- Department of Chemistry and Konstanz Research School Chemical Biology (KoRS-CB), University of Konstanz, Konstanz, Germany
The inverse electron-demand Diels-Alder (IEDDA or DAinv) reaction is an emerging bioorthogonal ligation reaction that finds application in all areas of chemistry and chemical biology. In this review we highlight its application in metabolic glycoengineering (MGE). MGE is a versatile tool to introduce unnatural sugar derivatives that are modified with a chemical reporter group into cellular glycans. The IEDDA reaction can then be used to modify the chemical reporter group allowing, for instance, the visualization or isolation of glycoconjugates. During the last years, many different sugar derivatives as well as reporter groups have been published. These probes are summarized, and their chemical and biological properties are discussed. Furthermore, we discuss examples of MGE and subsequent IEDDA reaction that highlight its suitability for application within living systems.
Introduction
The Diels-Alder reaction (Diels and Alder, 1928) is widely used for stereoselective synthesis of complex molecules, such as many natural products or pharmaceuticals (Brieger and Bennett, 1980; Nicolaou et al., 2002; Funel and Abele, 2013). In 1959, a variant thereof, the inverse-electron-demand Diels-Alder (IEDDA or DAinv) reaction of 1,2,4,5-tetrazines and electron-rich dienophiles was reported for the first time (Carboni and Lindsey, 1959). However, it took until 2008 when the IEDDA reaction between 1,2,4,5-tetrazines and trans-cyclooctenes (Blackman et al., 2008), cyclobutenes (Braun et al., 2008), or norbornenes (Devaraj et al., 2008) was applied in bioconjugation. The rate constants of the IEDDA reaction can span a range of many orders of magnitude depending on the used tetrazine and dienophile structure, and it can be performed in aqueous media which even accelerates the reaction (Wijnen et al., 1996). Among the dienophiles with fastest reaction kinetics are trans-cyclooctene (TCO) derivatives with a three-membered ring fused to the eight-membered cyclooctene (sTCO) resulting in second-order rate constants up to 106 M−1 s−1 (Taylor et al., 2011).
Although norbornene and especially TCO derivatives react rapidly in the IEDDA reaction even exceeding the kinetics of well-established bioorthogonal ligation reactions, such as the copper-catalyzed azide-alkyne [3 + 2] cycloaddition (CuAAC) (Rostovtsev et al., 2002; Tornøe et al., 2002), their size limits potential applications as reporter groups for metabolic labeling. From extensive studies of Sauer and coworkers it was known that 3-methyl-cyclopropene reacts rapidly with tetrazines (Thalhammer et al., 1990). However, cyclopropenes are prone to polymerization and were known to be unstable toward thiols and other potential nucleophiles present in a cell preventing their use in cellular systems. To overcome these limitations, the Deveraj (Yang et al., 2012) and Prescher (Patterson et al., 2012) groups investigated the effects of substituents on cyclopropene stability and reactivity. Methyl substituents at the double bond greatly increased the stability of the cyclopropenes toward nucleophiles as well as polymerization leading to the first dienophile-modified carbohydrate derivative suitable for metabolic labeling (Patterson et al., 2012).
The IEDDA reaction is not only fast but also chemoselective and irreversible due to a subsequent retro-Diels-Alder reaction under nitrogen release. Furthermore, it does not require toxic heavy metal catalysts. These features make the IEDDA reaction an excellent choice for numerous applications as bioorthogonal ligation reaction in all areas of chemistry and chemical biology (Knall and Slugovc, 2013; Wu and Devaraj, 2016; Mayer and Lang, 2017; Oliveira et al., 2017). One of these applications is metabolic glycoengineering (Kayser et al., 1992; Mahal et al., 1997; Palaniappan and Bertozzi, 2016; Sminia et al., 2016; Wratil et al., 2016). The pivotal roles that carbohydrates play in biology, for example during protein regulation, cell adhesion, or immune response, led to various approaches to study their biological functions. In metabolic glycoengineering (MGE), cells are incubated with synthetic carbohydrate derivatives which are equipped with an unnatural functional group, named a chemical reporter group (Prescher and Bertozzi, 2005). The sugars are metabolized by the enzymatic machinery of the cell and are incorporated into glycan structures in competition to their natural equivalents. Subsequent modification of the reporter group can be achieved by a bioorthogonal ligation reaction (Sletten and Bertozzi, 2009) to allow, for example, the visualization of glycosylation during different cellular conditions in health and disease, the isolation of glycoconjugates, or targeted delivery strategies (Wang et al., 2017). To facilitate cellular uptake by passive diffusion, the carbohydrate derivatives are usually employed in their O-acetylated form. Inside cells, O-deacetylation is catalyzed by non-specific esterases. The effect of added sugars on the carbohydrate metabolism of the cell is a topic of current investigations (Dold and Wittmann, 2021).
Multiple sugar analogues with different reporter groups have been synthesized over the years including a ketone-modified mannosamine derivative (Mahal et al., 1997), azido sugars (Saxon and Bertozzi, 2000), alkyne sugars (Hsu et al., 2007), isonitrile-modified sugars (Stairs et al., 2013), and others summarized in the above-mentioned review articles. More recently, dienophile-modified sugars that can be reacted in a IEDDA reaction have been added to the MGE toolbox, and they are the topic of this review. In the following section, we will first present a chronological order of dienophile-modified carbohydrates before we will highlight some applications of these derivatives.
Chronology of Dienophile-Modified Carbohydrates
In 2012, the Prescher group reported the first example of a dienophile-modified carbohydrate, a sialic acid with a methyl-substituted cyclopropene linked by an amide bond to the 9-position (9-Cp-NeuAc) (Patterson et al., 2012) (Figure 1). 9-Cp-NeuAc was incorporated into Jurkat cell surface glycans and labeled in a two-step process with a tetrazine-biotin and a streptavidin-dye conjugate. Successful incorporation was quantified with flow cytometry. Additionally, the concurrent utilization of methylcyclopropene and azide reporters for dual labeling was shown. In the following year, our group introduced terminal alkene-modified mannosamine derivatives with different chain length and an amide (Ac4ManNPtl and Ac4ManNHxl) or a carbamate linkage (Ac4ManNPeoc) (Niederwieser et al., 2013). MGE experiments and confocal fluorescence microscopy revealed their metabolic acceptance and incorporation into glycans on the surface of HEK 293T and HeLa S3 cells. The combination of IEDDA and click chemistry allowed for the first time the detection of two different carbohydrates–one modified with a terminal alkene, another with an azide—within one experiment. In the following, a variety of mannosamines derivatized with carbamate-linked terminal alkenes (Ac4ManNAloc, Ac4ManNBeoc, Ac4ManNHeoc) were synthesized and compared unraveling the correlation between staining intensity on the one hand and metabolic acceptance and reaction rate depending on the chain length on the other hand (Späte et al., 2014c). In addition, glucosamine analogues with carbamate-linked terminal alkenes were reported. However, cell experiments showed that these sugars are cell toxic at the concentrations necessary for MGE.
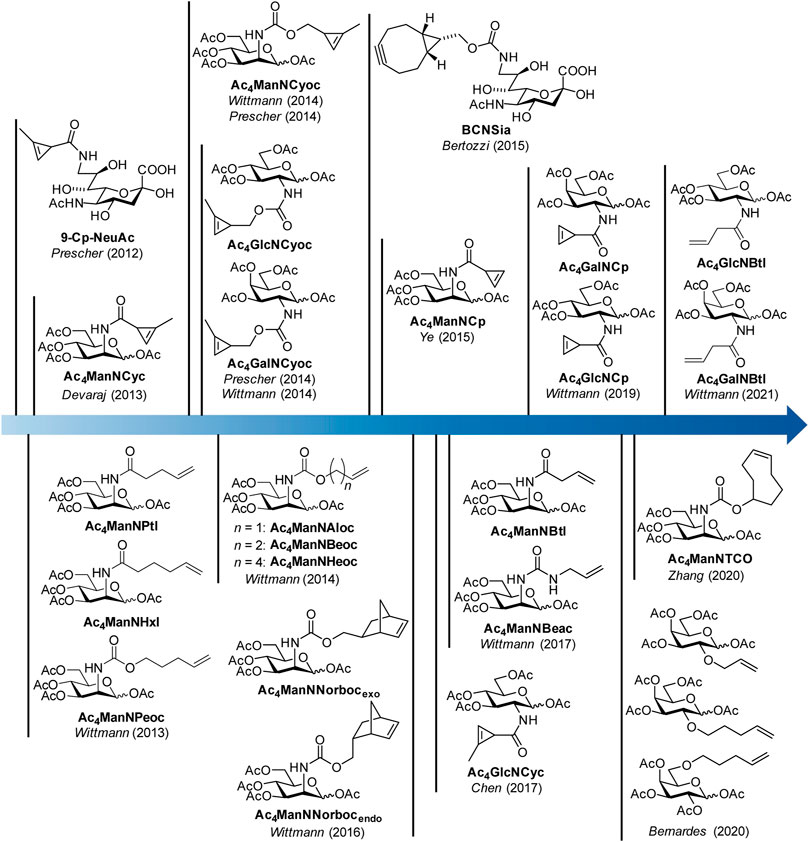
FIGURE 1. Timeline of synthetic carbohydrate derivatives for MGE with dienophile reporter groups for bioorthogonal labeling with the IEDDA reaction.
Also in 2013, the Devaraj group introduced the mannosamine analogue Ac4ManNCyc modified with the same amide-linked methylcyclopropene used by Prescher (Cole et al., 2013). They could show that the sugar is processed by different human cancer cell lines and displaced on the cell surface allowing visualization by confocal fluorescence microscopy after IEDDA labeling with a tetrazine-dye conjugate. Based on the observation of the Devaraj group (Yang et al., 2012) that carbamate-linked methylcyclopropenes react about 100 times faster with tetrazines than amide-linked ones, we (Späte et al., 2014a) and later on the Prescher group (Patterson et al., 2014) reported the mannosamine derivative Ac4ManNCyoc with a carbamate-linked cyclopropene. The higher IEDDA reactivity of the carbamate-linked cyclopropene significantly reduced the time needed for the labeling step compared to the amide-linked cyclopropene enabling an efficient one-step labeling approach with a tetrazine-dye conjugate. This improved methylcyclopropene reporter was also used to modify glucosamine (Ac4GlcNCyoc) as well as galactosamine (Ac4GlcNCyoc) (Späte et al., 2014b; Patterson et al., 2014).
In 2015, a bicyclononyne-modified sialic acid (BCNSia) was synthesized and incorporated into the glycans of various cell lines (Agarwal et al., 2015). Additionally, it was used to investigate sialylation in zebra fish embryos. In the same year, mannosamine with a minimal amide-linked cyclopropene reporter, Ac4ManNCp, was published by the Ye group (Xiong et al., 2015). This derivative appeared to be surprisingly stable although it lacks the methyl substituent and reacted 9-fold faster with a tetrazine than Ac4ManNCyc. In a direct comparison, the new sugar gave higher fluorescence intensities after IEDDA labeling than the amide-linked methylcyclopropene in MGE experiments in several cell lines.
In the following years, norbornene-modified mannosamine derivatives (Ac4ManNNorbocendo/exo) (Späte et al., 2016), a shorter amide-linked terminal alkene (Ac4ManNBtl) and an urea-linked terminal alkene (Ac4ManNBeac) (Dold et al., 2017) extended the pool of reporter groups for the IEDDA reaction. Additionally, the glucosamine derivative Ac4GlcNCyc of the amide-linked methylcyclopropene was synthetized (Zhu and Chen, 2017). In 2019, glucosamine and galactosamine derivatives of the unsubstituted amide-linked cyclopropene (Ac4GlcNCp and Ac4GalNCp) complemented the cyclopropene series (Hassenrück and Wittmann, 2019). Only recently, the use of galactose analogues modified with terminal alkenes (Kitowski and Bernardes, 2020), a TCO-modified mannosamine derivative (Ac4ManNTCO) (Zhang et al., 2020) and the butenoyl derivatives Ac4GlcNBtl and Ac4GalNBtl (Dold and Wittmann, 2021) were reported.
Reporter Groups
Cyclopropene Reporters
Cyclopropene reporters, that have been developed for MGE, comprise an amide-linked methylcyclopropene (Cyc), a carbamate-linked methylcyclopropene (Cyoc), and a minimal amide-linked cyclopropene (Cp). In the following, the mannosamine derivatives of these three cyclopropenes are compared (Hassenrück and Wittmann, 2019). The minimal amide-linked cyclopropene (Ac4ManNCp) has a higher IEDDA reactivity than the amide-linked methylcyclopropene (Ac4ManNCyc) and also a higher incorporation efficiency (IE)* in HEK 293T cells (Table 1) resulting in a brighter cell surface staining as determined by flow cytometry and confocal fluorescence microscopy. The lack of the methyl group clearly improves the reporter. Comparison of the methylcyclopropenes Ac4ManNCyc and Ac4ManNCyoc shows that the carbamate linkage increases the reactivity in the IEDDA reaction, while it significantly decreases the IE. In the end, cell surface staining was more intense for Ac4ManNCyoc. In this case, the higher reactivity of ManNCyoc outweighs the lower IE. For Ac4ManNCyoc and Ac4ManNCp, the observed cell surface staining is nearly the same. However, the smaller Ac4ManNCp is better accepted than Ac4ManNCyoc leading to a higher IE for the amide-linked minimal cyclopropene. The reaction kinetics behave vice versa. Here, the higher IE of ManNCp and the higher reactivity of ManNCyoc balance each other out.
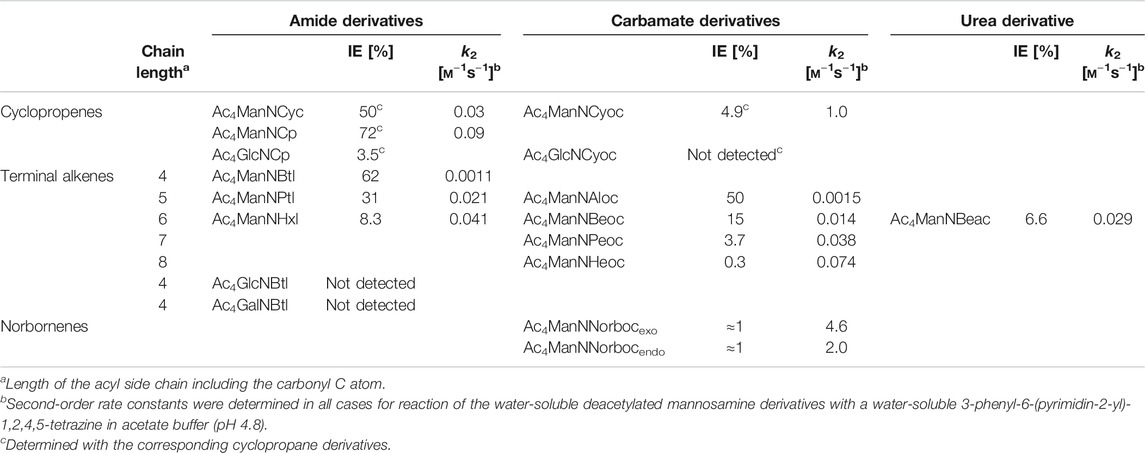
TABLE 1. Incorporation efficiencies (IE) as sialic acids in MGE experiments and second-order rate constants k2 of aminosugar derivatives with dienophile reporter groups (Niederwieser et al., 2013; Späte et al., 2014c; Späte et al., 2016; Dold et al., 2017; Hassenrück and Wittmann, 2019; Dold and Wittmann, 2021).
The Cyoc reporter was also attached to glucosamine and galactosamine and the resulting derivatives Ac4GlcNCyoc and Ac4GalNCyoc used for MGE. When Jurkat cell lysate was analyzed by Western blot, Ac4GlcNCyoc resulted in a more intense labeling than the corresponding azide derivative whereas Ac4ManNCyoc and Ac4GalNCyoc gave a similar intensity compared to the corresponding azide derivatives (Patterson et al., 2014). The three Cyoc derivatives were also used for MGE with HeLa S3 cells (Späte et al., 2014b). Western blot analysis of cell lysate resulted in a significantly stronger staining intensity for cells that had been cultivated with Ac4GlcNCyoc. Since the sample preparation included the fraction of intracellular proteins and O-GlcNAcylation is a modification primarily found for cytosolic and nuclear proteins, it was suggested that Ac4GlcNCyoc is suitable to target O-GlcNAcylated proteins. Analysis of cell-surface staining of HEK 293T and HeLa S3 cells by confocal microscopy on the other hand revealed that Ac4ManNCyoc induced the most intense staining of the three Cyoc derivatives.
When the Cp and Cyoc derivatives of glucosamine were compared in HEK 293T cells, Ac4GlcNCp showed a much brighter cell-surface staining than Ac4GlcNCyoc whereas Ac4GlcNCyoc resulted in a more intense labeling in a Western blot analysis of cell lysate (Hassenrück and Wittmann, 2019). DMB-labeling experiments with the corresponding cyclopropane derivatives suggest that GlcNCp is converted into the corresponding sialic acid whereas GlcNCyoc is not.
Ac4GlcNCyoc was successfully applied for the visualization of protein-specific O-GlcNAcylation inside living cells (Doll et al., 2016; Doll et al., 2018). Proteins of interest were tagged with GFP. After MGE and labeling with a cell permeable tetrazine-TAMRA conjugate, O-GlcNAcylation of the protein of interest as well as its localization within the cell was detected by FLIM-FRET microscopy. This example highlights the advantage of the IEDDA chemistry of not requiring a toxic catalyst enabling its application within a living cell.
Ac4GlcNCyoc was also used to image glycans in Arabidopsis roots (Hoogenboom et al., 2016). In comparison to CuAAC, the IEDDA reaction showed a more uniform fluorescence signal with less background staining. The handling of the IEDDA reagents was easier and the redundant need for copper did not damage the cell wall of the plants. Additionally, also Ac4GlcNCyc (named Ac4GlcNCp in this publication) was used in Arabidopsis thaliana to evaluate the reporter performance for visualizing root carbohydrate structures by fluorescence imaging (Zhu and Chen, 2017). The observation that the carbamate-linked methylcyclopropene Cyoc has a low reactivity toward nitrile imines that are photochemically generated during the photoclick reaction, allowed the use of Ac4ManNCyoc in combination with azido- and acryl-mannosamine derivatives in a triple-orthogonal labeling approach (Schart et al., 2019).
Terminal Alkene Reporters
Terminal alkenes were developed in parallel to cyclopropene reporters (Niederwieser et al., 2013) and extend the repertoire of reporter groups suitable for MGE. They are small, robust, and hardly found in biological systems. In proteins, they are completely absent. Both, their reactivity in the IEDDA reaction and metabolic acceptance are dependent on the chain length. Comparative studies with mannosamine derivatives (Dold et al., 2017) showed that shorter chain lengths are better accepted by the enzymes of the sialic acid biosynthesis. Longer chain lengths between the terminal alkene and the electron-withdrawing carbonyl group on the other hand increase the electron density of the double bond and, therefore, their reactivity in the IEDDA reaction. These two opposite effects lead to the observation that within a series of compounds a certain chain length represents the best balance between the two effects resulting in the most intense cell surface staining (Späte et al., 2014c).
Over the time, a pool of various terminal alkenes was developed (Niederwieser et al., 2013; Späte et al., 2014c; Dold et al., 2017; Dold and Wittmann, 2021). The compounds differed not only in their length of the side chain but also in the type of linkage to the amino sugar (amide, carbamate, urea). Table 1 gives an overview on the metabolic acceptance (in terms of IE values) and the second-order rate constants k2 of investigated compounds. Both within the series of amide derivatives and carbamate derivatives the two opposite effects on metabolic acceptance and reactivity mentioned above can be seen. Interesting is a comparison of three compounds that share the same length of the side chain but differ in the type of linkage: amide-linked Ac4ManNHxl, carbamate-linked Ac4ManNBeoc, and urea-linked Ac4ManNBeac. The carbamate is best accepted (IE = 15%), followed by the amide (IE = 8.3%) and the urea motif (IE = 6.6%). The order of reactivity is different with k2 values of 0.041 m−1 s−1 for the amide, 0.029 m−1 s−1 for the urea, and 0.014 m−1 s−1 for the carbamate. For the cell-surface staining intensity observed by confocal fluorescence microscopy, the balance of IE and reaction kinetics is crucial. Using HEK 293T cells, the brightest staining was observed for amide-linked Ac4ManNHxl. Carbamate-linked Ac4ManNBeoc showed a weak staining whereas the urea-linked Ac4ManNBeac showed barely any staining (Dold et al., 2017).
Only recently, terminal-alkene derivatives of galactose were used for investigation of glycans in Plasmodium-infected hepatic cells (Kitowski and Bernardes, 2020). In this case the O2 or O6 position was modified by ether-linked terminal olefins of different chain length.
Larger Ring-Strained Alkenes and Alkynes as Reporters
Ring-strained alkenes and alkynes, such as trans-cyclooctenes (TCO), bicyclononynes (BCN), or norbornenes, are among the fastest dienophiles for the IEDDA reaction (Lang et al., 2012). Unfortunately, their large size compared to other reporter groups can hamper the acceptance of unnatural sugar derivatives with these moieties by the enzymatic machinery (Jacobs et al., 2001; Pouilly et al., 2012; Dold et al., 2017). However, the following examples show that they are a viable alternative to cyclopropenes when reactivity and stability is more important than incorporation efficiency.
The first example is BCNSia, a sialic acid with a carbamate linkage to a BCN moiety at C-9 (Agarwal et al., 2015). This position was chosen for modification, since it is known that the enzymes involved in the incorporation of sialic acids tolerate even a larger alteration (Oetke et al., 2002; Han et al., 2005). BCN features a higher stability than cyclopropenes making it suitable for experiments with longer incubation times before further derivatization. BCNSia was employed to investigate sialylation in developing zebra fish embryos and besides known sialylation patterns new ones could be identified. Also this example highlights the benefit of the IEDDA reaction in living systems. With a tetrazine derivative that shows a strong increase in fluorescence after IEDDA reaction (Wu et al., 2014) it was possible to reduce background fluorescence.
Later, the potential of norbornene-modified mannosamine derivatives was explored (Späte et al., 2016). They react faster than cyclopropene derivatives with second order rate constants of 4.6 m−1 s−1 for ManNNorbocexo and 2.0 m−1 s−1 for ManNNorbocendo. The differences in reaction rates between exo and endo derivatives, that had also been reported earlier (Vrabel et al., 2013), were observable when human cells were incubated with the unnatural sugars and labeled with a dye for fluorescence microscopy, flow cytometry, or Western blot analysis. The incorporation efficiency by DMB-labeling was determined to be about 1% for both derivatives. Additionally, the possibility for dual labeling together with click chemistry was shown for the norbornene derivatives (Späte et al., 2016) as well as BCNSia (Agarwal et al., 2015).
A TCO-modified mannosamine derivative (ManNTCO) was synthesized and used for MGE in human cancer cells and xenograft mice (Zhang et al., 2020). IEDDA reaction with a tetrazine-coated multi-spectral upconversion nanophosphor probe allowed to label the cell-surface glycans and monitor their level in living mice in real time. The probe can be applied as a replacement for common organic dyes with the potential to avoid tissue penetration and spontaneous fluorescence.
Conclusion
The IEDDA reaction is a bioorthogonal ligation reaction that is now well-established for application in MGE. Many different carbohydrate reporters that differ in size and reactivity have been developed and chemically and biologically characterized. In combination with different tetrazines this represents a large toolbox that allows a fine tuning of a probe for the demands of different experiments. Whereas cyclopropene reporters feature high reactivity in combination with small size, terminal alkenes are chemically more stable enabling experiments with longer incubation times. The metabolic acceptance for probes that end up as sialic acids can be determined by DMB labeling. Depending on the size of the reporter group, the incorporation efficiency IE can vary greatly from below 1% up to more than 70%. This allows to select suitable probes for cases when the natural glycan structure should not be altered significantly or when an efficient incorporation is desired. Among the prime features of the IEDDA reaction is its compatibility with a living environment (cell, animal), because the reaction does not need toxic catalysts. This enables applications within living cells, such as the visualization of intracellular protein glycosylation in a spatially- and time-resolved manner. The option to combine the IEDDA reaction with other ligation reaction that can be orthogonal to the IEDDA reaction (click reaction, photoclick reaction) offers the option to perform dual- and even triple-labeling experiments. In this way, it becomes, for example, possible to quantify alterations of the levels of two or three carbohydrates (or other biomolecules) relative to each other in response to changing conditions. Its beneficial properties make the IEDDA reaction a valuable bioorthogonal ligation reaction not only for metabolic glycoengineering but for all sorts of applications in chemical biology.
Author Contributions
LH and MK wrote the first draft of the manuscript. All authors contributed to the conception of this work, to manuscript revision, and approved the final version.
Funding
This work was supported by the Deutsche Forschungsgemeinschaft (SFB 969, project B05; SPP 1623), the Ministerium für Wissenschaft, Forschung und Kunst Baden-Württemberg (33-7533-7-11.9/7/2), and COST Action GLYCONanoProbes (CA18132).
Conflict of Interest
The authors declare that the research was conducted in the absence of any commercial or financial relationships that could be construed as a potential conflict of interest.
Footnotes
*The IE is the percentage of cellular sialic acids that is modified with the reporter group after MGE and can be determined by the DMB-labeling method (Dold et al., 2017). Since cyclopropenes are not stable under the acidic labeling conditions, their IE values have been approximated by using the corresponding cyclopropane derivatives during MGE (Hassenrück and Wittmann, 2019).
References
Agarwal, P., Beahm, B. J., Shieh, P., and Bertozzi, C. R. (2015). Systemic fluorescence imaging of zebrafish glycans with bioorthogonal chemistry. Angew. Chem. Int. Ed. 54 (39), 11504–11510. doi:10.1002/anie.201504249
Blackman, M. L., Royzen, M., and Fox, J. M. (2008). Tetrazine ligation: fast bioconjugation based on inverse-electron-demand Diels−Alder reactivity. J. Am. Chem. Soc. 130 (41), 13518–13519. doi:10.1021/ja8053805
Braun, K., Wiessler, M., Ehemann, V., Pipkorn, R., Spring, H., Debus, J., et al. (2008). Treatment of glioblastoma multiforme cells with temozolomide-BioShuttle ligated by the inverse Diels-Alder ligation chemistry. Drug Des. Devel. Ther. 2, 289–301. doi:10.2147/dddt.s3572
Brieger, G., and Bennett, J. N. (1980). The intramolecular diels-alder reaction. Chem. Rev. 80 (1), 63–97. doi:10.1021/cr60323a004
Carboni, R. A., and Lindsey, R. V. (1959). Reactions of tetrazines with unsaturated compounds. A new synthesis of pyridazines. J. Am. Chem. Soc. 81, 4342–4346. doi:10.1021/ja01525a060
Cole, C. M., Yang, J., Šečkutė, J., and Devaraj, N. K. (2013). Fluorescent live-cell imaging of metabolically incorporated unnatural cyclopropene-mannosamine derivatives. ChemBioChem 14 (2), 205–208. doi:10.1002/cbic.201200719
Devaraj, N. K., Weissleder, R., and Hilderbrand, S. A. (2008). Tetrazine-based cycloadditions: application to pretargeted live cell imaging. Bioconjug. Chem. 19 (12), 2297–2299. doi:10.1021/bc8004446
Diels, O., and Alder, K. (1928). Synthesen in der hydroaromatischen Reihe. Justus Liebigs Ann. Chem. 460 (1), 98–122. doi:10.1002/jlac.19284600106
Dold, J. E. G. A., Pfotzer, J., Späte, A. K., and Wittmann, V. (2017). Dienophile-modified mannosamine derivatives for metabolic labeling of sialic acids: a comparative study. ChemBioChem 18 (13), 1242–1250. doi:10.1002/cbic.201700002
Dold, J. E. G. A., and Wittmann, V. (2021). Metabolic glycoengineering with azide‐ and alkene‐modified hexosamines: quantification of sialic acid levels. ChemBioChem. doi:10.1002/cbic.202000715
Doll, F., Buntz, A., Späte, A.-K., Schart, V. F., Timper, A., Schrimpf, W., et al. (2016). Visualization of protein-specific glycosylation inside living cells. Angew. Chem. Int. Ed. 55 (6), 2262–2266. doi:10.1002/anie.201503183
Doll, F., Hassenrück, J., Wittmann, V., and Zumbusch, A. (2018). “Chapter eleven - intracellular imaging of protein-specific glycosylation,” in Methods enzymol. Editor B. Imperiali (Cambridge, Massachusetts, US: Academic Press), 283–319.
Funel, J. A., and Abele, S. (2013). Industrial applications of the diels-alder reaction. Angew. Chem. Int. Ed. 52 (14), 3822–3863. doi:10.1002/anie.201201636
Han, S., Collins, B. E., Bengtson, P., and Paulson, J. C. (2005). Homomultimeric complexes of CD22 in B cells revealed by protein-glycan cross-linking. Nat. Chem. Biol. 1 (2), 93–97. doi:10.1038/nchembio713
Hassenrück, J., and Wittmann, V. (2019). Cyclopropene derivatives of aminosugars for metabolic glycoengineering. Beilstein J. Org. Chem. 15, 584–601. doi:10.3762/bjoc.15.54
Hoogenboom, J., Berghuis, N., Cramer, D., Geurts, R., Zuilhof, H., and Wennekes, T. (2016). Direct imaging of glycans in Arabidopsis roots via click labeling of metabolically incorporated azido-monosaccharides. BMC Plant Biol. 16 (1), 220. doi:10.1186/s12870-016-0907-0
Hsu, T.-L., Hanson, S. R., Kishikawa, K., Wang, S.-K., Sawa, M., and Wong, C.-H. (2007). Alkynyl sugar analogs for the labeling and visualization of glycoconjugates in cells. Proc. Natl. Acad. Sci. 104 (8), 2614. doi:10.1073/pnas.0611307104
Jacobs, C. L., Goon, S., Yarema, K. J., Hinderlich, S., Hang, H. C., Chai, D. H., et al. (2001). Substrate specificity of the sialic acid biosynthetic pathway†. Biochemistry 40 (43), 12864–12874. doi:10.1021/bi010862s
Kayser, H., Zeitler, R., Kannicht, C., Grunow, D., Nuck, R., and Reutter, W. (1992). Biosynthesis of a nonphysiological sialic acid in different rat organs, using N-propanoyl-D-hexosamines as precursors. J. Biol. Chem. 267 (24), 16934–16938. doi:10.1016/S0021-9258(18)41874-1
Kitowski, A., and Bernardes, G. J. L. (2020). A sweet galactose transfer: metabolic oligosaccharide engineering as a tool to study glycans in Plasmodium infection. ChemBioChem 21 (18), 2696–2700. doi:10.1002/cbic.202000226
Knall, A. C., and Slugovc, C. (2013). Inverse electron demand Diels-Alder (iEDDA)-initiated conjugation: a (high) potential click chemistry scheme. Chem. Soc. Rev. 42 (12), 5131–5142. doi:10.1039/C3CS60049A
Lang, K., Davis, L., Wallace, S., Mahesh, M., Cox, D. J., Blackman, M. L., et al. (2012). Genetic encoding of bicyclononynes and trans-cyclooctenes for site-specific protein labeling in Vitro and in live mammalian cells via rapid fluorogenic diels-alder reactions. J. Am. Chem. Soc. 134 (25), 10317–10320. doi:10.1021/ja302832g
Mahal, L. K., Yarema, K. J., and Bertozzi, C. R. (1997). Engineering chemical reactivity on cell surfaces through oligosaccharide biosynthesis. Science 276 (5315), 1125. doi:10.1126/science.276.5315.1125
Mayer, S., and Lang, K. (2017). Tetrazines in inverse-electron-demand diels–alder cycloadditions and their use in biology. Synthesis 49 (4), 830–848. doi:10.1055/s-0036-1588682
Nicolaou, K. C., Snyder, S. A., Montagnon, T., and Vassilikogiannakis, G. (2002). The diels-alder reaction in total synthesis. Angew. Chem. Int. Ed. 41 (10), 1668–1698. doi:10.1002/1521-3773(20020517)41:10<1668::AID-ANIE1668>3.0.CO;2-Z
Niederwieser, A., Späte, A.-K., Nguyen, L. D., Jüngst, C., Reutter, W., and Wittmann, V. (2013). Two-color glycan labeling of live cells by a combination of diels-alder and click chemistry. Angew. Chem. Int. Ed. 52 (15), 4265–4268. doi:10.1002/anie.201208991
Oetke, C., Brossmer, R., Mantey, L. R., Hinderlich, S., Isecke, R., Reutter, W., et al. (2002). Versatile biosynthetic engineering of sialic acid in living cells using synthetic sialic acid analogues. J. Biol. Chem. 277 (8), 6688–6695. doi:10.1074/jbc.M109973200
Oliveira, B. L., Guo, Z., and Bernardes, G. J. L. (2017). Inverse electron demand Diels-Alder reactions in chemical biology. Chem. Soc. Rev. 46 (16), 4895–4950. doi:10.1039/C7CS00184C
Palaniappan, K. K., and Bertozzi, C. R. (2016). Chemical glycoproteomics. Chem. Rev. 116 (23), 14277–14306. doi:10.1021/acs.chemrev.6b00023
Patterson, D. M., Nazarova, L. A., Xie, B., Kamber, D. N., and Prescher, J. A. (2012). Functionalized cyclopropenes as bioorthogonal chemical reporters. J. Am. Chem. Soc. 134 (45), 18638–18643. doi:10.1021/ja3060436
Patterson, D. M., Jones, K. A., and Prescher, J. A. (2014). Improved cyclopropene reporters for probing protein glycosylation. Mol. Biosyst. 10 (7), 1693–1697. doi:10.1039/C4MB00092G
Pouilly, S., Bourgeaux, V., Piller, F., and Piller, V. (2012). Evaluation of analogues of GalNAc as substrates for enzymes of the mammalian GalNAc salvage pathway. ACS Chem. Biol. 7 (4), 753–760. doi:10.1021/cb200511t
Prescher, J. A., and Bertozzi, C. R. (2005). Chemistry in living systems. Nat. Chem. Biol. 1 (1), 13–21. doi:10.1038/nchembio0605-13
Rostovtsev, V. V., Green, L. G., Fokin, V. V., and Sharpless, K. B. (2002). A stepwise huisgen cycloaddition process: copper(I)-Catalyzed regioselective "ligation" of azides and terminal alkynes. Angew. Chem. Int. Ed. 41 (14), 2596–2599. doi:10.1002/1521-3773(20020715)41:14<2596::AID-ANIE2596>3.0.CO;2-4
Saxon, E., and Bertozzi, C. R. (2000). Cell surface engineering by a modified staudinger reaction. Science 287 (5460), 2007. doi:10.1126/science.287.5460.2007
Schart, V. F., Hassenrück, J., Späte, A.-K., Dold, J. E. G. A., Fahrner, R., and Wittmann, V. (2019). Triple orthogonal labeling of glycans by applying photoclick chemistry. ChemBioChem 20 (2), 166–171. doi:10.1002/cbic.201800740
Sletten, E. M., and Bertozzi, C. R. (2009). Bioorthogonal chemistry: fishing for selectivity in a sea of functionality. Angew. Chem. Int. Ed. 48 (38), 6974–6998. doi:10.1002/anie.200900942
Sminia, T. J., Zuilhof, H., and Wennekes, T. (2016). Getting a grip on glycans: a current overview of the metabolic oligosaccharide engineering toolbox. Carbohydr. Res. 435, 121–141. doi:10.1016/j.carres.2016.09.007
Späte, A. K., Bußkamp, H., Niederwieser, A., Schart, V. F., Marx, A., and Wittmann, V. (2014a). Rapid labeling of metabolically engineered cell-surface glycoconjugates with a carbamate-linked cyclopropene reporter. Bioconjug. Chem. 25 (1), 147–154. doi:10.1021/bc4004487
Späte, A. K., Schart, V. F., Häfner, J., Niederwieser, A., Mayer, T. U., and Wittmann, V. (2014b). Expanding the scope of cyclopropene reporters for the detection of metabolically engineered glycoproteins by Diels-Alder reactions. Beilstein J. Org. Chem. 10, 2235–2242. doi:10.3762/bjoc.10.232
Späte, A. K., Schart, V. F., Schöllkopf, S., Niederwieser, A., and Wittmann, V. (2014c). Terminal alkenes as versatile chemical reporter groups for metabolic oligosaccharide engineering. Chem. Eur. J. 20 (50), 16502–16508. doi:10.1002/chem.201404716
Späte, A. K., Dold, J. E. G. A., Batroff, E., Schart, V. F., Wieland, D. E., Baudendistel, O. R., et al. (2016). Exploring the potential of norbornene-modified mannosamine derivatives for metabolic glycoengineering. ChemBioChem 17 (14), 1374–1383. doi:10.1002/cbic.201600197
Stairs, S., Neves, A. A., Stöckmann, H., Wainman, Y. A., Ireland-Zecchini, H., Brindle, K. M., et al. (2013). Metabolic glycan imaging by isonitrile-tetrazine click chemistry. ChemBioChem 14 (9), 1063–1067. doi:10.1002/cbic.201300130
Taylor, M. T., Blackman, M. L., Dmitrenko, O., and Fox, J. M. (2011). Design and synthesis of highly reactive dienophiles for the tetrazine-trans-cyclooctene ligation. J. Am. Chem. Soc. 133 (25), 9646–9649. doi:10.1021/ja201844c
Thalhammer, F., Wallfahrer, U., and Sauer, J. (1990). Reaktivität einfacher offenkettiger und cyclischer dienophile bei Diels-Alder-reaktionen mit inversem elektronenbedarf. Tetrahedron Lett. 31 (47), 6851–6854. doi:10.1016/S0040-4039(00)97188-0
Tornøe, C. W., Christensen, C., and Meldal, M. (2002). Peptidotriazoles on solid phase: [1,2,3]-Triazoles by regiospecific copper(I)-Catalyzed 1,3-dipolar cycloadditions of terminal alkynes to azides. J. Org. Chem. 67 (9), 3057–3064. doi:10.1021/jo011148j
Vrabel, M., Kölle, P., Brunner, K. M., Gattner, M. J., López-Carrillo, V., de Vivie-Riedle, R., et al. (2013). Norbornenes in inverse electron-demand diels-alder reactions. Chem. Eur. J. 19 (40), 13309–13312. doi:10.1002/chem.201301838
Wang, H., Wang, R., Cai, K., He, H., Liu, Y., Yen, J., et al. (2017). Selective in vivo metabolic cell-labeling-mediated cancer targeting. Nat. Chem. Biol. 13 (4), 415–424. doi:10.1038/nchembio.2297
Wijnen, J. W., Zavarise, S., Engberts, J. B. F. N., and Charton, M. (1996). Substituent effects on an inverse electron demand hetero Diels−Alder reaction in aqueous solution and organic solvents: cycloaddition of substituted styrenes to di(2-pyridyl)-1,2,4,5-tetrazine. J. Org. Chem. 61 (6), 2001–2005. doi:10.1021/jo9518563
Wratil, P. R., Horstkorte, R., and Reutter, W. (2016). Metabolic glycoengineering withN-acyl side chain modified mannosamines. Angew. Chem. Int. Ed. 55 (33), 9482–9512. doi:10.1002/anie.201601123
Wu, H., and Devaraj, N. K. (2016). Inverse electron-demand diels-alder bioorthogonal reactions. Top. Curr. Chem. (Z) 374 (1), 3. doi:10.1007/s41061-015-0005-z
Wu, H., Yang, J., Šečkutė, J., and Devaraj, N. K. (2014). In Situ synthesis of alkenyl tetrazines for highly fluorogenic bioorthogonal live-cell imaging probes. Angew. Chem. Int. Ed. 53 (23), 5805–5809. doi:10.1002/anie.201400135
Xiong, D. C., Zhu, J., Han, M.-J., Luo, H.-X., Wang, C., Yu, Y., et al. (2015). Rapid probing of sialylated glycoproteins in vitro and in vivo via metabolic oligosaccharide engineering of a minimal cyclopropene reporter. Org. Biomol. Chem. 13 (13), 3911–3917. doi:10.1039/C5OB00069F
Yang, J., Šečkutė, J., Cole, C. M., and Devaraj, N. K. (2012). Live-cell imaging of cyclopropene tags with fluorogenic tetrazine cycloadditions. Angew. Chem. Int. Ed. 51 (30), 7476–7479. doi:10.1002/anie.201202122
Zhang, R., Zheng, J., and Zhang, T. (2020). In vivo selective imaging of metabolic glycosylation with a tetrazine-modified upconversion nanoprobe. RSC Adv. 10 (27), 15990–15996. doi:10.1039/D0RA01832E
Keywords: inverse-electron-demand Diels-Alder reaction, carbohydrates, metabolic engineering, bioorthogonal chemistry, tetrazines
Citation: Haiber LM, Kufleitner M and Wittmann V (2021) Application of the Inverse-Electron-Demand Diels-Alder Reaction for Metabolic Glycoengineering. Front. Chem. 9:654932. doi: 10.3389/fchem.2021.654932
Received: 17 January 2021; Accepted: 18 February 2021;
Published: 13 April 2021.
Edited by:
Laura Russo, Università di Milano-Bicocca, ItalyReviewed by:
Matthew Robert Pratt, University of Southern California, United StatesSander Izaak Van Kasteren, Leiden University, Netherlands
Copyright © 2021 Haiber, Kufleitner and Wittmann. This is an open-access article distributed under the terms of the Creative Commons Attribution License (CC BY). The use, distribution or reproduction in other forums is permitted, provided the original author(s) and the copyright owner(s) are credited and that the original publication in this journal is cited, in accordance with accepted academic practice. No use, distribution or reproduction is permitted which does not comply with these terms.
*Correspondence: Valentin Wittmann, bWFpbEB2YWxlbnRpbi13aXR0bWFubi5kZQ==
†These authors have contributed equally to this work and share first authorship