- State Key Laboratory for Chemistry and Molecular Engineering of Medicinal Resources, School of Chemistry and Pharmaceutical Sciences, Guangxi Normal University, Guilin, China
Given the fact that excessive levels of reactive oxygen species (ROS) induce damage to proteins, lipids, and DNA, various ROS-generating agents and strategies have been explored to induce cell death and tumor destruction by generating ROS above toxic threshold. Unfortunately, hypoxia in tumor microenvironment (TME) not only promotes tumor metastasis but also enhances tumor resistance to the ROS-generated cancer therapies, thus leading to ineffective therapeutic outcomes. A variety of nanotechnology-based approaches that generate or release O2 continuously to overcome hypoxia in TME have showed promising results to improve the efficacy of ROS-generated cancer therapy. In this minireview, we present an overview of current nanomaterial-based strategies for advanced cancer therapy by modulating the hypoxia in the TME and promoting ROS generation. Particular emphasis is put on the O2 supply capability and mechanism of these nanoplatforms. Future challenges and opportunities of design consideration are also discussed. We believe that this review may provide some useful inspiration for the design and construction of other advanced nanomaterials with O2 supply ability for overcoming the tumor hypoxia-associated resistance of ROS-mediated cancer therapy and thus promoting ROS-generated cancer therapeutics.
Introduction
ROS (including singlet oxygen (1O2), superoxide radicals (O2•−), hydroxyl radicals (•OH), and peroxides (O22−)) play a concentration-dependent role in physiological activity (Gorrini et al., 2013). Low to moderate levels of ROS regulate cell signaling and promote cell proliferation, and elevated levels of cellular ROS are one of the unique characteristics of cancer, whereas excessive ROS will induce nonspecific damage to proteins, lipids, and DNA. Because of the heightened basal level of ROS in cancer cells, cancer cells are more susceptible to exogenous ROS, compared to normal cells that maintain redox homeostasis (Yang B. et al., 2019). Therefore, modulation of the ROS level at cancer cells has been emerging as promising strategy for the tumor destruction by generating ROS above toxic threshold. Hypoxia, mild acid, and overexpressed H2O2 are three characteristic features of tumor microenvironment (TME) (Dai et al., 2017; Kwon et al., 2019). Because of the aggressive proliferation of cancer cells and the insufficient blood supply in tumors, the O2 supply in solid tumors was usually insufficient (partial pressure of O2 < 2.5 mmHg). Hypoxia in TME not only promotes tumor metastasis but also enhances tumor resistance to the ROS-generated cancer therapies, such as photodynamic therapy (PDT), radiation therapy (RT), chemotherapy, chemodynamic therapy (CDT), and sonodynamic therapy (SDT), thus leading to ineffective therapeutic outcomes. Tumor oxygenation that aims at greatly increasing the oxygen concentrations in hypoxic tumors has been demonstrated to be an effective strategy to overcome tumor hypoxia and enhance the sensibility of hypoxic tumors toward the ROS-generated cancer therapy (Li et al., 2018; Yang B. et al., 2019).
To relieve hypoxia, hyperbaric oxygen therapy, which involves the breath of pure O2 in a pressurized chamber, has been developed. Unfortunately, its extensive application is limited by the intrinsic side effects including hyperoxic seizures and barotrauma as a result of the overproduced ROS in normal tissues (Kim et al., 2017). Also, angiogenesis inhibitors have been applied to transiently normalize the tumor vasculatures and suppress the consumption of O2. However, the oxygenation improvement resulting from the normalization of vessels only lasted for a few days (Liu J. N. et al., 2017). Promoted by recent advances in nanotechnology, a variety of nanotechnology-based approaches that generate or release O2 continuously to overcome hypoxia in TME have showed promising results to improve the efficacy of ROS-generated cancer therapy. In this minireview, we present an overview of current nanotechnology-based strategies for advanced cancer therapy by modulating the hypoxia in TME and promoting the generation of ROS. To amplify the therapeutic outcomes, the approach of modulating tumor hypoxia was usually applied in combination with other therapeutic/theranostic modalities. This minireview mainly focused on the O2 supply ability and mechanism of these nanoplatforms. Future challenges and opportunities of design consideration are also discussed and summarized.
Tumor Hypoxia-Regulating Approaches Based on Nanotechnology
Based on their different mechanisms and involved materials, nanotechnology-based tumor hypoxia-regulating approaches can be classified into the following categories: delivering O2 by natural or artificial oxygen-carrying materials, the hydrolysis of exogenous peroxide, catalytic decomposition of intracellular H2O2 by utilizing catalase or catalase-like nanozymes, and generating O2 by water-splitting photocatalysts.
Delivering O2 by Natural and Artificial Oxygen-Carrying Materials
Red blood cells (RBCs), the primary source of O2 in mammals, contain 270 million hemoglobin (Hb) molecules per cell; each Hb molecule binds up to four O2. Hb allows efficient binding of O2 under high O2 pressure and rapid O2 release under hypoxic environment. Because of the good biocompatibility and long circulation, RBCs have been widely investigated as biological drug carriers and O2 shuttles for cancer therapy (Squires, 2002; Wang et al., 2013; Wang et al., 2014; Sun et al., 2015; Wang et al., 2017). Tang et al. (2016) demonstrated that RBCs tethered with photosensitizers (ZnF16Pc) onto the RBCs surface (P-FRT-RBCs) could realize the codelivery of O2 and photosensitizers (Figure 1A). The sustained O2 supply adjacent to photosensitizers by RBCs enabled efficient PDT even under hypoxic conditions. However, the micrometer sizes of RBCs may limit their extravascular diffusion ability and reduce their chance to approach tumor cell. The oxygen-carrying ability of RBCs is limited by the inherent oxygen-binding ability of Hb. However, cell-free Hb suffers from severe problems, including short circulation time, potential side effect, and poor stability. Hb-based O2 carriers via chemical modification or encapsulation with biodegradable materials could overcome the disadvantages of cell-free Hb and demonstrate the similar oxygen-carrying capability as that of natural RBCs (Gundersen and Palmer, 2008; Duan et al., 2012; Jia et al., 2012; Paciello et al., 2016; Zhou et al., 2016; Cao et al., 2018; Jansman and Hosta-Rigau, 2018; Yu et al., 2018; Hu et al., 2020). Compared to RBCs with micrometer sizes, nanodimensional Hb-based O2 carriers can perfuse tumor tissues within the narrow vascular structure and thus can supply more O2 in hypoxic tumor (Jia et al., 2016; Luo et al., 2016; Zhao et al., 2016). Inspired by the biological nature of RBCs, Liu W. L. et al. (2018) developed an aggressive man-made RBC (AmmRBC) as oxygen self-supplied PDT system to combat the hypoxia-mediated resistance of tumors to PDT (Figure 1B). This biomimetic platform was prepared by encapsulating methylene blue (MB) adsorbed Hb-polydopamine complex into the biovesicle engineered from the recombined RBC membranes. Polydopamine played the role of the antioxidative enzymes to prevent Hb from the oxidation damage during the circulation.
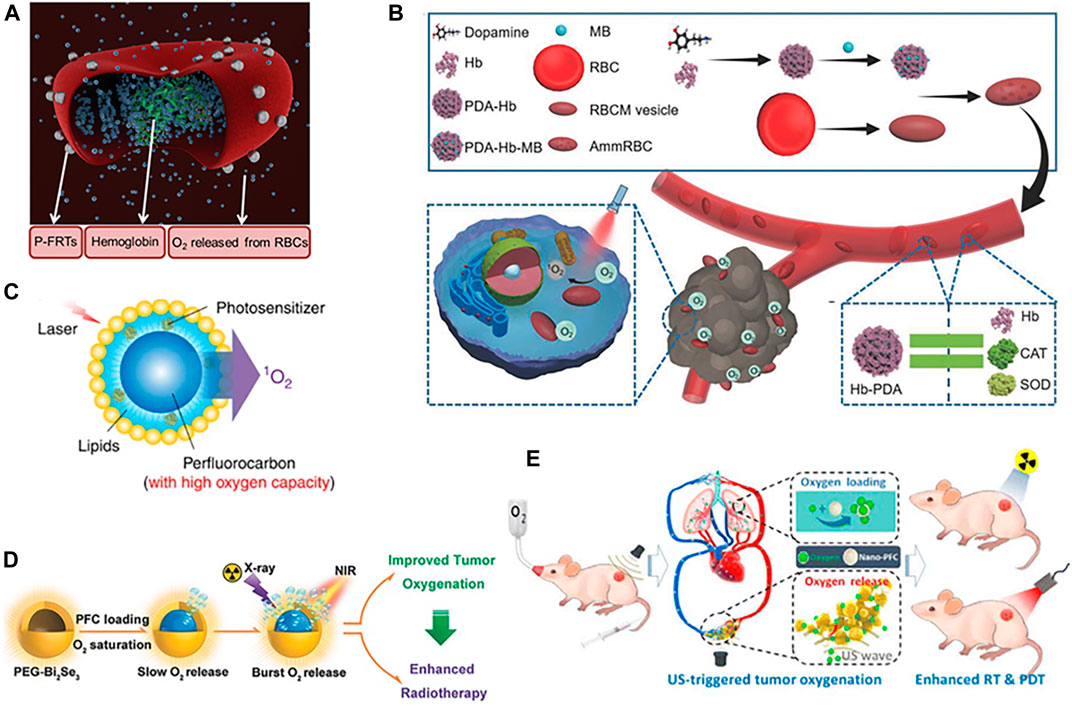
FIGURE 1. (A) Schematic illustration of the formation and working mechanism of P-FRT-RBCs (Tang et al., 2016) (Copyright 2016, reproduced with permission from John Wiley and Sons). (B) Schematic illustration of AmmRBCs that accumulate in the tumor site and boost 1O2 generation for enhanced PDT. Polydopamine (PDA) in AmmRBC functions like CAT and superoxide dismutase (SOD) in RBCs to protect Hb from oxidant damage during the circulation (Liu W. L. et al., 2018) (Copyright 2018, reproduced with permission from John Wiley and Sons). (C) Schematic illustration of the structure and design of the Oxy-PDT agent. Photosensitizer and perfluorocarbon are coencapsulated by lipids. Photosensitizers are uniformly dispersed inside the lipid monolayer and PFC in the core of the nanoparticle. When irradiated by laser, photosensitizer (PS) transfers energy to the oxygen enriched in PFC, producing 1O2 (Cheng et al., 2015) (Copyright 2015, reproduced with permission from Nature Publishing Group). (D) Schematic illustration of hollow PEG-Bi2Se3 nanoparticles with PFC loading as an oxygen carrier and the burst release of oxygen under stimulation by a NIR laser (Song G. S., Liang C. et al., 2016) (Copyright 2016, reproduced with permission from John Wiley and Sons). (E) Schematic illustration of the mechanism of US-triggered local oxygenation in the tumor using nano-PFC as the oxygen shuttle (Song X. J. et al., 2016) (Copyright 2016, reproduced with permission from American Chemical Society).
In recent years, an artificial blood product, perfluorocarbon (PFC) compounds with good biocompatibility and high oxygen dissolving ability, has been extensively used as O2 carriers to modulate the hypoxic TME (Squires, 2002; Lee et al., 2015; Que et al., 2016; Liang et al., 2020). By loading a near-infrared photosensitizer (IR780) into PFCs nanodroplets, Cheng et al. (2015) developed an oxygen self-enriching PDT (Oxy-PDT) nanoplatform (Figure 1C). Owing to the higher oxygen capacity and longer 1O2 lifetime of PFCs, the PDT effect of the loaded photosensitizer was significantly enhanced. Gao et al. (2017) reported erythrocyte-membrane coated PFC nanoparticles as artificial RBCs to deliver O2 and enhance radiation response.
Though having high oxygen solubility, PFC releases O2 simply by diffusion through the O2 concentration gradient, usually resulting in a low delivery efficiency. Using near-infrared (NIR) light or ultrasound (US) as trigger could accelerate the release of O2 and promote the tumor oxygenation (Song G. S., Liang C. et al., 2016; Chen et al., 2017). Song et al. utilized the photothermal effect of Bi2Se3 induced by NIR laser irradiation to trigger the burst release of O2 from PFC loaded inside the hollow Bi2Se3 nanoparticles, thereby greatly promoting the tumor oxygenation and overcoming the hypoxia-associated radioresistance of tumors (Song G. S., Liang C. et al., 2016) (Figure 1D). Song X. J. et al. (2016) used an external low-frequency/low-power US treatment to trigger the release of O2 from nano-PFC to relief tumor hypoxia for enhanced PDT and RT (Figure 1E). Given that several formulations of PFC emulsions have been either approved for clinical application or in late-phase clinical trials as blood substitutes, PFC-based nanomaterials may hold great potential in cancer treatment for future clinical translation. However, extensive exposure to PFCs may cause some side effects, including hypotension, cutaneous flushing, fever, pulmonary hypertension, chest tightness, and elevated central venous pressure (Zhou et al., 2016).
Hydrolysis of Exogenous Peroxide to Produce O2
Because the hydrolysis of peroxide will generate O2, various peroxides (such as hydrogen peroxide, calcium peroxide, sodium percarbonate, and pyridine endoperoxides) have been utilized as O2-producing materials (Harrison et al., 2007; Oh et al., 2009; Wang et al., 2011; Li et al., 2012; Pedraza et al., 2012; Benz et al., 2013). However, the release of O2 by the hydrolysis of exogenous peroxide in the absence of a catalyst or trigger was usually slow and limited. It will be more favorable if on-demand and uniform O2 delivery to the cells for a sufficiently long time period can be achieved (Liu J. N. et al., 2017). Huang et al. (2016) reported an implantable oxygen-generating depot by coloading CaO2 and catalase into the Ca2+-crosslinked microencapsulated alginate pellets. Catalase (CAT) in the alginate pellets could catalyze the breakdown of H2O2 into O2, whereas the Ca2+-crosslinked alginate matrix could temper the hydrolytic reactivity of CaO2/catalase by limiting the infiltration of H2O into the pellets, thus prolonging the generation of O2. Upon implantation close to the tumor, this in situ oxygen-generating depot effectively alleviated the hypoxic regions in tumor and thus resulted in increased chemotherapeutic effect of DOX by promoting ROS production. Liu L. H. et al. (2017) encapsulated CaO2 and methylene blue (MB) into liposome to fabricate an O2 self-sufficient nanoplatform (LipoMB/CaO2) to enhance PDT efficacy in hypoxic tumor. CaO2 inside liposomes could react with H2O or weak acid to release O2 slowly. Upon laser irradiation, 1O2 activated by the photosensitizer could induce lipid peroxidation to break the liposome and then enlarge the contact area of CaO2 with H2O, resulting in accelerated O2 release.
Catalytic Decomposition of Intracellular H2O2 by Utilizing Catalase or Catalase-Like Nanozymes.
Due to the overexpressed H2O2 in tumor (100 μM–1 mM), various natural enzymes (catalase) and metals or metal-oxide based nanozymes have been applied to overcome tumor hypoxia by catalyzing the in situ transformation of endogenous H2O2 to O2. Catalase (CAT) is a catalytic enzyme with a high turnover number to decompose H2O2 into O2 and thus can be employed to relieve tumor hypoxia. However, the nonnegligible disadvantages of CAT, including immunogenicity, the protease-induced degradation, and short half-life, usually restrict its in vivo functions after systemic administration. Chemical modification or encapsulating CAT within inorganic or polymer nanostructures has been demonstrated to be an effective approach to overcome these limitations (Chen et al., 2015; Cheng et al., 2016; Zhang R. et al., 2017; Li et al., 2017). Chen et al. (2014) chose PLGA nanoparticles as a carrier to load CAT and platinum anticancer drug. Synergistic release of anticancer drugs and O2 triggered by H2O2 could overcome hypoxia-induced multidrug resistance and thus resulted in improved therapeutic efficacy. By encapsulating CAT into hollow tantalum oxide (TaOx), Song et al. obtained a bionanoreactor (TaOx@Cat-PEG) combining high-Z element (Ta) and CAT for relieving tumor hypoxia and enhancing RT outcomes. The mesoporous shell of TaOx protected CAT from outside proteases to improve its stability (Song G. S., Chen Y. Y., et al., 2016). Wang H. et al. (2018) reported an in situ free radical polymerization method by using a photosensitizer (meso-tetra(p-hydroxyphenyl) porphine (THPP)) as the crosslinker to modify CAT for tumor hypoxia modulation and enhanced PDT. In the obtained CAT-THPP-PEG nanocapsules, the PEG chains polymerized on the surface of CAT could prevent the direct contact between serum proteins and CAT and thus enhanced the enzyme stability, maintained its catalytic activity, and reduced its immunogenicity. Phua et al. (2019) reported that the integration of hyaluronic acid (HA) with CAT could not only improve the physiological stability of the system but also enable active targeting to tumors. The photosensitizer (Ce6)-loaded nanosystem (HA-CAT@aCe6) could target CD44-overexpressed cancer cells, relieve hypoxia by converting endogenous H2O2 to O2, and consequently improve PDT efficacy.
Apart from natural enzymes, various nanomaterial-based artificial enzymes show catalase-like activity; one of the typical representatives is MnO2. Various MnO2 nanostructures have been designed and incorporated into multifunctional nanoplatforms to induce the decomposition of endogenous H2O2 into O2, thus alleviating tumor hypoxia and improving therapeutic efficacy (Prasad et al., 2014; Fan et al., 2015; Abbasi et al., 2016; Yi et al., 2016; Wang Z. et al., 2018). Moreover, MnO2 could be decomposed into soluble Mn2+ in TME, thus reducing unwanted in vivo accumulation and long-term toxicity (Zhu et al., 2016). The released Mn2+ could mediate the Fenton-like reaction to convert H2O2 into the highly reactive •OH, further enhancing the therapeutic potency by introducing extra CDT (Sun et al., 2020). Apart from the abovementioned benefits, MnO2 could also be used for drug release, glutathione (GSH) depletion, the regulation of pH, and T1-weighted magnetic resonance (MR) imaging, consequently achieving multimodal theranostic effects and tumor-specific enhanced combination therapy (Fan et al., 2016; Zhang C. et al., 2017; Zhu P. et al., 2018; Zhu H. et al., 2018; Yang G. et al., 2018; Zhang et al., 2019; Pu et al., 2020). For example, Yang et al. (2017) designed an intelligent theranostic platform based on hollow mesoporous MnO2 (HMnO2) nanoshells for tumor-targeted drug delivery, pH-triggered controllable release, and TME-responsive generation of O2 to alleviate tumor hypoxia. Ce6 and DOX were coloaded into HMnO2 to achieve combined chemo-photodynamic therapy (Figure 2A). Fluorescence signal of Ce6 and T1-weighted MR signals of the released Mn2+ were applied to track the nanoparticles after the injection. Despite great progresses and promising results, the rapid consumption of MnO2 during the reaction in TME may restrict its extensive application to a certain extent (Zhang et al., 2018).
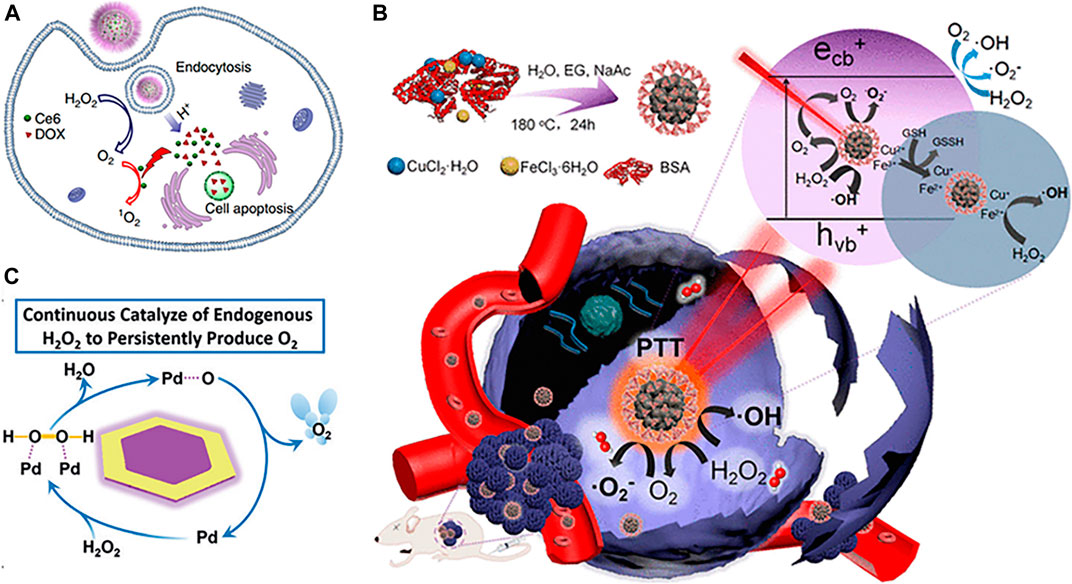
FIGURE 2. (A) Schematic illustration of H-MnO2-PEG loaded with DOX and Ce6 for pH-responsive drug delivery and oxygen-elevated PDT (Yang et al., 2017) (Copyright 2017, reproduced with permission from Nature Publishing Group). (B) Schematic illustration of synthetic process and therapeutic mechanism of CuFe2O4 nanospheres (Liu Y. et al., 2018) (Copyright 2018, reproduced with permission from American Chemical Society). (C) Schematic illustration of Pd@Au for catalysis of H2O2 and continuous production of O2 (Yang Y. et al., 2019) (Copyright 2016, reproduced with permission from John Wiley and Sons).
Differentiated from the aforementioned self-sacrificing MnO2, ferrite materials with catalase-like activity and enhanced stability could be served as a superior candidate for continuous O2 supply. For example, Kim et al. (2017) developed continuous O2-evolving MnFe2O4 nanoparticle-anchored mesoporous silica nanoparticles to enhance the PDT effects against hypoxic tumor. The MnFe2O4 nanoparticles were not consumed during the catalytic reaction and thus could continuously catalyze H2O2 into O2, enabling the subsequent ROS generation from activated photosensitizer Ce6. Yin et al. (2019) reported that MnFe2O4@MOFs core-shell nanostructure exhibited dual catalytic ability in continuously triggering the decomposition of H2O2 to release O2 and persistently depleting endogenous GSH, resulting in improved PDT. Also, MnFe2O4 nanoparticles were not consumed during the reaction. Liu Y. et al. (2018) developed CuFe2O4 nanospheres that integrated PDT, PTT, photoenhanced CDT, and MR imaging functions along with TME-modulating capacity. The CuFe2O4 nanospheres regulated the TME through the decomposition of H2O2 to O2 and the depletion of GSH, which relieved the tumor hypoxia and antioxidant capability, thus further improving the photoenhanced CDT and PDT efficiency (Figure 2B).
Various Fe-doped nanoplatforms have been reported to catalyze the conversion of endogenous H2O2 to O2 and thus could enhance the therapeutic effects against hypoxic tumor, including Fe-doped polydiaminopyridine nanofusiforms (Fe-PDAP) (Bai et al., 2018), FeIII doped C3N4 nanosheets (Ma et al., 2016), and Fe3+-driven assembly of fluorenylmethyloxycarbonyl (Fmoc) protected amino acids (Fmoc-Cys/Fe) (Li Y. et al., 2020). Lan et al. (2018) developed a nanoscale MOF (Fe-TBP, constructed from Fe3O clusters and 5,10,15,20-tetra(p-benzoato)porphyrin (TPB)) as a nanophotosensitizer to overcome tumor hypoxia for PDT-primed cancer immunotherapy. Intracellular H2O2 could be decomposed by the Fe3O clusters to generate O2 through a Fenton-like reaction, whereas the produced O2 was converted to cytotoxic singlet oxygen (1O2) by photoexcited porphyrins. Prussian blue (PB), a clinical medicine approved by U.S. FDA for the treatment of radioactive exposure, has been proven with catalase-like activity (Cai et al., 2016; Zhou et al., 2018). Yang Z. L. et al. (2018) fabricated a PB-based integrated nanoplatform to elevate O2 and ROS for highly efficient PDT.
Other noble metals or metal oxide–based nanozymes with catalase-like activity have also been applied to overcome tumor hypoxia via H2O2-activated catalytic O2 generation, thereby augmenting effect of ROS-generated cancer therapy, such as CeO2 (Dong et al., 2020), RuO2 (Huang et al., 2020; Xu et al., 2020), V2O5 (Li C. et al., 2020), mesoporous manganese cobalt oxide derived from MOFs (Wang et al., 2019), Pd@Pt nanoplates (Wei et al., 2018), gold nanoclusters (Liu, C. P., et al., 2017), MOF–Au nanohybrid (He et al., 2019), Pt nanoparticles decorated on MOFs (Zhang et al., 2018), Pt-based core-shell nanoplatform (Wang X. S. et al., 2018), two-dimensional Pd@Au bimetallic core-shell nanostructure (Yang Y. et al., 2019), etc. By taking the advantage of dual enzyme-mimic catalytic activity of ultrasmall CeO2, Dong et al. (2020) fabricated a nanocomposite with hyperthermia-enhanced peroxidase-like activity, catalase-mimic activity, and GSH depletion for efficient tumor therapy in the NIR-II window. Huang et al. (2020) reported that a multifunctional artificial metalloprotein nanoanalogue, RuO2-hybridized ovalbumin (OVA) nanoanalogues, not only exhibited photothermal/photodynamic effect under NIR light irradiation but also effectively alleviated tumor hypoxia via catalysis of intracellular H2O2 to produce O2, thereby concurrently enhancing PDT and reversing the immunosuppressive TME. Yang B. et al. (2019) reported a two-dimensional Pd@Au core-shell nanostructure (TPAN) that could continuously catalyze endogenous H2O2 to generate O2 for relieving tumor hypoxia to overcome hypoxia-induced RT resistance. Moreover, the catalytic activity of TPAN toward H2O2 could be enhanced via the surface plasmon resonance effect triggered by NIR-II laser irradiation (Figure 2C). Wei et al. (2018) reported that Pd@Pt-PEG-Ce6 nanocomposite could not only deliver photosensitizers to tumor sites but also trigger the decomposition of endogenous H2O2 to produce O2 for a long period of time. Moreover, the moderate photothermal effect of Pd@Pt-PEG-Ce6 under 808 nm laser irradiation accelerated its catalytic decomposition of H2O2 to O2. Liu C. P. et al. (2017) reported that the amine-terminated, PAMAM dendrimer-encapsulated gold nanoclusters (AuNCs-NH2) can produce O2 to improve PDT via the catalase-like activity. Importantly, AuNCs-NH2 exhibited the catalase-like activity over a broad pH range (pH 4.8–7.4).
Generating O2 by Water-Splitting Photocatalysts
Compared to the limited intracellular concentration of H2O2, H2O is the most abundant compound in living organisms. Consequently, using H2O as an alternative O2-generating reactant, the water-splitting strategy could provide unlimited raw materials for in vivo O2 release. As a typical paradigm, Zheng et al. (2016) reported the use of carbon-dot-decorated C3N4 nanocomposite as a water-splitting catalyst to produce O2 to overcome tumor hypoxia and improve the PDT effect. The carbon dots were doped to decrease the band gap of C3N4, and a 630 nm laser was applied as the trigger to induce the water splitting. Chen et al. (2020) reported that in situ photocatalysis of TiO porphyrin encapsulated in folate liposome could not only conquer tumor hypoxia but also generate sufficient ROS to suppress the tumor growth. Analogous to the aforementioned photocatalysts, the photosensitizer nanoparticle-loaded photosynthetic bacteria were developed for tumor-targeted photosensitizer (indocyanine green, ICG) delivery and in situ photocatalyzed O2 generation. This biomimetic system combined the photosynthetic capability of Synechococcus 7942 (a natural photosynthetic cyanobacterium) and the theranostic effect of ICG-encapsulated human serum albumin nanoparticles (Liu et al., 2020). Since hypoxic tumors are usually located in the deep tissues, the penetration depth of the laser is a limitation.
Conclusion and Challenges
We herein present an overview of current strategies to overcome the tumor hypoxia in ROS-generated cancer therapy. Despite great progresses and promising results, most attempts still remain at early stages of development. These strategies suffer from some disadvantages, for example, side effects after intravenous injection, H2O2 dependence in H2O2-mediated O2 production, rapid consumption or easy inactivation/instability of natural enzyme and nanozymes, and poor light penetration in photoactivated O2 production. Moreover, to achieve enhanced therapeutic efficacy, integration of multiple therapeutic/diagnostic capability and oxygen-supply ability into one nanosystem has become the most commonly used strategy to treat hypoxic tumors. Consequently, complicated and tedious preparation procedures are usually needed. To maximize their capabilities and minimize the side effects, toxicity and immunogenicity of all the involved components should be comprehensively evaluated before clinical trials. In addition, the degradability of the materials should be guaranteed, which will enable the body to clear them after performing the designated pharmacological functions.
Author Contributions
CR conceived the review topic and wrote the manuscript. All authors contributed to the final manuscript and approved the submitted version.
Funding
This work was supported by National Natural Science Foundation of China (51762007) and the Natural Science Foundation of Guangxi Province (2017GXNSFBA198208, GuikeAD19110018).
Conflict of Interest
The authors declare that the research was conducted in the absence of any commercial or financial relationships that could be construed as a potential conflict of interest.
References
Abbasi, A. Z., Gordijo, C. R., Amini, M. A., Maeda, A., Rauth, A. M., DaCosta, R. S., et al. (2016). Hybrid manganese dioxide nanoparticles potentiate radiation therapy by modulating tumor hypoxia. Cancer Res. 76, 6643–6656. doi:10.1158/0008-5472.CAN-15-3475
Bai, J., Jia, X., Zhen, W., Cheng, W., and Jiang, X. (2018). A facile ion-doping strategy to regulate tumor microenvironments for enhanced multimodal tumor theranostics. J. Am. Chem. Soc. 140, 106–109. doi:10.1021/jacs.7b11114
Benz, S., Nötzli, S., Siegel, J. S., Eberli, D., and Jessen, H. J. (2013). Controlled oxygen release from pyridone endoperoxides promotes cell survival under anoxic conditions. J. Med. Chem. 56, 10171–10182. doi:10.1021/jm4016137
Cai, X., Gao, W., Zhang, L., Ma, M., Liu, T., Du, W., et al. (2016). Enabling prussian blue with tunable localized surface plasmon resonances: simultaneously enhanced dual-mode imaging and tumor photothermal therapy. ACS Nano 10, 11115–11126. doi:10.1021/acsnano.6b05990
Cao, H., Wang, L., Yang, Y., Li, J., Qi, Y., Li, Y., et al. (2018). An assembled nanocomplex for improving both therapeutic efficiency and treatment depth in photodynamic therapy. Angew. Chem. Int. Ed. Engl. 57, 7759–7763. doi:10.1002/anie.201802497
Chen, H., Ma, A., Yin, T., Chen, Z., Liang, R., Pan, H., et al. (2020). In Situ photocatalysis of TiO-Porphyrin-Encapsulated nanosystem for highly efficient oxidative damage against hypoxic tumors. ACS Appl. Mater. Inter. 12, 12573–12583. doi:10.1021/acsami.0c00921
Chen, H., He, W., and Guo, Z. (2014). An H2O2-responsive nanocarrier for dual-release of platinum anticancer drugs and O2: controlled release and enhanced cytotoxicity against cisplatin resistant cancer cells. Chem. Commun. 50, 9714–9717. doi:10.1039/c4cc03385j
Chen, H., Tian, J., He, W., and Guo, Z. (2015). H2O2-Activatable and O2-evolving nanoparticles for highly efficient and selective photodynamic therapy against hypoxic tumor cells. J. Am. Chem. Soc. 137, 1539–1547. doi:10.1021/ja511420n
Chen, J., Luo, H., Liu, Y., Zhang, W., Li, H., Luo, T., et al. (2017). Oxygen-self-produced nanoplatform for relieving hypoxia and breaking resistance to sonodynamic treatment of pancreatic cancer. ACS Nano 11, 12849–12862. doi:10.1021/acsnano.7b08225
Cheng, H., Zhu, J.-Y., Li, S.-Y., Zeng, J.-Y., Lei, Q., Chen, K.-W., et al. (2016). An O2 self-sufficient biomimetic nanoplatform for highly specific and efficient photodynamic therapy. Adv. Funct. Mater. 26, 7847–7860. doi:10.1002/adfm.201603212
Cheng, Y., Cheng, H., Jiang, C., Qiu, X., Wang, K., Huan, W., et al. (2015). Perfluorocarbon nanoparticles enhance reactive oxygen levels and tumour growth inhibition in photodynamic therapy. Nat. Commun. 6, 8785. doi:10.1038/ncomms9785
Dai, Y., Xu, C., Sun, X., and Chen, X. (2017). Nanoparticle design strategies for enhanced anticancer therapy by exploiting the tumour microenvironment. Chem. Soc. Rev. 46, 3830–3852. doi:10.1039/c6cs00592f
Dong, S., Dong, Y., Jia, T., Liu, S., Liu, J., Yang, D., et al. (2020). GSH‐Depleted nanozymes with hyperthermia‐enhanced dual enzyme‐mimic activities for tumor nanocatalytic therapy. Adv. Mater. 32, 2002439. doi:10.1002/adma.202002439
Duan, L., Yan, X., Wang, A., Jia, Y., and Li, J. (2012). Highly loaded hemoglobin spheres as promising artificial oxygen carriers. ACS Nano 6, 6897–6904. doi:10.1021/nn301735u
Fan, W., Bu, W., Shen, B., He, Q., Cui, Z., Liu, Y., et al. (2015). Intelligent MnO2Nanosheets anchored with upconversion nanoprobes for concurrent pH-/H2O2-responsive UCL imaging and oxygen-elevated synergetic therapy. Adv. Mater. 27, 4155–4161. doi:10.1002/adma.201405141
Fan, H., Yan, G., Zhao, Z., Hu, X., Zhang, W., Liu, H., et al. (2016). A smart photosensitizer-manganese dioxide nanosystem for enhanced photodynamic therapy by reducing glutathione levels in cancer cells. Angew. Chem. Int. Ed. Engl. 55, 5477–5482. doi:10.1002/anie.201510748
Gao, M., Liang, C., Song, X., Chen, Q., Jin, Q., Wang, C., et al. (2017). Erythrocyte-membrane-enveloped perfluorocarbon as nanoscale artificial red blood cells to relieve tumor hypoxia and enhance cancer radiotherapy. Adv. Mater. Weinheim 29, 1701429. doi:10.1002/adma.201701429
Gorrini, C., Harris, I. S., and Mak, T. W. (2013). Modulation of oxidative stress as an anticancer strategy. Nat. Rev. Drug Discov. 12, 931–947. doi:10.1038/nrd4002
Gundersen, S. I., and Palmer, A. F. (2008). Hemoglobin-based oxygen carrier enhanced tumor oxygenation: a novel strategy for cancer therapy. Biotechnol. Prog. 24, 1353–1364. doi:10.1002/btpr.56
Harrison, B. S., Eberli, D., Lee, S. J., Atala, A., and Yoo, J. J. (2007). Oxygen producing biomaterials for tissue regeneration. Biomaterials 28, 4628–4634. doi:10.1016/j.biomaterials.2007.07.003
He, Z., Huang, X., Wang, C., Li, X., Liu, Y., Zhou, Z., et al. (2019). A catalase-like metal-organic framework nanohybrid for O2 -evolving synergistic chemoradiotherapy. Angew. Chem. Int. Ed. Engl. 58, 8752–8756. doi:10.1002/anie.201902612
Hu, J., Wang, Q., Wang, Y., You, G., Li, P., Zhao, L., et al. (2020). Polydopamine-based surface modification of hemoglobin particles for stability enhancement of oxygen carriers. J. Colloid Interf. Sci. 571, 326–336. doi:10.1016/j.jcis.2020.03.046
Huang, C. C., Chia, W. T., Chung, M. F., Lin, K. J., Hsiao, C. W., Jin, C., et al. (2016). An implantable depot that can generate oxygen in situ for overcoming hypoxia-induced resistance to anticancer drugs in chemotherapy. J. Am. Chem. Soc. 138, 5222–5225. doi:10.1021/jacs.6b01784
Huang, R., Ding, Z., Jiang, B. P., Luo, Z., Chen, T., Guo, Z., et al. (2020). Artificial metalloprotein nanoanalogues: in situ catalytic production of oxygen to enhance photoimmunotherapeutic inhibition of primary and abscopal tumor growth. Small 16, 2004345. doi:10.1002/smll.202004345
Jansman, M. M. T., and Hosta-Rigau, L. (2018). Recent and prominent examples of nano- and microarchitectures as hemoglobin-based oxygen carriers. Adv. Colloid Interf. Sci. 260, 65–84. doi:10.1016/j.cis.2018.08.006
Jia, Y., Duan, L., and Li, J. (2016). Hemoglobin-based nanoarchitectonic assemblies as oxygen carriers. Adv. Mater. Weinheim 28, 1312–1318. doi:10.1002/adma.201502581
Jia, Y., Cui, Y., Fei, J., Du, M., Dai, L., Li, J., et al. (2012). Construction and evaluation of hemoglobin-based capsules as blood substitutes. Adv. Funct. Mater. 22, 1446–1453. doi:10.1002/adfm.201102737
Kim, J., Cho, H. R., Jeon, H., Kim, D., Song, C., Lee, N., et al. (2017). Continuous O2-evolving MnFe2O4 nanoparticle-anchored mesoporous silica nanoparticles for efficient photodynamic therapy in hypoxic cancer. J. Am. Chem. Soc. 139, 10992–10995. doi:10.1021/jacs.7b05559
Kwon, S., Ko, H., You, D. G., Kataoka, K., and Park, J. H. (2019). Nanomedicines for reactive oxygen species mediated approach: an emerging paradigm for cancer treatment. Acc. Chem. Res. 52, 1771–1782. doi:10.1021/acs.accounts.9b00136
Lan, G., Ni, K., Xu, Z., Veroneau, S. S., Song, Y., and Lin, W. (2018). Nanoscale metal-organic framework overcomes hypoxia for photodynamic therapy primed cancer immunotherapy. J. Am. Chem. Soc. 140, 5670–5673. doi:10.1021/jacs.8b01072
Lee, H. Y., Kim, H. W., Lee, J. H., and Oh, S. H. (2015). Controlling oxygen release from hollow microparticles for prolonged cell survival under hypoxic environment. Biomaterials 53, 583–591. doi:10.1016/j.biomaterials.2015.02.117
Li, S. Y., Cheng, H., Xie, B. R., Qiu, W. X., Zeng, J. Y., Li, C. X., et al. (2017). Cancer cell membrane camouflaged cascade bioreactor for cancer targeted starvation and photodynamic therapy. ACS nano 11, 7006–7018. doi:10.1021/acsnano.7b02533
Li, X., Kwon, N., Guo, T., Liu, Z., and Yoon, J. (2018). Innovative strategies for hypoxic-tumor photodynamic therapy. Angew. Chem. Int. Ed. Engl. 57, 11522–11531. doi:10.1002/anie.201805138
Li, Z., Guo, X., and Guan, J. (2012). An oxygen release system to augment cardiac progenitor cell survival and differentiation under hypoxic condition. Biomaterials 33, 5914–5923. doi:10.1016/j.biomaterials.2012.05.012
Li, C., Zheng, X., Chen, W., Ji, S., Yuan, Y., and Jiang, X. (2020). Tumor microenvironment-regulated and reported nanoparticles for overcoming the self-confinement of multiple photodynamic therapy. Nano Lett. 20, 6526–6534. doi:10.1021/acs.nanolett.0c02272
Li, Y., Sun, P., Zhao, L., Yan, X., Ng, D. K. P., and Lo, P. C. (2020). Ferric ion driven assembly of catalase-like supramolecular photosensitizing nanozymes for combating hypoxic tumors. Angew. Chem. Int. Ed. Engl. 59, 23228–23238. doi:10.1002/anie.202010005
Liang, X., Chen, M., Bhattarai, P., Hameed, S., and Dai, Z. (2020). Perfluorocarbon@porphyrin nanoparticles for tumor hypoxia relief to enhance photodynamic therapy against liver metastasis of colon cancer. ACS Nano 14, 13569–13583. doi:10.1021/acsnano.0c05617
Liu, L., He, H., Luo, Z., Zhou, H., Liang, R., Pan, H., et al. (2020). In Situ photocatalyzed oxygen generation with photosynthetic bacteria to enable robust immunogenic photodynamic therapy in triple‐negative breast cancer. Adv. Funct. Mater. 30, 1910176. doi:10.1002/adfm.201910176
Liu, C. P., Wu, T. H., Liu, C. Y., Chen, K. C., Chen, Y. X., Chen, G. S., et al. (2017). Self-supplying O2 through the catalase-like activity of gold nanoclusters for photodynamic therapy against hypoxic cancer cells. Small 13. doi:10.1002/smll.201700278
Liu, J. N., Bu, W., and Shi, J. (2017). Chemical design and synthesis of functionalized probes for imaging and treating tumor hypoxia. Chem. Rev. 117, 6160–6224. doi:10.1021/acs.chemrev.6b00525
Liu, L. H., Zhang, Y. H., Qiu, W. X., Zhang, L., Gao, F., Li, B., et al. (2017). Dual-stage light amplified photodynamic therapy against hypoxic tumor based on an O2 self-sufficient nanoplatform. Small 13. doi:10.1002/smll.201701621
Liu, W. L., Liu, T., Zou, M. Z., Yu, W. Y., Li, C. X., He, Z. Y., et al. (2018). Aggressive man-made red blood cells for hypoxia-resistant photodynamic therapy. Adv. Mater. 30, e1802006. doi:10.1002/adma.201802006
Liu, Y., Zhen, W., Jin, L., Zhang, S., Sun, G., Zhang, T., et al. (2018). All-in-one theranostic nanoagent with enhanced reactive oxygen species generation and modulating tumor microenvironment ability for effective tumor eradication. ACS nano 12, 4886–4893. doi:10.1021/acsnano.8b01893
Luo, Z., Zheng, M., Zhao, P., Chen, Z., Siu, F., Gong, P., et al. (2016). Self-monitoring artificial red cells with sufficient oxygen supply for enhanced photodynamic therapy. Sci. Rep. 6, 23393. doi:10.1038/srep23393
Ma, Z., Zhang, M., Jia, X., Bai, J., Ruan, Y., Wang, C., et al. (2016). FeIII -doped two-dimensional C3N4 nanofusiform: a new O2 -evolving and mitochondria-targeting photodynamic agent for MRI and enhanced antitumor therapy. Small 12, 5477–5487. doi:10.1002/smll.201601681
Oh, S. H., Ward, C. L., Atala, A., Yoo, J. J., and Harrison, B. S. (2009). Oxygen generating scaffolds for enhancing engineered tissue survival. Biomaterials 30, 757–762. doi:10.1016/j.biomaterials.2008.09.065
Paciello, A., Amalfitano, G., Garziano, A., Urciuolo, F., and Netti, P. A. (2016). Hemoglobin-conjugated gelatin microsphere as a smart oxygen releasing biomaterial. Adv. Healthc. Mater. 5, 2655–2666. doi:10.1002/adhm.201600559
Pedraza, E., Coronel, M. M., Fraker, C. A., Ricordi, C., and Stabler, C. L. (2012). Preventing hypoxia-induced cell death in beta cells and islets via hydrolytically activated, oxygen-generating biomaterials. Proc. Natl. Acad. Sci. U.S.A. 109, 4245–4250. doi:10.1073/pnas.1113560109
Phua, S. Z. F., Yang, G., Lim, W. Q., Verma, A., Chen, H., Thanabalu, T., et al. (2019). Catalase-Integrated hyaluronic acid as nanocarriers for enhanced photodynamic therapy in solid tumor. ACS Nano 13, 4742–4751. doi:10.1021/acsnano.9b01087
Prasad, P., Gordijo, C. R., Abbasi, A. Z., Maeda, A., Ip, A., Rauth, A. M., et al. (2014). Multifunctional albumin-MnO₂ nanoparticles modulate solid tumor microenvironment by attenuating hypoxia, acidosis, vascular endothelial growth factor and enhance radiation response. ACS Nano 8, 3202–3212. doi:10.1021/nn405773r
Pu, Y., Zhou, B., Xiang, H., Wu, W., Yin, H., Yue, W., et al. (2020). Tyrosinase-activated prodrug nanomedicine as oxidative stress amplifier for melanoma-specific treatment. Biomaterials 259, 120329. doi:10.1016/j.biomaterials.2020.120329
Que, Y., Liu, Y., Tan, W., Feng, C., Shi, P., Li, Y., et al. (2016). Enhancing photodynamic therapy efficacy by using fluorinated nanoplatform. ACS Macro Lett. 5, 168–173. doi:10.1021/acsmacrolett.5b00935
Song, G. S., Chen, Y. Y., Liang, C., Yi, X., Liu, J., Sun, X., Shen, S., et al. (2016). Catalase-Loaded TaOx nanoshells as bio-nanoreactors combining high-Z element and enzyme delivery for enhancing radiotherapy. Adv. Mater. Weinheim 28, 7143–7148. doi:10.1002/adma.201602111
Song, G. S., Liang, C., Yi, X., Zhao, Q., Cheng, L., Yang, K., and Liu, Z. (2016). Perfluorocarbon-loaded hollow Bi2Se3 Nanoparticles for timely supply of oxygen under near-infrared light to enhance the radiotherapy of cancer. Adv. Mater. 28, 2716–2723. doi:10.1002/adma.201504617
Song, X. J., , Feng, L., Liang, C., Yang, K., and Liu, Z. (2016). Ultrasound triggered tumor oxygenation with oxygen-shuttle nanoperfluorocarbon to overcome hypoxia-associated resistance in cancer therapies. Nano Lett. 16, 6145–6153. doi:10.1021/acs.nanolett.6b02365
Sun, P., Deng, Q., Kang, L., Sun, Y., Ren, J., and Qu, X. (2020). A smart nanoparticle-laden and remote-controlled self-destructive macrophage for enhanced chemo/chemodynamic synergistic therapy. ACS Nano 14, 13894–13904. doi:10.1021/acsnano.0c06290
Sun, X., Wang, C., Gao, M., Hu, A., and Liu, Z. (2015). Remotely controlled red blood cell carriers for cancer targeting and near-infrared light-triggered drug release in combined photothermal-chemotherapy. Adv. Funct. Mater. 25, 2386–2394. doi:10.1002/adfm.201500061
Tang, W., Zhen, Z., Wang, M., Wang, H., Chuang, Y. J., Zhang, W., et al. (2016). Red blood cell-facilitated photodynamic therapy for cancer treatment. Adv. Funct. Mater. 26, 1757–1768. doi:10.1002/adfm.201504803
Wang, C., Sun, X., Cheng, L., Yin, S., Yang, G., Li, Y., et al. (2014). Multifunctional theranostic red blood cells for magnetic-field-enhanced in vivo combination therapy of cancer. Adv. Mater. Weinheim 26, 4794–4802. doi:10.1002/adma.201400158
Wang, D., Wu, H., Lim, W. Q., Phua, S. Z. F., Xu, P., Chen, Q., et al. (2019). A mesoporous nanoenzyme derived from metal-organic frameworks with endogenous oxygen generation to alleviate tumor hypoxia for significantly enhanced photodynamic therapy. Adv. Mater. 31, 1901893. doi:10.1002/adma.201901893
Wang, J., Zhu, Y., Bawa, H. K., Ng, G., Wu, Y., Libera, M., et al. (2011). Oxygen-generating nanofiber cell scaffolds with antimicrobial properties. ACS Appl. Mater. Inter. 3, 67–73. doi:10.1021/am100862h
Wang, L. Y., Shi, X. Y., Yang, C. S., and Huang, D. M. (2013). Versatile RBC-derived vesicles as nanoparticle vector of photosensitizers for photodynamic therapy. Nanoscale 5, 416–421. doi:10.1039/c2nr32506c
Wang, P., Li, X., Yao, C., Wang, W., Zhao, M., El-Toni, A. M., et al. (2017). Orthogonal near-infrared upconversion co-regulated site-specific O2 delivery and photodynamic therapy for hypoxia tumor by using red blood cell microcarriers. Biomaterials 125, 90–100. doi:10.1016/j.biomaterials.2017.02.017
Wang, H., Chao, Y., Liu, J., Zhu, W., Wang, G., Xu, L., et al. (2018). Photosensitizer-crosslinked in-situ polymerization on catalase for tumor hypoxia modulation & enhanced photodynamic therapy. Biomaterials 181, 310–317. doi:10.1016/j.biomaterials.2018.08.011
Wang, X.-S., Zeng, J.-Y., Zhang, M.-K., Zeng, X., and Zhang, X.-Z. (2018). A versatile Pt-based core-shell nanoplatform as a nanofactory for enhanced tumor therapy. Adv. Funct. Mater. 28, 1801783. doi:10.1002/adfm.201801783
Wang, Z., Zhang, Y., Ju, E., Liu, Z., Cao, F., Chen, Z., et al. (2018). Biomimetic nanoflowers by self-assembly of nanozymes to induce intracellular oxidative damage against hypoxic tumors. Nat. Commun. 9, 3334. doi:10.1038/s41467-018-05798-x
Wei, J., Li, J., Sun, D., Li, Q., Ma, J., Chen, X., et al. (2018). A novel theranostic nanoplatform based on Pd@Pt-PEG-Ce6 for enhanced photodynamic therapy by modulating tumor hypoxia microenvironment. Adv. Funct. Mater. 28, 1706310. doi:10.1002/adfm.201706310
Xu, P., Wang, X., Li, T., Wu, H., Li, L., Chen, Z., et al. (2020). Biomineralization-inspired nanozyme for single-wavelength laser activated photothermal-photodynamic synergistic treatment against hypoxic tumors. Nanoscale 12, 4051–4060. doi:10.1039/c9nr08930f
Yang, G., Xu, L., Chao, Y., Xu, J., Sun, X., Wu, Y., et al. (2017). Hollow MnO2 as a tumor-microenvironment-responsive biodegradable nano-platform for combination therapy favoring antitumor immune responses. Nat. Commun. 8, 902. doi:10.1038/s41467-017-01050-0
Yang, B., Chen, Y., and Shi, J. (2019). Reactive oxygen species (ROS)-based nanomedicine. Chem. Rev. 119, 4881–4985. doi:10.1021/acs.chemrev.8b00626
Yang, G., Zhang, R., Liang, C., Zhao, H., Yi, X., Shen, S., et al. (2018). Manganese dioxide coated WS2@Fe3O4/SiO2 nanocomposites for pH-responsive MR imaging and oxygen-elevated synergetic therapy. Small 14, 1702664–1739602. doi:10.1002/smll.201702664
Yang, Y., Chen, M., Wang, B., Wang, P., Liu, Y., Zhao, Y., et al. (2019). NIR-II driven plasmon-enhanced catalysis for a timely supply of oxygen to overcome hypoxia-induced radiotherapy tolerance. Angew. Chem. Int. Ed. Engl. 58, 15069–15075. doi:10.1002/anie.201906758
Yang, Z. L., Tian, W., Wang, Q., Zhao, Y., Zhang, Y. L., Tian, Y., et al. (2018). Oxygen-evolving mesoporous organosilica coated prussian blue nanoplatform for highly efficient photodynamic therapy of tumors. Adv. Sci. (Weinh) 5, 1700847. doi:10.1002/advs.201700847
Yi, X., Chen, L., Zhong, X., Gao, R., Qian, Y., Wu, F., et al. (2016). Core-shell Au@MnO2 nanoparticles for enhanced radiotherapy via improving the tumor oxygenation. Nano Res. 9, 3267–3278. doi:10.1007/s12274-016-1205-8
Yin, S. Y., Song, G., Yang, Y., Zhao, Y., Wang, P., Zhu, L. M., et al. (2019). Persistent regulation of tumor microenvironment via circulating catalysis of MnFe2O4 @Metal-Organic frameworks for enhanced photodynamic therapy. Adv. Funct. Mater. 29, 1901417. doi:10.1002/adfm.201901417
Yu, C., Huang, X., Qian, D., Han, F., Xu, L., Tang, Y., et al. (2018). Fabrication and evaluation of hemoglobin-based polydopamine microcapsules as oxygen carriers. Chem. Commun. (Camb) 54, 4136–4139. doi:10.1039/c8cc00095f
Zhang, D., Ye, Z., Wei, L., Luo, H., and Xiao, L. (2019). Cell membrane-coated porphyrin metal-organic frameworks for cancer cell targeting and O2-evolving photodynamic therapy. ACS Appl. Mater. Inter. 11, 39594–39602. doi:10.1021/acsami.9b14084
Zhang, Y., Wang, F., Liu, C., Wang, Z., Kang, L., Huang, Y., et al. (2018). Nanozyme decorated metal-organic frameworks for enhanced photodynamic therapy. ACS nano 12, 651–661. doi:10.1021/acsnano.7b07746
Zhang, C., Chen, W.-H., Liu, L.-H., Qiu, W.-X., Yu, W.-Y., and Zhang, X.-Z. (2017). An O2 self-supplementing and reactive-oxygen-species-circulating amplified nanoplatform via H2O/H2O2 splitting for tumor imaging and photodynamic therapy. Adv. Funct. Mater. 27, 1700626. doi:10.1002/adfm.201700626
Zhang, R., Song, X., Liang, C., Yi, X., Song, G., Chao, Y., et al. (2017). Catalase-loaded cisplatin-prodrug-constructed liposomes to overcome tumor hypoxia for enhanced chemo-radiotherapy of cancer. Biomaterials 138, 13–21. doi:10.1016/j.biomaterials.2017.05.025
Zhao, P., Zheng, M., Luo, Z., Fan, X., Sheng, Z., Gong, P., et al. (2016). Oxygen nanocarrier for combined cancer therapy: oxygen-boosted ATP-responsive chemotherapy with amplified ROS lethality. Adv. Healthc. Mater. 5, 2161–2167. doi:10.1002/adhm.201600121
Zheng, D. W., Li, B., Li, C. X., Fan, J. X., Lei, Q., Li, C., et al. (2016). Carbon-dot-decorated carbon nitride nanoparticles for enhanced photodynamic therapy against hypoxic tumor via water splitting. ACS nano 10, 8715–8722. doi:10.1021/acsnano.6b04156
Zhou, B., Jiang, B. P., Sun, W., Wei, F. M., He, Y., Liang, H., et al. (2018). Water-dispersible prussian blue hyaluronic acid nanocubes with near-infrared photoinduced singlet oxygen production and photothermal activities for cancer theranostics. ACS Appl. Mater. Inter. 10, 18036–18049. doi:10.1021/acsami.8b01387
Zhou, Z., Song, J., Nie, L., and Chen, X. (2016). Reactive oxygen species generating systems meeting challenges of photodynamic cancer therapy. Chem. Soc. Rev. 45, 6597–6626. doi:10.1039/c6cs00271d
Zhu, W., Dong, Z., Fu, T., Liu, J., Chen, Q., Li, Y., et al. (2016). Modulation of hypoxia in solid tumor microenvironment with MnO2 nanoparticles to enhance photodynamic therapy. Adv. Funct. Mater. 26, 5490–5498. doi:10.1002/adfm.201600676
Zhu, H., Li, J., Qi, X., Chen, P., and Pu, K. (2018). Oxygenic hybrid semiconducting nanoparticles for enhanced photodynamic therapy. Nano Lett. 18, 586–594. doi:10.1021/acs.nanolett.7b04759
Keywords: reactive oxygen species, tumor hypoxia, cancer therapy, nanomaterials, O2 supply, tumor oxygenation
Citation: Ruan C, Su K, Zhao D, Lu A and Zhong C (2021) Nanomaterials for Tumor Hypoxia Relief to Improve the Efficacy of ROS-Generated Cancer Therapy. Front. Chem. 9:649158. doi: 10.3389/fchem.2021.649158
Received: 04 January 2021; Accepted: 12 February 2021;
Published: 19 March 2021.
Edited by:
Jianhua Liu, Second Affiliated Hospital of Jilin University, ChinaCopyright © 2021 Ruan, Su, Zhao, Lu and Zhong. This is an open-access article distributed under the terms of the Creative Commons Attribution License (CC BY). The use, distribution or reproduction in other forums is permitted, provided the original author(s) and the copyright owner(s) are credited and that the original publication in this journal is cited, in accordance with accepted academic practice. No use, distribution or reproduction is permitted which does not comply with these terms.
*Correspondence: Changping Ruan, Y3BydWFuQG1haWxib3guZ3hudS5lZHUuY24=