- School of Iron and Steel, Soochow University, Suzhou, China
Na2Fe0.6Mn0.4PO4F/C composite materials are synthesized with various carbon sources via a simple spray-drying method in this study, and the effect of carbon sources on structure, morphology, and electrochemical properties of Na2Fe0.6Mn0.4PO4F/C materials are investigated in detail. XRD and SEM results indicate that the reduction ability of carbon sources has a key impact on the structure and morphology of Na2Fe0.6Mn0.4PO4F/C composite materials. Among these Na2Fe0.6Mn0.4PO4F/C materials, the sample prepared with ascorbic acid presents a uniform hollow spherical architecture. Electrochemical analysis demonstrates that the Na2Fe0.6Mn0.4PO4F/C sample prepared with ascorbic acid has optimal electrochemical performance. The sample shows high discharge capacities of 95.1 and 48.1 mAh g−1 at 0.05C and 1C rates, respectively, and it exhibits an improved cycle stability (91.7% retention after 100 cycles at 0.5C), which are superior to Na2Fe0.6Mn0.4PO4F/C materials prepared with other carbon sources. This study demonstrates that the reduction ability of carbon sources significantly influences the electrochemical properties of fluorophosphate/C composite materials. This work also provides a promising strategy to obtain high performance cathode materials for sodium-ion batteries.
Introduction
In recent years, the demand for lithium-ion batteries (LIBs) has increased sharply due to the rapid development of large-scale energy storage and electric vehicles (Wu et al., 2016; Li et al., 2020; Nie et al., 2020a; Shen et al., 2020; Sui et al., 2020a). However, the available lithium resources in the Earth's crust are very limited. By contrast, the reserves of sodium are much more abundant than lithium (Sui et al., 2020b; Zhou et al., 2020). Therefore, the sodium-ion battery is a promising alternative to LIBs, and has attracted extensive attention in recent years (Liu et al., 2021). Nevertheless, the operating voltage and energy density of sodium-ion batteries (SIBs) are generally lower than those of LIBs, as the standard electrode potential of Na/Na+ (−2.71 V) is higher than that of Li/Li+ (−3.04 V) (Zhu et al., 2013; Wu et al., 2019; Nie et al., 2020b). Obviously, it is very important to develop new cathode materials with high voltages to improve the energy density of SIBs (Wu et al., 2018a; Zheng et al., 2018).
The development of fluorophosphate cathode materials with high working potential and high theoretical capacity has becoming a research hotspot, and various fluorophosphate materials have been reported, including Na2MPO4F (M = Fe, Mn, Co and Ni) (Barpanda et al., 2018; Li et al., 2018; Wu et al., 2018b), NaVPO4F, and Na3V2(PO4)2F3 (Cai et al., 2018; Subramanyan et al., 2021). Among these fluorophosphate materials, Na2MnPO4F is attracting significant attention due to its optimal theoretical capacity and suitable working potential. Firstly, the theoretical specific capacity of Na2MnPO4F is as high as 250 mAh g−1 when the two sodium-ions are completely removed (~125 mAh g−1 for one sodium-ion) (Wu et al., 2018b; Sharma et al., 2020). Secondly, based on the strong induction effect and strong negative charge of F−, Na2MnPO4F owns high working potential (3.66 and 4.67 V vs. Na/Na+) and excellent thermal stability (Wu et al., 2018b), and its working potential is within the stable window of electrolytes. In addition, manganese is low cost and abundant in the Earth. These advantages mean Na2MnPO4F has a broad application potential. Although Na2MnPO4F shows such great potential advantages, there are some problems that impede the application of Na2MnPO4F. Na2MnPO4F is a broadband insulator, and the low electronic conductivity seriously affects its electrochemical performance. For example, the Na2MnPO4F sample synthesized by solid state method (Ellis et al., 2010) shows almost no electrochemical activity. At present, there are few reports on the modification of Na2MnPO4F cathode materials. Most recently, we have prepared a series of solid solution materials of Na2Mn1−xFexPO4F/C (0 < x < 1) by introducing Fe element and coating with carbon to improve the electrochemical properties of Na2MnPO4F (Tang et al., 2020). Our results show that the introduction of Fe and carbon coating can significantly improve the electrochemical activity of Na2MnPO4F. In a series of the Na2Mn1−xFexPO4F/C samples, Na2Fe0.6Mn0.4PO4F/C shows the best electrochemical performance. However, the above report did not investigate the effect of carbon sources on the structure and properties of the material, and did not optimize the type of carbon source. Thus, this paper aims to optimize the carbon source to increase the porosity of Na2Fe0.6Mn0.4PO4F/C, optimize the carbon coating effect to inhibit the primary particle overgrowth, and thus build a good conductive network to further improve the electrochemical performance of Na2Fe0.6Mn0.4PO4F.
Experimental
Chemicals
Sodium carbonate (Na2CO3, 99.8%) was purchased from Aladdin. Iron citrate (FeC6H5O7, A.R.), manganese acetate tetrahydrate (Mn(CH3COO)2·4H2O, A.R.), ammonium dihydrogen phosphate (NH4H2PO4, A.R.), sodium fluoride (NaF, 99%), oxalate acid (H2C2O4, 99.8%), ascorbic acid (C6H8O6, 99.8%), citric acid (C6H8O7, 99.5%), and glucose (C6H12O6, 99.5%) were purchased from Sinopharm Chemical Reagent Co., Ltd. All chemicals were used directly without further purification.
Materials Synthesis
The Na2Fe0.6Mn0.4PO4F/C composite materials were synthesized by a simple spray-drying method. Firstly, 3.18g Na2CO3, 3.45 g NH4H2PO4, 1.26 g NaF, 4.41 g C6H5O7Fe·H2O, 2.23 g Mn(CH3COO)2·4H2O, and carbon source (1.98 g oxalate acid, 3.87 g ascorbic acid, 4.72 g citric acid, or 4.35 g glucose) were dissolved in 200 ml deionized water to form a mixed solution. Considering the burning loss, the theoretical mass of carbon sources was calculated as 10 wt% of the product (Na2Fe0.6Mn0.4PO4F/C). Secondly, the above solution was dried by a spray drier (Shanghai Attainpak DC1500) to prepare the spherical precursor powders. The spraying speed is 250 ml h−1, and the inlet and outlet air temperatures are 200° and 80°C, respectively. Finally, the precursor powders were heated at 300°C for 3 h and then calcined at 625°C for 6 h in an argon atmosphere to obtain the final Na2Fe0.6Mn0.4PO4F/C products.
Characterization
The crystalline structure of Na2Fe0.6Mn0.4PO4F/C was studied by X-ray diffraction (XRD, Rigaku Ultima IV). The morphology of samples was observed by scanning electron microscope (SEM, Hitachi SU5000). Carbon content of Na2Fe0.6Mn0.4PO4F/C was measured with a carbon sulfur analyzer (HCS-140).
Battery Fabrication and Electrochemical Tests
Firstly, Na2Fe0.6Mn0.4PO4F/C powders and acetylene black and poly (vinylidene fluoride) in the weight ratio of 8:1:1 were blended in N-methyl pyrrolidinone to form a homogeneous slurry, then the slurry was spread uniformly on an aluminum foil and dried at 120°C for 12 h in vacuum. The dried foil was pressed into discs (Φ = 12 mm) to act as the cathode plate. An Na-foil (Φ = 14 mm) and glass fiber film disc (Φ = 16 mm) were used as the anode and separator, respectively. A solution of 1 M NaClO4 dissolved in 95% PC and 5% FEC was used as the electrolyte. The cells were tested with LAND battery test system at room temperature between the voltage range of 1.5–4.5 V (vs. Na/Na+). The electrochemical impedance spectroscopy (EIS, amplitude: 5 mV; frequency: 0.01–100 kHz) was measured by a CHI660D electrochemical workstation.
Results and Discussion
The XRD patterns of Na2Fe0.6Mn0.4PO4F/C synthesized with different carbon sources are shown in Figure 1. As shown, all diffraction peaks of the samples can be fully indexed to typical Na2Fe0.6Mn0.4PO4F material (PDF#87-0467) with space group P21/n. The Na2Fe0.6Mn0.4PO4F/C samples synthesized with ascorbic acid, citric acid, and glucose were all pure and no impurities were detected. However, for the Na2Fe0.6Mn0.4PO4F/C sample prepared with oxalic acid, the diffraction peaks of Na3PO4 (PDF#71-1918) can be observed, indicating that impurities are produced in the preparation of the Na2Fe0.6Mn0.4PO4F/C sample. There are no obvious diffraction peaks related to C that can be observed in the XRD patterns, mainly because the carbon in Na2Fe0.6Mn0.4PO4F/C is amorphous. The carbon contents of Na2Fe0.6Mn0.4PO4F/C samples prepared with oxalic acid, ascorbic acid, citric acid, and glucose are determined by carbon sulfur analyzing instrument, and the results are 7.1, 7.4, 7.9, and 8.1 wt%, respectively. It can also be seen that the synthesized Na2Fe0.6Mn0.4PO4F/C samples with different carbon sources show different peak intensities and widths: the sample prepared with glucose exhibits the highest peak intensity, citric acid takes the second place, and the sample prepared with oxalic acid presents the lowest peak intensity. Normally, high peak intensity and narrow half-peak width indicate large particle sizes of the sample, which will result in unfavorable diffusion and transmission of sodium ions in electrochemical reactions. Interestingly, the order of the reduction ability of the carbon sources in this work is glucose < citric acid < ascorbic acid < oxalic acid, and the difference of reduction ability of four different carbon sources might cause a discrepancy of peak intensity and width. In other words, the relatively high reduction ability of oxalic acid made it difficult to prepare pure Na2Fe0.6Mn0.4PO4F/C material.
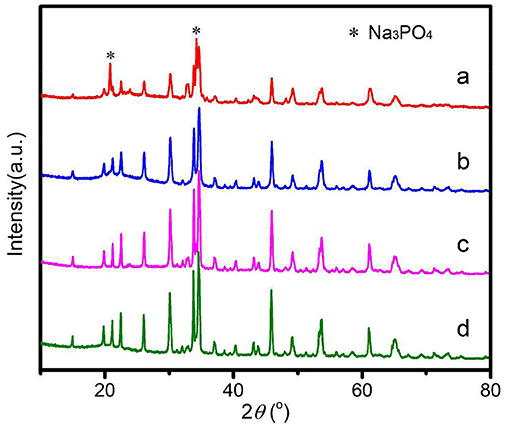
Figure 1. XRD patterns of Na2Fe0.6Mn0.4PO4F/C prepared with various carbon sources. (a) Oxalic acid; (b) Ascorbic acid; (c) Citric acid; (d) Glucose.
SEM was employed to analyze the structure and morphology of synthesized Na2Fe0.6Mn0.4PO4F/C samples with different carbon sources. It is obvious that carbon sources play a key role in structural construction of Na2Fe0.6Mn0.4PO4F/C material. As shown in Figure 2A, the synthesized Na2Fe0.6Mn0.4PO4F/C material with oxalic acid exhibits an irregular porous agglomerates morphology with an unclear crystal border, and no spherical structure was observed. It is noted that the formation of the porous structure might be relevant to the decomposition of oxalic acid. The Na2Fe0.6Mn0.4PO4F/C material prepared with ascorbic acid, as shown in Figure 2B, displays a hollow spherical shape with a compact surface, and the size distribution is in the range of 1–2 μm. For the Na2Fe0.6Mn0.4PO4F/C material prepared with citric acid (Figure 2C), the particles fail to keep the uniform hollow spherical shape, which gradually turned into irregular spheres/agglomerates. Using glucose as a carbon source, the synthesized Na2Fe0.6Mn0.4PO4F/C material (Figure 2D) exhibits a damaged spherical shape with irregular particles, and the average size of the damaged spheres is about 3–5 μm. Interestingly, the particle size is decreased with the increase of reduction ability of carbon sources. This phenomenon may provide guidance for the synthesis and optimization of Na2Fe1−xMnxPO4F series materials.
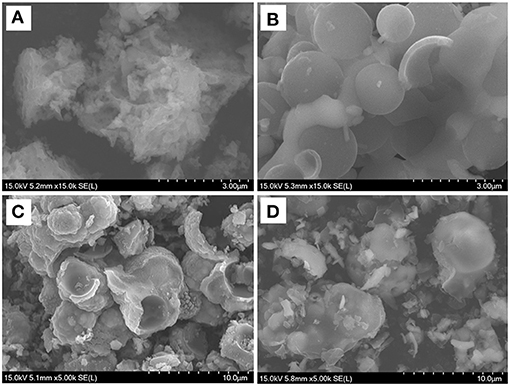
Figure 2. SEM images of Na2Fe0.6Mn0.4PO4F/C prepared with various carbon sources. (A) Oxalic acid; (B) ascorbic acid; (C) citric acid; (D) glucose.
The rate performance of Na2Fe0.6Mn0.4PO4F/C materials synthesized with different carbon sources is shown in Figure 3. As shown in Figure 3A, the Na2Fe0.6Mn0.4PO4F/C material prepared with oxalic acid delivers a specific capacity of 86.9 mAh g−1 at 0.05C, and the corresponding coulombic efficiency is 92.3%. The discharge capacities can achieve 65.7, 47.5, 29.8, and 16.8 mAh g−1 at current densities of 0.1, 0.2, 0.5, and 1C, respectively (Figure 3A). For the Na2Fe0.6Mn0.4PO4F/C material prepared with ascorbic acid, the discharge capacities rise to 95.1, 83.5, 72.7, 60.0, and 48.1 mAh g−1 at current densities of 0.05, 0.1, 0.2, 0.5, and 1C, respectively (Figure 3B). However, the rate performance of Na2Fe0.6Mn0.4PO4F/C material prepared with citric acid and glucose is unsatisfactory (e.g., the discharge capacities are only 30 and 24.0 mAh g−1 at 1C, Figures 3C,D). Obviously, the discharge capacities and rate performance of Na2Fe0.6Mn0.4PO4F/C materials are closely related to the type of carbon sources, and the material prepared with ascorbic acid shows improved rate performance compared with the materials prepared with the other three carbon sources.
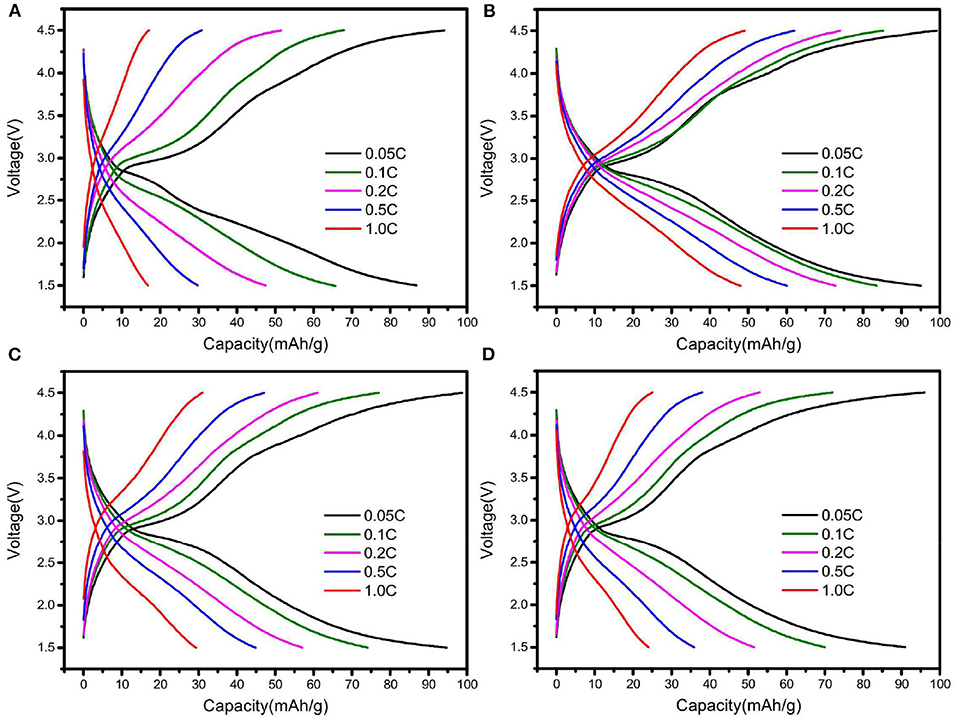
Figure 3. Charge and discharge curves of Na2Fe0.6Mn0.4PO4F/C samples prepared with various carbon sources: (A) oxalic acid; (B) ascorbic acid; (C) citric acid; (D) glucose.
Figures 4A–D shows the rate-cycling performance of Na2Fe0.6Mn0.4PO4F/C synthesized with different carbon sources. All Na2Fe0.6Mn0.4PO4F/C samples exhibit stable cycle performance at high current densities. Normally, a low current density corresponds to deep charge/discharge behavior, which may cause volume expansion and the irreversible structural change of cathode material. Conversely, a high current density corresponds to shallow charge/discharge behavior, and the structural change of the cathode material is unapparent. The discharge capacity of Na2Fe0.6Mn0.4PO4F/C sample prepared with ascorbic acid is as high as 95 mAh g−1 after the test, reverting to 0.05C after cycling at various current rates, while the values are only 84, 93, and 88 mAh g−1 for the materials prepared with oxalic acid, citric acid, and glucose. The long cycle performance of Na2Fe0.6Mn0.4PO4F/C samples synthesized with different carbon sources are shown in Figure 4E. The material prepared with ascorbic acid endures 91.7% of its initial capacity after 100 cycles at 0.5C, while only 44.6%, 88.6%, and 78.3% of the initial capacities are retained for the materials synthesized with oxalic acid, citric acid, and glucose. The result further demonstrates that the Na2Fe0.6Mn0.4PO4F/C material prepared with ascorbic acid exhibits outstanding electrochemical performance.
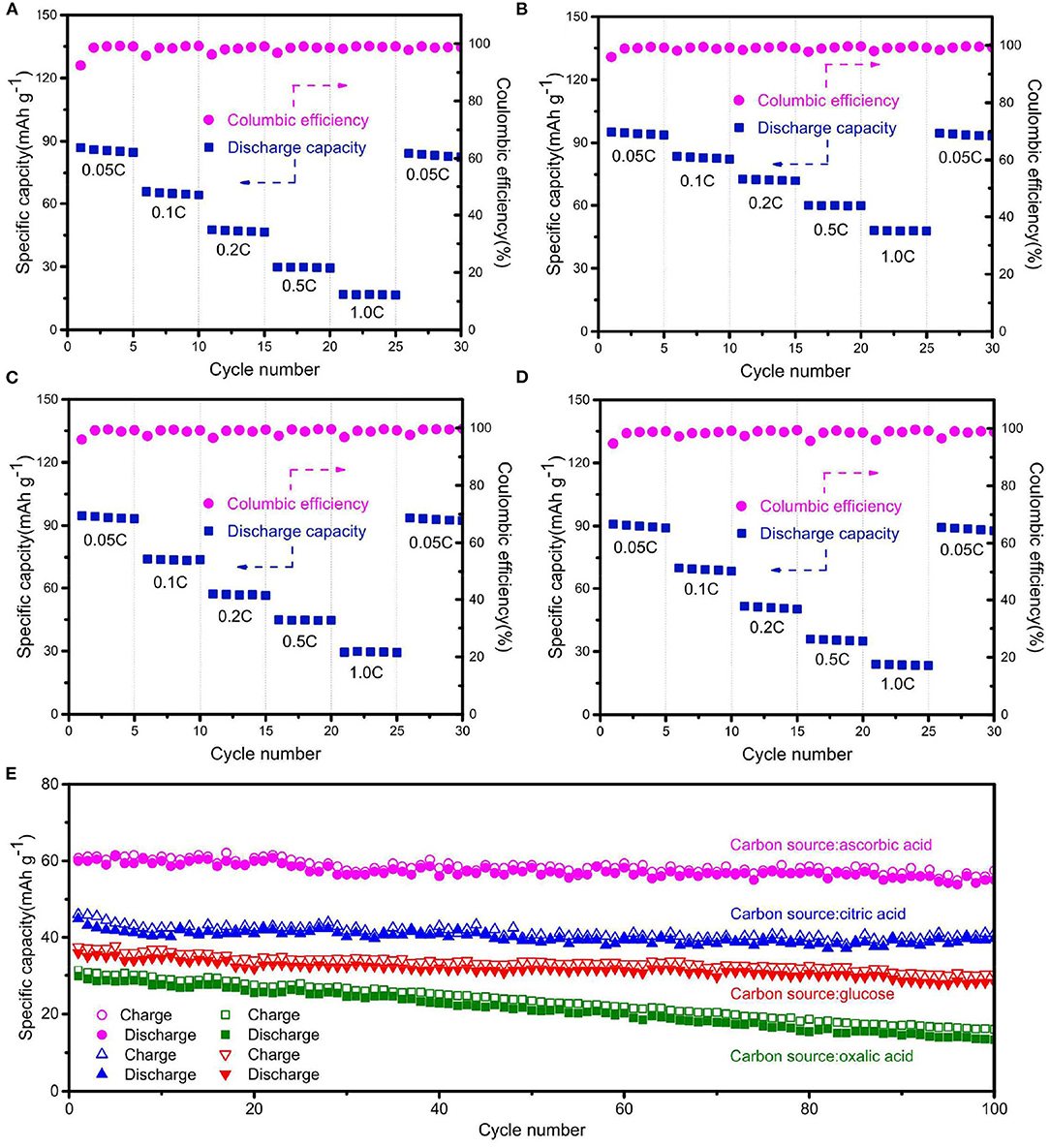
Figure 4. Rate-cycling curves of the Na2Fe0.6Mn0.4PO4F/C samples prepared with (A) oxalic acid, (B) ascorbic acid, (C) citric acid, and (D) glucose. (E) Long-term cycling performance of Na2Fe0.6Mn0.4PO4F/C samples at 0.5C rate.
In order to further reveal the effect of various carbon sources on the electrochemical properties of Na2Fe0.6Mn0.4PO4F/C samples, electrode reaction kinetics were investigated by EIS. Figure 5A shows Nyquist plots of four synthesized Na2Fe0.6Mn0.4PO4F/C by using an equivalent circuit, and all curves consist of a diagonal line and a semicircle. In the equivalent circuit, Rs and Rct represent the equivalent ohmic resistance of the cell and charge transfer, respectively. Considering the surface of the working electrode is not absolutely smooth, CPE is used to describe the double layer capacitance, and W refers to Warburg impedance (Cui et al., 2016). As can be seen from Figure 5B, the equivalent ohmic resistance (Rs) of these four Na2Fe0.6Mn0.4PO4F/C materials is similar, while the material prepared with ascorbic acid exhibits much lower charge-transfer resistance (Rct) than the other three synthesized materials, which indicates that the material prepared with ascorbic acid presents the lowest electrochemical polarization.
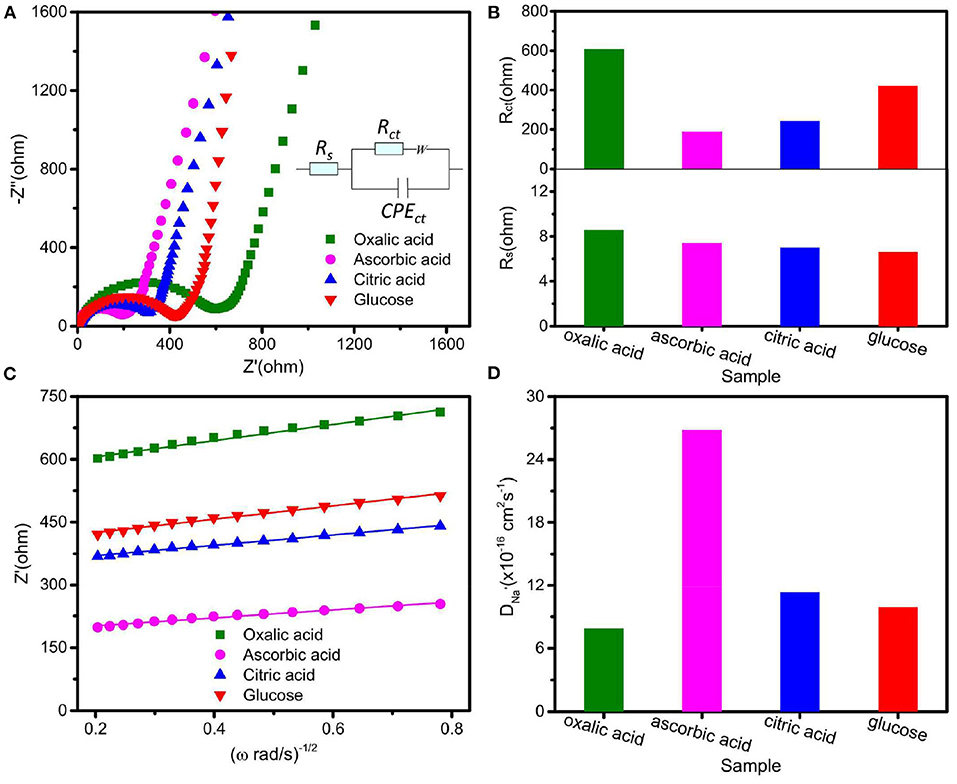
Figure 5. (A) Nyquist plots of the prepared electrodes at first full-discharge state with equivalent circuit inset; (B) fitting parameters of Nyquist plots; (C) relationship between Z′ and ω−1/2; (D) Na+ diffusion coefficients of the prepared electrodes.
The sodium ion diffusion coefficient (D) was also calculated by the following formula.
Where R, T, A, n, F, C, σw, and ω represent the gas constant, the absolute temperature, the electrode surface area, the charge transfer number of the electrochemical reaction, Faraday's constant, concentration of Na+ ions, Warburg impedance coefficient, and angular frequency, respectively. By calculation (as shown in Figures 5C,D), it is found that the material prepared with ascorbic acid presents the highest sodium ion diffusion coefficient, 2.68 × 10−15 cm2 s−1, which might be due to its high contact area between the electrode material and electrolyte as well as its low crystallite size. The impurities are unfavorable for the sodium ion diffusion; that is why the Na2Fe0.6Mn0.4PO4F/C sample prepared with oxalic acid shows the lowest sodium ion diffusion coefficient. These results indicate that the Na2Fe0.6Mn0.4PO4F/C prepared with ascorbic acid exhibits high specific capacity, improved rate performance, and excellent cycle stability, and it is expected to be a promising cathode material with high energy density and power density.
Conclusions
In summary, the effect of various carbon sources on the structure and electrochemical properties of synthesized Na2Fe0.6Mn0.4PO4F/C cathode materials is investigated in this study. The results show that the reduction ability of carbon sources plays a key role in the morphology of Na2Fe0.6Mn0.4PO4F/C materials, and the material prepared with ascorbic acid presents the most perfect hollow spherical shape. The electrochemical tests demonstrate that the Na2Fe0.6Mn0.4PO4F/C material prepared with ascorbic acid exhibits outstanding electrochemical performances. Its high discharge capacities (95.1 mAh g−1 at 0.05C), improved rate property (48.1 mAh g−1 at 1C), and long cycle stability (endures 91.7% of its initial capacity after 100 cycles at 0.5C) specifically are superior to Na2Fe0.6Mn0.4PO4F/C materials prepared with other carbon sources. Electrode reaction kinetics show that the material prepared with ascorbic acid presents lower electrochemical polarization than other materials, which is in accordance with the results of electrochemical tests. This study points out the relationship between the reduction ability of carbon sources and electrochemical properties of Na2Fe0.6Mn0.4PO4F/C materials, and it also provides a promising strategy to achieve cathode materials with high energy density and power density.
Data Availability Statement
The original contributions presented in the study are included in the article/supplementary material, further inquiries can be directed to the corresponding author/s.
Author Contributions
ST, YS, and LW did the main experiment and wrote the manuscript. YS and LW were involved in the discussion of the experiment and revised the manuscript and also provided the financial support. XZ and BW assisted with the material synthesis and properties analysis. All authors contributed to the article and approved the submitted version.
Funding
This study was supported by the National Natural Science Foundation of China (51774210, 51974190, and 51904194), Natural Science Research of Jiangsu Higher Education Institutions of China (19KJB450001), and the Prospective Applied Research from the Technological Innovation Project of Key Industry of Suzhou (SYG201931).
Conflict of Interest
The authors declare that the research was conducted in the absence of any commercial or financial relationships that could be construed as a potential conflict of interest.
References
Barpanda, P., Lander, L., Nishimura, S. I., and Yamada, A. (2018). Polyanionic insertion materials for sodium-ion batteries. Adv. Energy Mater. 8:1703055. doi: 10.1002/aenm.201703055
Cai, Y., Cao, X., Luo, Z., Fang, G., Liu, F., Zhou, J., et al. (2018). Caging Na3V2(PO4)2F3 microcubes in cross-linked graphene enabling ultrafast sodium storage and long-term cycling. Adv. Sci. 5:1800680. doi: 10.1002/advs.201800680
Cui, D., Chen, S., Han, C., Ai, C., and Yuan, L. (2016). Carbothermal reduction synthesis of carbon coated Na2FePO4F for lithium ion batteries. J. Power Sources 301, 87–92. doi: 10.1016/j.jpowsour.2015.09.123
Ellis, B.L., Michael Makahnouk, W. R., Rowan-Weetaluktuk, W. N., Ryan, D. H., and Nazar, L. F. (2010). Crystal sturcture and electrochemical properties of A2MPO4F fluorophoshpates (A=Na, Li; M=Fe, Mn, Co, Ni). Chem. Mater. 22, 1059–1070. doi: 10.1021/cm902023h
Li, L., Xia, L., Yang, H., Zhan, X., Chen, J., Chen, Z., et al. (2020). Solid-state synthesis of lanthanum-based oxides Co-coated LiNi0.5Co0.2Mn0.3O2 for advanced lithium ion batteries. J. Alloys Compounds 832:154959. doi: 10.1016/j.jallcom.2020.154959
Li, Q., Liu, Z., Zheng, F., Liu, R., Lee, J., Xu, G. L., et al. (2018). Identifying the structural evolution of the sodium ion battery Na2FePO4F cathode. Angew. Chem. Int. Edition 57, 11918–11923. doi: 10.1002/anie.201805555
Liu, Z., Li, L., Chen, J., Yang, H., Xia, L., Chen, J., et al. (2021). Effects of chelating agents on electrochemical properties of Na0.9Ni0.45Mn0.55O2 cathode materials. J. Alloys Compounds 855:157485. doi: 10.1016/j.jallcom.2020.157485
Nie, Y., Xiao, W., Miao, C., Fang, R., Kou, Z., Wang, D., et al. (2020a). Boosting the electrochemical performance of LiNi0.8Co0.15Al0.05O2 cathode materials in-situ modified with Li1.3Al0.3Ti1.7(PO4)3 fast ion conductor for lithium-ion batteries. Electrochim. Acta 353:136477. doi: 10.1016/j.electacta.2020.136477
Nie, Y., Xiao, W., Miao, C., Xu, M., and Wang, C. (2020b). Effect of calcining oxygen pressure gradient on properties of LiNi0.8Co0.15Al0.05O2 cathode materials for lithium ion batteries. Electrochim. Acta 334:135654. doi: 10.1016/j.electacta.2020.135654
Sharma, L., Adiga, S. P., Alshareef, H. N., and Barpanda, P. (2020). Fluorophosphates: next generation cathode materials for rechargeable batteries. Adv. Energy Mater. 10:2001449. doi: 10.1002/aenm.202001449
Shen, C., Zhang, K., You, Y., Wang, H., Ning, R., Qi, Y., et al. (2020). Inducing rapid polysulfide transformation through enhanced interfacial electronic interaction for lithium-sulfur batteries. Nanoscale 12, 13980–13986. doi: 10.1039/D0NR02429E
Subramanyan, K., Lee, Y. S., and Aravindan, V. (2021). Impact of carbonate-based electrolytes on the electrochemical activity of carbon-coated Na3V2(PO4)2F3 cathode in full-cell assembly with hard carbon anode. J. Colloid and Interface Sci. 582, 51–59. doi: 10.1016/j.jcis.2020.08.043
Sui, Y., Hao, Y., Zhang, X., Zhong, S., Chen, J., Li, J., et al. (2020b). Spray-drying synthesis of P2-Na2/3Fe1/2Mn1/2O2 with improved electrochemical properties. Adv. Powder Techn. 31, 190–197. doi: 10.1016/j.apt.2019.10.010
Sui, Y., Zhou, J., Wang, X., Wu, L., Zhong, S., and Li, Y. (2020a). Recent advances in black phosphorus based materials for electrochemical energy storage. Mater. Today. doi: 10.1016/j.mattod.2020.09.005. [Epub ahead of print].
Tang, S., Wu, L., Sui, Y., and Zhong, S. (2020). Spray-drying synthesis of Na2Fe1−xMnxPO4F/C cathodes: a facile synergetic strategy harvesting superior sodium storage. Ad. Powder Tech. 31, 1564–1573. doi: 10.1016/j.apt.2020.01.034
Wu, L., Hu, Y., Zhang, X., Liu, J., Zhu, X., and Zhong, S. (2018b). Synthesis of carbon-coated Na2MnPO4F hollow spheres as a potential cathode material for Na-ion batteries. J. Power Sources 374, 40–47. doi: 10.1016/j.jpowsour.2017.11.029
Wu, L., Shi, S., Zhang, X., Yang, Y., Liu, J., Tang, S., et al. (2018a). Room-temperature pre-reduction of spinning solution for the synthesis of Na3V2(PO4)3/C nanofibers as high-performance cathode materials for Na-ion batteries. Electrochim. Acta 274, 233–241. doi: 10.1016/j.electacta.2018.04.122
Wu, L., Zheng, J., Wang, L., Xiong, X., Shao, Y., Wang, G., et al. (2019). PPy-encapsulated SnS2 nanosheets stabilized by defects on TiO2 support as durable anode material for lithium ion battery. Angew. Chem. Int. Edn. 58, 811–815. doi: 10.1002/anie.201811784
Wu, X., Li, Y., Xiang, Y., Liu, Z., He, Z., Wu, X., et al. (2016). The electrochemical performance of aqueous rechargeable battery of Zn/Na0.44MnO2 based on hybrid electrolyte. J. Power Sources 336, 35–39. doi: 10.1016/j.jpowsour.2016.10.053
Zheng, J. C., Yang, B. Y., Wang, X. W., Zhang, B., Tong, H., Yu, W. J., et al. (2018). Comparative investigation of Na2FeP2O7 sodium insertion material synthesized by using different sodium sources. ACS Sustain. Chem. Eng. 6, 4966–4972. doi: 10.1021/acssuschemeng.7b04516
Zhou, Z., Luo, Z., He, Z., Zheng, J., and Li, Y. (2020). A novel hollow porous structure designed for Na0.44Mn2/3Co1/6Ni1/6O2 cathode material of sodium-ion batteries. J. Power Sources 479:228788. doi: 10.1016/j.jpowsour.2020.228788
Keywords: sodium-ion batteries, cathode materials, Na2Fe0.6Mn0.4PO4F, spray drying, electrochemical performance
Citation: Tang S, Zhang X, Sui Y, Wang B, Li J and Wu L (2021) A Comparative Study on Na2Fe0.6Mn0.4PO4F/C Cathode Materials Synthesized With Various Carbon Sources for Na-ion Batteries. Front. Chem. 8:633949. doi: 10.3389/fchem.2020.633949
Received: 26 November 2020; Accepted: 11 December 2020;
Published: 13 January 2021.
Edited by:
Wei Xiao, Yangtze University, ChinaReviewed by:
Chao Shen, Northwestern Polytechnical University, ChinaXianwen Wu, Jishou University, China
Copyright © 2021 Tang, Zhang, Sui, Wang, Li and Wu. This is an open-access article distributed under the terms of the Creative Commons Attribution License (CC BY). The use, distribution or reproduction in other forums is permitted, provided the original author(s) and the copyright owner(s) are credited and that the original publication in this journal is cited, in accordance with accepted academic practice. No use, distribution or reproduction is permitted which does not comply with these terms.
*Correspondence: Yulei Sui, c3VpeXVsZWlAc3VkYS5lZHUuY24=; Ling Wu, bHd1QHN1ZGEuZWR1LmNu