosa) (Akbari et al., 2019) and effective antibacterial activity against Methicillin resistant S. aureus (MRSA) strains (Choi et al., 2015). Jelleines are a family of very small peptides (8-9 amino acid residues long) with +2 positive charge, which are isolated from the royal jelly of honey bees (Fontana et al., 2004) (Figure 2A). Jelleines I-III possess antimicrobial properties against yeast, fungi, gram-positive and gram-negative bacteria (Fontana et al., 2004; Jia et al., 2018).
Magainins are a family of small Gly rich cationic AMPs which are isolated from the skin of African clawed frog Xenopus laevis (Figure 2B). They are active against a large number of bacterial and fungal species and cause osmotic lysis of the protozoa. Magainins form amphipathic α-helical conformations in membrane lipid environments. Esculetins are a family of cationic AMPs (46 amino acids long) isolated from frog skin possessing broad-spectrum antimicrobial properties (Simmaco et al., 1994; Ponti et al., 1999). Microbial membranes are the major target of this class of AMPs (Mangoni et al., 2006). Esculentin-2CHa is a 37 residue cationic antimicrobial peptide isolated from the skin of amphibians like Lithobates chiricahuensis (Conlon et al., 2011). This peptide is predominantly α-helical in structure with broad-spectrum antimicrobial properties (Vasu et al., 2017a) and low cytotoxicity. Temporins are yet another family of very small α-helical cationic AMPs, with low net positive charge, that are isolated from frog skin (Mangoni, 2006). They are highly potent against a wide range of pathogens like bacteria, fungi, viruses, yeasts and protozoa. Temporins are effective against gram-positive bacterial strains like MRSA and vancomycin resistant E. faecium and Enterococcus faecalis (E. faecalis) (Giacometti et al., 2005). They are non-cytotoxic to mammalian cells and preserve their activity in the presence of serum (Mangoni et al., 2005). Nigrocin 1 and 2 isolated from the skin of Rana nigromaculata, manifested broad-spectrum antimicrobial activity against various microorganisms through membranolytic mode of action (Park C. H. et al., 2001). Buforin II, a 21 amino acid residue long cationic AMP was derived from another less active AMP called Buforin I, isolated from Asian toad Bufo bufo garagrizans (Park et al., 1996). Buforin II has broad-spectrum antimicrobial activity. Buforin II is non membranolytic and has a very strong affinity for the DNA and the RNA present in the cells (Park et al., 1998). Buforin II has a helix-hinge-helix structure with the hinge at Pro11 residue. N terminal helix contains residues 5–10, while the C terminal helix spans across residues 12–21. Systematic structure activity studies revealed that the α-helical content of the peptide is critical for the antimicrobial potency of Buforin (Yi et al., 1996). Additionally, Pro hinge is extremely important for the cell-penetrating ability of the peptide. Mutation of this Pro converted the peptide into a regular membrane active AMP (Park et al., 2000).
Cathelicidins are a class of human AMPs which are basic in nature. The mature protein was isolated from the neutrophils (Gudmundsson et al., 1996), human skin (Yamasaki et al., 2006) and sweat (Murakami et al., 2002). The peptide contains 37 amino acids and starts with a di-Leucine (Leu) (LL) unit and hence is called LL-37 peptides. LL-37 is active against a whole range of bacteria like Escherichia Coli (E. coli), S. aureus, P. aeruginosa and others. Hepcidins are cationic AMPs, 20–25 residues long with low positive charges and are highly disulfide bridged. Human Liver expressed AMP (LEAP-1) was discovered from human blood filtrate (Krause et al., 2000) and urine (Park S. et al., 2001) and was named as Hepcidin 25. It is involved in the iron homeostasis in the body (Pigeon et al., 2001). AMP LEAP-2 is 40 amino acid residues long and does not have any role in iron regulation of the body (Krause et al., 2003).
Some human proteins are antimicrobial in nature. Lysozyme, a positively charged enzyme that is present in animals, forms part of the innate immune system. Lysozyme is a glycosidic hydrolase that catalyses the hydrolysis of 1-4 β-linkages in between the N acetyl muramic acid and N acetyl D glucosamine residues in peptidoglycan that forms active components of the gram-positive bacterial cell wall. This hydrolysis compromises the integrity of the cell wall and causes lysis of the cell. Lysozyme is present abundantly in the secretions like tears, saliva, mucous, human milk, in macrophages and in neutrophils (Manchenko, 1994). Five of the eight ribonucleases have antimicrobial activity. RNase 2 and RNase 3 are both cationic proteins which have anti-parasitic (Hamann et al., 1990) and antiviral activity (Domachowske et al., 1998). RNase 3 also possesses bacterial agglutinating property (Pulido et al., 2013). RNase 7 isolated from human skin (Harder and Schroder, 2002) is active against bacteria and yeast even at 4°C (Huang et al., 2007) and is one of the most abundant innate defense peptide present in the urinary tract (Spencer et al., 2013). RNase 5 is found to have toxic effects against gram-positive bacteria and fungi (Hooper et al., 2003).
Cyclic Antimicrobial Peptides
Cyclic AMPs derived from microbes are produced by non-ribosomal pathways by non-ribosomal protein synthetases (NRPs). They contain both proteinogenic and unnatural amino acid residues in them. Functional diversity of these molecules are enhanced by the introduction of various chemical modifications, some of which are epimerization, N-methylation, and heterocyclization (Hur et al., 2012). A large number of cyclic peptides have been isolated from natural resources (Figure 3; Table 1). Cyclic AMPs disrupt the structural integrity of the cells, by disrupting the membrane or targeting the biosynthesis of components of the microbial cell envelope like chitin, glucan, mannoproteins and sphingolipids. Some cyclic AMPs bind to the membrane and cause membranolysis, efflux of intracellular materials and cell death, while others bind to the membrane, traverse them and act upon specific membrane bound structures like ion channels, transporters and receptors. Natural cyclic peptides can be classified depending on the mode of cyclization (Figure 4) or their physicochemical properties. Homodetic cyclic AMPs are formed from the cyclization in between the terminal amino group and the carboxylic acid group. Argyrin B, guangomide, valinomycin (Figure 3A) are some representative examples of homodetic cyclic AMPs. Heterodectic cyclic AMPs are formed from the cyclization in between the side chains of two amino acid residues or in between side chain of one amino acid residue and a terminal amino/carboxylic acid group. Coibamide A, didemnin B, selenamide, luzopeptin are some of the representative examples of this class. Complex cyclic peptides are formed from the mixture of homodetic and heterodetic linkages and may be bicyclic in nature as in the case of amatoxins, amanitin (Figure 3B), phalloidin and triostin A (Lee and Kim, 2015). Cyclic peptides can be charged as in gramicidine S (Figure 3C), polymyxin B (Figure 3D) and bactenecin, non-polar as in argyrin B, aureobasidin and guanomide A, of mixed polarities as in kahalilide F, largamide and pseudodesmin A or containing cyclic cysteine knot (CCK) like kalata B1.
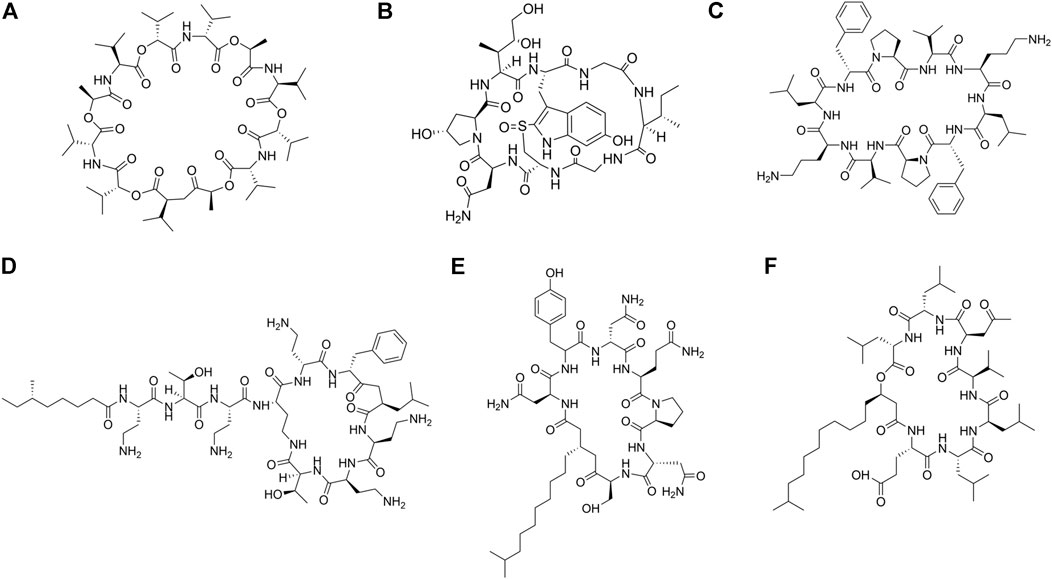
FIGURE 3. Examples of natural cyclic AMPs. (A) Valinomycin. (B) Alpha-Amanitin. (C) Gramicidin S. (D) Polymyxin B. (E) Iturin A. (F) Surfactin C.
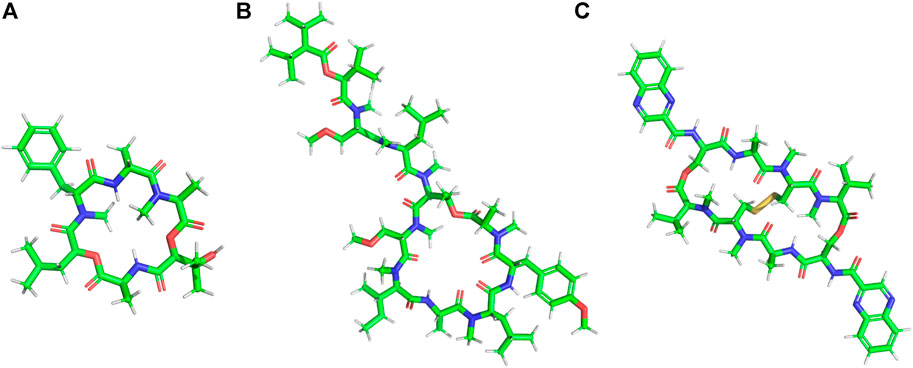
FIGURE 4. Different modes of cyclization in cyclic AMPs. (A) Homodectic cyclic peptides (head-to-tail cyclized): Guangomide A. (B) Heterodectic cyclic peptide (head/tail-to-side chain or side chain-to-side chain cyclized): Coibamide A. (C) Complex cyclic peptide: Triostin A.
Cyclic Lipopeptides (CLPs) contain a fatty acid tail attached to a cyclic peptide skeleton. These peptides possess broad-spectrum antifungal and antibiotic properties. They mostly act through membrane integration and formation of pores, thereby causing the leakage of the intracellular components and causing eventual cell death. Syringomycins and syringopeptides derived from Pseudomonas syringae, possess strong inhibitory activity against the growth of gram-positive bacteria (Grgurina et al., 2005), mycelia (Lavermicocca et al., 1997) and antagonistic property against apple scab causing agent (Burr et al., 1996). Lipodepsipeptide Tolaasin D derived from Pseudomonas tolaasi prevents growth of Rhizoctania solani and gram-positive bacteria Rhodococcus fascians (Bassarello et al., 2004). Gram-negative bacteria Pseudomonas fluorescence produces several classes of cyclic AMPs. Pseudophomins A and B produced by pseudomonas fluorescens BRG100 significantly inhibit the growth of Phoma lingam, Alternaria brassicae and Sclerotinia sclerotiorum (Pedras et al., 2003). Massetolide A produced by P. fluorescens SS101 possesses a biosurfactant property that affects plant pathogenic oomycetes. Amphisin, lokisin, tensin, and viscosinamide produced by P. fluorescens spp. possess in vitro antagonistic activities against the oomycete Pythium ultimum and the basidiomycete Rhizoctonia solani (R. solani) (Nielsen et al., 2002; Nielsen and Sorensen, 2003). Bacillus species are major producers of CLP’s. Fusaricidins, iturins (Figure 3E), fengycins, polymixins, and agrastatins have been identified in culture extracts of several species of Bacillus (Stein, 2005). These CLPs are composed of seven (surfactins (Figure 3F), iturins) or ten (fengycins) α-amino acids linked to one unique β-amino (iturins) or β-hydroxy (surfactins, fengycins) fatty acid. The carbon numbers of β-amino fatty acid chain in surfactins, iturins, and fengycins are C13-C16, C14-C17, and C14-C18, respectively. Multiple derivatives of each lipopeptide family are usually co-produced by a strain (Leenders et al., 1999; Akpa et al., 2001). Iturins have antifungal activity against plant pathogens by pore formation in the cell membranes, causing an efflux of potassium and other vital ions eventually causing cell death (Thimon et al., 1992). Fengycin type of cyclic peptides produced by B. subtilis (Bacillus subtilis) M4 prevents the mycelial growths of Fusarium oxysporum, R. solani and B. cinerea and prevents grey mold disease of apples caused by B. cinerea during storage (Ongena et al., 2005). B. subtilis CMB32 produces iturin A, fengycin and surfactin A, which are able to suppress the growth of Colletotrichum gloeosporioides, the causative agent of anthracnose in a variety of crops (Kim et al., 2010). Surfactins produced by the Bacillus subtlis are one of the most effective bio-surfactant known till date and is known to have strong antimicrobial activity against C. albicans, Lactococcus lacti subsp. Lactis, Mycobacterium smegmatis, S. aureus, and Streptococcus mutant (Peypoux et al., 1999; Singh and Cameotra, 2004). Balliomycin produced by Bacillus species contains seven α-amino acids and β-amino fatty acids with 14–16 carbon atoms and can control a broad range of plant diseases. Mycosubtilin are heptapeptides containing alternating D and L amino acid residues closed by a β amino acid linkage. B. subtilis strains overproducing mycosubtilin are effective in controlling Pythium damping off on tomato plants than the parent strain (Leclère et al., 2005). Polymixin B produced by the Paenibacillus sp. strain B2 have antifungal activity against root pathogenic fungus Fusarium solani and Fusarium acuminatum (Selim et al., 2005). Fusaridins are a group of depsipeptides produced by the Paenibacillus polymyxa E681 that control the Phytopthora blight infection in red pepper caused by Phytopthora capsica (Lee et al., 2013). Antibiotic Agrastatin A produced by B. subtilis AQ713 has a broad fungicidal spectrum in vitro (Heins et al., 2000). Gramicidin S produced by Brevibacillus Brevis has both antifungal activity and sporicidal activity (Murray et al., 1986). Daptomycin is a CLP antibiotic that is isolated from soil bacteria Streptomyces roseosporus. It is used in treating life threatening infections caused by gram-positive bacteria including MRSA and vancomycin resistant Enterococci. Daptomycin has a unique mechanism of action that leads to the destruction of membrane potential (Steenbergen et al., 2005). It is commercially available as a peptide antibiotic drug. Several cyclopeptides are produced by Streptomyces species. Valinomycin, first identified from Streptomyces griseus has insecticidal, acaricidal (Heisey et al., 1988), antifungal (Park et al., 2008) and antioomecyte activity (Lim et al., 2007). It acts as an ionophore and modulates the transport of potassium ions across biological membranes.
Some natural cyclic AMPs block the biosynthesis of the cellular components. Chitin is a linear homopolymer of β-(1,4)-linked N-acetylglucosamine (GlcNAc) residues, and a major component of the fungal cell walls. Polyoxins and Nikkomycins are nucleoside peptide antibiotics that are competitive inhibitors of chitin synthase (Isono and Suzuki, 1979). Arthrichitin is a cyclic depsipeptide that inhibits chitin biosynthesis and works as an antifungal agent (Vijayakumar et al., 1996). Thiopeptide antibiotics are an important class of natural products that are formed from the post‐translational modifications of the ribosomally synthesized peptides. Cyclothiazomycin is a typical example that has a unique bridged macrocyclic structure derived from an 18 amino acid residue peptide (Wang et al., 2010). Cyclothiazomycin B1 produced by Streptomyces species acts as an antifungal cyclic thiopeptide that binds to chitin in the fungal cell wall and disrupts the crystallization of the chitin chain. This mechanism inhibits the growth of filamentous fungi and causes swelling of the hyphae and the spores (Mizuhara et al., 2011). Glucans are homopolymers of glucose that form a network of fibrils around the fungal cell that helps to maintain the mechanical strength and the osmotic resistance of the fungal cell wall (Smits et al., 1999). The enzyme β-(1,3)-glucan synthase catalyzes polymerization of glucan. Neopeptins, produced by Streptomyces species have potent antifungal properties inhibiting mannoprotein, proteoheteroglycan and β-1-3-glycan synthases found in Sacharomyces cerevisiae (Satomi et al., 1982). Growth of several microbes like Glomerella cingulata, Pleomorphomonas oryzae (P. oryzae), Cochliobolus miyabeanus, and Colletotrichum lagenaium can be completely inhibited by neopeptins. Aureobasidins are cyclic depsipeptide antifungal antibiotics that are produced from Aureobasidium pullulans (Takesako et al., 1991). These are composed of eight lipophilic amino acid residues and one α-hydroxy acid (Ikai et al., 1991a), Ikai et al., 1991b)) and are effective against a large range of fungi and protozoa. Aureobasidin A inhibits inositol phosphorylceramide (IPC) synthase, a key enzyme catalyzing sphingolipid synthesis in fungi.
Cyclotides are groups of homodectic circular proteins that are isolated from plants and animals (Pelegrini et al., 2007; Craik, 2010). Cyclotides have high sequence similarity. They contain three intramolecular disulfide bridges arranged in the form of a CCK(Colgrave and Craik, 2004). The CCK provides cyclotides with exceptional stability towards various proteolytic and degradative processes (Ireland et al., 2010). Kalata B8 isolated from Oldenlandia affins (Pelegrini et al., 2007) has a diverse range of biological activities, including uterotonic, anti-HIV, antimicrobial, insecticidal, antihelmintic, and molluscidal properties (Craik, 2010). Kalata B1 interacts with the phosphotidylethanolamine phospholipid present in the membranes leading to membrane bending and vesicle formation. Hevein is a small Cys rich chitin binding protein that is present in the lutoid bodies of the rubber tree (Van Parijs et al., 1991), that inhibits the hyphal growth of fungi. Different hevein like proteins with antimicrobial properties have been isolated from other plants (Koo et al., 1998; Kiba et al., 2003; Huang et al., 2004; Porto et al., 2012). These peptides are small, 20–40 amino acids long, rich in Cys and Gly residues at conserved positions. Hevein like peptides have eight (Li and Claeson, 2003), six (Huang et al., 2000) or ten (Huang et al., 2002) Cys residues in them, giving rise to conserved disulfide bridges.
Ranacyclins E and T are 17 residue cyclic peptides from amphibians that exhibit antimicrobial and antifungal activity (Mangoni et al., 2003a). Theta (θ) defensins found in non-human primates are18 residue cyclic peptides formed by head-to-tail cyclization of the peptide (Tang et al., 1999; Selsted, 2004). Like the α and the β-defensins, this class of peptides contain three disulfide bridges in between the Cys I-IV, Cys II-V and Cys III-IV. These genes are not expressed in humans due to the presence of a premature stop codon. Like monkey θ defensins, synthetic versions of the human analogs have anti-HIV activity (Cole et al., 2004; Yang et al., 2005).
Antimicrobial Peptides rich in specific amino acid residues (Tryptophan/Proline/Histidine/Glycine)
Many AMPs have very high content of a certain amino acid residues like Trp (Chan et al., 2006; Dong et al., 2012), Pro (Kragol et al., 2002), Gly (Baumann et al., 2010; Ili´c et al., 2013) or His (Oppenheim et al., 1988) (Figure 5; Table 3). These AMPs are usually extended having no regular structure and may be cationic or anionic in nature. Many of these peptides are known to have membrane permeability and intracellular targets.
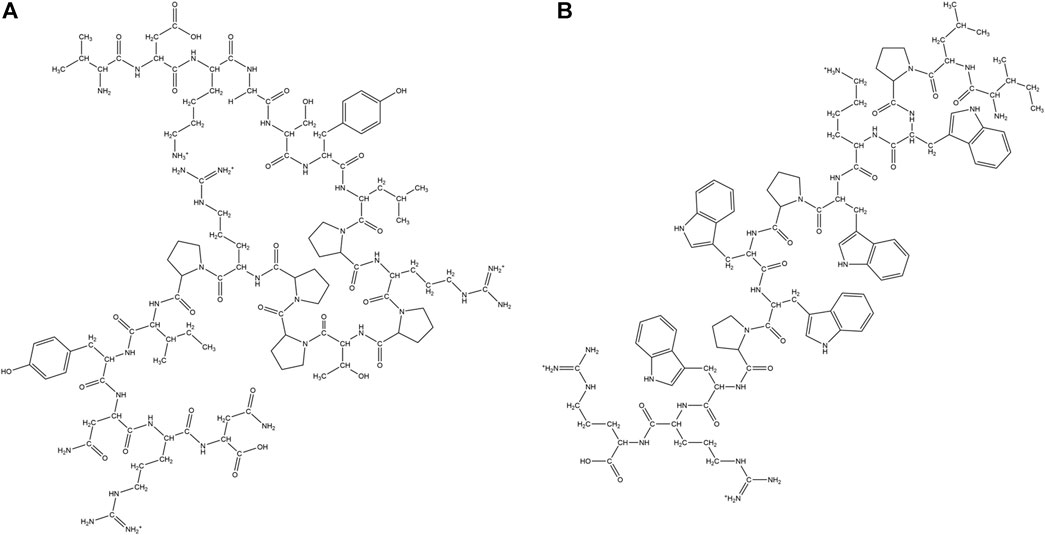
FIGURE 5. Examples of natural AMPs rich in Pro and Trp amino acid residues. (A) Pro rich AMP: Pyrrhocoricin. (B) Trp rich AMP: Indolicine.
Trp residues are found in high proportions in a sub-class of short peptides (TrAMPs) (Shagaghi et al., 2016). The Trp residue usually appears near the C terminal end of the peptides (Schibli et al., 2002). TrAMPs are also usually Arg rich. Most of the TrAMPs permeate bacterial membranes and acts on intracellular targets without disrupting membrane integrity. Only a very few like Indolicidin have membrane-disruptive activities. These peptides are active against different species of bacteria and fungi.
Lactoferricins are mammalian TrAMPs, obtained from the peptide mediated digestion of lactoferrins which exhibit antimicrobial (against bacteria, fungi and viruses) (Wakabayashi et al., 2003; Gifford et al., 2005) and anticancer activity (Eliassen et al., 2002). Lactoferricins are cationic, amphipathic in nature. While the bovine lactoferricin is 25 amino acid residue long and mostly forms β-sheets (Hunter et al., 2005), the human lactoferricin is 49 residues long and forms coiled coils (Gifford et al., 2005). Indolicidin is a 13 residue (ILPWKWPWWPWRR-NH2) cationic TrAMP isolated from bovine neutrophils (Figure 5A). Indolicidin has broad-spectrum activity against bacteria, fungi, protozoa and virus (Subbalakshmi and Sitaram, 1998). It is also active against MRSA, HIV virus (Robinson et al., 1998) and herpes simplex virus. The peptide directly inactivates the virus particles without being cytotoxic to the Vero cell line (Albiol Matanic and Castilla, 2004). Tritrpticin is another 13 amino acid residue long Tr-AMP that is derived from a precursor protein in the porcine bone marrow with high homology to the indolicidins. They have bactericidal and fungicidal activities against several clinical microorganisms (Lawyer et al., 1996).
Pro rich AMPs (Pr-AMPs) usually have conserved repeats of Pro and Arg. They do not adopt amphipathic structure but β chain type polyproline or cyclic helix structure instead, as in Nisin and PR-39. Pr-AMPs are cationic peptides expressed in arthropods as well as animals. They have low sequence homology but high structural homology of about 30% Pro content and several Pro-Arg-Pro motifs. They usually kill bacteria by non-lytic mechanisms. Pro rich peptides like Drosocin (Bulet et al., 1993), Pyrrhocoricin (Figure 5B) (Gagnon et al., 2016), Metalnikowin 1 (Gagnon et al., 2016) are known to inhibit protein biosynthesis by binding to the 70S ribosomal subunit, inhibiting heat shock proteins like DnaK and GroEL and binding to the lipopolysaccharides (LPS). In general Pro rich peptides enter into the cell through translocation of the inner membrane via a transporter mediated uptake mechanism by membrane protein SbmA (Runti et al., 2013). Several other Pr-AMPs like gonocin, apedaicin, drosocin are known to cross the blood brain barrier (Lalatsa et al., 2014; Stalmans et al., 2014). Apidaecins are the first class of Pr-AMPs that were isolated from honey bees. The mature peptide is linear, 19 residues long, heat stable and non-helical in nature. They are known to be active against a large number of plant associated bacteria and human pathogens through a bactericidal pathway rather than a lytic process (Casteels et al., 1989). Abaecin is a 32 amino acids long Pr-AMPs, isolated from honeybee Apis mellifera with strong antimicrobial and antifungal activity (Casteels et al., 1990). Lebocins are 32 amino acids long glycosylated Pr-AMPs isolated from silkworm Bombyx mori (Hara and Yamakawa, 1995; Liu et al., 2000). Lebocins have 41% sequence similarity with the baecin, a 34 residue antimicrobial peptide isolated from honeybee (Casteels et al., 1990). Drosocin is a post-translationally modified PrAMP isolated from Drosophila melanogaster (Bulet et al., 1993; McManus et al., 1999). Drosocin contains Pro-His repeats, along with a Threonine (Thr) residue and 5 N terminal residues critical for its activity (Bulet et al., 1993). Drosocin is active against E. coli and fungi (Imler and Bulet, 2005). Drosocin binds to bacterial DnaK, inhibiting cellular pathways (Zahn et al., 2013) and microbial ribosomes preventing protein translation (Florin et al., 2017). Pyrrhocoricin is a cyclic PrAMP isolated from Pyrrhocoris apterus (Cociancich et al., 1994; Rosengren et al., 2004) that binds and promotes ATPase activity in the heat shock protein DnaK (Kragol et al., 2001; Chesnokova et al., 2004). Closely related to drosocin and abaecin in sequence and function, this acts as a delivery vehicle in transducing peptides across cell membranes of the important human parasite pathogen Cryptosporidium parvum (Boxell et al., 2008). Metchnikowin, an immune inducible linear Pr-AMP peptide from D. megalogaster is selectively potent against gram-positive bacteria and fungi with no activity against gram-negative bacteria (Levashina et al., 1995). Metchnikowin interacts with fungal enzyme β (1,3)-glucanosyl transferase Gel1, that is involved in the fungal cell wall synthesis (Levashina et al., 1998). Diptericins (8 kDa) constitute a family of Pro and Gly rich AMP from Dipteran hemolymph proteins, which are about 82 amino acids long. Dipterin A is active against a few gram-negative bacteria like E. coli K12, Erwinia hericola T and Erwinia carotovora 113. This AMP acts on the cytoplasmic membrane of the growing bacteria (Keppi et al., 1989). Bacternecin is a mammalian PrAMP that is found in mammalian species like cow, goat and sheep. It is referred to as Bac 5 or 7 depending on the molecular weight of the mature peptides (Gennaro et al., 1989). Bac5 (43 amino acids) and Bac7 (60 amino acids) enter bacterial cells through the SmbA transporter and work on the intracellular targets (Gennaro et al., 2002; Runti et al., 2017). In pigs, Pr-AMP corresponding to the Bac 7 is called PR-39 after the number of constituent amino acids (Agerberth et al., 1991).
Human histatins are His rich AMPs. Histantins 1 and 3 are isolated from human saliva (Oppenheim et al., 1988). Histatin 5 is present in the secretions of human submandibular and parotid glands (Oppenheim et al., 1988). All the three histatins can kill pathogenic yeast C. albicans. Calcitermin C is a 15 residue AMP found in human airways and is effective against the gram-negative bacteria. It contains three His residues at the N terminus and a potential to adopt a helical conformation in the membranes (Cole et al., 2001).
Several Gly rich peptides have been isolated from the bacteria, plants, insects, spiders, nematodes, fish and amphibians KDAMP is a keratin derived Gly rich AMP that is present in the bactericidal lysate fractions of the human corneal epithelial cells (Tam et al., 2012). Attacins are Gly rich proteins (10–22%) first isolated from Hyalophora ceropia (Hultmark et al., 1983) and effective against gram-negative bacteria (Carlsson et al., 1991). Attacins are subdivided into subclasses A-F. Among these, Attacin A-D constitute the basic group while sub-classes E-F constitute acidic group of peptides. Attacins act via preventing the synthesis of the major outer membrane proteins, that disturb the integrity of the cell wall leading to formation of chains of bacteria (Carlsson et al., 1998). Attacins are mostly random coil in structure owing to the presence of high quantities of Gly residues. Persulcatusin is a Gly rich (20%) cationic AMP that is isolated from the mid-gut region of tick Ixodes persulcatus (Saito et al., 2009). This peptide adopts both α-helical and β-sheet structures that is stabilized by three disulfide bonds (Miyoshi et al., 2016, 2017). Persulcatusin can inhibit the growth of Methycilin sensitive S. aureus (MSSA) and Methycilin resistant S. aureus (MSRA) (Mylonakis et al., 2016) and multi drug resistant (MDR) S. aureus strains (Miyoshi et al., 2017). Ponericins are another family of Gly rich peptides isolated from the venom of the predatory ants. Ponericins are sub-divided into three classes depending on the primary sequence of the peptides. Ponericin G have sequence similarity with cecropin kind of peptides, ponericin W resembles melittin and ponericin L is similar with dermaceptins. They have α helical structures in cell membranes. Ponericins show hemolytic and insecticidal activities against cricket larvae.
Drawbacks of Natural Antimicrobial Peptides
In spite of a very large repertoire of natural AMPs identified, isolated and characterized from various classes of living organisms, there is only a limited success in the commercialization of these peptides. This is due to several drawbacks of the natural AMPs.
1) Long AMP sequences are economically and synthetically challenging for pharmaceutical scale synthesis.
2) AMP sequences often contain complex disulfide bridges and CCKs which are challenging to be synthetically mimicked.
3) Folding of synthetic AMPs into secondary structures (α-helical or β-sheet), critical for their activity, may be challenging, leading to their inactivity.
4) Many AMPs that are produced in the other organisms may show toxicity and high immunogenicity when administered in humans, making them therapeutically inappropriate.
5) Natural AMPs have short serum half-life due to their susceptibility to the protease degradation and physiological salt concentrations.
6) Site directed delivery of AMPs to the site of infection is challenging.
To overcome the shortcomings of the natural AMPs, there has been a surge of interest in generating synthetic AMPs. Several approaches have been adopted for the design of synthetic peptides as discussed below.
1) Template based synthesis: Involves de novo sequencing of the synthetic peptides based on natural AMP sequences to improve their activity, cytotoxicity, proteolytic stability and salt tolerance This method includes truncation of sequences, mutation of specific amino acid residues, enrichment of primary sequence with certain types of residues, altering of the charge, hydrophobicity, secondary structure of the designed peptides, fusion of different peptide sequences, incorporation of unnatural amino acid residues, D amino acid residues, peptidomimetic fragments, cyclization, lipidation etc. with an intension to improvise on the shortcomings of the template natural AMP sequence. This is the most convenient and commonly practiced way of synthetic AMP design.
2) Rigorous biophysical modelling: In this process, the prospective synthetic peptides are first modelled and are studied in hydrophobic membrane environments to have an atomistic idea of the designed peptides and their activities.
3) Virtual screening: Virtual screening of the library of existing peptides is done to identify potential AMP sequences. This screening may be based on certain conserved primary sequence elements or secondary structural elements.
Truncation of the sequences introduces economic viability and facility in synthesis without compromising the antimicrobial potency. Improvement in the antimicrobial potency is attempted by mutation of/enrichment with certain amino acid residues, increase of the net charge/hydrophobicity of the peptide, optimization of the hydrophilic/lipophilic balance etc. Protease resistance of the AMPs is achieved by introducing chemical modifications like cyclization, terminal protection (Jenssen, et al., 2006) and incorporation of D-amino acid residues (Vunnam et al., 1998; Chen et al., 2006; Jung et al., 2007) unnatural amino acid residues (Lu et al., 2020) or peptidimimetic fragments. For efficient in vitro delivery, nanostructured AMP conjugates are designed. Hydrogels (Borro et al., 2020) and nano-assemblies based on metal/metal oxide nanoparticles (Pal et al., 2019) are being explored recently as prospective methods of AMP delivery.
Synthetic Antimicrobial Peptides
In this section, we are going to describe some representative examples of synthetic AMPs which are cationic, cyclic and rich in amino acid residues like Trp, Pro, His and Gly (Table 2).
Synthetic Cationic Antimicrobial Peptides
As cationic AMPs are the most abundant type of AMPs in nature, a lot of synthetic designs are based on cationic sequences. Blazyk et al. designed linear cationic peptides to demonstrate that amphipathicity in secondary structure was more important than the kind of secondary structure. (KIGAKI)3 which formed amphipathic β-sheet was more potent than (KIAGKIA)3 which formed a non-amphipathic α-helix upon membrane binding (Blazyk et al., 2001). In a subsequent study, two families (α-helices and β-sheets) of synthetic linear cationic peptides with same charge and C terminal amidation were studied. Only peptides able to form highly amphipathic structure (α-helical/β-sheet like) exhibited significant antimicrobial activities (Jin et al., 2005).
Zhang et al. designed α-helical cationic AMPs with the variable number of Pro residues and demonstrated that Pro had a deactivating effect on the potency of the membranolytic cationic α-helical AMPs (Zhang et al., 1999). Jadavpour et al. designed amphipathic Lys rich 7-mer, 14-mer and 21-mer peptides. 14 and 21 mer peptides exhibited high inhibition against gram-negative E. coli and P. aeruginosa and gram-positive S. aureus. 7-mers were inactive and unstructured, 14-mers peptides had low cytotoxicity while 21-mer peptides showed high cytotoxicity except for (KLGKKLG)3 and (KAAKKAA)3 against human erythrocytes as well as mouse fibroblasts. 14-mer and 21-mer peptides adopted helical structures as a function of the length of the peptides and the length of the hydrophobic side chain residues. The propensity of the AMPs to form helical conformation was proportional to their cytotoxicity and inversely proportional to their activity (Javadpour et al., 1996). Pandit et al. designed a series of short cationic heptapeptides. Peptides P4: LKWLRRL-NH2, P5: LRWLRRL-NH2 had broad-spectrum antimicrobial activity against several members of ESKAPE pathogens (E. coli, P. aeruginosa, Klebsiella pneumoniae (K. pneumoniae), S.typhi, and S.aureus) and pathogenic fungi like C. albicans and C.grubii. P4 and P5 were non-hemolytic, non-cytotoxic and membranolytic in their mode of action (Pandit et al., 2018). In another study, Pandit et al. demonstrated that in a series of three 14 amino acid residue containing synthetic AMPs LL-14, VV-14 and ββ-14 having identical charge and sequence but differing in the hydrophobic amino acid residues, the length of the side-chain played an important role in the activity and the cytotoxicity of the peptides. Long hydrophobic side chain in Leu led to the best activity and highest cytotoxicity in LL-14 of all the three peptides. VV-14 was optimally active and non-cytotoxic (Pandit et al., 2021) (Figure 6A).While LL-14 and VV-14 formed α-helices in presence of membrane mimics, ββ-14 remained unstructured. In yet another study, a family of cationic, highly potent (against ESKAPE pathogens, C. albicans, Cryptococcus grubii (C. grubii)), non-cytotoxic undecapeptides were designed (Pandit et al., 2020). Huang et al. designed a series of short triblock amphiphilic peptides (KnFmKn) with high potency against both gram-positive (S. aureus) and gram-negative bacteria (E. coli) with low cytotoxicity towards mammalian cell lines (Huang et al., 2020).
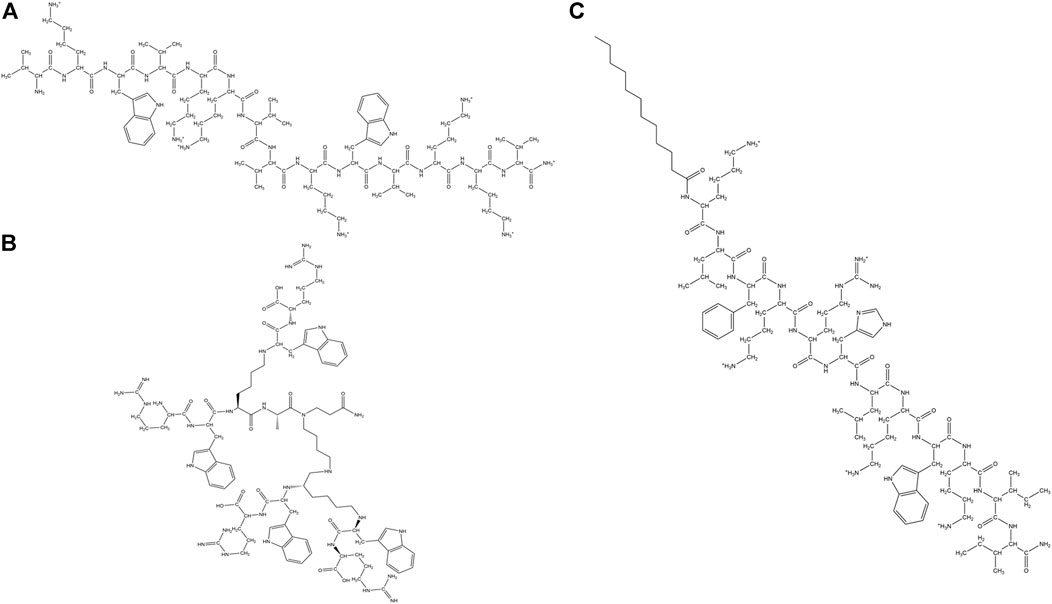
FIGURE 6. Examples of synthetic cationic AMPs. (A) VV-14 (Pandit et al., 2020). (B) Dendrimeric peptide: (RW)4D (Hou et al., 2009). (C) C12-SC4 (Chu-Kung et al., 2004).
Three cationic synthetic peptides JH8194(KRLFRRWQ), JH8195(KRLFRRLLFSMKKY) and JH8944(FKCKKVVISLRRY) were shown to have greater anticandidicidal effects than naturally occurring antifungals like Histantin 5 and Lactoferricin B (Nikawa et al., 2004). dF21-10K, the most potent AMP of the family of synthetic cationic peptides called kaxins could completely eradicate the preformed layer of biofilm formed by C. albicans and Candida tropicalis (C. tropicalis) (Burrows et al., 2006).
Dendrimers are tree like molecules that have radially distributed layers of branches called generations. They can form covalent or noncovalent assembly of multiple ligands and are known to manifest the dendrimeric effect or non-linear enhancement of certain properties like drug potency or bioavailability. Polylysine dendrons (PLL) are a class of dendrimers proposed by Tam et al. (Tam et al., 2002). Such molecules have an inner matrix constituted of several layers of Lys connected by the α and ε-amino groups. The amino groups of Lys that are located on the surface can bind to one or more peptides. Recently, PLL dendrons have been used as scaffolds for carrying 2 or 4 ten residue peptide (RGRKVVRRKK) which exhibited a very strong activity against P. aeruginosa and a very low activity against fungi (Lakshminarayanan et al., 2014). Divalent and tetravalent dendrons expressed non-linear enhancement of activity against three reference Candida strains and two clinical isolates of C. albicans. Additionally, dendrimeric AMPs retain their activity against high salt concentrations or in the presence of trypsin, blood serum and tear fluid. Small dendrimeric cationic lipopeptides acquired selective activity against Candida sp. and exhibited low hemolytic activities. These lipopeptides produced potassium leakage from fungal cells, caused morphological alterations of the fungal cells and inhibited activity of candidal β (1,3) glucan synthase (Janiszewska et al., 2012).
Kallenbach and coworkers designed a dendrimeric peptide (RW)4D based on the short cationic peptide sequence (Figure 6B). The peptide exhibited activity against multi drug resistant strains of S. aureus and E. coli D31 (resistant against ampicillin and streptomycin) and Actinobacter baumanni. Additionally, the peptide was shown to inhibit E. coli 437 in the planktonic and biofilm states. Most bacteria in the preformed biofilm lost their viability after being treated with this peptide (Hou et al., 2009).
N terminal of the Esculatin-1b, Esc (1–18) retained the antimicrobial property of the whole native peptide (Mangoni et al., 2003b). Similarly, N-terminal 21 amino acid residue peptide from Esculatin 1a Esc(1–21)NH2 inhibited the growth of Saccharomyces cerevisiae by modulating the synthesis of proteins that are involved in the metabolism of cell membranes (Gamberi et al., 2007). The activity of Esc (1–21)NH2 against P. aeruginosa was salt tolerant. This led to its administration to P. aeruginosa keratitis in the murine model that caused a significant reduction of the infection (Kolar et al., 2015). Esc(1–21)NH2 diastereomer Esc(1–21)-1C also exhibited activity against free-living and sessile forms of P. aeruginosa (Cappiello et al., 2016). Biondi et al. designed Esculatin (1–21)NH2 analogs with the incorporation of unnatural amino acid residue α-amino isobutyric acid for improving the activity of the parent peptide towards gram-positive bacterial strains. The designed analogs improved in helicity, activity against gram-positive bacterial strains with no induction of cytotoxic effects (Biondi et al., 2017).
Gopal et al. modified a previously reported peptide HPA3NT3 (FKRLKKLFKKIWNWK) by substituting Arg and Asparagine (Asn) with Lys and two Trps with Leu to synthesize analog FKKLKKLFKKILKLK-NH2 with an enhanced cationic character. Both the peptides were membranolytic and considerably active against several bacterial and fungal strains. The analog was more potent against gram-positive bacterial strains and less hemolytic (4.2%) compared to the parent peptide (71%) against hRBCs (Gopal et al., 2009).
Dinh et al. synthesized a doubly stapled peptide Ac-DS-14W based on Alanine (Ala)/Lys model sequence Ac-KXAKAXKKXAKAXWK-NH2. This peptide was designed to have two oct-4-enyl staples, which cross-linked residues at positions 2 and 6 and positions 9 and 13, respectively. For comparison, two more analogs of Ac-DS-14W, one singly stapled Ac-SS-14W and the other unstapled Ac-UM-14W were synthesized. Helical content of the peptides increased with the number of staplings in the peptides. Unstapled peptide was found to be inactive against all the tested microbes. Antimicrobial activity of the peptides increased with the number of staples, though doubly stapled peptides were found to have less activity against gram-negative bacteria K. pneumonia. Proteolytic resistance as well as cytotoxicity of the peptides increased with the number of staples in the peptides (Dinh et al., 2015).
Castelletto et al. designed a surfactant-like peptide with a single Arg residue Ala6-Arg (A6R) in which both the termini were capped/uncapped. Cap-A6R had a specific activity towards gram-positive Listeria monocytogenes while A6R was active against all the gram-positive and negative bacterial strains. The difference in activity was attributed to the selective interaction of the peptides with the membrane mimics. (Castelletto et al., 2018).
Dimeric temporin analog (TAd), all L modified temporin analog (TAL-L512) and all D temporin analog (TAD-L512) were synthesized. All the peptides showed antibacterial activity towards S. aureus. However, other than TAL-L512 all the other analogs were cytotoxic towards human keratinocytes (Mantyla et al., 2005). A synthetic analog of melittin named MeIP5, was shown to have very advanced pore forming ability in comparison to the parent peptide. The design involved the change of four positively charged amino acid residues in the nonpolar face of the amphipathic α-helix structure of melittin to hydrophobic amino acid residues (Wiedman et al., 2014). Reyes et al. studied the effect of trimethylation of the ε-amino groups of Lys at the selected positions of the antimicrobial hybrid peptide cecropin A-melittin KWKLFKKIGAVLKVL. Increase in Lys-(Me)3 residues, led to a decrease in both the antimicrobial activity against Leishmania, gram-positive bacteria like S. aureus and gram-negative bacteria like Acinetobactor baumannii. Membrane permeabilization and depolarization were the mechanism of action of the AMPs. The proteolytic stability of the peptides increased with the number of trimethylated Lys (Fernandez-Reyes et al., 2010). Svenson et al. designed a library of tripeptides by employing Biphenylalanine (Bip) as the central residue flanked by Arg/Arg-like amino acids. Unnatural amino acids like phenylalanine (Phe) derivatives L-2-amino-3-(4-aminophenyl)- propanoic acid (App) and L-2-amino-3-(4-guanidinophenyl) propanoic acid (Gpp) were included to establish the influence of hydrophobic bulk in direct conjunction with the cationic charge on the antibacterial property of the peptides. Tripeptides containing two Gpp residues were most active followed by those containing one Arg and another Gpp residue. All the peptides showed low haemolytic activity (Svenson et al., 2009). Hicks et al. used multiple repeats of two unnatural amino acid residues Tetrahydroisoquinolinecarboxylic acid (Tic) and Octahydroindolecarboxylic acid (Oic), spaced by two amino acid residues for constructing amphipathic α-helical AMPs. The peptides showed broad-spectrum antimicrobial activities against ESKAPE pathogens and haemolytic activities. Few of these designed AMPs showed activity in the in vivo mouse wound healing model (Hicks et al., 2007, 2013). Kang et al. designed analogs of AMP Cbf-14 by the mutation of Lys and Leu residues with unnatural amino acid analogs Ornithine (Orn) and Nor-Leucine (Nle) respectively. An all D-amino acid residue containing analog was also developed. All the peptides had similar or better activity in comparison to Cbf-14 and negligible hemolysis in sheep RBCs. Peptides adopted helical conformation in the presence of membrane mimetic environments. One of the AMPs, Cbf-14–2 (Figure 7A) exhibited in vitro as well as in vivo activity against penicillin resistant bacteria, while retaining low toxicity against mammalian cells (Kang et al., 2017).
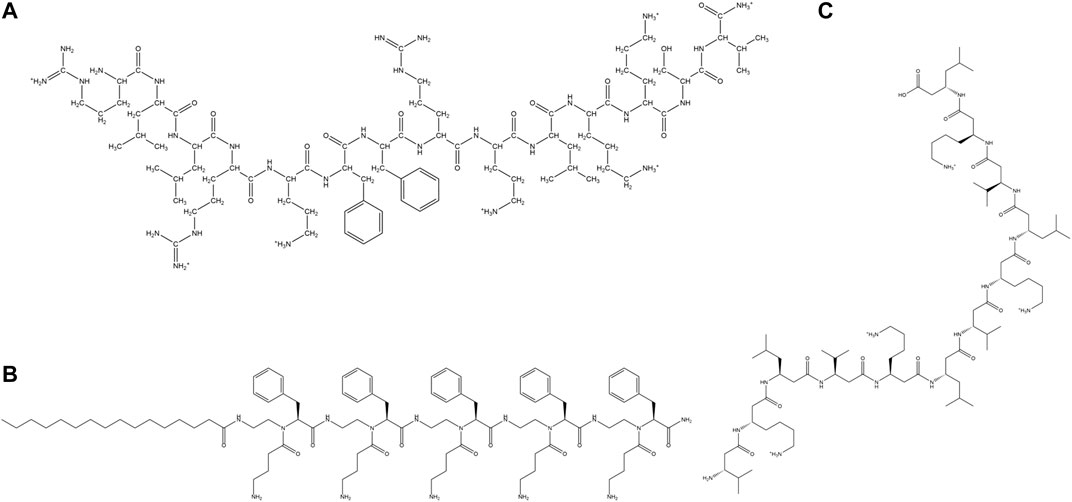
FIGURE 7. Examples of synthetic cationic AMPs containing unnatural amino acid residues. (A) Cbf-14–2 (Kang et al., 2017). (B) NB-119–2 (Hu et al., 2012). (C) β-amino acid containing peptide H-(β3-H-Val- β3-H-Lys- β3-H-Leu)4-OH (Liu and De Grado, 2001).
Short lipopeptides were synthesized from tethering cationic tetrapeptide composed of one D-Phe, two L-2,4 diamino butyric acid and a L-Phe residue to fatty acids of different chain lengths. All the peptides were non-hemolytic and showed high activity towards gram-positive bacteria. (Juhaniewicz-Dębinśka et al., 2020). Chu-Kung et al. designed lauric acid derivates of three peptides AKK, SC4, and KAK. Lipidated AKK and SC4 showed improved antimicrobial potency in comparison to the non-lipidated analogs, in contrast to lipidated KAK, owing to the increased helical content of lipidated AKK with respect to KAK in the presence of microbial membrane mimetics. The cytotoxicity of lipidated AKK and KAK peptides was low in comparison to SC4 due to the ability of the later to indiscriminately adopt helical conformation in microbial and mammalian zwitterionic membrane mimic. This study concluded that lipidation could alter the antimicrobial potency and cytotoxicity of the peptides by altering the secondary structure of the peptides (Chu-Kung et al., 2004). (Figure 6C) Hu et al. synthesized several short cationic lipidated α-AA-peptides containing unnatural N-acylated-N-aminoethyl amino acid residues with broad-spectrum antimicrobial properties against clinically relevant bacterial and fungal strains. NB-123, NB-119–2 (Figure 7B), and NB-119–3 peptides exhibited better antimicrobial activity and selectivity in comparison to the clinical therapeutic pexiganan. The peptides were membranolytic in their activity which helped overcome the problem of the development of resistance against them (Hu et al., 2012). Short (RW)3 sequences with side chain lipidated terminal Lys residues had broad-spectrum antimicrobial activity against gram-positive and gram-negative bacteria. Both N and C-terminally lipidated peptides showed high activity against a broad range of pathogens including P. aeruginosa and A. baumannii, though the C terminal peptide had higher hemolytic activity (Albadaet al., 2012). Zhong et al. designed fatty acid derivatives of AMP Anoplin (GLLKRIKTLL-NH2) and its D isomer. The fatty acid chains (4–16 carbon atoms long) were added to the side chains of the D-amino acid residues. The peptides showed excellent activity against a range of bacteria, especially the multidrug resistant ones, resistance to trypsin, serum, salts, pH environments and low tendency to develop antibacterial resistance. The lipidated AMPs showed membrane activity (outer/inner membrane permeability, membrane depolarization, deformation) in addition to possessing intracellular targets. Some of the peptides possessed in vivo antimicrobial potency, anti-inflammatory activity and endotoxin neutralization ability (Zhong et al., 2020). Niu et al. synthesized a series of cationic lipo-γ-AA peptides with unsaturated lipid chains tethered to peptides composed of unnatural amino acid residues with positive side chains. The lipo-γ-AA peptides showed high broad-spectrum antimicrobial activities, low hemolytic activity and low resistance development abilities (Niu et al., 2012). Karlsson et al. designed cationic amphipathic 14-helical β-peptides formed from β amino acid residues with potent antifungal activity against C. albicans. Decamer β3Tyr-(Aminocyclohexane carboxylic acid (ACHC)-β3Valine (Val)- β3Lys)3 exhibited high potency and a moderate degree of hemolysis (Karlsson et al., 2006). Degrado and coworkers designed helical membranolytic peptides H-(β3-HAla-β3-HLys-β3-HVal)n-NH2 (where n = 4, 5) (Figure 7C) with high antimicrobial but low hemolytic potencies (Liu and DeGrado, 2001). AMPs containing E-vinylogous γ-amino acids in cationic (αγ)2 lipopeptides showed broad-spectrum antimicrobial property against several bacterial and fungal strains, when the side chains of the γ-amino acids were that of Ala, tyrosine (Tyr) and Phe. Self-assembled nanostructures played an important role in the activity and the hemolytic activity of the peptides (Shankar et al., 2013).
Synthetic Cyclic Peptides:
Cyclic peptides are found to have a superior affinity to the target receptors as compared to that of the linear form. Firstly, cyclization improves the proteolytic susceptibility (Joo, 2012) of linear AMPs. Cyclization of peptides removes the reactive C and N-termini and acts as shielding components against the proteolytic enzymes. Secondly, cyclization improves binding the affinity of the AMPs for the microbial membranes. The flexibility of the linear AMPs, lead to an entropic penalty upon membrane binding. Cyclic AMPs with rigid structures do not incur entropic penalty upon membrane binding, which leads to its higher membrane binding affinity and hence activity. Figure 8 and Table 2 depicts some representative synthetic cyclic peptides.
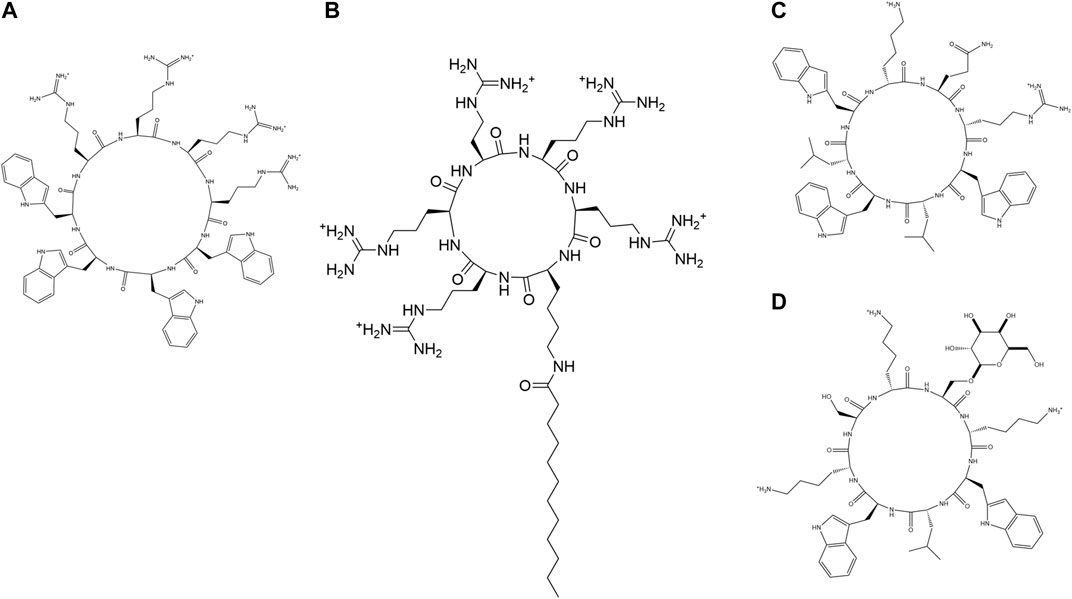
FIGURE 8. Examples of synthetic cyclic AMPs. (A) (R4W4) (Oh et al., 2014). (B) C12-R5 (Oh et al., 2014). (C) (kQrWlWlW) (Fernandez-Lopez et al., 2001). (D) (WlWkUkSk), U = Ser(α-Man) (Motiei et al., 2009).
Synthetic cyclic peptide (Gly)6ccTP, an analog of the β-stranded AMP tachyplesin, showed salt tolerant activity against several microbial strains. (Gly)6ccTP contained eight mutated Gly residues instead of hydrophobic counterparts in the parent AMP and retained all the three disulfide bridges in the cyclic peptide backbone (Tam et al., 2000). Oh et al. studied two classes of amphiphilic cyclic cell-penetrating peptides (CPPs): 1) cyclic peptides containing Trp and Arg amino acid residues and 2) fatty acylated cyclic polyarginine peptides. Cyclic (R4W4) peptide had an enhanced activity in comparison to the linear R4W4 towards MRSA (Figure 8A). Antimicrobial potency of cyclo (R4W4) (2XMIC) with tetracyclin demonstrated was enhanced by 4-8 folds against MRSA and 2-8 folds against E. Coli with respect to the antibiotic alone. Among fatty acylated cyclic peptides, dodecanoyl (Figure 8B) and hexadecanoyl KR5 and dodecanoyl KR6 exhibited the highest potency against MRSA. The antimicrobial potency of the peptides was in the same order as their cell-penetrating abilities (Oh et al., 2014). In another study, cyclic AMPs with 6–12 amino residues were synthesized keeping in mind Ghadiri’s alternate D, L sequence design (Figure 8A) (Bong et al., 2001; Fernandez-lopez et al., 2001; Dartois et al., 2005; Fletcher et al., 2007) and cyclized via a xylene double thioether bridge connecting a pair of cys residues placed at both ends of a linear sequence to promote aggregation and serum stability (Timmerman et al., 2005). RH11 had good activity against P. aeruginosa including MDR clinical isolates, good serum stability and moderate hemolysis. These peptides were membranolytic in action (He et al., 2017).
Nanotubes formed from cyclic peptides are another important class of antimicrobials. These nanotubes are stable against proteolytic degradation and easily formed on the charged membranes of bacteria. Unique supramolecular arrangements, made development of bacterial resistance against them. Ghadhiri et al. were the first to assemble cyclic peptide nanotubes by acidification of a pH-responsive octapeptide (Ghadiri et al., 1993). Cyclic peptide assemblies from cyclic gamma or chiral β3 amino acid residues were much more stable owing to rigid backbone of the cyclic γ amino acid residues in comparison to the α amino acid residues. Antimicrobial activity of the CNPT against microbes is based on several factors like ring size, nature of constituent amino acid residues, nature and number of positively charged amino acid residues (Chapman et al., 2012). Fernandez-Lopez et al. demonstrated that the antimicrobial activity of the cyclic peptides was way enhanced than their linear counterparts. Cyclic 8-mer AMPs showed better activity than the 6-mers. Antimicrobial and haemolytic activity remained the same on changing the chirality of the basic amino acids (Fernandez-Lopez et al., 2001) (Figure 8C). Motiei et al. studied membrane activity as well as in vitro activity of cationic cyclic D, L-α-glycopeptides bearing D-glucosamine (GlcNH2), D-galactose (Gal), or D-mannose (Man) glycosyl side chains. A membrane active amphiphilic parent peptide cyclic D, L-α-(WLWKSKSK) was modified to glycopeptides of the same charge but containing glycosyl side chains (Figure 8D). Glycosylation improved the activity against gram-positive bacterial strains including MSRA. The glycosylated peptides were protease resistant, had membrane permeation mode of action and cytotoxicity decided by the position and type of glycosyl residues (Motiei et al., 2009).
Tryptophan, Proline, Histidine, Glycine Rich Synthetic Antimicrobial Peptides
Learning from nature, several synthetic peptides have been developed which are rich in certain amino acid residues like Trp, Pro, His and Gly (Figure 9; Table 2). Due to its unique characteristics, several Trp rich short peptide antibiotics have been designed. A minimum of two Trp residues with a C terminal amidation was essential for the antimicrobial property. Additionally, about six amino acid residues were required for attaining maximum antimicrobial activity against gram-negative bacteria like E. coli or gram-positive bacteria like S. aureus. Increasing the number of Trp residues beyond six, marginally increased the activity while decreasing the number of Trp residues in hexa/heptapetides by mutation with Tyr residue led to significant inactivation of the peptide, especially against gram-negative bacterial strains.
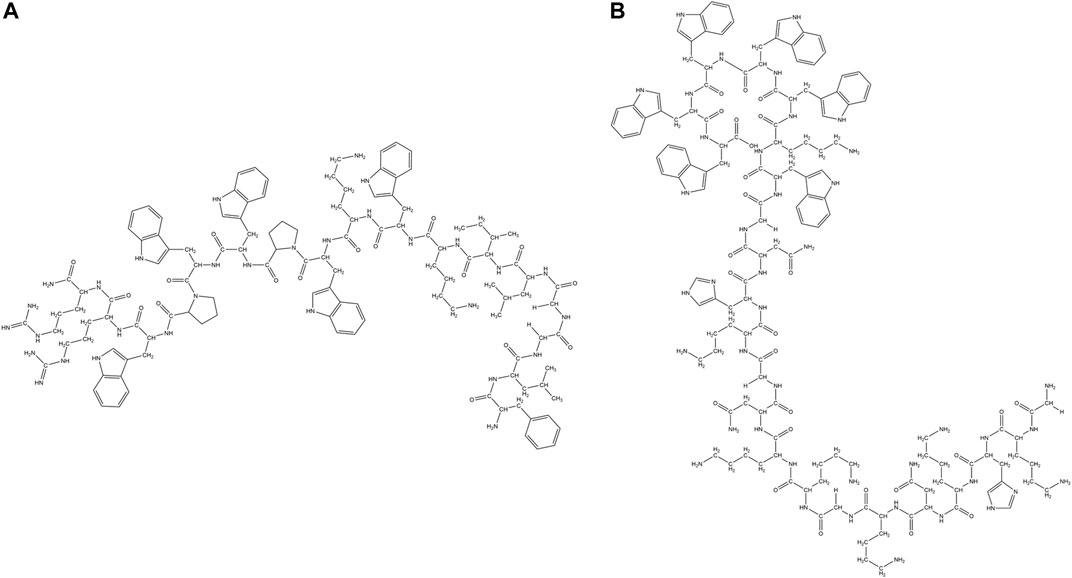
FIGURE 9. Examples of synthetic AMPs rich in specific amino acid residues (Trp, Pro, His and Gly). (A) Trp-Pro rich peptide: RN7-IN10 (Jindal et al., 2017). (B) Trp-Gly rich peptide: GKH17WWWWW (Pasupuleti et al., 2009).
PAF26 (Acetyl-RKKWFW-NH2), a Trp rich antifungal hexapeptide was obtained from combinatorial chemistry, that is active against Penicillium italicum, Penicillium digitatum and Botrytis cinerea (Muñoz et al., 2006; Lopez-García et al., 2000). Cyclic analog of the PAF26 was found to be active against gram-positive bacteria like S. aureus and S. sanguis and gram-negative bacteria like E. coli. It is also found to be active against pathogenic fungi like C. albicans (Blondelle et al., 1996). LfcinB4-9, RRWQWR was identified as the active antibacterial component of the LfcinB (Tomita et al., 1994). Position of the Trp residues was as/more important than the number of Trp residues. Synthetic peptides KCM11(TWWRWW-NH2), KCM12(KWRWIW-NH2), KCM21(KWWWRW-NH2) and KCM22(WRWFIH-NH2) were identified from the positional screening of the synthetic peptide library and were shown to have activity against phytopathogenic bacteria Pectobacterium spp., Xanthomonas spp., and Pseudomonas spp. (Choi and Moon, 2009). LysH and Lys C were two cationic Trp rich peptides containing 7 and 15 amino acid residues respectively which were designed from the C terminal end of the human and egg while lysozyme. Both the peptides showed broad-spectrum antibacterial activity against gram-positive bacterial strains like B. subtilis, S. aureus, Streptococcus zooepidemicus and several gram-negative bacterial strains like E. coli, K. pneumonia, P. aeruginosa, Serratia marcescens (Pellegrini et al., 1997). A rationally designed synthetic hexapeptide (RW)3 was highly active against bacterial strains like E. coli and S. aureus (Liu et al., 2007) and pathogenic fungal strains like Fusarium solani and Fusarium oxysporum (F. oxysporum) (Gopal et al., 2012). Grain softness protein (MPLSWFFPRTWGKR-NH2) derived from the wheat grain (Phillips et al., 2011) exhibited antibacterial (E. coli, S. aureus) and antifungal activities (Colletotrichum graminicola, R. solani). GAK4AL, exhibited antibiotic activities against gram-negative bacterial strains like E. coli, K. pneumonia, Shigella dysenteriae, Salmonella typhimurium (S. typhimurium) and gram-positive bacterial strains like S. aureus, Staphylococcus epidermidis (S. epidermidis), P. aeruginosa, B. subtilis, Micrococcus luteus (M. luteus) (Won et al., 2011). PW2, a synthetic peptide isolated from the phage display library (HPLKQYWWRPSI) exhibited antifungal activity against Eimeriatenella, Eimeria acervulina, C. albicans, A. nidulans (Aspergillus nidulans) (Da silva et al., 2002).A synthetic linear and cyclic analog of the Indolicidin peptide CP-11(ILKKWPWWPWRRK-NH2) and cycloCP-11, with an increased positive charge and amphipathicity was shown to have enhanced activity against gram-negative bacteria. Both the peptides were found to have similar kind of structure and binding to membrane mimics, thus resulting in similar activity. However, the cyclic analog had much enhanced resistance to protease degradation in comparison to the linear analog (Rozek et al., 2003). The carboxymethylated derivative CP-11C exhibited improved activity against gram-negative bacteria and C. albicans, while it retained activity against Staphylococci in comparison to Indolicidin (Falla and Hancock, 1997). Hybrid peptides RN7-IN10 (Figure 9A), RN7-IN9, RN7- IN8, and RN7-IN6, based on two natural AMPs Indolicidin and Ranalexin, were shown to have broad-spectrum antimicrobial activity against S. aureus, MRSA, and E. coli. The hybrid AMPs were non-cytotoxic and non-hemolytic, and exhibited potent antibacterial activity against 30 different pneumococcal clinical isolates. Hybrid AMPs were more potent against K. Pneumoniae than standard drugs like erythromycin and ceftriaxone (Jindal et al., 2015). A truncated 13 residue synthetic fragment (Puro A), containing the Trp rich domain of Puroindoline A was shown to exhibit native-like antimicrobial property and hence considered to be responsible for the bactericidal activity of Puroindoline A (Jing et al., 2003). Pasupuleti et al. designed two peptides GKHKNKGKKNGKHNGWK and KNKGKKNGKH from kininogen and added various length of Trp and Phe tags (Figure 9B). The antimicrobial potency of the AMPs increased with the length of the tags, which was related to the efficacy of lysis of the bacterial walls and to more pronounced bacterial lipopolysaccharide binding. The end tagged analogs were protease, salt, serum, and anionic macromolecular scavenger tolerant (Pasupuleti et al., 2009). Lee et al. designed a series of peptides based on the basic sequence (XWZX)n (n = 2–4, X: Lys or Arg, Z: Leu, Tyr, Val, or Gly). All the peptides showed low cytotoxicity and high antimicrobial properties against drug-susceptible and drug-resistant strains through membranolytic mechanisms (Lee et al., 2018). N terminal modified metallocenoyl {ferrocenoyl [FcC(O)-] or ruthenocenoyl [RcC(O)-]} derivatives of all L and diastereomeric WRWRW-NH2 were synthesized. Mostly all the derivatives were active against gram-positive bacterial strains like S. aureus, MRSA and B. subtilis. Most of the peptides were inactive against gram-negative strains and had limited hemolytic activity. Diasteromeric peptides had better activity than the all L peptides while FcC(O)- derivatives were more potent than the corresponding RcC(O)- derivatives (Albada et al., 2014). Four ultrashort Arg and Trp based peptides RW, WR, WRW and RWR were synthesized and covalently bound to two different trivalent scaffold molecules namely 1,3,5-tris(azidomethyl)- benzene and a 1,3,5-tris(azidomethyl)-2,4,6-triethylbenzene using the copper(I)-catalyzed alkyne–azide cycloaddition (CuAAC) reaction (Figure 9C) to check the effect of preorganization of the AMPs on their antibacterial property. The AMPs were more active against the gram-positive compared to the gram-negative bacteria. Comparison of the activity with the Linear (RW)3-NH2 suggests that the presence of certain number of amino acids in the close vicinity is more important than their relative spatial orientation (Hoffknecht et al., 2015). High antimicrobial property was obtained by increasing the hydrophobic volume of the side chains of the amino acid residues. Hydrophobic chains such as Bip and 2.5.7 tri-tert-butyl Trp (Tbt) generated the most potent peptides when associated with either C terminal benzyl esters or amides (Hang et al., 2004). These peptides had much higher selectivity against gram-positive bacteria in comparison to the gram-negative bacteria. Applying Tbt amino acid residue instead of Trp in the RXR motif, highly active AMPs were obtained. An AMP RWRWRWA-(Bpa), with a photoactive group p-benzoyl-phenylalanine (Bpa) at the C- terminus was attached to a reverse osmosis (RO) membrane surface, upon UV radiation. Bacterial viability on the modified surfaces decreased by 55% with reduction of biofilm caused by P. aeruginosa. The viability of gram-positive bacteria E. faecalis on the peptide coated surface exhibited 29–40% bacterial inhibition (Mao et al., 2018). Hybrid Trp rich cationic peptides KU2 and KU3, generated from the fusion of two peptides KABT-AMP and Uperin 3.6 showed high antifungal potency against C. albicans. Though the peptides were non-hemolytic, they showed significant toxicity against human epithelial cell lines (Lum et al., 2015).
Synthetic Pr-AMP PR-26, derived from the N terminus of the PR-39 exhibited higher activity towards enteric gram-negative bacteria. Pr-26 potentiated neutrophil phagocytosis of Salmonella choleraesuis and decreased the invasion of S. typhimurium into the intestinal epithelial cells. Pr-26 facilitated the pore forming AMPs like magainin A, thus potentiating the innate host defense against the gram-negative bacterial infections (Shi et al., 1996). Synthetic analogs of Pr-AMP Bac5, namely Bac(1–25) and Bac(1–31) exhibited excellent activity towards E. coli, while Bac(1–15) was inactive. Bac(1–25) and Bac(1–31) were shown to prevent protein synthesis in vitro and in vivo. PMAP-23 amphipathic α-helical peptide isolated from porcine was modified by incorporation of Trp residues at positions 10, 13 and 14 of the hydrophobic face of the peptide to generate improved antifungal activities. This increased the fungicidal and antitumor activities of the peptide (Lee et al., 2002).
The His and Gly rich synthetic homodimer di-K19Hc designed from domain 1 of AMP Halocidin, was salt-tolerant and active against bacteria, yeast and filamentous fungi, such as C. albicans, C. neoformans (Cryotococcus neoformans), A. niger (Aspergillus niger), A. terreus (Aspergillus terreus) and F. oxysporum (Jang et al., 2006). One reported method to increase the salt tolerance of the synthetic AMPs was to replace the amino acid residues like His and Trp with bulky residues like β-naphthylalanine and β-(4,40 -Bip (Yu et al., 2011). However, these substitutions induced cytotoxicity and hemolytic activities in the peptides. Pep6 (FRLKFH), obtained from combinatorial chemistry, is a hexapeptide rich in Phe and His residues that is active against several plant pathogens like F. oxysporum f. sp. lycopersici, Rhizoctonia solani, Ceratocystis fagacearum and Pythium ultimum (Reed et al., 1997).
N terminal tethering of fatty acids (palmitic/undecanoic) to the inactive diastereomers of Gly rich magainin (D4-magainin) or to very weakly active diastereomeric lytic peptide D-K5L7 conferred antifungal activity against Cryptococcus neoformans. Particularly D-K5L7-UA showed potency against all the different microbes like bacteria, fungi and yeast (Avrahami and Shai, 2003). AMP derivatives were synthesized by simultaneous introduction of positive charges as well as Pro residues in a typical antimicrobial peptide F5W-magainin 2 (MG2). The former and the later contributed to the increase in the antimicrobial activity and the reduction in the cytotoxicity of the peptides respectively, increasing the overall therapeutic index of the peptides. Results were sensitive to the position of the Pro substitution. The antimicrobial mechanism involved Lipopolysaccharide binding, membrane permeabilization, and DNA (Azuma et al., 2020).
AMP Delivery Vehicles
Another major concern in the commercialization of the AMPs as therapeutics arises from their modes of administration/delivery. It is now an accepted fact that the efficacy of any drug depends on its mode of delivery. In these lines, nanostructure based drug delivery systems have been found to be particularly promising. The nanostructured AMPs are more potent as they enhance the bio-availability of the drug, facilitate sustained release and improve retention of the AMPs in the bloodstream due to their enhanced retention and permeability effect. Poorer renal clearance of nanostructured AMPs leads to full exploitation of the materials in the blood stream due to enhanced retention and permeability effect (EPR effect). Nanoscale sized delivery vehicles improve the half-life and the stability of the AMPs. Table 3 summarizes some nanostructured AMPs.
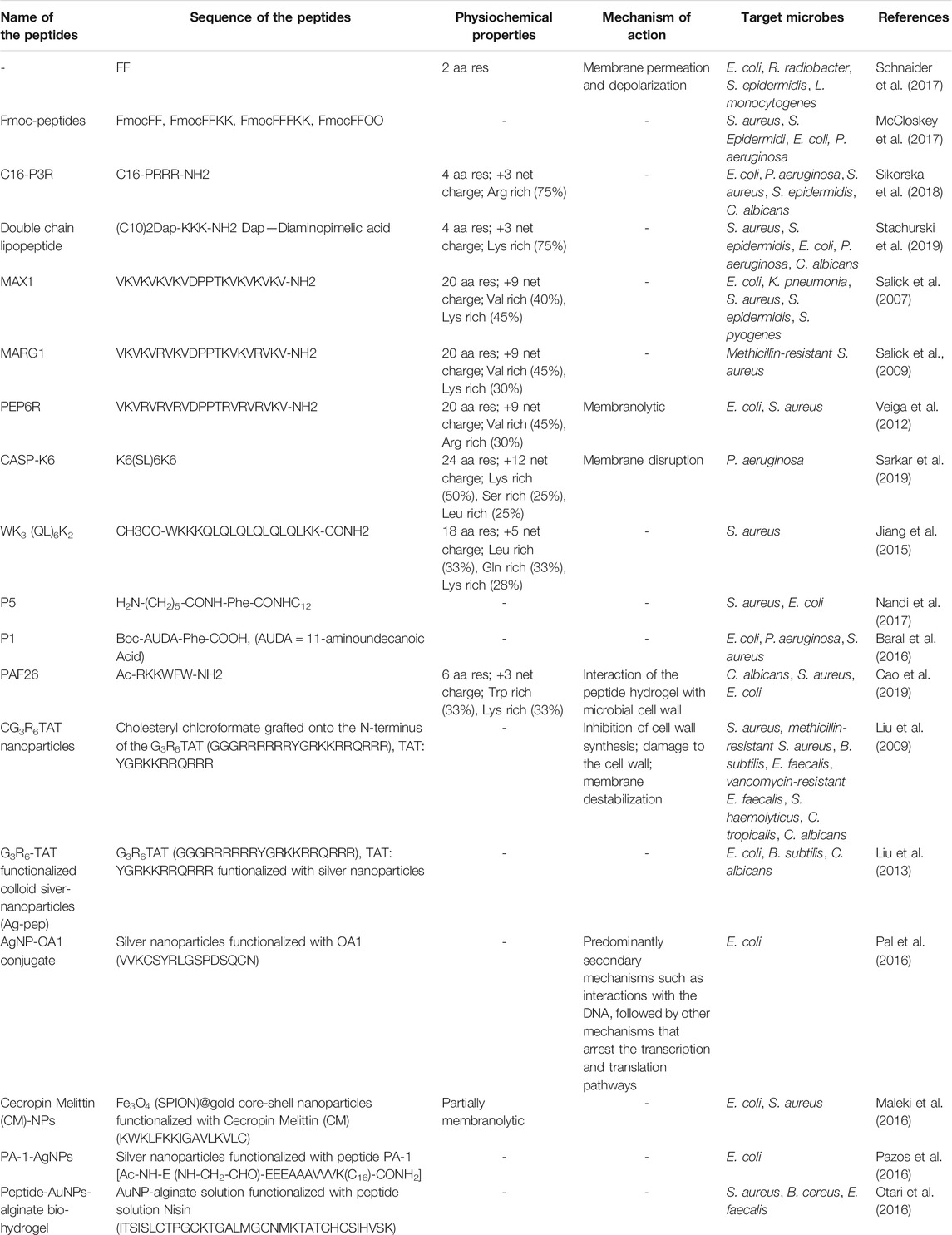
TABLE 3. Antimicrobial peptides: delivery vehicles, peptide hydrogels and peptide-nanoparticle conjugates.
Certain small AMPs have the ability to self-assemble and form hydrogel (Figure 10). In the literature, there are reports of ultrashort AMPs (McCloskey et al., 2017; Schnaider et al., 2017), AMPs containing lipid chains (Sikorska et al., 2018; Stachurski et al., 2019) and non-natural amino acids (Melchionna et al., 2016) which have the ability to form macroscopic hydrogels. Hydrogels impregnated with the AMPs act as a protective shield and prevent the enzymatic, thermal and chemical degradation of the AMPs (Moorcroft et al., 2020). These hydrogels may be constituted by synthetic polymers (Osada and Kajiwara, 2001), natural polymers like collagen, silk, or peptides (Jonker et al., 2012).
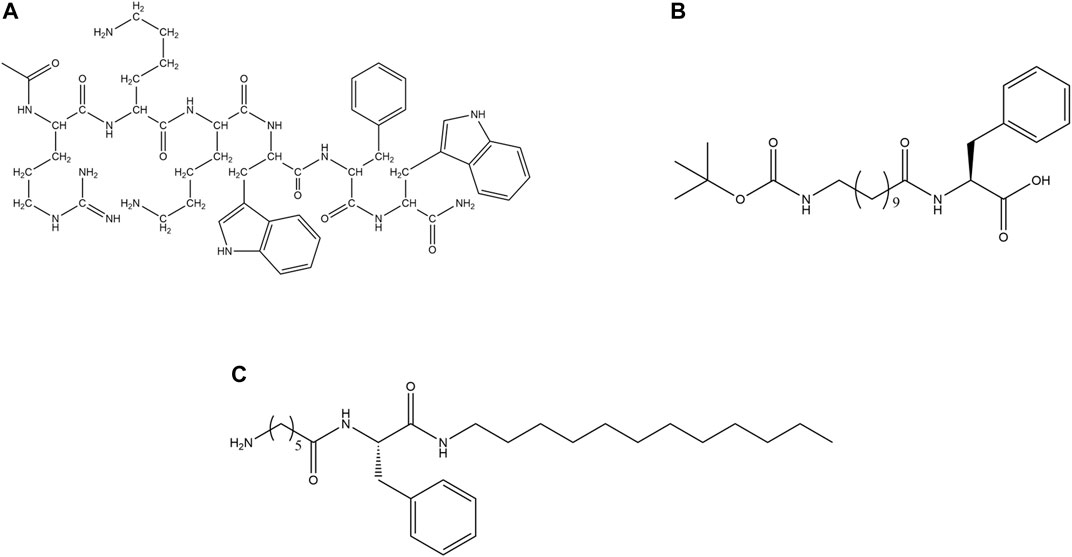
FIGURE 10. AMPs forming hydrogels. (A) PAF26 (Cao et al., 2019). (B) Boc-AUDA-Phe-COOH (P1), (AUDA = 11-aminoundecanoic acid) (Baral et al., 2016). (C) H2N-(CH2)5-CONH-Phe-CONH-C12 (P5) (Nandi et al., 2017).
MAXI, a twenty residue peptide, having two eight residue strands of alternating Val and Lys amino acids connected by a tetrapeptide sequence (-VDPPT-), could form a hydrogel composed of a network of interconnected fibrils rich in β-sheet, in DEMN cell culture at pH 7.4. The fibrils were composed of bilayers of intermolecularly H-bonded hairpins. This rigid hydrogel with β-hairpin conformation could kill both gram-positive and gram-negative bacteria through membranolytic mechanism of action (Salick et al., 2007) In a separate study, Salick et al. designed a 20 residue amphiphilic β-hairpin peptide MARGI, which self-assembled into a fibrillar network resulting in a rigid hydrogel. This peptide contained two Arg groups at the 6th and 17th positions. The enhanced activity of MARGI over MAX1 against MRSA suggested that the inclusion of Arg might be critical for the enhanced action (Salick et al., 2009). Veiga et al. reported an optimized peptide containing six Arg residues, PEP6R from PEP8R, which folded into an amphiphilic β-hairpin conformation in buffer solution, rapidly self-assembling into a β-sheet rich fibrillar network, forming a hydrogel. The hydrogel exhibited distinct antibacterial efficiency toward E. coli and S. aureus via membranolytic mechanism. Also, the hydrogel was found to have a shear-thinning property and, thus could be used as a potential injectable material (Veiga et al., 2012).
CASP-K6, a cationic self-assembling AMP formed nano-fibrous hydrogel with membrane disrupting antimicrobial activity against P. aeruginosa colonies. CASP-K6 was a proteolytically stable and injectable hydrogel (Sarkar et al., 2019). Jiang et al. demonstrated that the antimicrobial activity of the hydrogels was related to their surface and rheological properties (Jiang et al., 2015).
AMP PAF26 formed hydrogel (Figure 10A) that exhibited antimicrobial activity against C. albicans, S. aureus, and E. coli through a membrane disruptive mechanism of action (Cao et al., 2019). Co-assembled hydrogels obtained from Fmoc-Phe and Fmoc -Leu amino acid derivatives showed activity against gram-positive bacteria through membrane disruptive process. Baral et al. reported a proteolytically stable N-Boc protected dipeptide (P1: Boc-AUDA-Phe-COOH) (Figure 10B) based injectable hydrogel, which showed excellent antibacterial activity against gram-negative bacteria (E. coli and P. aeruginosa), and biocompatibility with human red blood cells and human fibroblast cells (Baral et al., 2016). A series of five different peptide amphiphiles with a long fatty acyl chain of general chemical formula [H2N-(CH2)nCONH-Phe-CONHC12 (n = 1-5, C12 = dodecylamine)] (Figure 10C) formed hydrogels at pH 6.6. Hydrogels with longer alkyl chain lengths were found to have higher membrane affinity and antibacterial activity against both gram-positive (B. subtilis and S. aureus) and gram-negative bacteria (E. coli). These hydrogels induced a small amount of hemolysis at their MIC for B. subtilis and E. coli and exhibited proteolytic resistance to enzymes proteinase K and chymotrypsin (Nandi et al., 2017).
Antimicrobial efficacy of AMP-NP systems depends on the rate, extent and the method of controlled release of the antimicrobials. Gold nanoparticle (Au-NP) based micro/nano structures are one of the most commonly used drug delivery systems owing to their biocompatibility with the mammalian systems due to their chemical inertness and stability (Rajchakit and Sarojoni, 2017). Gold nanoclusters (Au-NC) retain the biocompatibility of the AU-NP with an additional luminescent property that helps in bioimaging purposes. Antimicrobial activity of Au-NCs occur due to membrane disruption, DNA damage and generation of intracellular reactive oxygen species. Silver, oxides of metals such as titanium, copper, zinc and iron, are some of the excellent antimicrobial agents known (Singh et al., 2008; Raghunath and Perumal, 2017). However, despite their excellent antimicrobial properties, many nanoparticle based antimicrobial agents have potential cytotoxicity that needs to be addressed. Immobilization of AMPs to metallic nanoparticles reduces cytotoxicity and improves the antimicrobial potency of the individual components synergistically (Allahverdiyev et al., 2011). Some of the inherent drawbacks of AMPs, such as susceptibility to proteases and poor permeability across biological barriers, are also eliminated on conjugation with nanoparticles (Rajchakit and Sarojini, 2017). Figure 11 schematically represents the synthesis of AMP-NP conjugates.
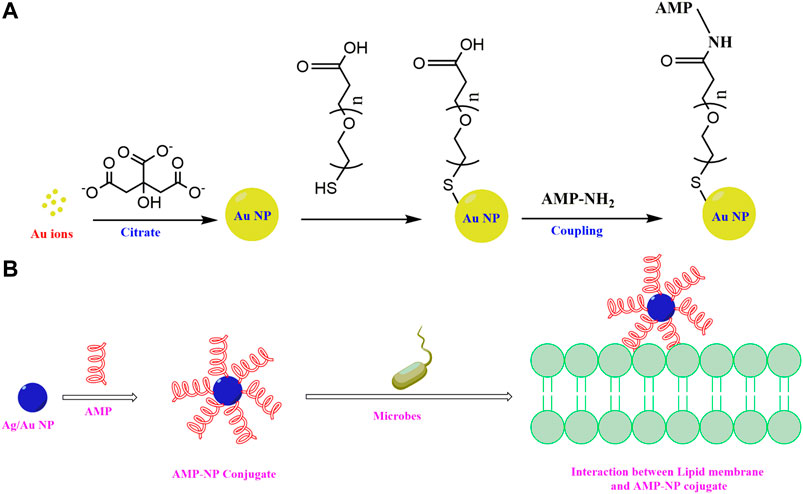
FIGURE 11. Schematic of (A) Stepwise synthesis of AMP-AuNP conjugates and (B) Formation of AMP-NP conjugates and their action on lipid membrane of the microbes.
Cell-penetrating peptide G3R6TAT (Liu et al., 2009) was used as the stabilizer and reductant to produce colloid silver nanoparticles. Though the antimicrobial activity of the Ag-peptide was lower (against E. Coli, B. subtilis and C. albicans) compared to Ag-Citrate, Ag-SDS and Au@Ag, the Ag-peptide was much less hemolytic compared to the others (Liu et al., 2013). Pal et al. conjugated cationic antimicrobial peptide Odorranain-A-OA1 (VVKCSYRLGSPDSQCN) extracted from the skin of Chinese odorous frogs with AgNPs. AgNP-OA1 was more potent against E. coli and less cytotoxic in comparison to the AgNP alone (Pal et al., 2016). Maleki et al. reported the preparation of biocompatible antimicrobial SPION@gold core shell NPs based on the covalent immobilization of the AMP cecropin-melittin (CM). Antimicrobial activities of the AMP-NP conjugate against gram-positive (S. aureus) and gram-negative (E. Coli) bacteria were much better than the soluble AMPs. AMP-NPs demonstrated better destruction of the microbial membranes and less hemolytic activity compared to bare NPs (Maleki et al., 2016). Pazos et al. designed PA nanofibers modified with functional groups that induced the formation of AgNPs over PA fibres. Peptide PA1 had an N-terminal aldehyde while the control peptide PA2 did not. Both the peptides formed nanofibres and on treating with Tollens reagent formed PA-AgNPs. Metallized PA nanofibers inhibited the growth of E. Coli inoculated in LB medium, whereas PA nanofibers did not. PA nanofibers were 30 times less toxic to the eukaryotic cells compared to the bacterial cells. Additionally, PA1-AgNPs formed antimicrobial gels with CaCl2 (Pazos et al., 2016). Otari et al. designed gold-nanoparticle alginate (Au-peptide-alginate) biohydrogel using a thermostable AMP nisin, effective against a variety of gram-positive bacteria. Au-peptide-alginate biohydrogel had significant antimicrobial activity against human bacterial pathogens S. aureus, B. cereus (Bacillus cereus), and E. faecalis, while the AuNP alginate did not (Otari et al., 2016).
Conclusion
With the development of rapid resistance in microbes against standard antibiotics, the ability of the AMPs to invoke delayed resistance makes them an extremely potential therapeutic agent. Several AMPs in combination with conventional antibiotics have been shown to inhibit multiple drug-resistant strains which were resistant to the antibiotic alone, due to synergistic effect. Synthetic AMPs have been shown to have improved potency, diminished cytotoxicity, increased protease-resistance over the natural AMPs. A lot of potential therapeutic molecules can be developed by taking inspiration from the vast repertoire of AMPs that nature has to offer. Understanding the mechanisms of action of the AMPs will enable in the design of synthetic AMPs with improved specificity against specific microbial strains as well.
Author Contributions
The review was written partially by TS and MC under the guidance of SC. TS made the figures tables. MC compiled the references. SC planned the overall outline of the review and wrote major parts of the review.
Funding
SC acknowledges Department of Biotechnology (No. DBT01118) and CSIR India (01 (2984)/19/EMR-II) for funding. TS, and MC thank IIT Guwahati for funding.
Conflict of Interest
The authors declare that the research was conducted in the absence of any commercial or financial relationships that could be construed as a potential conflict of interest.
References
Agerberth, B., Lee, J. Y., Bergman, T., Carlquist, M., Boman, H. G., Mutt, V., et al. (1991). Amino Acid Sequence of PR-39. Isolation from Pig Intestine of a New Member of the Family of Proline-Arginine-Rich Antibacterial Peptides. Eur. J. Biochem. 202, 849–854. doi:10.1111/j.1432-1033.1991.tb16442.x
Akbari, R., Hakemi Vala, M., Pashaie, F., Bevalian, P., Hashemi, A., and PooshangBagheri, K. (2019). Highly Synergistic Effects of Melittin with Conventional Antibiotics against Multidrug-Resistant Isolates of Acinetobacter Baumannii and. Pseudomonas aeruginosa. Microb. Drug Resist. 25, 193–202. doi:10.1089/mdr.2018.0016
Akpa, E., Jacques, P., Wathelet, B., Paquot, M., Fuchs, R., Budzikiewicz, H., et al. (2001). Infuence of Culture Conditions on Lipopeptide Production by. Bacillus Subtilis. Appl. Biochem. Biotechnol. 91, 551–561. doi:10.1385/abab:91-93:1-9:551
Albada, H. B., Prochnow, P., Bobersky, S., Bandow, J. E., and Metzler-Nolte, N. (2014). Highly Active Antibacterial Ferrocenoylated or Ruthenocenoylated Arg-Trp Peptides Can Be Discovered by an L-To-D Substitution Scan. Chem. Sci. 5, 4453–4460. doi:10.1039/C4SC01822B
Albada, H. B., Prochnow, P., Bobersky, S., Langklotz, S., Schriek, P., Bandow, J. E., et al. (2012). Tuning the Activity of a Short Arg-Trp Antimicrobial Peptide by Lipidation of a C- or N-Terminal Lysine Side-Chain. ACS Med. Chem. Lett. 3, 980–984. doi:10.1021/ml300148v
Albiol Matanic, V. C., and Castilla, V. (2004). Antiviral Activity of Antimicrobial Cationic Peptides against Junin Virus and Herpes Simplex Virus. Int. J. Antimicrob. Agents 23, 382–389. doi:10.1016/j.ijantimicag.2003.07.022
Allahverdiyev, A. M., Kon, K. V., Abamor, E. S., Bagirova, M., and Rafailovich, M. (2011). Coping with Antibiotic Resistance: Combining Nanoparticles with Antibiotics and Other Antimicrobial Agents. Expert Rev. Anti-infect. Ther. 9, 1035–1052. doi:10.1586/eri.11.121
Andersen, J. B., Koch, B., Nielsen, T. H., Sørensen, D., Hansen, M., Nybroe, O. C., et al. (2003). Surface Motility in Pseudomonas Sp. DSS73 Is Required for Efficient Biological Containment of the Root Pathogenic Micro Fungi Pythium Ultimum and Rhizoctonia solani. Microbiology 149, 37–46. doi:10.1099/mic.0.25859-0
Arima, K., Kakinuma, A., and Tamura, G. (1968). Surfactin, a Crystalline Peptide Lipid Surfactant Produced by Bacillus Subtilis: Isolation, Characterization and its Inhibition of Fibrin Clot Formation. Biothem. Biophys. Res. Commun. 31, 488–494. doi:10.1016/0006-291x(68)90503-2
Attoub, S., Mechkarska, M., Sonnevend, A., Radosavljevic, G., Jovanovic, I., Lukic, M. L., et al. (2012). Esculentin-2CHa: a Host-Defense Peptide with Differential Cytotoxicity against Bacteria, Erythrocytes and Tumor Cells. Peptides. 39, 95–102. doi:10.1016/j.peptides.2012.11.004
Avrahami, D., and Shai, Y. (2003). Bestowing Antifungal and Antibacterial Activities by Lipophilic Acid Conjugation to D, L-Amino Acid-Containing Antimicrobial Peptides: A Plausible Mode of Action. Biochemistry 42, 14946–14956. doi:10.1021/bi035142v
Azuma, E., Choda, N., Odaki, M., Yano, Y., and Matsuzaki, K. (2020). Improvement of Therapeutic index by the Combination of Enhanced Peptide Cationicity and Proline Introduction. ACS Infect. Dis. 6, 2271–2278. doi:10.1021/acsinfecdis.0c00387
Bagheri, M., Nikolenko, H., Arasteh, S., Rezaei, N., Behzadi, M., Dathe, M., et al. (2020). Bacterial Aggregation Triggered by Fibril Forming Tryptophan-Rich Sequences: Effects of Peptide Side Chain and Membrane Phospholipids. ACS Appl. Mater. Inter. 12, 26852–26867. doi:10.1021/acsami.0c04336
Bals, R., Wang, X., Wu, Z., Freeman, T., Bafna, V., Zasloff, M., et al. (1998). Human β-defensin 2 Is a Salt-Sensitive Peptide Antibiotic Expressed in Human Lung. J. Clin. Invest. 102, 874–880. doi:10.1172/JCI2410
Baral, A., Roy, S., Ghosh, S., Merino, D. H., Hamley, I. W., and Banerjee, A. (2016). A Peptide-Based Mechano-Sensitive, Proteolytically Stable Hydrogel with Remarkable Antibacterial Properties. Langmuir 32, 1836. doi:10.1021/acs.langmuir.5b03789
Bassarello, C., Lazzaroni, S., Bifulco, G., Cantore, P. L., Iacobellis, N. S., et al. (2004). Tolaasins A-E, Five New Lipodepsipeptides Produced by. Pseudomonas Tolaasii. J. Nat. Prod. 67, 811–816. doi:10.1021/np0303557
Baumann, T., Kämpfer, U., Schürch, S., Schaller, J., Largiadèr, C., Nentwig, W., et al. (2010). Ctenidins: Antimicrobial Glycine-Rich Peptides from the Hemocytes of the Spider. Cupiennius Salei. Cel. Mol. Life Sci. 67, 2787–2798. doi:10.1007/s00018-010-0364-0
Bechinger, B., and Lohner, K. (2006). Detergent-like Actions of Linear Amphipathic Cationic Antimicrobial Peptides. Biochim. Biophys. Acta 1758, 1529–1539. doi:10.1016/j.bbamem.2006.07.001
Biondi, B., Casciaro, B., Grazia, A. D., Cappiello, F., Luca, V., Crisma, M., et al. (2017). Effects of Aib Residues Insertion on the Structural–Functional Properties of the Frog Skin-Derived Peptide esculentin-1a(1–21)NH2. Amino Acids 49, 139–150. doi:10.1007/s00726-016-2341-x
Blazyk, J., Wiegand, R., Klein, J., Hammer, J., Epand, R. M., Epand, R. F., et al. (2001). A Novel Linear Amphipathic β-Sheet Cationic Antimicrobial Peptide with Enhanced Selectivity for Bacterial Lipids. J. Biol. Chem. 276 (2001), 27899–27906. doi:10.1074/jbc.M102865200
Blondelle, S. E., PérezPayá, E., and Houghten, R. A. (1996). Synthetic Combinatorial Libraries: Novel Discovery Strategy for Identification of Antimicrobial Agents. Antimicrob. Agents Chemother. 40, 1067–1071. doi:10.1128/AAC.40.5.1067
Bohlman, H., and Apel, K. (1991). Thionins. Annu. Rev. Plant Physiol. Plant Mol. Biol. 42, 227–240. doi:10.1146/annurev.pp.42.060191.001303
Bong, D. T., Clark, T. D., Granja, J. R., and Ghadiri, M. R. (2001). Self-assembling Organic Nanotubes. Angew. Chem. Int. Ed. Engl. 40, 988–1011. doi:10.1002/1521-3773(20010316)40:6%3C988::AID-ANIE9880%3E3.0.CO;2-N
Borro, B. C., Nordström, R., and Malmsten, M. (2020). Microgels and Hydrogels as Delivery Systems for Antimicrobial Peptides. Colloids Surf. B Biointerfaces. 187, 110835. doi:10.1016/j.colsurfb.2020.110835
Boxell, A., Lee, S. H. C., Jefferies, R., Watt, P., Hopkins, R., Reid, S., et al. (2008). Pyrrhocoricinasa Potential Drug Delivery Vehicle for Cryptosporidium Parvum.. Exp. Parasitol. 119, 301–303. doi:10.1016/j.exppara.2008.02.005
Braff, M. H., and Gallo, R. L. (2006). Antimicrobial Peptides: an Essential Component of the Skin Defensive Barrier. Curr. Top. Microbiol. Immunol. 306, 91–110. doi:10.1007/3-540-29916-5_4
Brockmann, H., and Scttmidt-Kastner, G. (1955). Valinomycin I XXVII uber Antibiotica aus Actinomyceten. Chem. Ber. 88, 57–61. doi:10.1002/cber.19550880111
Brogden, K. A. (2005). Antimicrobial Peptides: Pore Formers or Metabolic Inhibitors in Bacteria?. Nat. Rev. Microbiol. 3, 238–250. doi:10.1038/nrmicro1098
Brown, K. L., and Hancock, R. E. W. (2006). Cationic Host Defense (Antimicrobial) Peptides. Curr. Opin. Immunol. 18, 24–30. doi:10.1016/j.coi.2005.11.004
Bulet, P., Dimarcq, J. L., Hetru, C., Lagueux, M., Charlet, M., Hegy, G., et al. (1993). A Novel Inducible Antibacterial Peptide of Drosophila Carries an O-Glycosylated Substitution. J. Biol. Chem. 268, 14893–14897. doi:10.1016/S0021-9258(18)82417-6
Burr, T., Matteson, M., Smith, C., Corral-Garcia, M., and Huang, T-C. (1996). Effectiveness of Bacteria and Yeasts from Apple Orchards as Biological Control Agents of Apple Scab. Biol. Control. 6, 151–157. doi:10.1006/bcon.1996.0019
Burrows, L. L., Stark, M., Chan, C., Glukhov, E., Sinnadurai, S., and Deber, C. M. (2006). Activity of Novel Non-amphipathic Cationic Antimicrobial Peptides against Candida Species. J. Antimicrob. Chemother. 57, 899–907. doi:10.1093/jac/dkl056
Cao, F., Mei, L., Zhu, G., Song, M., and Zhang, X. (2019). An Injectable Molecular Hydrogel Assembled by Antimicrobial Peptide PAF26 for Antimicrobial Application. RSC Adv. 9, 30803. doi:10.1039/c9ra06130d
Cappiello, F., Grazia, A. D., Segev-Zarko, L-A., Scali, S., Ferrera, L., Galietta, L., et al. (2016). Esculentin-1a-derived Peptides Promote Clearance of Pseudomonas aeruginosa Internalized in Bronchial Cells of Cystic Fibrosis Patients and Lung Cell Migration: Biochemical Properties and a Plausible Mode of Action. Antimicrob. Agents Chemother. 60, 7252–7262. doi:10.1128/AAC.00904-16
Carlsson, A., Engström, P., Palva, E. T., and Bennich, H. (1991). Attacin, an Antibacterial Protein from Hyalophora Cecropia, Inhibits Synthesis of Outer Membrane Proteins in Escherichia coli by Interfering with Omp Gene Transcription. Infect. Immun. 59, 3040–3045. doi:10.1128/IAI.59.9.3040-3045.1991
Carlsson, A., Nyström, T., de Cock, H., and Bennich, H. (1998). Attacin—An Insect Immune Protein—Binds LPS and Triggers the Specific Inhibition of Bacterial Outer-Membrane Protein Synthesis. Microbiology 144, 2179–2188. doi:10.1099/00221287-144-8-2179
Cascales, J. J. L., Zenak, S., de la Torre, J. G., Lezama, O. G., Garro, A., and Enriz, R. D. (2018). Small Cationic Peptides: Influence of Charge on Their Antimicrobial Activity. ACS Omega 3, 5390–5398. doi:10.1021/acsomega.8b00293
Casciaro, B., Cappiello, F., Loffredo, M. R., Ghirga, F., and Mangoni, M. L. (2020). The Potential of Frog Skin Peptides for Anti-infective Therapies: The Case of Esculentin-1a(1-21)NH2. Curr. Med. Chem. 27, 1405–1419. doi:10.2174/0929867326666190722095408
Casteels, P., Ampe, C., Jacobs, F., Vaeck, M., and Tempst, P. (1989). Apidaecins: Antibacterial Peptides from Honeybees. EMBO J. 8, 2387–2391. doi:10.1002/j.1460-2075.1989.tb08368.x
Casteels, P., Ampe, C., Riviere, L., Van Damme, J., Elicone, C., Fleming, M., et al. (1990). Isolation and Characterization of Abaecin, a Major Antibacterial Response Peptide in the Honeybee (Apis mellifera). Eur. J. Biochem. 187, 381–386. doi:10.1111/j.1432-1033.1990.tb15315.x
Castelletto, V., Barnes, R. H., Karatzas, K-A., Edwards-Gayle, C. J. C., Greco, F., Hamley, I. W., et al. (2018). Arginine Containing Surfactant-like Peptides: Interaction with Lipid Membranes and Antimicrobial Activity. Biomacromolecules 19, 2782–2794. doi:10.1021/acs.biomac.8b00391
Chan, D. I., Prenner, E. J., and Vogel, H. J. (2006). Tryptophan and Arginine-Rich Antimicrobial Peptides: Structures and Mechanisms of Action. Biochim. Biophys. Acta 1758, 1184–1202. doi:10.1016/j.bbamem.2006.04.006
Chapman, R., Danial, M., Koh, M. L., Jolliffe, K. A., and Perrier, S. (2012). Design and Properties of Functional Nanotubes from the Self-Assembly of Cyclic Peptide Templates. Chem. Soc. Rev. 41, 6023–6041. doi:10.1039/C2CS35172B
Chen, J., Guan, S. M., Sun, W., and Fu, H. (2016). Melittin, the Major Pain Producing Substance of Bee Venom. Neurosci. Bull. 32, 265–272. doi:10.1007/s12264-016-0024-y
Chen, Y. X., Vasil, A. I., Rehaume, L., Mant, C. T., Burns, J. L., Vasil, M. L., et al. (2006). Comparison of Biophysical and Biologic Properties of Alpha-Helical Enantiomeric Antimicrobial Peptides. Chem. Biol. Drug Des. 67, 162–173. doi:10.1111/j.1747-0285.2006.00349.x
Chesnokova, L. S., Slepenkov, S. V., and Witt, S. N. (2004). The Insect Antimicrobial Peptide, L-Pyrrhocoricin, Binds to and Stimulates the ATPase Activity of Both Wild-type and Lidless DnaK. FEBS Lett. 565, 65–69. doi:10.1016/j.febslet.2004.03.075
Choi, J. H., Jang, A. Y., Lin, S., Lim, S., Kim, D., Park, K., et al. (2015). Melittin, a Honeybee Venom-Derived Antimicrobial Peptide, May Target Methicillin-Resistant Staphylococcus aureus. Mol. Med. Rep. 12, 6483–6490. doi:10.3892/mmr.2015.4275
Choi, J., and Moon, E. (2009). Identification of Novel Bioactive Hexapeptides against Phytopathogenic Bacteria through Rapid Screening of a Synthetic Combinatorial Library. J. Microbiol. Biotechnol. 19, 792–802. doi:10.4014/jmb.0809.497
Chu-Kung, A. F., Bozzelli, K. N., Lockwood, N. A., Haseman, J. R., Mayo, K. H., and Tirrell, M. V. (2004). Promotion of Peptide Antimicrobial Activity by Fatty Acid Conjugation Bioconjug. Chem. 15, 530–535. doi:10.1021/bc0341573
Cociancich, S., Dupont, A., Hegy, G., Lanot, R., Holder, F., Hetru, C., et al. (1994). Novelinducible Antibacterial Peptides from a Hemipteran Insect, the Sap-Sucking Bug. Pyrrhocoris apterus. Biochem. J. 300, 567–575. doi:10.1042/bj3000567
Cole, A. M., Kim, Y. H., Tahk, S., Hong, T., Weis, P., Waring, A. J., et al. (2001). Calcitermin, a Novel Antimicrobial Peptide Isolated from Human Airway Secretions. FEBS Lett. 504, 5–10. doi:10.1016/s0014-5793(01)02731-4
Cole, A. M., Wang, W., Waring, A. J., and Lehrer, R. I. (2004). Retrocyclins: Using Past as Prologue. Curr. Protein Pept. Sci. 5, 373–381. doi:10.2174/1389203043379657
Colgrave, M. L., and Craik, D. J. (2004). Thermal, Chemical, and Enzymatic Stability of the Cyclotide Kalata B1 the Importance of the Cyclic Cystine Knot. Biochemistry 43, 5965–5975. doi:10.1021/bi049711q
Conlon, J. M., and Mechkarska, M. (2014). Host-defense Peptides with Therapeutic Potential from Skin Secretions of Frogs from the Family Pipidae. Pharmaceuticals 7, 58–77. doi:10.3390/ph7010058
Conlon, J. M., Mechkarska, M., Coquet, L., Jouenne, T., Leprince, J., Vaudry, H., et al. (2011). Characterization of Antimicrobial Peptides in Skin Secretions from Discrete Populations of Lithobates Chiricahuensis (Ranidae) from central and Southern Arizona. Peptides 32, 664–669. doi:10.1016/j.peptides.2011.01.018
Craik, D. J. (2010). Discovery and Applications of Plant Cyclotides. Toxicon 56, 1092–1102. doi:10.1016/j.toxicon.2010.02.021
Craik, D. J., Daly, N. L., Mulvenna, J., Plan, M. R., and Trabi, M. (2004). Discovery, Structure and Biological Activities of the Cyclotides. Curr. Protein Pept. Sci. 5, 297–315. doi:10.2174/1389203043379512
Da Silva, A., Kawazoe, U., Freitas, F. F. T., Gatti, M. S. V., Dolder, H., Schumacher, R. I., et al. (2002). Avian Anticoccidial Activity of a Novel Membrane-Interactive Peptide Selected from Phage Display Libraries. Mol. Biochem. Parasitol. 120, 53–60. doi:10.1016/s0166-6851(01)00439-x
Daly, N. L., Clark, R. J., Plan, M. R., and Craik, D. J. (2006). Kalata B8, a Novel Antiviral Circular Protein, Exhibits Conformational Flexibility in the Cystine Knot Motif. Biochem. J. 393, 619–626. doi:10.1042/BJ20051371
Dartois, V., Sanchez-Quesada, J., Cabezas, E., Chi, E., Dubbelde, C., Dunn, C., et al. (2005). Systemic Antibacterial Activity of Novel Synthetic Cyclic Peptides. Antimicrob. Agents Chemother. 49, 3302–3310. doi:10.1128/AAC.49.8.3302–3310.2005
Diamond, G., Beckloff, N., Weinberg, A., and Kisich, K. O. (2009). The Roles of Antimicrobial Peptides in Innate Host Defense. Curr. Pharm. Des. 15, 2377–2392. doi:10.2174/138161209788682325
Dimarcq, J. L., Keppi, E., Dunbar, B., Lambert, J., Reichhart, J. M., Hoffmann, D., et al. (1988). Insect Immunity Purification and Characterization of a Family of Novel Inducible Antibacterial Proteins from Immunized Larvae of the Dipteran Phormia Terranovae and Complete Amino-Acid Sequence of the Predominant Member, Diptericin A. Eur. J. Biochem. 171, 17–22. doi:10.1111/j.1432-1033.1988.tb13752.x
Dinh, T. T. T., Kim, D-H., Luong, H. X., Lee, B-J., and Kim, Y-W. (2015). Antimicrobial Activity of Doubly-Stapled Alanine/lysine-Based Peptides. Bioorg. Med. Chem. Lett. 25, 4016–4019. doi:10.1016/j.bmcl.2015.06.053
Domachowske, J. B., Bonville, C. A., Dyer, K. D., and Rosenberg, H. F. (1998). Evolution of Antiviral Activity in the Ribonuclease A Gene Superfamily: Evidence for a Specific Interaction between Eosinophil-Derived Neurotoxin (EDN/RNase 2) and Respiratory Syncytial Virus. Nucleic Acids Res. 26, 5327–5332. doi:10.1093/nar/26.23.5327
Dong, N., Ma, Q., Shan, A., Lv, Y., Hu, W., Gu, Y., et al. (2012). Strand Length-dependent Antimicrobial Activity and Membrane-Active Mechanism of Arginine- and Valine-Rich β-hairpin-like Antimicrobial Peptides. Antimicrob. Agents Chemother. 56, 2994–3003. doi:10.1128/AAC.06327-11
Dutta, P. D., and Das, S. (2016). Mammalian Antimicrobial Peptides: Promising Therapeutic Targets against Infection and Chronic Inflammation. Curr. Top. Med. Chem. 16, 99–129. doi:10.2174/1568026615666150703121819
Eliassen, L. T., Berge, G., Sveinbjørnsson, B., Svendsen, J. S., Vorland, L. H., and Rekdal, Ø. (2002). Evidence for a Direct Antitumor Mechanism of Action of Bovine Lactoferricin. Anticancer Res. 22, 2703–2710.
Epand, R. M., Epand, R. F., Arnusch, C. J., Papahadjopoulos-Sternberg, B., Wang, G., and Shai, Y. (2010). Lipid Clustering by Three Homologous Arginine-Rich Antimicrobial Peptides Is Insensitive to Amino Acid Arrangement and Induced Secondary Structure. Biochim. Biophys. Acta 1798, 1272–1280. doi:10.1016/j.bbamem.2010.03.012
Epand, R. M., and Vogel, H. J. (1999). Diversity of Antimicrobial Peptides and Their Mechanisms of Action. Biochim. Biophys. Acta 1462, 11–28. doi:10.1016/s0005-2736(99)00198-4
Fahrner, R. L., Dieckmann, T., Harwig, S. S. L., Lehrer, R. I., Eisenberg, D., and Feigon, J. (1996). Solution Structure of Protegrin-1, a Broad-Spectrum Antimicrobial Peptide from Porcine Leukocytes. Chem. Biol. 3, 543–550. doi:10.1016/s1074-5521(96)90145-3
Falla, T. J., and Hancock, R. E. (1997). Improved Activity of a Synthetic Indolicidin Analog. Antimicrob. Agents Chemother. 41, 771–775. doi:10.1128/AAC.41.4.771
Fehlbaum, P., Bulet, P., Michaut, L., Lagueux, M., Broekaert, W. F., Hetru, C., et al. (1994). Insect Immunity. Septic Injury of Drosophila Induces the Synthesis of a Potent Antifungal Peptide with Sequence Homology to Plant Antifungal Peptides. J. Biol. Chem. 269, 33159–33163. doi:10.1016/S0021-9258(20)30111-3
Fernandez-Lopez, S., Kim, H. S., Choi, E. C., Delgado, M., Granja, J. R., Khasanov, A., et al. (2001). Antibacterial Agents Based on the Cyclic D, L-Alpha-Peptide Architecture. Nature 412, 452–455. doi:10.1038/35086601
Fernandez-Reyes, M., Diaz, D., dela Torre, B. G., Cabrales-Rico, A., Valles-Miret, M., Jimenez-Barbero, J., et al. (2010). Lysine N(epsilon)-Trimethylation, a Tool for Improving the Selectivity of Antimicrobial Peptides. J. Med. Chem. 53, 5587–5596. doi:10.1021/jm100261r
Fernández-Vidal, M., Jayasinghe, S., Ladokhin, A. S., and White, S. H. (2007). Folding Amphipathic Helices into Membranes: Amphiphilicity Trumps Hydrophobicity. J. Mol. Biol. 2007 (370), 459–470. doi:10.1016/j.jmb.2007.05.016
Findlay, B., Zhanel, G. G., and Schweizer, F. (2010). Cationic Amphiphiles, a New Generation of Antimicrobials Inspired by the Natural Antimicrobial Peptide Scaffold. Antimicrob. Agents Chemother. 54, 4049–4058. doi:10.1128/AAC.00530-10
Fleming, A. (1992). On a Remarkable Bacteriolytic Element Found in Tissues and Secretions. Proc. R. Soc. 93, 306–317. doi:10.1098/rspb.1922.0023
Fletcher, J. T., Finlay, J. A., Callow, M. E., Callow, J. A., and Ghadiri, M. R. (2007). A Combinatorial Approach to the Discovery of Biocidal Six-Residue Cyclic D, L-α-Peptides against the Bacteria Methicillin-Resistant Staphylococcus aureus (MRSA) and E. coli and the Biofouling Algae Ulva Linza and. Navicula Perminuta. Chem.–Eur. J. 13, 4008–4013. doi:10.1002/chem.200601583
Florin, T., Maracci, C., Graf, M., Karki, P., Klepacki, D., Berninghausen, O., et al. (2017). An Antimicrobial Peptide that Inhibits Translation by Trapping Release Factors on the Ribosome. Nat. Struct. Mol. Bio. 24, 752–757. doi:10.1038/nsmb.3439
Fontana, R., Mendes, M. A., deSouza, B. M., Konno, K., César, L. M., Malaspina, O., et al. (2004). Jelleines: A Family of Antimicrobial Peptides from the Royal Jelly of Honeybees (Apis mellifera). Peptides 25, 919–928. doi:10.1016/j.peptides.2004.03.016
Fukuchi, N., Isogai, A., Nakayama, J., Takayama, S., Yamashita, S., Suyama, K., et al. (1992). Structure and Stereochemistry of Three Phytotoxins, Syringomycin, Syringotoxin and Syringostatin, Produced by pseudomonas Syringae Pv. Syringae. J. Chem. Soc. Perkin Trans. 1, 1149–1157. doi:10.1039/P19920001149
Gagnon, M. G., Roy, R. N., Lomakin, I. B., Florin, T., Mankin, A. S., and Steitz, T. A. (2016). Structures of Proline-Rich Peptides Bound to the Ribosome Reveal a Common Mechanism of Protein Synthesis Inhibition. Nucleic Acids Res. 44, 2439–2450. doi:10.1093/nar/gkw018
Gamberi, T., Cavalieri, D., Magherini, F., Mangoni, M. L., De Filippo, C., Borro, M., et al. (2007). An Integrated Analysis of the Effects of Esculentin 1–21 on. Saccharomyces cerevisiae. Biochim. Biophys. Acta 1774, 688–700. doi:10.1016/j.bbapap.2007.04.006
Ganz, T. (2001). Defensins in the Urinary Tract and Other Tissues. J. Infect. Dis. 183, 41–42. doi:10.1086/318838
Gao, A. G., Hakimi, S. M., Mittanck, C. A., Wu, Y., Woerner, B. M., Stark, D. M., et al. (2000). Fungal Pathogen protection in Potato by Expression of a Plant Defensin Peptide. Nat. Biotechnol. 18, 1307–1310. doi:10.1038/82436
Gause, G. F., and Brazhnikova, M. G. (1944). Gramicidin S and its Use in the Treatment of Infected Wounds. Nature 154, 703. doi:10.1038/154703a0
Gennaro, R., Skerlavaj, B., and Romeo, D. (1989). Purification, Composition, and Activity of Two Bactenecins, Antibacterial Peptides of Bovine Neutrophils. Infect. Immun. 57, 3142–3146. doi:10.1128/IAI.57.10.3142-3146.1989
Gennaro, R., Zanetti, M., Benincasa, M., Podda, E., and Miani, M. (2002). Pro-rich Antimicrobial Peptides from Animals: Structure, Biological Functions and Mechanism of Action. Curr. Pharm. Des. 8, 763–778. doi:10.2174/1381612023395394
Ghadiri, M. R., Granja, J. R., Milligan, R. A., McRee, D. E., and Khazanovich, N. (1993). Self-assembling Organic Nanotubes Based on a Cyclic Peptide Architecture. Nature 366, 324–327. doi:10.1038/366324a0
Giacometti, A., Cirioni, O., Kamysz, W., D’Amato, G., Silvestri, C., Prete, Del., et al. (2005). In Vitro activity and Killing Effect of Temporin A on Nosocomial Isolates of Enterococcus faecalis and Interactions with Clinically Used Antibiotics. J. Antimicrob. Chemother. 55, 272–274. doi:10.1093/jac/dkh545
Gifford, J. L., Hunter, H. N., and Vogel, H. J. (2005). Lactoferricin: a Lactoferrin-Derived Peptide with Antimicrobial, Antiviral, Antitumor and Immunological Properties. Cell Mol Life Sci. 62, 2588–2598. doi:10.1007/s00018-005-5373-z
Goldman, M. J., Anderson, G. M., Stolzenberg, E. D., Kari, U. P., Zasloff, M., and Wilson, J. M. (1997). Human β-defensin-1 Is a Salt-Sensitive Antibiotic in Lung that Is Inactivated in Cystic Fibrosis. Cell 88, 553–560. doi:10.1016/s0092-8674(00)81895-4
Gomes, P. S., and Fernandes, M. H. (2010). Defensins in the Oral Cavity: Distribution and Biological Role. J. Oral Pathol. Med. 39, 1–9. doi:10.1111/j.1600-0714.2009.00832.x
Gopal, R., Na, H., Seo, C. H., and Park, Y. (2012). Antifungal Activity of (KW)n or (RW)n Peptide against Fusariumsolani and Fusarium Oxysporum. Int. J. Mol. Sci. 13, 15042–15053. doi:10.3390/ijms131115042
Gopal, R., Park, S-C., Ha, K-J., Cho, S. J., Kim, S. W., Song, P. I., et al. (2009). Effect of Leucine and Lysine Substitution on the Antimicrobial Activity and Evaluation of the Mechanism of the HPA3NT3 Analog Peptide. J. Pept. Sci. 15, 589–594. doi:10.1002/psc.1155
Grgurina, I., Bensaci, M., Pocsfalvi, G., Mannina, L., Cruciani, O., Fiore, A., et al. (2005). Novel Cyclic Lipodepsipeptide from Pseudomonas syringae Pv. Lachrymans Strain 508 and Syringopeptin Antimicrobial Activities. Antimicrob. Agents Chemother. 49, 5037–5045. doi:10.1128/AAC.49.12.5037-5045.2005
Gudmundsson, G. H., Agerbeth, B., Odeberg, J., Bergman, T., Olsson, B., and Salcedo, R. (1996). The Human Gene FALL-39 and Processing of the Cathelin Precursor to the Antibacterial Peptide LL-37 in Granulocytes. Eur. J. Biochem. 238, 325–332. doi:10.1111/j.1432-1033.1996.0325z.x
Hamann, K. J., Gleich, G. J., Checkel, J. L., Loegering, D. A., McCall, J. W., and Barker, R. L. (1990). In Vitro killing of Microfilariae of Brugia Pahangi and Brugia malayi by Eosinophil Granule Proteins. J. Immunol. 144, 3166–3173.
Hancock, R. E. W., and Rozek, A. (2002). Role of Membranes in the Activities of Antimicrobial Cationic Peptides. FEMS Microbiol. Lett. 206, 143–149. doi:10.1111/j.1574-6968.2002.tb11000.x
Hancock, R. E. W., and Sahl, H-G. (2006). Antimicrobial and Host-Defense Peptides as New Anti-infective Therapeutic Strategies. Nat. Biotechnol. 24, 1551–1557. doi:10.1038/nbt1267
Hara, S., and Yamakawa, M. (1995). A Novel Antibacterial Peptide Family Isolated from the Silkworm,. Bombyx mori.Biochem. J. 310, 651–656. doi:10.1042/bj3100651
Harder, J., Bartels, J., Christophers, E., and Schroeder, J. M. (2001). Isolation and Characterization of Human Deta-Defensin-3, a Novel Human Inducible Peptide Antibiotic. J. Biol. Chem. 276, 5707–5713. doi:10.1074/jbc.M008557200
Harder, J., and Schroder, J. M. (2002). RNase 7, a Novel Innate Immune Defense Antimicrobial Protein of Healthy Human Skin. J. Biol. Chem. 277, 46779–46784. doi:10.1074/jbc.M207587200
Harris, F., Dennison, S. R., and Phoenix, D. A. (2009). Anionic Antimicrobial Peptides from Eukaryotic Organisms. Curr. Protein Pept. Sci. 10, 585–606. doi:10.2174/138920309789630589
He, R., Bonaventura, I. D., Visini, R., Gan, B. H., Fu, Y., Probst, D., et al. (2017). Design, crystal Structure and Atomic Force Microscopy Study of Thioether Ligated D, L-cyclic Antimicrobial Peptides Against Multidrug Resistant Pseudomonas aeruginosa. Chem. Sci. 8, 7464–7475. doi:10.1039/c7sc01599b
Heins, S. D., Jimenez, D. R., Manker, D. C., Marrone, P. G., McCoy, R. J., and Orjala, J. E. (2000). Strain of bacillus for Controlling Plant Diseases and Corn Rootworm. US Patent 6060051 A.
Heisey, R. M., Huang, J., Mishra, S. K., Keller, J. E., Miller, J. R., Putnam, A. R., et al. (1988). Production of Valinomycin, an Insecticidal Antibiotic, by Streptomyces Griseus Var. Exipertum Var. Nov. J. Agric. Food Chem. 36, 1283–1286. doi:10.1021/jf00084a039
Hetru, C., Troxler, L., and Hoffmann, J. A. (2003). Drosophiola Melanogaster Antimicrobial Defense. J. Infect. Dis. 187, S327–S334. doi:10.1086/374758
Hicks, R. P., Abercrombie, J. J., Wong, R. K., and Leung, K. P. (2013). Antimicrobial Peptides Containing Unnatural Amino Acid Exhibit Potent Bactericidal Activity against ESKAPE Pathogens. Bioorg. Med. Chem. 21, 205–214. doi:10.1016/j.bmc.2012.10.039
Hicks, R. P., Bhonsle, J. B., Venugopal, D., Koser, B. W., and Magill, A. J. (2007). De Novo design of Selective Antibiotic Peptides by Incorporation of Unnatural Amino Acids. J. Med. Chem. 2007 (50), 3026–3036. doi:10.1021/jm061489v
Hilchie, A. L., Wuerth, K., and Hancock, R. E. W. (2013). Immune Modulation by Multifaceted Cationic Host Defense (Antimicrobial) Peptides. Nat. Chem. Biol. 9, 761–768. doi:10.1038/nchembio.1393
Hoffknecht, B. C., Albada, H. B., Sturm, M., Prochnow, P., Bandow, J. E., and Metzler-Nolte, N. (2015). Synthesis and Antibacterial Activity of Trivalent Ultrashort Arg-Trp-Based Antimicrobial Peptides (AMPs). Med. Chem. Commun. 6, 372–376. doi:10.1039/C4MD00327F
Hoffmann, J. A., and Hetru, C. (1992). Insect Defensins: Inducible Antibacterial Peptides. Immunol. Today 13, 411–415. doi:10.1016/0167-5699(92)90092-L
Hooper, L. V., Stappenbeck, T. S., Hong, C. V., and Gordon, J. I. (2003). Angiogenins: A New Class of Microbicidal Proteins Involved in Innate Immunity. Nat. Immunol. 4, 269–273. doi:10.1038/ni888
Hou, S., Zhou, C., Liu, Z., Young, A. W., Shi, Z., Ren, D., et al. (2009). Antimicrobial Dendrimer Active against Escherichia coli Biofilms. Bioorg. Med. Chem. Lett. 19, 5478–5481. doi:10.1016/j.bmcl.2009.07.077
Hu, Y., Amin, M. N., Padhee, S., Wang, R. E., Qiao, Q., Bai, G., et al. (2012). Lipidated Peptidomimetics with Improved Antimicrobial Activity. ACS Med. Chem. Lett. 3, 683–686. doi:10.1021/ml3001215
Huang, R. H., Xiang, Y., Liu, X. Z., Zhang, Y., Hu, Z., and Wang, D. C. (2002). Two Novel Antifungal Peptides Distinct with a Five-Disulfide Motif from the Bark of Eucommia Ulmoides Oliv. FEBS Lett. 521, 87–90. doi:10.1016/s0014-5793(02)02829-6
Huang, R. H., Xiang, Y., Tu, G. Z., Zhang, Y., and Wang, D. C. (2004). Solution Structure of Eucommia Antifungal Peptide a Novel Structural Model Distinct with a Five-Disulfide Motif. Biochemistry 43, 6005–6012. doi:10.1021/bi036263y
Huang, T., Qian, Y., Fu, X., Huang, S., Li, Y., and Zhou, C. (2020). De Novo design of Triblock Amphiphilic Short Antimicrobial Peptides. ACS Appl. Polym. Mater. 9, 3988–3992. doi:10.1021/acsapm.0c00640
Huang, X., Xie, W., and Gong, Z. (2000). Characteristics and Antifungal Activity of a Chitin Binding Protein from. Ginkgo Biloba. FEBS Lett. 478, 123–126. doi:10.1016/s0014-5793(00)01834-2
Huang, Y. C., Lin, Y. M., Chang, T. W., Wu, S. J., Lee, Y. S., Chang, M. D., et al. (2007). The Flexible and Clustered Lysine Residues of Human Ribonuclease 7 Are Critical for Membrane Permeability and Antimicrobial Activity. J. Biol. Chem. 2007 (282), 4626–4633. doi:10.1074/jbc.M607321200
Hultmark, D., Engström, A., Andersson, K., Steiner, H., Bennich, H., and Boman, H. G. (1983). Insect Immunity. Attacins a Family of Antibacterial Proteins from Hyalophora Cecropia. EMBO J. 2, 571–576. doi:10.1002/j.1460-2075.1983.tb01465.x
Hunter, H. N., Demcoe, A. R., Jenssen, H., Gutteberg, T. J., and Vogel, H. J. (2005). Human Lactoferricin Is Partially Folded in Aqueous Solution and Is Better Stabilized in a Membrane Mimetic Solvent. Antimicrob. Agents Chemother. 49, 3387–3395. doi:10.1128/AAC.49.8.3387-3395.2005
Hur, G. H., Vickery, C. R., and Burkart, M. D. (2012). Explorations of Catalytic Domains in Non-ribosomal Peptide Synthetase Enzymology. Nat. Prod. Rep. 29, 1074–1098. doi:10.1039/c2np20025b
Hussain, S., Güzel, Y., Pezzei, C., Rainer, M., Huck, C. W., and Bonn, G. K. (2014). Solid-phase Extraction of Plant Thionins Employing Aluminum Silicate Based Extraction Columns. J. Sep. Sci. 37, 2200–2207. doi:10.1002/jssc.201400385
Hwang, P. M., Zhou, N., Shan, X., Arrowsmith, C. H., and Vogel, H. J. (1998). Three-dimensional Solution Structure of Lactoferricin B, an Antimicrobial Peptide Derived from Bovine Lactoferrin. Biochemistry 37, 4288–4298. doi:10.1021/bi972323m
Ikai, K., Shiomi, K., Takesako, K., Mizutani, S., Yamamoto, J., Ogawa, Y., et al. (1991a). Structures of Aureobasidins B to R. J. Antibiot. 44, 1187–1198. doi:10.7164/antibiotics.44.1187
Ikai, K., Takesako, K., Shiomi, K., Moriguchi, M., Umeda, Y., Yamamoto, J., et al. (1991b). Structure of Aureobasidin A. J. Antibiot. 44, 925–933. doi:10.7164/antibiotics.44.925
Ili´c, N., Novkovi´c, M., Guida, F., Xhindoli, D., Benincasa, M., Tossi, A., et al. (2013). Selective Antimicrobial Activity and Mode of Action of Adepantins, Glycine-Rich Peptide Antibiotics Based on Anuran Antimicrobial Peptide Sequences. Biochim. Biophys. Acta 1828, 1004–1012. doi:10.1016/j.bbamem.2012.11.017
Imler, J. L., and Bulet, P. (2005). Antimicrobial Peptides in Drosophila: Structures, Activities and Gene Regulation. Chem. Immunol. Allergy 86, 1–21. doi:10.1159/000086648.104
Ireland, D. C., Clark, R. J., Daly, N. L., and Craik, D. J. (2010). Isolation, Sequencing, and Structure–Activity Relationships of Cyclotides. J. Nat. Prod. 73, 1610–1622. doi:10.1021/np1000413
Isono, K., and Suzuki, S. (1979). The Polyoxins-Pyrimidine Nucleoside Peptide Antibiotics Inhibiting Fungal Cell-wall Biosynthesis. Heterocycles 13, 333–351.
Jamasbi, E., Lucky, S. S., Li, W., Hossain, M. A., Gopalakrishnakone, P., and Separovic, F. (2018). Effect of Dimerized Melittin on Gastric Cancer Cells and Antibacterial Activity. Amino Acids. 50, 1101–1110. doi:10.1007/s00726-018-2587-6
Jamasbi, E., Mularski, A., and Separovic, F. (2016). Model Membrane and Cell Studies of Antimicrobial Activity of Melittin Analogues. Curr. Top. Med. Chem. 16, 40–45. doi:10.2174/1568026615666150703115919
Jang, W. S., Kim, H. K., Lee, K. Y., Kim, S. A., Han, Y. S., and Lee, I. H. (2006). Antifungal Activity of Synthetic Peptide Derived from Halocidin, Antimicrobial Peptide from the Tunicate. Halocynthia Aurantium. FEBS Lett. 580, 1490–1496. doi:10.1016/j.febslet.2006.01.041
Janiszewska, J., Sowinska, M., Rajnisz, A., Solecka, J., Lacka, I., Milewski, S., et al. (2012). Novel Dendrimeric Lipopeptides with Antifungal Activity. Bioorg. Med. Chem. Lett. 22, 1388–1393. doi:10.1016/j.bmcl.2011.12.051
Javadpour, M. M., Juban, M. M., Lo, W-C. J., Bishop, S. M., Alberty, J. B., Cowell, S. M., et al. (1996). De Novo antimicrobial Peptides with Low Mammalian Cell Toxicity. J. Med. Chem. 39, 3107–3113. doi:10.1021/jm9509410
Jenssen, H., Hamill, P., and Hancock, R. E. (2006). Peptide Antimicrobial Agents. Clin. Microbiol. Rev. 19, 491–511. doi:10.1128/CMR.00056-05
Jia, F., Wang, J., Peng, J., Zhao, P., Kong, Z., Wang, K., et al. (2018). The In Vitro, In Vivo Antifungal Activity and the Action Mode of Jelleine-I against Candida Species. Amino Acids. 50, 229–239. doi:10.1007/s00726-017-2507-1
Jiang, L., Xu, D., Sellati, T. J., and Dong, H. (2015). Self-assembly of Cationic Multidomain Peptide Hydrogels: Supramolecular Nanostructure and Rheological Properties Dictate Antimicrobial Activity. Nanoscale 7, 19160–19169. doi:10.1039/C5NR05233E
Jin, Y., Hammer, J., Pate, M., Zhang, Y., Zhu, F., Zmuda, E., et al. (2005). Antimicrobial Activities and Structures of Two Linear Cationic Peptide Families with Various Amphipathic β-sheet and Alpha-Helical Potentials. Antimicrob. Agents Chemother. 49, 4957–4964. doi:10.1128/AAC.49.12.4957-4964.2005
Jindal, H. M., Le, C. F., Yusof, M. Y., Velayuthan, R. D., Lee, V. S., et al. (2015). Antimicrobial Activity of Novel Synthetic Peptides Derived from Indolicidin and Ranalexin against Streptococcus pneumoniae. Plos ONE. 10 (6), e0128532. doi:10.1371/journal.pone.0128532
Jindal, H. M., Zandi, K., Ong, K. C., Velayuthan, R. D., Rasid, S. M., Raju, C. S., et al. (2017). Mechanisms of Action and In Vivo Antibacterial Efficacy Assessment of Five Novel Hybrid Peptides Derived from Indolicidin and Ranalexin against Streptococcus pneumoniae. PeerJ 5, e3887. doi:10.7717/peerj.3887
Jing, W., Demcoe, A. R., and Vogel, H. J. (2003). Conformation of a Bactericidal Domain of Puroindoline a: Structure and Mechanism of Action of a 13-Residue Antimicrobial Peptide. J. Bacteriol. 185, 4938–4947. doi:10.1128/JB.185.16.4938-4947.2003
Jonker, A. M., Löwik, D. W. P. M., and van Hest, V. J. C. M. (2012). Peptide- and Protein-Based Hydrogels. Chem. Mater. 24 (5), 759–773. doi:10.1021/cm202640w
Joo, S. H. (2012). Cyclic Peptides as Therapeutic Agents and Biochemical Tools. Biomol. Ther. 20, 19–26. doi:10.4062/biomolther.2012.20.1.019
Juhaniewicz-Dębinśka, J., Lasek, R., Tymecka, D., Burdach, K., Bartosik, D., and Sęk, S. (2020). Physicochemical and Biological Characterization of Novel Membrane-Active Cationic Lipopeptides with Antimicrobial Properties. Langmuir 36, 12900–12910. doi:10.1021/acs.langmuir.0c02135
Jung, H. J., Park, Y., Sung, W. S., Suh, B. K., Lee, J., Hahm, K. S., et al. (2007). Fungicidal Effect of Pleurocidin by Membrane Active Mechanism and Design of Enantiomeric Analogue for Proteolytic Resistance. Biochim. Biophys. Acta 1768, 1400–1405. doi:10.1016/j.bbamem.2007.02.024
Kajimura, Y., and Kaneda, M. (1997). Fusaricidins B, C and D, New Depsipeptide Antibiotics Produced by Bacillus Polymyxa KT-8: Isolation, Structure Elucidation and Biological Activity. J. Antibiot. 7, 50220–50228.
Kang, W., Liu, H., Ma, L., Wang, M., Wei, S., Sun, P., et al. (2017). Effective Antimicrobial Activity of a Peptide Mutant Cbf-14-2 against Penicillin-Resistant Bacteria Based on its Unnatural Amino Acids. Eur. J. Pharm. Sci. 105, 169–177. doi:10.1016/j.ejps.2017.05.030
Kang, X., Dong, F., Shi, C., Liu, S., Sun, J., Chen, J., et al. (2019). DRAMP 2.0, an Updated Data Repository of Antimicrobial Peptides. Scientific data 6. doi:10.1038/s41597-019-0154-y
Karlsson, A. J., Pomerantz, W. C., Weisblum, B., Gellman, S. H., and Palecek, S. P. (2006). Antifungal Activity from 14-helical β-peptides. J. Am. Chem. Soc. 128, 12630–12631. doi:10.1021/ja064630y
Karstad, R., Isaksen, G., Brandsdal, B. O., Svendsen, J. S., and Svenson, J. (2010). Unnatural Amino Acid Side Chains as S1, S1′, and S2′ Probes Yield Cationic Antimicrobial Peptides with Stability toward Chymotryptic Degradation. J. Med. Chem. 53, 5558–5566. doi:10.1021/jm1006337
Keppi, E., Pugsley, A. P., Lambert, J., Wicker, C., Dimarcq, J. L., Hoffmann, J. A., et al. (1989). Mode of Action of Diptericin A. A Bactericidal Peptide Induced Hemolymph Phormia Terranovae Larvae. Insect Biochem. Physiol. 10, 229–239. doi:10.1002/arch.940100306
Kiba, A., Saitoh, H., Nishihara, M., Omiya, K., and Yamamura, S. (2003). C- Terminal Domain of a Hevein-like Protein from Wasabia Japonica Has Potent Antimicrobial Activity. Plant Cel Physiol. 44, 296–303. doi:10.1093/pcp/pcg035
Kim, P. I., Ryu, J., Kim, Y. H., and Chi, Y.-T. (2010). Production of Biosurfactant Lipopeptides Iturin A, Fengycin and Surfactin A from Bacillus Subtilis CMB32 for Control of Colletotrichum Gloeosporioides. J. Microbiol. Biotechnol. 20, 138–145. doi:10.4014/jmb.0905.05007
Kolar, S. S. N., Luca, V., Baidouri, H., Mannino, G., McDermott, A. M., and Mangoni, M. L. (2015). Esculentin-1a(1-21)NH2: a Frog Skin-Derived Peptide for Microbial Keratitis. Cell Mol. Life Sci. 72, 617–627. doi:10.1007/s00018-014-1694-0
Koo, J. C., Lee, S. Y., Chun, H. J., Cheong, Y. H., Choi, J. S., Kawabata, S., et al. (1998). Lee, B.l., Cho, M. JTwo Hevein Homologs Isolated from the Seed of Pharbitis Nil L Exhibit Potent Antifungal Activity. Biochim. Biophys. Acta 1382, 80–90. doi:10.1016/s0167-4838(97)00148-9
Kragol, G., Hoffmann, R., Chattergoon, M. A., Lovas, S., Cudic, M., Bulet, P., et al. (2002). Identification of Crucial Residues for the Antibacterial Activity of the Proline-Rich Peptide, Pyrrhocoricin. Eur. J. Biochem. 269, 4226–4237. doi:10.1046/j.1432-1033.2002.03119.x
Kragol, G., Lovas, S., Varadi, G., Condie, B. A., Hoffmann, R., and Otvos, L. (2001). The Antibacterial Peptide Pyrrhocoricin Inhibits the ATPase Actions of DnaK and Prevents Chaperone-Assisted Protein Folding. Biochemistry 40, 3016–3026. doi:10.1021/bi002656a
Krause, A., Neitz, S., Magert, H-J., Axel Schulz, A., Wolf-Georg Forssmann, W-G., Schulz-Knappe, P., et al. (2000). LEAP-1, a Novel Highly Disulfide-Bonded Human Peptide, Exhibits Antimicrobial Activity. FEBS Lett. 480, 147–150. doi:10.1016/S0014-5793(00)01920-7
Krause, A., Sillard, R., Kleemeier, B., Klüver, E., Maronde, E., Conejo-García, J. R., et al. (2003). Isolation and Biochemical Characterization of LEAP-2, a Novel Blood Peptide Expressed in the Liver. Protein Sci. 12, 143–152. doi:10.1110/ps.0213603
Kul'ko, A. B., Kisl, O. V., Sadykova, V. S., Mikhailov, V. F., Vasilieva, I. M., Shulenina, L. V., et al. (2016). Investigation of Thionins from Black Cumin (Nigella Sativa L.) Seeds Showing Cytotoxic, Regulatory and Antifungal Activity. Antibiot. Khimioter 61, 8–16.
Lai, R., Liu, H., Hui Lee, W., and Zhang, Y. (2002). An Anionic Antimicrobial Peptide from Toad Bombina Maxima. Biochem. Biophys. Res. Commun. 295, 796–799. doi:10.1016/s0006-291x(02)00762-3
Lakshminarayanan, R., Liu, S., Li, J., Nandhakumar, M., Aung, T. T., Goh, E., et al. (2014). Synthetic Multivalent Antifungal Peptides Effective against Fungi. PLoS One. 9, e87730. doi:10.1371/journal.pone.0087730
Lalatsa, A., Schatzlein, A. G., and Uchegbu, I. F. (2014). Strategies to Deliver Peptide Drugs to the Brain. Mol. Pharm. 2014 (11), 1081–1093. doi:10.1021/mp400680d
Lavermicocca, P., Sante Iacobellis, N., Simmaco, M., and Graniti, A. (1997). Biological Properties and Spectrum of Activity of Pseudomonas syringae Pv. Syringae Toxins. Physiol. Mol. Plant Pathol. 50, 129–140. doi:10.1006/pmpp.1996.0078
Lawyer, C., Pai, S., Watabe, M., Borgia, P., Mashimo, T., Eagleton, L., et al. (1996). Antimicrobial Activity of a 13 Amino Acid Tryptophan-Rich Peptide Derived from a Putative Porcine Precursor Protein of a Novel Family of Antibacterial Peptides. FEBS Lett. 390, 95–98. doi:10.1016/0014-5793(96)00637-0
Leclère, V., Béchet, M., Adam, A., Guez, J.-S., Wathelet, B., Ongena, M., et al. (2005). Mycosubtilin Overproduction by Bacillus Subtilis BBG100 Enhances the Organism’s Antagonistic and Biocontrol Activities. Appl. Environ. Microbiol. 71, 4577–4584. doi:10.1128/AEM.71.8.4577-4584.2005
Lee, D. G., Kim, P. I., Park, Y., Woo, E. R., Choi, J. S., Choi, C. H., et al. (2002). Design of Novel Peptide Analogs with Potent Fungicidal Activity, Based on PMAP-23 Antimicrobial Peptide Isolated from Porcine Myeloid. Biochem. Biophys. Res. Commun. 293, 231–238. doi:10.1016/s0006-291x(02)00222-x
Lee, D. W., and Kim, B. S. (2015). Antimicrobial Cyclic Peptides for Plant Disease Control. Plant Pathol. J. 31, 1–11. doi:10.5423/PPJ.RW.08.2014.0074
Lee, G., and Bae, H. (2016). Anti-Inflammatory Applications of Melittin, a Major Component of Bee Venom: Detailed Mechanism of Action and Adverse Effects. Molecules 21, 616. doi:10.3390/molecules21050616
Lee, J., and Lee, D. G. (2014). Melittin Triggers Apoptosis in Candida Albicans through the Reactive Oxygen Species-Mediated Mitochondria/caspase Dependent Pathway. FEMS Microbiol. Lett. 355, 36–42. doi:10.1111/1574-6968.12450
Lee, M. Y., Park, S.-C., Jung, M., Shin, M.-K., Kang, H-L., Baik, S-C., et al. (2018). Cell-selectivity of Tryptophan and Tyrosine in Amphiphilic α-Helical Antimicrobial Peptides against Drug-Resistant Bacteria. Biochem. Biophys. Res. Commun. 505, 478–484. doi:10.1016/j.bbrc.2018.09.095
Lee, S. H., Cho, Y. E., Park, S.-H., Balaraju, K., Park, J. W., Lee, S. W., et al. (2013). An Antibiotic Fusaricidin: a Cyclic Depsipeptide from Paenibacillus Polymyxa E681 Induces Systemic Resistance against Phytophthora Blight of Red-Pepper. Phytoparasitica 41, 49–58. doi:10.1007/s12600-012-0263-z
Leenders, F., Stein, T. H., Kablitz, B., Franke, P., and Vater, J. (1999). Rapid Typing of Bacillus Subtilis Strains by Their Sec- Ondary Metabolites Using Matrix-Assisted Laser Desorption/Ionization Mass Spectrometry of Intact Cells. Rapid Commun. Mass. Spectrom. 13, 943–949. doi:10.1002/(SICI)1097-0231(19990530)13:10<943::AID-RCM591>3.0.CO;2–0
Levashina, E. A., Ohresser, S., Bulet, P., Reichhart, J. M., Hetru, C., and Hoffmann, J. A. (1995). Metchnikowin, a Novel Immune-Inducible Proline-Rich Peptide from Drosophila with Antibacterial and Antifungal Properties. Eur. J. Biochem. 233, 694–700. doi:10.1111/j.1432-1033.1995.694_2.x
Levashina, E. A., Ohresser, S., Lemaitre, B., and Imler, J. L. (1998). Two Distinct Pathways Can Control Expression of the Gene Encoding the Drosophila Antimicrobial Peptide Metchnikowin. J. Mol. Biol. 278, 515–527. doi:10.1006/jmbi.1998.1705
Li, S. S., and Claeson, P. (2003). Cys/Gly-rich Proteins with a Putative Single Chitin-Binding Domain from Oat (Avena Sativa) Seeds. Phytochemistry 63, 249–255. doi:10.1016/s0031-9422(03)00116-x
Lim, T. H., Kwon, S. Y., Seo, H. W., Min, B. S., and Lim, C. H. (2007). Antifungal Activity of Valinomycin, a Cyclo Depsipeptide from Streptomyces Padanus TH-04. Nat. Prod. Sci. 13, 144–147.
Liu, D., and DeGrado, W. F. (2001). De Novo design, Synthesis, and Characterization of Antimicrobial β-peptides. J. Am. Chem. Soc. 123, 7553–7559. doi:10.1021/ja0107475
Liu, G., Kang, D., and Steiner, H. (2000). Trichoplusia Ni Lebocin, an Inducible Immune Gene with a Downstream Insertion Element. Biochem. Biophys. Res. Commun. 269, 803–807. doi:10.1006/bbrc.2000.2366
Liu, L., Xu, K., Wang, H., Tan, J., Fan, W., Venkatraman, S. S., et al. (2009). Self-assembled Cationic Peptide Nanoparticles as an Efficient Antimicrobial Agent. Nat. Nanotechnol. 4, 457–463. doi:10.1038/nnano.2009.153
Liu, L., Yang, J., Xie, J., Luo, Z., Jiang, J., Yang, Y. Y., et al. (2013). The Potent Antimicrobial Properties of Cell Penetrating Peptide-Conjugated Silver Nanoparticles with Excellent Selectivity for Gram-Positive Bacteria over Erythrocytes. Nanoscale 5, 3834–3840. doi:10.1039/C3NR34254A
Liu, Z., Brady, A., Young, A., Rasimick, B., Chen, K., Zhou, C., et al. (2007). Length Effects in Antimicrobial Peptides of the (RW)n Series. Antimicrob. Agents Chemother. 51, 597–603. doi:10.1128/AAC.00828-06
Lopez-Garcia, B., Gonzalez-Candelas, L., Perez-Paya, E., and Marcos, J. F. (2000). Identification and Characterization of a Hexapeptide with Activity against Phytopathogenic Fungi that Cause Postharvest Decay in Fruits. Mol. Plant-microbe Interact. 13, 837–846. doi:10.1094/MPMI.2000.13.8.837
Lopez-García, B., Pérez-Payá, E., and Marcos, J. F. (2002). Identification of Novel Hexapeptides Bioactive against Phytopathogenic Fungi through Screening of a Synthetic Peptide Combinatorial Library. Appl. Environ. Microbiol. 68, 2453–2460. doi:10.1128/AEM.68.5.2453–2460.2002,
Lu, J., Xu, H., Xia, J., Ma, J., Xu, J., Li, Y., et al. (2020). D- and Unnatural Amino Acid Substituted Antimicrobial Peptides with Improved Proteolytic Resistance and Their Proteolytic Degradation Characteristics. Front. Microbiol. 11, 563030. doi:10.3389/fmicb.2020.563030
Lum, K. Y., Tay, S. T., Le, C. F., Lee, V. S., Sabri, N. H., Velayuthan, R. D., et al. (2015). Activity of Novel Synthetic Peptides against. Candida Albicans. Sci. Rep. 5, 9657–9669. doi:10.1038/srep09657
Maget-Dana, R., and Peypoux, F. (1994). Iturins, a Special Class of Pore-Forming Lipopeptides: Biological and Physicochemical Properties. Toxicology 87, 151–174. doi:10.1016/0300483x(94)90159-7
Maleki, H., Rai, A., Pinto, S., Evangalista, M., Cardosa, R. M. S., Paulo, C., et al. (2016). High Antimicrobial Activity and Low Human Cell Cytotoxicity of Core–Shell Magnetic Nanoparticles Functionalized with an Antimicrobial Peptide. ACS Appl. Mater. Inter. 8, 11366–11378. doi:10.1021/acsami.6b03355
Manchenko, G. P. (1994). Lysozyme. Handbook of Detection of Enzymes on Electrophoretic Gels. Boca Raton, Fla: CRC Press, 223.
Mangoni, M. L. (2006). Temporins, Anti-infective Peptides with Expanding Properties. Cell. Mol. Life Sci. 63, 1060–1069. doi:10.1007/s00018-005-5536-y
Mangoni, M. L., Fiocco, D., Mignogna, G., Barra, D., and Simmaco, M. (2003). Functional Characterisation of the 1–18 Fragment of Esculentin-1b, an Antimicrobial Peptide from. Rana esculenta. Peptides 24, 1771–1777. doi:10.1016/j.peptides.2003.07.029
Mangoni, M. L., Marcellini, H. G. L., and Simmaco, M. (2007). Biological Characterization and Modes of Action of Temporins and Bombinins H, Multiple Forms of Short and Mildly Cationic Anti-microbial Peptides from Amphibian Skin. J. Pept. Sci. 13, 603–613. doi:10.1002/psc.853
Mangoni, M. L., Papo, N., Mignogna, G., Andreu, D., Shai, Y., Barra, D., et al. (2003b). Ranacyclins, a New Family of Short Cyclic Antimicrobial Peptides: Biological Function, Mode of Action, and Parameters Involved in Target Specificity. Biochemistry 42, 14023–14035. doi:10.1021/bi034521l
Mangoni, M. L., Papo, N., Saugar, J. M., Barra, D., Shai, Y., Simmaco, M., et al. (2006). Effect of Natural L- to D-Amino Acid Conversion on the Organization, Membrane Binding, and Biological Function of the Antimicrobial Peptides Bombinins H. Biochemistry 45, 4266–4276. doi:10.1021/bi052150y
Mangoni, M. L., Saugar, J. M., Dellisanti, M., Barra, D., Simmaco, M., and Rivas, L. (2005). Temporins, Small Antimicrobial Peptides with Leishmanicidal Activity. J. Biol. Chem. 280, 984–990. doi:10.1074/jbc.M410795200
Mantyla, T., Sirola, H., Kansanen, E., Korjamo, T., Lankinen, H., LAppalainen, K., et al. (2005). Effect of Temporin A Modifications on its Cytotoxicity and Antimicrobial Activity. APMIS 113, 497–505. doi:10.1111/j.1600-0463.2005.apm_107.x
Mao, C., Mohanraj, G., Kandiyote, N. S., Kasher, R., and Arnusch, C. J. (2018). UV Mediated Attachment of Short Arginine-Tryptophan Antimicrobial Peptides on Reverse Osmosis Membrane Surfaces Inhibit Pseudomonas aeruginosa Biofilm. Desalination 431, 73–79. doi:10.1016/j.desal.2017.12.027
Mardirossian, M., Barriere, Q., Timchenko, T., Muller, C., Pacor, S., Margaert, P., et al. (2018). Fragments of the Nonlytic Proline-Rich Antimicrobial Peptide Bac5 Kill Escherichia coli Cells by Inhibiting Protein Synthesis. Antimicrob. Agents Chemother. 62, e00534–18. doi:10.1128/AAC.00534-18
Mathur, S., and Singh, R. (2005). Antibiotic Resistance in Food Lactic Acid Bacteria—A Review. Int. J. Food Microbiol. 105, 281–295. doi:10.1016/j.ijfoodmicro.2005.03.008
McCloskey, A. P., Draper, E. R., Gilmore, B. F., and Laverty, G. (2017). Ultrashort Self-Assembling Fmoc-Peptide Gelators for Anti-infective Biomaterial Applications. J. Pept. Sci. 23, 131–140. doi:10.1002/psc.2951
McManus, A. M., Otvos, L., Hoffmann, R., and Craik, D. J. (1999). Conformational Studies by NMR of the Antimicrobial Peptide, Drosocin, and its Non-glycosylated Derivative: Effects of Glycosylation on Solution Conformation. Biochemistry 38, 705–714. doi:10.1021/bi981956d
Melchionna, M., Styan, K., and Marchesan, S. (2016). The Unexpected Advantages of Using D- Amino Acids for Peptide Self-Assembly into Nanostructured Hydrogels for Medicine. Curr. Top. Med. Chem. 16, 2009–2018. doi:10.2174/1568026616999160212120302
Mishra, A. K., Choi, J., Moon, E., and Baek, K-H. (2018). Tryptophan-rich and Proline-Rich Antimicrobial Peptides. Molecules 23, 815. doi:10.3390/molecules23040815
Miyoshi, N., Isogai, E., Hiramatsu, K., and Sasaki, T. (2017). Activity of Tick Antimicrobial Peptide from Ixodes Persulcatus (Persulcatusin) against Cell Membranes of Drug-Resistant. Staphylococcus aureus. J. Antibiot. 70, 142–146. doi:10.1038/ja.2016.101
Miyoshi, N., Saito, T., Ohmura, T., Kuroda, K., Suita, K., Ihara, K., et al. (2016). Functional Structure and Antimicrobial Activity of Persulcatusin, an Antimicrobial Peptide from the Hard Tick. Ixodes Persulcatus. Parasites Vectors 9, 85. doi:10.1186/s13071-016-1360-5
Mizuhara, N., Kuroda, M., Ogita, A., Tanaka, T., Usuki, Y., and Fujita, K.-I. (2011). Antifungal Thiopeptide Cyclothiazomycin B1 Exhibits Growth Inhibition Accompanying Morphological Changes via Binding to Fungal Cell wall Chitin. Bioorg. Med. Chem. 19, 5300–5310. doi:10.1016/j.bmc.2011.08.010
Mookherjee, N., Anderson, M. A., Haagsman, H. P., and Davidson, D. J. (2020). Antimicrobial Host Defence Peptides: Functions and Clinical Potential. Nat. Rev. Drug Discov. 19, 311–332. doi:10.1038/s41573-019-0058-8
Moorcroft, S. C. T., Roach, L., Jayne, D. G., Ong, Z. Y., and Evans, S. D. (2020). Nanoparticle-loaded Hydrogel for the Light-Activated Release and Photothermal Enhancement of Antimicrobial Peptides. ACS Appl. Mater. Inter. 12, 24544–24554. doi:10.1021/acsami.9b22587
Moore, A. J., Beazley, W. D., Bibby, M. C., and Devine, D. A. (1996). Antimicrobial Activity of Cecropins. J. Antimicrobiol. Chemother. 37, 1077–1089. doi:10.1093/jac/37.6.1077
Motiei, L., Rahimipour, S., Thayer, D. A., Wong, C. H., and Ghadiri, M. R. (2009). Antibacterial Cyclic D, L-α-Glycopeptides. Chem. Commun. 49, 3693–3695. doi:10.1039/B902455G
Muñoz, A., López-García, B., and Marcos, J. F. (2006). Studies on the Mode of Action of the Antifungal Hexapeptide PAF26. Antimicrob. Agents Chemother. 50, 3847–3855. doi:10.1128/AAC.00650-06
Murakami, M., Ohtake, T., Dorschner, R. A., Schittek, B., Garbe, C., and Gallo, R. L. (2002). Cathelicidin Antimicrobial Peptide Expression in Sweat, an Innate Defense System for the Skin. J. Invest. Dermatol. 119, 1090–1095. doi:10.1046/j.1523-1747.2002.19507.x
Murray, T., Leighton, F. C., and Seddon, B. (1986). Inhibition of Fungal Spore Germination by Gramicidin S and its Potential Use as a Biocontrol against Fungal Plant Pathogens. Lett. Appl. Microbiol. 3, 5–7. doi:10.1111/j.1472-765X.1986.tb01534.x
Mygind, P. H., Fischer, R. L., Schnorr, K. M., Hansen, M. T., Sonksen, C. P., Ludvigsen, S., et al. (2005). Plectasin Is a Peptide Antibiotic with Therapeutic Potential from a Saprophytic Fungus. Nature 437, 975–980. doi:10.1038/nature04051
Mylonakis, E., Podsiadlowski, L., Muhammed, M., and Vilcinskas, A. (2016). Diversity, Evolution and Medical Applications of Insect Antimicrobial Peptides. Philos. Trans. R. Soc. Lond. B Biol. Sci. 371, 20150290. doi:10.1098/rstb.2015.0290
Nandi, N., Gayen, K., Ghosh, S., Bhunia, D., Kirkham, S., Sen, S. K., et al. (2017). Amphiphilic Peptide-Based Supramolecular, Non-cytotoxic, Stimuli-Responsive Hydrogels with Antibacterial Activity. Biomacromolecules 18, 3621–3629. doi:10.1021/acs.biomac.7b01006
Nawrot, R., Barylski, J., Nowicki, G., Broniarczyk, J., Buchwald, W., and Goździcka-Józefiak, A. (2014). Plant Antimicrobial Peptides. Folia Microbiol. 59, 181–196. doi:10.1007/s12223-013-0280-4
Ngai, P. H., and Ng, T. B. (2005). Phaseococcin, an Antifungal Protein with Antiproliferative and Anti-HIV-1 Reverse Transcriptase Activities from Small Scarlet Runner Beans. Biochem. Cel Biol. 83, 212–220. doi:10.1139/o05-037
Nicolas, P. (2009). Multifunctional Host Defense Peptides: Intracellular Targeting Antimicrobial Peptides. FEBS J. 276, 6483–6496. doi:10.1111/j.1742-4658.2009.07359.x
Nielsen, T. H., Christophersen, C., Anthoni, U., and Sorensen, J. (1999). Viscosinamide, a New Cyclic Depsipeptide with Surfactant and Antifungal Properties Produced by Pseudomonas Fluorescens DR54. J. Appl. Microbiol. 87, 80–90. doi:10.1046/j.1365-2672.1999.00798.x
Nielsen, T. H., Sorensen, D., Tobiasen, C., Andersen, J. B., Christophersen, C., Givskov, M., and Sorensen, J. (2002). Antibiotic and Biosurfactant Properties of Cyclic Lipopeptides Produced by Fluorescent Pseudomonas Spp. from the Sugar Beet RhizoSphere. Appl. Environ. Microbiol. 68, 3416–3423. doi:10.1128/aem.68.7.3416-3423.2002
Nielsen, T. H., and Sorensen, J. (2003). Production of Cyclic Lipo- Peptides by Pseudomonas Uorescens Strains in Bulk Soil and in the Sugar Beet Rhizosphere. Appl. Environ. Microbiol. 69, 861–868. doi:10.1128/AEM.69.2.861-868.2003
Nielsen, T. H., Thrane, C., Christophersen, C., Anthoni, U., and Sørensen, J. (2000). Structure, Production Characteristics and Fungal Antagonism of Tensin—A New Antifungal Cyclic Lipopeptide from Pseudomonas Fluorescens Strain 96.578. J. Appl. Microbiol. 89, 992–1001. doi:10.1046/j.1365-2672.2000.01201.x
Nikawa, N., Fukushima, H., Makihira, S., Hamada, T., and Samaranayake, L. P. (2004). Fungicidal Effect of Three New Synthetic Cationic Peptides against. Candida Albicans. Oral Dis. 10, 221–228. doi:10.1111/j.1601-0825.2004.01010.x
Niu, Y., Padhee, S., Wu, H., Bai, G., Qiao, Q., Hu, Y., et al. (2012). Lipo-γ-AA Peptides as a New Class of Potent and Broad-Spectrum Antimicrobial Agents. J. Med. Chem. 55, 4003–4009. doi:10.1021/jm300274p
Oh, D., Sun, J., Shirazi, A. N., LaPlante, K. L., Rowley, D. C., and Parang, K. (2014). Activities of Amphiphilic Cyclic Cell-Penetrating Peptides against Multidrug-Resistant Pathogens. Mol. Pharm. 11, 3528–3536. doi:10.1021/mp5003027
Ongena, M., Jacques, P., Tour, Y., Destain, J., Jabrane, A., and Thonart, P. (2005). Involvement of Fengycin-type Lipopeptides in the Multifaceted Biocontrol Potential of. Bacillus Subtilis. Appl. Microbiol. Biotechnol. 69, 29–38. doi:10.1007/s00253-005-1940-3
Oppenheim, F. G., Xu, T., McMillian, F. M., Levitz, S. M., Diamond, R. D., Offner, G. D., et al. (1988). Histatins, a Novel Family of Histidine-Rich Proteins in Human Parotid Secretion. Isolation, Characterization, Primary Structure, and Fungistatic Effects on. Candida Albicans. J. Biol. Chem. 263, 7472–7477. doi:10.1016/S0021-9258(18)68522-9
Osada, Y., and Kajiwara, K. ed. (2001). Gels Hand Book, assoc. ed. T. Fushimi, O. Hirasa, Y. Hirokawa, T. Matsunaga, T. Shimomura, and L. Wang, Vols. 1–3, Academic Press, San Diego.
Otari, S. V., Patel, S. K. S., Jeong, J. H., Lee, J. H., and Lee, J. K. (2016). A green Chemistry Approach for Synthesizing Thermostable Antimicrobial Peptide-Coated Gold Nanoparticles Immobilized in an Alginate Biohydrogel. RSC Adv. 6, 86808–86816. doi:10.1039/C6RA14688K
Ouyang, L., Xu, X., Freed, S., Gao, Y., Yu, J., Wang, S., et al. (2015). Cecropins from Plutella Xylostella and Their Interaction with Metarhizium Anisopliae. PLoS ONE 10, e0142451. doi:10.1371/journal.pone.0142451
Pal, I., Bhattacharyya, D., Kar, R. K., Zarena, D., Bhunia, A., and Atreya, H. S. (2019). A Peptide-Nanoparticle System with Improved Efficacy against Multidrug Resistant Bacteria. Sci. Rep. 14, 4485. doi:10.1038/s41598-019-41005-7
Pal, I., Brahmkhatri, V. P., Bera, S., Bhattacharyya, D., Quirishi, Y., Bhunia, A., et al. (2016). Enhanced Stability and Activity of an Antimicrobial Peptide in Conjugation with Silver Nanoparticle. J. Colloid Interf. Sci. 483, 385–393. doi:10.1016/j.jcis.2016.08.043
Pandit, G., Biswas, K., Ghosh, S., Debnath, S., Bidkar, A. P., Satpati, P., et al. (2020). Rationally Designed Antimicrobial Peptides: Insight into the Mechanism of Eleven Residue Peptides against Microbial Infections. Biochim. Biophys. Acta Biomembr. 1862, 183177–183193. doi:10.1016/j.bbamem.2020.183177
Pandit, G., Ilyas, H., Ghosh, S., Bidkar, A. P., Mohid, Sk. A., Bhunia, A., et al. (2018). Insights into the Mechanism of Antimicrobial Activity of Seven- Residue Peptides. J. Med. Chem. 61, 7614–7629. doi:10.1021/acs.jmedchem.8b00353
Pandit, G., Chowdhury, N., Mohid, S. A., Bidkar, A. P., Bhunia, A., and Chatterjee, A. (2021). Effect of Secondary Structure and Side Chain Length of Hydrophobic Amino Acid Residues on the Antimicrobial Activity and Toxicity of 14-Residue-Long de novo AMPs. ChemMedChem. 16, 355–367. doi:10.1002/cmdc.202000550
Papo, N., and Shai, Y. (2003). Can We Predict Biological Activity of Antimicrobial Peptides from Their Interactions with Model Phospholipid Membranes?. Peptides 24, 1693–1703. doi:10.1016/j.peptides.2003.09.013
Park, C. B., Kim, H. S., and Kim, S. C. (1998). Mechanism of Action of the Antimicrobial Peptide Buforin II: Buforin II Kills Microorganisms by Penetrating the Cell Membrane and Inhibiting Cellular Functions. Biochem. Biophys. Res. Commun. 244, 253–257. doi:10.1006/bbrc.1998.8159
Park, C. B., Kim, M. S., and Kim, S. C. (1996). A Novel Antimicrobial Peptide from Bufo bufo Gargarizans. Biochem. Biophys. Res. Comm. 218, 408–413. doi:10.1006/bbrc.1996.0071
Park, C. B., Yi, K.-S., Matsuzaki, K., Kim, M. S., and Kim, S. C. (2000). Structure–activity Analysis of Buforin II, a Histone H2A-Derived Antimicrobial Peptide: The Proline Hinge Is Responsible for the Cell-Penetrating Ability of Buforin II. PNAS 97, 8245–5820. doi:10.1073/pnas.150518097
Park, C. H., Valore, E. V., Waring, A. J., and Ganz, T. (2001a). Hepcidin, a Urinary Antimicrobial Peptide Synthesized in the Liver. J. Biol. Chem. 276, 7806–7801. doi:10.1074/jbc.M008922200
Park, C. N., Lee, J. M., Lee, D., and Kim, B. S. (2008). Antifungal Activity of Valinomycin, a Peptide Antibiotic Produced by Streptomyces Sp. Strain M10 Antagonistic Botrytis Cinerea. J. Microbiol. Biotechnol. 18, 880–884.
Park, S., Park, S. H., Ahna, H. C., Kimb, S., Kimb, S. S., Lee, B. J., et al. (2001). Structural Study of Novel Antimicrobial Peptides, Nigrocins, Isolated from. Rana nigromaculata. FEBS Lett. 507, 95–100. doi:10.1016/s0014-5793(01)02956-8
Pasupuleti, M., Chalupka, A., Mörgelin, M., Schmidtchen, A., and Malmsten, M. (2009). Tryptophan End-Tagging of Antimicrobial Peptides for Increased Potency against Pseudomonas aeruginosa. Biochim. Biophys. Acta 1790, 800–808. doi:10.1016/j.bbagen.2009.03.029
Patocka, J., Nepovimova, E., Klimova, B., Wu, Q., and Kuca, K. (2019). Antimicrobial Peptides: Amphibian Host Defense Peptides. Curr. Med. Chem. 26, 5924–5946. doi:10.2174/0929867325666180713125314
Pazos, E., Sleep, E., Pérez, C. M. R., Lee, S. S., Tantakitti, F., and Stupp, S. I. (2016). Nucleation and Growth of Ordered Arrays of Silver Nanoparticles on Peptide Nanofibers: Hybrid Nanostructures with Antimicrobial Properties. J. Am. Chem. Soc. 138, 5507–5510. doi:10.1021/jacs.6b01570
Pedras, M. S. C., Ismail, N., Quail, J. W., and Boyetchko, S. M. (2003). Structure, Chemistry, and Biological Activity of Pseudophomins A and B, New Cyclic Lipodepsipeptides Isolated from the Biocontrol Bacterium. Pseudomonas Fluorescens. Phytochemistry 62, 1105–1114. doi:10.1016/s0031-9422(02)00617-9
Pelegrini, P. B., Quirino, B. F., and Franco, O. L. (2007). Plant Cyclotides: an Unusual Class of Defense Compounds. Peptides 28, 1475–1481. doi:10.1016/j.peptides.2007.04.025
Pellegrini, A., Thomas, U., Bramaz, N., Klauser, S., Hunziker, P., and Von Fellenberg, R. (1997). Identification and Isolation of a Bactericidal Domain in Chicken Egg white Lysozyme. J. Appl. Microbiol. 82, 372–378. doi:10.1046/j.1365-2672.1997.00372.x
Peypoux, F., Besson, F., Michel, G., and Delcambe, L. (1981). Structure of Bacillomycin D, a New Antibiotic of the Iturin Group. Eur. J. Biochem./FEBS 118, 323–327. doi:10.1111/j.1432-1033.1981.tb06405.x
Peypoux, F., Bonmatin, J., and Wallach, J. (1999). Recent Trends in the Biochemistry of Surfactin. Appl. Microbiol. Biotechnol. 51, 553–563. doi:10.1007/s002530051432
Phillips, R. L., Palombo, E. A., Panozzo, J. F., and Bhave, M. (2011). Puroindolines, Pin Alleles, Purodoindolines and Grain Softness Proteins Are Sources of Bactericidal and Fungicidal Peptides. J. Cereal Sci. 53, 112–117. doi:10.1016/j.jcs.2010.10.005
Pigeon, C., Ilyin, G., Courselaud, B., Leroyer, P., Turlin, B., Brissot, P., et al. (2001). A New Mouse Liver-specific Gene, Encoding a Protein Homologous to Human Antimicrobial Peptide Hepcidin, Is Overexpressed during Iron Overload. J. Biol. Chem. 276, 7811–7819. doi:10.1074/jbc.M008923200
Ponti, D., Mignogna, G., Mangoni, M. L., De Biase, D., Simmaco, M., and Barra, D. (1999). Expression and Activity of Cyclic and Linear Analogues of Esculentin-1, an Anti-microbial Peptide from Amphibian Skin. Eur. J. Biochem. 263, 921–927. doi:10.1046/j.1432-1327.1999.00597.x
Porto, W. F., Souza, V. A., Nolasco, D. O., and Franco, O. L. (2012). In Silico identification of Novel Hevein-like Peptide Precursors. Peptides 38, 127–136. doi:10.1016/j.peptides.2012.07.025
Powers, J.-P. S., and Hancock, R. E. W. (2003). The Relationship between Peptide Structure and Antibacterial Activity. Peptides 24, 1681–1691. doi:10.1016/j.peptides.2003.08.023
Pulido, D., Torrent, M., Andreu, D., Nogués, M. V., and Boix, E. (2013). Two Human Host Defense Ribonucleases against Mycobacteria, the Eosinophil Cationic Protein (RNase 3) and RNase 7. Antimicrob. Agents Chemother. 57, 3797–3805. doi:10.1128/AAC.00428-13
Rabanal, F., and Cajal, Y. (2016). “Therapeutic Potential of Antimicrobial peptidesSpringer International Publishing: Cham,” in New Weapons to Control Bacterial Growth. Editors T. G. Villa, and M. Vinas, 433–451.Switzerland
Rady, I., Siddiqui, I. A., Rady, M., and Mukhtar, H. (2017). Melittin, a Major Peptide Component of Bee Venom, and its Conjugates in Cancer Therapy. Cancer Lett. 402, 16–31. doi:10.1016/j.canlet.2017.05.010
Raghunath, A., and Perumal, E. (2017). Metal Oxide Nanoparticles as Antimicrobial Agents: a Promise for the Future. Int. J. Antimicrob. Agents 49, 137–152. doi:10.1016/j.ijantimicag.2016.11.011
Rajchakit, U., and Sarojini, V. (2017). Recent Developments in Antimicrobial-Peptide-Conjugated Gold Nanoparticles. Bioconjug. Chem. 2017 (28), 2673–2686. doi:10.1021/acs.bioconjchem.7b00368
Reed, J. D., Edwards, D. L., and Gonzalez, C. F. (1997). Synthetic Peptide Combinatorial Libraries: a Method for the Identification of Bioactive Peptides against Phytopathogenic Fungi. Mol. Plant Microbe Interact. 10, 537–549. doi:10.1094/MPMI1997.10.5.537
Robinson, W. E., McDougall, B., Tran, D., and Selsted, M. E. (1998). Anti-HIV-1activity of Indolicidin, an Antimicrobial Peptide from Neutrophils. J. Leukoc. Biol. 63, 94–100. doi:10.1002/jlb.63.1.94
Rosengren, K. J., Göransson, U., Otvos, L., and Craik, D. J. (2004). Cyclization of Pyrrhocoricin Retains Structural Elements Crucial for the Antimicrobial Activity of the Native Peptide. Biopolymers 76, 446–458. doi:10.1002/bip.20159
Roskoski, R., Gevers, W., Kleinkauf, H., and Lipmann, F. (1970). Tyrocidine Biosynthesis by Three Complementary Fractions from Bacillus Brevis (ATCC 8185). Biochemistry 9, 4839–4845. doi:10.1021/bi00827a002
Rozek, A., Powers, J-P. S., Friedrich, C. L., and Hancock, R. E. W. (2003). Structure-Based Design of an Indolicidin Peptide Analogue with Increased Protease Stability. Biochemistry 42, 14130–14138. doi:10.1021/bi035643g
Runti, G., Benincasa, M., Giuffrida, G., Devescovi, G., Venturi, V., Gennaro, R., et al. (2017). The Mechanism of Killing by the Proline-Rich Peptide Bac7(1–35) against Clinical Strains of Pseudomonas aeruginosa Differs from that against Other Gram-Negative Bacteria. Antimicrob. Agents Chemother. 61, e01660–16. doi:10.1128/AAC.01660-16
Runti, G., Lopez Ruiz, M. D. C., Stoilova, T., Hussain, R., Jennions, M., Choudhury, H. G., et al. (2013). Functional Characterization of SbmA, a Bacterial Inner Membrane Transporter Required for Importing the Antimicrobial Peptide Bac7(1-35). J. Bacteriol. 195, 5343–5351. doi:10.1128/JB.00818-13
Saito, Y., Konnai, S., Yamada, S., Imamura, S., Nishikado, H., Ito, T., et al. (2009). Identification and Characterization of Antimicrobial Peptide, Defensin, in the Taiga Tick. Ixodes Persulcatus. Insect Mol. Biol. 18, 531–539. doi:10.1111/j.1365-2583.2009.00897.x
Salick, D. A., Kretsinger, J. K., Pochan, D. J., and Schneider, J. P. (2007). Inherent Antibacterial Activity of a Peptide-Based Beta-Hairpin Hydrogel. J. Am. Chem. Soc. 129, 14793–14799. doi:10.1021/ja076300z
Salick, D. A., Pochan, D. J., and Schneider, J. P. (2009). Design of an Injectable β-hairpin Peptide Hydrogel that Kills Methicillin-Resistant Staphylococcus aureus. Adv. Mater. 21, 4120–4123. doi:10.1002/adma.200900189
Sarkar, B., Siddiqui, Z., Nguyen, P. K., Namita Dube, N., Fu, W., et al. (2019). Membrane-disrupting Nanofibrous Peptide Hydrogels. ACS Biomater. Sci. Eng. 5, 4657–4670. doi:10.1021/acsbiomaterials.9b0096
Satomi, T., Kusakabe, H., Nakamura, G., Nishio, T., Uramoto, M., and Isono, K. (1982). Neopeptins A and B, New Antifungal Antibiotics. Agric. Biol. Chem. 46, 2621–2623. doi:10.1080/00021369.1982.10865486
Schauber, J., and Gallo, R. L. (2008). Antimicrobial Peptides and the Skin Immune Defense System. J. Allergy Clin. Immunol. 122, 261–266. doi:10.1016/j.jaci.2008.03.027
Schibli, D. J., Epand, R. F., Vogel, H. J., and Epand, R. M. (2002). Tryptophan-rich Antimicrobial Peptides: Comparative Properties and Membrane Interactions. Biochem. Cel Biol. 80, 667–677. doi:10.1139/o02-147
Schmidtche, A., Pasupuleti, M., and Malmsten, M. (2014). Effect of Hydrophobic Modifications in Antimicrobial Peptides. Adv. Colloid Interf. Sci. 205, 265–274. doi:10.1016/j.cis.2013.06.009
Schnaider, L., Brahmachari, S., Schmidt, N. W., Mensa, B., Shaham-Niv, S., Bychenko, D., et al. (2017). Self-assembling Dipeptide Antibacterial Nanostructures with Membrane Disrupting Activity. Nat. Commun. 8, 1365. doi:10.1038/s41467-017-01447-x
Scocchi, M., Mardirossian, M., Runti, G., and Benincasa, M. (2016). Non-membrane Permeabilizing Modes of Action of Antimicrobial Peptides on Bacteria. Curr. Top. Med. Chem. 16, 76–88. doi:10.2174/1568026615666150703121009
Scott, M. G., Davidson, D. J., Gold, M. R., Bowdish, D., and Hancock, R. E. W. (2002). The Human Antimicrobial Peptide Ll-37 Is a Multifunctional Modulator of Innate Immune Responses. J. Immunol. 169, 3883–3891. doi:10.4049/jimmunol.169.7.3883
Segura, A., Moreno, M., Molina, A., and García-Olmedo, F. (1998). Novel Defensin Subfamily from Spinach (Spinacia Oleracea). FEBS Lett. 435, 159–162. doi:10.1016/s0014-5793(98)01060-6
Selim, S., Negrel, J., Govaerts, C., Gianinazzi, S., and van Tuinen, D. (2005). Isolation and Partial Characterization of Antagonistic Peptides Produced by Paenibacillus Sp. Strain B2 Isolated from the. Sorghum Mycorrhizosphere. Appl. Environ. Micro- Biol. 71, 6501–6507. doi:10.1128/AEM.71.11.6501-6507.2005
Selsted, M. E. (2004). Theta-defensins: Cyclic Antimicrobial Peptides Produced by Binary Ligation of Truncated Alpha-Defensins. Curr. Protein Pept. Sci. 5, 365–371. doi:10.2174/1389203043379459
Selsted, M. E., Brown, D. M., DeLange, R. J., Harwig, S. S., and Lehrer, R. I. (1985a). Primary Structures of Six Antimicrobial Peptides of Rabbit Peritoneal Neutrophils. J. Biol. Chem. 260, 4579–4584. doi:10.1016/S0021-9258(18)89110-4
Selsted, M. E., Harwig, S. S., Ganz, T., Schilling, J. W., and Lehrer, R. I. (1985b). Primary Structures of Three Human Neutrophil Defensins. J. Clin. Invest. 76, 1436–1439. doi:10.1172/JCI112121
Shagaghi, N., Palombo, E. A., Clayton, A. H. A., and Bhave, M. (2016). Archetypal Tryptophan-Rich Antimicrobial Peptides: Properties and Applications. World J. Microbiol. Biotechnol. 32, 31. doi:10.1007/s11274-015-1986-z
Shankar, S. S., Benke, S. N., Nagendra, N., Srivastava, P. L., Thulasiram, H. V., and Gopi, H. N. (2013). Self-Assembly to Function: Design, Synthesis, and Broad-Spectrum Antimicrobial Properties of Short Hybrid E-Vinylogous Lipopeptides. J. Med. Chem. 56, 8468–8474. doi:10.1021/jm400884w
Shi, J., Ross, C. R., Chengappa, M. M., Sylte, M. J., McVey, D. S., and Blecha, F. (1996). Antibacterial Activity of a Synthetic Peptide (PR-26) Derived from PR-39, a Proline-Arginine-Rich Neutrophil Antimicrobial Peptide. Antimicrob. Agents Chemother. 40, 115–121. doi:10.1128/AAC.40.1.115
Shi, W., Li, C., Li, M., Zong, X., Han, D., and Chen, Y. (2016). Antimicrobial Peptide Melittin against Xanthomonas Oryzae Pvoryzae, the Bacterial Leaf Blight Pathogen in rice. Appl. Microbiol. Biotechnol. 100, 5059–5067. doi:10.1007/s00253-016-7400-4
Sikorska, E., Stachurski, O., Neubauer, D., Małuch, I., Wyrzykowski, D., Bauer, M., et al. (2018). Short Arginine-Rich Lipopeptides: from Self-As- Sembly to Antimicrobial Activity. Biochim. Biophys. Acta Biomembr. 1860, 2242–2251. doi:10.1016/j.bbamem.2018.09.004
Simmaco, M., Mignogna, G., Barra, D., and Bossa, F. (1994). Antimicrobial Peptides from Skin Secretions of Rana esculenta. Molecular Cloning of cDNAs Encoding Esculentin and Brevinins and Isolation of New Active Peptides. J. Biol. Chem. 269, 11956–11961. doi:10.1016/S0021-9258(17)32666-2
Simmaco, M., Mignogna, G., Canofeni, S., Miele, R., Mangoni, M. L., and Barra, D. (1996). Temporins, Antimicrobial Peptides from the European Red Frog Rana Temporaria. Eur. J. Biochem. 242, 788–792. doi:10.1111/j.1432-1033.1996.0788r.x
Singh, M., Singh, S., Prasad, S., and Gambhir, I. S. (2008). Nanotechnology in Medicine and Antibacterial Effect of Silver Nanoparticles. Dig. J. Nanomater. Biostruct. 3, 115–122.
Singh, P., and Cameotra, S. S. (2004). Potential Applications of Microbial Surfactants in Biomedical Sciences. Trends Biotech. 22, 142–146. doi:10.1016/j.tibtech.2004.01.010
Sitaram, N. (2006). Antimicrobial Peptides with Unusual Amino Acid Compositions and Unusual Structures. Curr. Med. Chem. 13, 679–696. doi:10.2174/092986706776055689
Sitaram, N., and Nagaraj, R. (1999). Interaction of Antimicrobial Peptides with Biological and Model Membranes: Structural and Charge Requirements for Activity. Biochim. Biophys. Act. 1462, 29–54. doi:10.1016/s0005-2736(99)00199-6
Smits, G. J., Kapteyn, J. C., van den Ende, H., and Klis, F. M. (1999). Cell wall Dynamics in Yeast. Curr. Opin. Microbiol. 2, 348–352. doi:10.1016/s1369-5274(99)80061-7
Sørensen, D., Nielsen, T. H., Sørensen, J., and Christophersen, C. (2002). Cyclic Lipoundecapeptide Lokisin from Pseudomonas Sp. Strain DSS41. Tetrahedron Lett. 43, 4421–4423. doi:10.1016/S0040-4039(02)00856-0
Spencer, J. D., Schwaderer, A. L., Wang, H., Bartz, J., Kline, J., Eichler, T., et al. (2013). Ribonuclease 7, an Antimicrobial Peptide Upregulated during Infection, Contributes to Microbial Defense of the Human Urinary Tract. Kidney Int. 83, 615–625. doi:10.1038/ki.2012.410
Stachurski, O., Neubauer, D., Małuch, I., Wyrzykowski, D., Bauer, M., Bartoszewska, S., et al. (2019). Effect of Self-Assembly on Antimicrobial Activity of Double-Chain Short Cationic Lipopeptides. Bioorg. Med. Chem. 27, 115129. doi:10.1016/j.bmc.2019.115129
Stalmans, S., Wynendaele, E., Bracke, N., Knappe, D., Hoffmann, R., Peremans, K., et al. (2014). Blood-brain Barrier Transport of Short Proline-Rich Antimicrobial Peptides. Protein Pept. Lett. 21, 399–406. doi:10.2174/09298665113206660110
Stec, B. (2006). Plant Thionins—The Structural Perspective. Cel. Mol. Life Sci. 63, 1370–1385. doi:10.1007/s00018-005-5574-5
Steenbergen, J. N., Alder, J., Thorne, G. M., and Tally, F. P. (2005). Daptomycin: a Lipopeptide Antibiotic for the Treatment of Serious Gram-Positive Infections. J. Antimicrob. Chemother. 55 (2005), 283–288. doi:10.1093/jac/dkh546
Steffen, H., Rieg, S., Wiedemann, I., Kalbacher, H., Deeg, M., Sahl, H.-G., et al. (2006). Naturally Processed Dermcidin-Derived Peptides Do Not Permeabilize Bacterial Membranes and Kill Microorganisms Irrespective of Their Charge. Antimicrob. Agents Chemother. 50, 2608–2620. doi:10.1128/AAC.00181-06
Stein, T. (2005). Bacillus Subtilis Antibiotics: Structures, Syntheses and Specific Functions. Mol. Microbiol. 56, 845–857. doi:10.1111/j.1365-2958.2005.04587.x
Steiner, H., Hultmark, D., Engstrom, A., Bennich, H., and Boman, H. G. (1981). Sequence and Specificity of Two Antibacterial Proteins Involved in Insect Immunity. Nature 292, 246–248. doi:10.1038/292246a0
Subbalakshmi, C., and Sitaram, N. (1998). Mechanism of Antimicrobial Action of Indolicidin. FEMS Microbiol. Lett. 160, 91–96. doi:10.1111/j.1574-6968.1998.tb12896.x
Svenson, J., Karstad, R., Flaten, G. E., Brandsdal, B. O., Brandl, M., and Svendsen, J. S. (2009). Altered Activity and Physicochemical Properties of Short Cationic Antimicrobial Peptides by Incorporation of Arginine Analogues. Mol. Pharmaceutics 6, 996–1005. doi:10.1021/mp900057k
Takesako, K., Ikai, K., Haruna, F., Endo, M., Shimanaka, K., Sono, E., et al. (1991). Aureobasidins, New Antifungal Antibiotics. Taxonomy, Fermentation, Isolation, and Properties. J. Antibiot. 44, 919–924. doi:10.7164/antibiotics.44.919
Tam, C., Mun, J. J., Evans, D. J., and Fleiszig, S. M. (2012). Cytokeratins Mediate Epithelial Innate Defense through Their Antimicrobial Properties. J. Clin. Invest. 122, 3665–3677. doi:10.1172/JCI64416
Tam, J. P., Lu, Y-A., and Yang, J-L. (2000). Design of Salt-Insensitive Glycine-Rich Antimicrobial Peptides with Cyclic Tricystine Structures. Biochemistry 39, 7159–7169. doi:10.1021/bi0003487
Tam, J. P., Lu, Y. A., and Yang, J. L. (2002). Antimicrobial Dendrimeric Peptides. Eur. J. Biochem. 269, 923–932. doi:10.1046/j.0014-2956.2001.02728.x
Tam, J. P., Wang, S., Wong, K. H., and Tan, W. L. (2015). Antimicrobial Peptides from Plants. Pharmaceuticals 8, 711–757. doi:10.3390/ph8040711
Tang, Y. Q., Yuan, J., Osapay, G., Osapay, K., Tran, D., Miller, C. J., et al. (1999). A Cyclic Antimicrobial Peptide Produced in Primate Leukocytes by the Ligation of Two Truncated Alpha-Defensins. Science 286, 498–502. doi:10.1126/science.286.5439.498
Thimon, L., Peyoux, F., Maget-Dana, R., and Michel, G. (1992). Surface-active Properties of Antifungal Lipopeptides Produced by. Bacillus Subtilis. J. Am. Oil. Chem. Soc. 69, 92–93. doi:10.1007/bf02635884
Timmerman, P., Beld, J., Puijk, W. C., and Meloen, R. H. (2005). Rapid and Quantitative Cyclization of Multiple Peptide Loops onto Synthetic Scaffolds for Structural Mimicry of Protein Surfaces. Chem. Bio Chem. 6, 821–824. doi:10.1002/cbic.200400374
Tomita, M., Takase, M., Bellamy, W., and Shimamura, S. (1994). A Review: The Active Peptide of Lactoferrin. Pediatr. Int. 36, 585–591. doi:10.1111/j.1442-200X.1994.tb03250.x
Tran, H., Ficke, A., Asiimwe, T., Höfte, M., and Raaijmakers, J. M. (2007). Role of the Cyclic Lipopeptide Massetolide A in Biological Control of Phytophthora Infestans and in Colonization of Tomato Plants by. Pseudomonas Fluorescens. New Phytol. 175, 731–742. doi:10.1111/j.1469-8137.2007.02138.x
Van der Weerden, N. L., Hancock, R. E., and Anderson, M. A. (2010). Permeabilization of fungal hyphae by the plant defensin NaD1 occurs through a cell wall-dependent process. J. Biol. Chem. 285, 37513–37520. doi:10.1074/jbc.M110.134882
Van Parijs, J., Broekaert, W. F., Goldstein, I. J., and Peumans, W. J. (1991). Hevein an Antifungal Protein from Rubber-Tree (Hevea Braziliensis) Latex. Planta 183, 258–264. doi:10.1007/BF00197797
Vanittanakom, N., Loeffler, W., Koch, U., and Jung, G. (1986). Fengycin - a Novel Antifungal Lipopeptide Antibiotic Produced by Bacillus Subtilis F-29-3. J. Antibiot. (Tokyo). 39, 888–901. doi:10.7164/antibiotics.39.888
Vasu, S., McGahon, M. K., Moett, R. C., Curtis, T. M., Conlon, J. M., Abdel-Wahab, Y. H. A., et al. (2017a). Esculentin-2CHa(1–30) and its Analogues: Stability and Mechanisms of Insulinotropic Action. J. Endocrinol. 232, 423–435. doi:10.1530/JOE-16-0453
Vasu, S., Ojo, O. O., Moffett, R. C., Conlon, J. M., Flatt, P. R., and Abdel-Wahab, Y. H. A. (2017b). Anti-diabetic Actions of esculentin-2CHa(1–30) and its Stable Analogues in a Diet-Induced Model of Obesity-Diabetes. Amino Acids 49, 1705–1717. doi:10.1007/s00726-017-2469-3
Veiga, A. S., Sinthuvanich, C., Gaspar, D., Franquelim, H. G., Castanho, M. R. B., and Schneider, J. P. (2012). Arginine-rich Self-Assembling Peptides as Potent Antibacterial Gels. Biomaterials 33, 8907–8916. doi:10.1016/j.biomaterials.2012.08.046
Ventola, C. L. (2015). The Antibiotic Resistance Crisis Part 1: Causes and Threats. P T. 40, 277–283.
Vijayakumar, E., Roy, K., Chatterjee, S., Deshmukh, S., Ganguli, B., Fehlhaber, H.-W., et al. (1996). Arthrichitin. A. New Cell Wall Active Metabolite Arthrinium Phaeospermum. J. Org. Chem. 61, 6591–6593. doi:10.1021/jo960769n
Vunnam, S., Juvvadi, P., Rotondi, K. S., and Merrifield, R. B. (1998). Synthesis and Study of normal, Enantio, Retro, and Retroenantio Isomers of Cecropin A-Melittin Hybrids, Their End Group Effects and Selective Enzyme Inactivation. J. Pept. Res. 51, 38–44. doi:10.1111/j.1399-3011.1998.tb00414.x
Waghu, F. H., and Thomas, S. I. (2020). Collection of Antimicrobial Peptides Database and its Derivatives: Applications and beyond. Protein Sci. 29, 36–42. doi:10.1002/pro.3714
Wakabayashi, H., Takase, M., and Tomita, M. (2003). Lactoferricin Derived from Milk Protein Lactoferrin. Curr. Pharm. Des. 9, 1277–1287. doi:10.2174/1381612033454829
Walton, R. B., and Woodruff, H. B. (1949). A Crystalline Antifungal Agent, Mycosubtilin, Isolated from. Subtilin Broth. J. Clin. Invest. 28, 924–926. doi:10.1172/JCI102180
Wang, G. (2014). Human Antimicrobial Peptides and Proteins. Pharmaceuticals 7, 545–594. doi:10.3390/ph7050545
Wang, G., Li, X., and Wang, Z. (2016). APD3: The Antimicrobial Peptide Database as a Tool for Research and Education. Nucleic Acids Res. 44, D1087–D1093. doi:10.1093/nar/gkv1278
Wang, J., Yu, Y., Tang, K., Liu, W., He, X., Huang, X., et al. (2010). Identication and Analysis of the Biosynthetic Gene Cluster Encoding the Thiopeptide Antibiotic Cyclothiazomycin in Streptomyces Hygroscopicus 10-22. Appl. Environ. Microbiol. 76, 2335–2344. doi:10.1128/AEM.01790-09
Wiedman, G., Fuselier, T., He, J., Searson, P. C., Hristova, K., and Wimley, W. C. (2014). Highly Efficient Macromolecule-Sized Poration of Lipid Bilayers by a Synthetically Evolved Peptide. J. Am. Chem. Soc. 136, 4724–4731. doi:10.1021/ja500462s
Wijaya, R., Neumann, G. M., Condron, R., Hughes, A. B., and Polya, G. M. (2000). Defense Proteins from Seed of cassia Fistula Include a Lipid Transfer Protein Homologue and a Protease Inhibitory Plant Defensin. Plant Sci. 159, 243–255. doi:10.1016/s0168-9452(00)00348-4
Won, H.-S., Kang, S.-J., Choi, W.-S., and Lee, B.-J. (2011). Activity Optimization of an Undecapeptide Analogue Derived from a Frog-Skin Antimicrobial Peptide. Mol. Cell 31, 49–54. doi:10.1007/s10059-011-0005-y
Wong, J. H., and Ng, T. B. (2005). Sesquin, a Potent Defensin-like Antimicrobial Peptide from Ground Beans with Inhibitory Activities toward Tumor Cells and HIV-1 Reverse Transcriptase. Peptides 26, 1120–1126. doi:10.1016/j.peptides.2005.01.003
Yamasaki, K., Schauber, J., Coda, A., Lin, H., Dorschner, R. A., Schechter, N. M., et al. (2006). Kallikrein-mediated Proteolysis Regulates the Antimicrobial Effects of Cathelicidins in Skin. FASEB J. 20, 2068–2080. doi:10.1096/fj.06-6075com
Yang, C., Boone, L., Nguyen, T. X., Rudolph, D., Limpakarnjanarat, K., Mastro, T. D., et al. (2005). Theta-Defensin Pseudogenes in HIV-1-Exposed, Persistently Seronegative Female Sex-Workers from Thailand. Infect. Genet. Evol. 5, 11–15. doi:10.1016/j.meegid.2004.05.006
Yi, G. S., Park, C. B., Kim, S. C., and Cheong, C. (1996). Solution Structure of an Antimicrobial Peptide Buforin II. FEBS Lett. 398, 87–90. doi:10.1016/s0014-5793(96)01193-3
Yu, G., Baeder Desiree, Y., Regoes, R. R., and Rolff, J. (2018). Predicting Drug Resistance Evolution: Insights from Antimicrobial Peptides and Antibiotics. Proc. R. Soc. Lond. B Biol. Sci. 285, 20172687. doi:10.1098/rspb.2017.2687
Yu, H. Y., Tu, C. H., Yip, B. S., Chen, H. L., Cheng, H. T., Huang, K. C., et al. (2011). Easy Strategy to Increase Salt Resistance of Antimicrobial Peptides. Antimicrob. Agents Chemother. 55, 4918–4921. doi:10.1128/aac.00202-11
Zahn, M., Berthold, N., Kieslich, B., Knappe, D., Hoffmann, R., and Sträter, N. (2013). Structural Studies on the Forward and Reverse Binding Modes of Peptides to the Chaperone DnaK. J. Mol. Bio 425, 2463–2479. doi:10.1016/j.jmb.2013.03.041
Zanjani, N. T., Miranda-Saksena, M., Cunningham, A. L., and Dehghani, F. (2018). Antimicrobial Peptides of marine Crustaceans: The Potential and Challenges of Developing Therapeutic Agents. Curr. Med. Chem. 25, 2245–2259. doi:10.2174/0929867324666171106155936
Zasloff, M. (1987). Magainins, a Class of Antimicrobial Peptides from Xenopus Skin: Isolation, Characterization of Two Active Forms, and Partial cDNA Sequence of a Precursor. Proc. Natl. Acad. Sci. USA. 84, 5449–5453. doi:10.1073/pnas.84.15.5449
Zhang, L., Benz, R., and Hancock, R. E. (1999). Influence of Proline Residues on the Antibacterial and Synergistic Activities of α-helical Peptides. Biochemistry 38, 8102–8111. doi:10.1021/bi9904104
Zhang, S. K., Ma, Q., Li, S. B., Gao, H. W., Tan, Y. X., Gong, F., et al. (2016). RV-23, a Melittin-Related Peptide with Cell-Selective Antibacterial Activity and High Hemocompatibility. J. Microbiol. Biotechnol. 26, 1046–1056. doi:10.4014/jmb.1510.10074
Zhong, C., Zhu, N., Zhu, Y., Liu, T., Gou, S., Xie, J., et al. (2020). Antimicrobial Peptides Conjugated with Fatty Acids on the Side Chain of D-Amino Acid Promises Antimicrobial Potency against Multidrug-Resistant Bacteria. Eur. J. Pharm. Sci. 141, 105123. doi:10.1016/j.ejps.2019.105123
Glossary
Ala Alanine
ACHC Aminocyclohexanecarboxylic acid
AMPs Antimicrobial peptides
App L-2-amino-3-(4-aminophenyl)-propanoic acid
Arg Arginine
Bip Biphenylalanine
Asn Asparagine
CCK Cyclic cysteine knot
CLPs Cyclic lipopeptides
CPPs Cell penetrating peptides
Cys Cysteine
Gly Glycine
Gpp L-2-amino-3-(4-guanidinophenyl) propanoic acid
His Histidine
Leu Leucine
Lys Lysine
MDR Multi drug resistant
Nle Nor-Leucine
NRPs Non-ribosomal protein synthetases
Oic Octahydroindolecarboxylic acid
Orn Ornithine
PINs Puroindolins
Phe Phenylalanine
PLL Polylysine dendrons
Pr-AMPs Proline rich AMPs
Pro Proline
ROS Reactive oxygen species
Tbt tri-tert-butyl tryptophan
Tic Tetrahydroisoquinolinecarboxylic acid
Thr Threonine
TrAMPs Tryptophan rich AMPs
Tyr Tyrosine
Trp Tryptophan
Val Valine
A. nidulans Aspergillus nidulans
A. niger Aspergillus niger
A. terreus Aspergillus terreus
B. cereus Bacillus cereus
B. subtilis Bacillus subtilis
C. albicans Candida albicans
C. neoformans Cryptococcus neoformans
C. graminicola Colletotrichum graminicola
C. grubii Cryptococcus neoformans var. grubii
C. tropicalis Candida tropicalis
E. coli Escherichia coli
E. faecalis Enterococcus faecalis
F. oxysporum Fusarium oxysporum
K. pneumoniae Klebsiella pneumoniae
M. luteus Micrococcus luteus
MRSA Methicillin resistant Staphylococcus Aureus
MSSA Methicillin sensetive Staphylococcus Aureus
P. aeruginosa Pseudomonas aeruginosa
P. oryzae Pleomorphomonas oryzae
R. solani Rhizoctonia solani
S. aureus Staphylococcus aureus
S. dysenteriae Shigella dysenteriae
S. epidermidis Staphylococcus epidermidis
S. typhimurium Salmonella typhimurium
S. typhi Salmonella typhi
Keywords: antimicrobial peptides, antimicrobial resistance, natural and synthetic AMPs, antimicrobial activity, cytotoxicity, protease resistance, AMP delivery strategies
Citation: Sarkar T, Chetia M and Chatterjee S (2021) Antimicrobial Peptides and Proteins: From Nature’s Reservoir to the Laboratory and Beyond. Front. Chem. 9:691532. doi: 10.3389/fchem.2021.691532
Received: 06 April 2021; Accepted: 27 May 2021;
Published: 18 June 2021.
Edited by:
Maria Luisa Mangoni, Sapienza University of Rome, ItalyReviewed by:
Edith Amuhaya, United States International University-Africa, KenyaGyula Batta, University of Debrecen, Hungary
Copyright © 2021 Sarkar, Chetia and Chatterjee. This is an open-access article distributed under the terms of the Creative Commons Attribution License (CC BY). The use, distribution or reproduction in other forums is permitted, provided the original author(s) and the copyright owner(s) are credited and that the original publication in this journal is cited, in accordance with accepted academic practice. No use, distribution or reproduction is permitted which does not comply with these terms.
*Correspondence: Sunanda Chatterjee, c3VuYW5kYS5jQGlpdGcuYWMuaW4=