- 1Department of Pharmacy, School of Medicine, Zhejiang University City College, Hangzhou, China
- 2Department of Pharmaceutics, College of Pharmaceutical Sciences, Zhejiang University, Hangzhou, China
- 3College of Chemical and Biological Engineering, Zhejiang University, Hangzhou, China
- 4Department of Cardiology, The First Affiliated Hospital, Zhejiang University School of Medicine, Hangzhou, China
Chemotherapy is an important anti-tumor treatment in clinic to date, however, the effectiveness of traditional chemotherapy is limited by its poor selectivity, high systemic toxicity, and multidrug resistance. In recent years, mesoporous silica nanoparticles (MSNs) have become exciting drug delivery systems (DDS) due to their unique advantages, such as easy large-scale production, adjustable uniform pore size, large surface area and pore volumes. While mesoporous silica-based DDS can improve chemotherapy to a certain extent, when used in combination with other cancer therapies MSN based chemotherapy exhibits a synergistic effect, greatly improving therapeutic outcomes. In this review, we discuss the applications of MSN DDS for a diverse range of chemotherapeutic combination anti-tumor therapies, including phototherapy, gene therapy, immunotherapy and other less common modalities. Furthermore, we focus on the characteristics of each nanomaterial and the synergistic advantages of the combination therapies. Lastly, we examine the challenges and future prospects of MSN based chemotherapeutic combination therapies.
Introduction
Despite the rapid development of medicine, the incidence and mortality of cancers are consistently rising and cancer remains one of the most terrible threats to human lives (Siegel et al., 2020). Traditional chemotherapy is one of the most common cancer treatments and is the most effective systemic treatment, playing an irreplaceable role in current treatment modality (Dai et al., 2016). However, the clinical application of chemotherapy is limited by several deficiencies: First, most chemotherapy drugs have poor aqueous solubility or short half-life in vivo, leading to low drug utilization. Second, chemotherapeutic drugs show poor tumor selectivity (Akhtar et al., 2014), resulting in the undifferentiated killing of both tumor and healthy cells. This non-targeted lethality not only reduces the therapeutic effect against carcinomas but also causes severe side effects. Last, is the multidrug resistance (MDR) induced by chemotherapy. MDR refers to the resistance of cancer cells to a variety of drugs which are structurally and functionally unassociated (Kong et al., 2017). This phenomenon is one of the primary causes of chemotherapy failure, leading to the recurrence of tumors, patient relapse, or even death (Wang J. et al., 2017).
Recent years have witnessed many efforts to overcome the shortcomings of conventional chemotherapy. One of the most promising is the development of nano drug delivery systems (nano-DDS), which can increase the solubility and bioavailability of drugs, prolong the circulation time of drugs, increase the accumulation of drugs in tumor tissues, and improve the pharmacokinetic behavior in vivo, improving the curative effect of therapies while reducing the side effects (Mu et al., 2020). Common nanocarriers include polymers (Alsehli, 2020), liposomes (Allen and Cullis, 2013), dendrimers (Dias et al., 2020), inorganic nanoparticles like gold nanoparticles (GNPs) (Ajnai et al., 2014), and carbon nanomaterials (Chen D. et al., 2015). Among these nano-DDS, mesoporous silica nanoparticles (MSNs) are a class of materials that have garnered particular focused by many researchers, due to their facile large-scale production, adjustable uniform pore size (Bouchoucha et al., 2016), and large surface area and pore volume (Farjadian et al., 2019). These properties endow MSNs with good drug encapsulation efficiency and delivery, with uncomplicated preparation like the sol–gel “chimiedouce” methods in aqueous solutions (Croissant et al., 2018). Since silica-based materials have been considered safe by Food and Drug Administration, dedicated efforts have been made to utilize MSNs to construct nanoplatforms for drug delivery and cancer chemotherapy (Li T. et al., 2019). MSN DDS design has been extremely versatile. Some researchers have used active targeting groups to improve MSNs tumor targeting and improve chemotherapy selectivity (Cheng et al., 2015; Chen L. et al., 2016; Murugan et al., 2017), while others rely on the characteristics of the tumor microenvironment, such as lower pH and higher glutathione (GSH) content than normal cells. Many pH and/or redox responsive MSNs to release chemotherapeutic drugs have been designed (Cheng et al., 2017; Murugan et al., 2017; Cheng Y.-J. et al., 2019). Enzyme, thermal, and ultrasound responsive MSN DDS have also been studied (Chang et al., 2013; Li X. et al., 2018; Zhu et al., 2019). More recently, dual therapeutic agents co-delivered by MSNs to exert synergistic action and improve the effect of chemotherapy have been investigated (Zhang Y. et al., 2015; Murugan et al., 2016; Wang L. et al., 2018; Li X. et al., 2019; Xing et al., 2020) (Figure 1).
The mechanisms of cancer occurrence involves multiple pathways (Xu et al., 2015; Qiu L. et al., 2018), therefore it is unlikely that a single therapeutic mechanism will be sufficient to completely eradicate cancer. In support of this supposition, every therapy mode including chemotherapy, has demonstrated drawbacks (Fan et al., 2017). Combining chemotherapy with other treatment modalities is a good strategy to combat these shortcomings and augment therapeutic efficacy. Furthermore, chemotherapeutic combination therapies can reduce drug dosage to patients, lightening side effects while enhancing efficacy (Yu et al., 2018; Shrestha et al., 2019; Zhang et al., 2019). Combination chemotherapy possesses great potential for cancer treatment (Goldin, 1980).
In this review, we summarize the progress made on MSN based chemo-combination therapies according to the different combination treatment modalities (Figure 2). We focus on the synergistic therapeutic effects achieved by these combined systems, emphasizing the advantages of combination therapy over monotherapy and highlighting how a successful combination compensates for the shortcomings of chemotherapy. Then, we conclude with the challenges faced by MSN based combination chemotherapy systems and what improvements are needed for these treatment systems to become mainstays in cancer therapy.
Chemotherapy and Phototherapy
Phototherapy, including photodynamic therapy (PDT) and photothermal therapy (PTT), is a non-invasive therapeutic strategy commonly used as a supplement to chemotherapy in order to overcome the deficits of the monotherapy (Qin et al., 2018; Cheng et al., 2020). In the presence of light, photoactive therapeutics (photosensitizers) of PDT or PTT are excited to produce reactive oxygen species (ROS) or hyperthermia to kill cancer cells (Robertson et al., 2009; Chen J. et al., 2019). The high surface area, large pore size and pore volume of MSNs make them ideal candidates for multi-drug loading and therefore, a combined platform for photo-chemotherapy. In the following section we summarize photoactive mesoporous silica-based chemotherapeutic nanoplatforms according to the modality of phototherapy used in the combination (Table 1).
Photodynamic-Chemotherapy
PDT is an emerging therapeutic procedure in cancer treatment that has attracted significant attention due to its high selectivity, non-invasive nature and minimal side effects, when compared to conventional therapy (Liao et al., 2016). Photosensitizer (PS) selection, tissue oxygen levels, and light wavelength are all key factors of PDT. Briefly summarized, under a specific wavelength of light, the PS transfers absorbed energy to oxygen, inducing a transformation from its triplet ground state to its singlet excited state and instigating cytotoxic effects (Leonidova et al., 2014). Due to the limited tissue penetration depths of most wavelengths used to activate PSs, PDT is non-viable for deep-seated tumors or metastasis. However, since the ROS generation from PDT has been reported to promote anti-cancer drug release (Chen Y.-W. et al., 2016; Cheng K. et al., 2019; Wong et al., 2020), a combination of PDT and chemotherapy could to enhance the therapeutic outcomes of both treatments.
MSNs have attracted substantial attention as a potential PDT partner in recent years, due to their structural merits. Many PSs aggregate easily (reducing their efficacy) and have a poor intracellular uptake, limiting their applicability in solid tumors (Ding et al., 2018; Oh et al., 2019; Fu et al., 2020). Integration with MSNs can prevent the aggregation of PSs as well as improve the targeting ability and biocompatibility of PSs, leading to reduced side-effects and stronger anticancer efficacy (Zhao et al., 2010; Yao et al., 2015; Yang G. et al., 2016). Several MSN vehicles have been reported to be able to co-deliver anti carcinogens and PSs into cancer cells (Zhang et al., 2016; Kankala et al., 2017; Chen et al., 2020; Fu et al., 2020; Wang H. et al., 2020), and these studies showed several advantages to these combination systems such as enhanced biocompatibility, improved cellular uptake of the payloads, and enhanced therapeutic efficiency (Yan et al., 2018). In one such example, Fang et al. (2019) designed a hollow MSN nanoparticles (HMSNs) based nanoplatform into which the chemotherapeutic agent DOX and photosensitizer chlorine e6 (Ce6) were co-loaded at 9.56 and 16.68% (w/w), respectively. The HMSNs-DOX-Ce6 were further modified with bovine serum albumin integrated manganese dioxide nanoparticles (BSA-MnO2) to construct a multifunctional therapeutic nanoplatform the authors named BMHDC. In which, BSA is intended to improve biocompatibility and tumor accumulation while MnO2 serves to elevate the oxygen content within the hypoxic tumors. Combination index (CI) analysis indicated a great synergy between PDT and chemotherapy in BMHDC (CI = 0.21). In another study, Guo et al. (2020) decorated MSNs with Au nanoparticles as PSs and mPEG-SH as a GSH-triggered gatekeeper to create a reduction-responsive MSN-Au-PEG nanoplatform. The spherical structure of MSN-Au-PEG was maintained, with a particle size of ~155 nm. The pore diameter of MSN-Au decreased from 3.37 to 2.67 nm after coating with mPEG-SH. The particles achieved a drug loading content (DLC) and drug loading efficiency (DLE) for DOX of 12.3 and 43.25%, respectively. Cytotoxicity assays in Hela cells demonstrated that MSN-Au-PEG@DOX with laser irradiation exhibited the lowest cell viability (30%) compared with the non-illuminated group (40%) or the free DOX group (35%). The above results indicated that the nanoplatform displayed a significant enhancement to carcinoma inhibition due to the synergistic effect of PDT-chemotherapy.
Photothermal-Chemotherapy
PTT employs a photothermal agent (PA) to convert light energy into heat, as opposed to ROS in PDT, and induce the thermal ablation of cancer cells (Zhi et al., 2020). Similarly to PDT, PTT is a non-invasive therapeutic modality with advantages of simplicity, minimal side effects, and remote activation. However, as with PDT limited light penetration and inevitable light scattering make PTT alone insufficient to completely eliminate tumors (Li Z. et al., 2018). Since the hyperthermia produced by PTT can enhance cellular metabolism and cell membrane permeability, the concept behind combination photothermal-chemotherapy is to not only improve the uptake of chemotherapy drugs but also prevent tumor recurrence (Zheng et al., 2013; Wang X. et al., 2017). As tumors have a higher sensitivity to many chemotherapeutics at elevated temperatures (Hauck et al., 2008), the cytotoxicity of chemotherapy can be increased, thereby the dosage of anticancer drugs can be reduced and systemic side-effects minimized (Yang Y. et al., 2017).
In recent years, MSNs have emerged as powerful candidates for DDSs and they have been widely used for the codelivery of PA and chemotherapy drugs as a combination therapy (Shu et al., 2018; Tian et al., 2018). Copper sulfide nanoparticles (CuS NPs) (Chen F. et al., 2015; Zhang et al., 2015a,b; Zhang Y. et al., 2015; Peng et al., 2017; Wang F. et al., 2018; Li et al., 2020), polydopamine (PDA) (Zhang et al., 2018; Chen C. et al., 2019), gold nanorods (AuNR) (Zhang et al., 2012; Liu et al., 2015; Huang et al., 2017; Ramasamy et al., 2018; Sun X. et al., 2018; Wang Y. et al., 2019), gold shells (Rahman et al., 2017), reduced graphene oxide (rGO) (Liu et al., 2019), GO (Tang et al., 2015; Tran et al., 2018) and carbon dots (CDs) (Singh et al., 2016; Zhang et al., 2020b) are common PAs introduced in the PTT-chemotherapy systems. Wang and coworkers (Wang et al., 2015) developed a DOX-loaded amino-modified MSNs (DOX@MSN-NH2) with the DOX loading content of 20.9 wt% and modified with reduced graphene oxide (rGO) as a heating gatekeeper coat to achieve a multifunctional DDS. rGO possess a strong NIR absorption at 980 nm (a wavelength with good tissue penetration) and acts as the PA in this work, converting NIR light energy intothermal energy to kill cancer cells. This nanocomposite was able to kill 68% of HEp-2 cells in synergistic therapy, compared with 54% in PTT and 33% in chemotherapy alone. This in vitro result illustrates that the combination of PTT and chemotherapy enables a better therapeutic outcome than the monotherapies. Another rGO and MSNs based nanoplatform (162 nm) loaded with (S)-(+)-camptothecin (CPT) for PTT-chemotherapy was also reported to have a great synergistic effect. While DOX killed 33.4% of cells, and PTT killed 52% of cells, their combination was able to kill 68% of cells (Chen et al., 2014).
Black phosphorus (BP) is a new PTT agent featuring low cytotoxicity, good biocompatibility, and efficient photothermal performance (Qiu M. et al., 2018). In one study utilizing this new PA, Ren et al. (2020) constructed a MSNs based platform (150 nm) loaded with DOX and black phosphorus quantum dots (BPQDs) together. The in vitro results showed that the multimodal therapy of PTT and chemotherapy could induce a higher cell death rate (73.5%) in tumors compared to chemotherapy alone (64.78%).
Photodynamic-Photothermal-Chemotherapy
Since both PDT and PTT are triggered by light irradiation, integrating both methods with chemotherapy into a trimodal nanosystem seems a viable approach. Indeed, this combination has already proven to have superior therapeutic efficacy than any mono or dual therapy (Yang D. et al., 2016) and there have been numerous attempts to integrate both of the phototherapeutics and chemotherapeutics into MSN-based single formulation (Luo et al., 2016; Sun Q. et al., 2018; Yan et al., 2020). Fang et al. (2017) synthesized mesoporous silica-coated gold nanorods (100 nm) loaded with 5-fluorouracil (5-FU) and conjugated to indocyanine green (ICG). With 5-FU, ICG, and the gold nanorods (GNR) responsible for the chemotherapy, the PDT and the PTT, respectively. The addition on an MSN coating was able to improve both the photostability and the loading capacity of the GNR. The as-synthesized GNR@SiO2-5-FU-ICG realized a trimodal synergistic therapy of PDT, PTT and chemotherapy under multimodal imaging guidance. Quantitative tumor growth inhibition ratio in nude mice treated by GNR@SiO2-5-FU-ICG under laser irradiation was 100%, while those treated with GNR@SiO2-5-FU under laser and GNR@SiO2-NH2 were 88.27 and 69.43%, respectively (saline groups were regarded as 0%) (Figure 3). The nanoplatform was able to completely eradicate tumor without recurrence, demonstrating the superiority of the combination therapy. Wen and coworkers (Xiao et al., 2019) also designed a trimodal nanoplatform (250 nm) by introducing tellurium nanodots (Te NDs) into MSNs through in situ formation and then loading the system with paclitaxel (PTX). Here, the Te NDs work as both PS and PA concurrently, producing ROS and heat under NIR irradiation. When the concentration of PTX was 80 μM, MTT assay showed that HepG2 cells treated with MT@L-PTX@FA under irradiation had the lowest cell viability (~25%), significantly out performing MT@L with irradiation (~45%), MT@L-PTX@FA without irradiation (~40%), and free PTX (~45%). The results prove that this synergistic approach was able to enhance therapeutic outcomes.
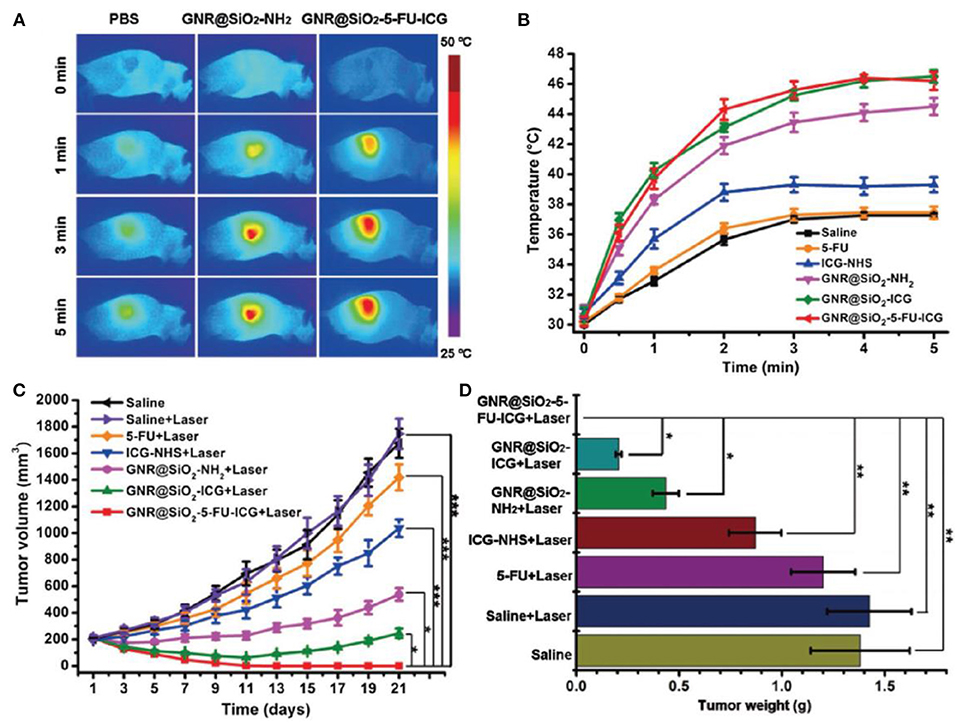
Figure 3. (A) Infrared thermal images of A375 tumor-bearing mice with different treatments at different time points upon 808 nm laser irradiation 24 h post-injection. (B) Temperature changes in the tumor region of the A375 tumor-bearing mice treated with saline, 5-FU, free ICG-NHS, GNR@SiO2-NH2, GNR@SiO2-ICG, and GNR@SiO2-5-FU-ICG, which irradiated at 24 h post-injection (808 nm, 1.0 W cm−2, 5 min). (C) Tumor growth of mice received different treatments. (D) Tumor weights of nude mice on day 21 after different treatments (Fang et al., 2017). Copyright 2017, Wiley. *p < 0.05; **p < 0.01; ***p < 0.001.
Chemotherapy and Gene Therapy
Traditional cancer therapies focus on killing cancer cells directly, which can achieve short-term effects but has little effect on drug resistance or metastasis and so does little to prevent tumor relapse. The occurrence of cancer is closely related to gene structure and function changes, which provides us with another strategy, gene therapy. With the sequencing of human genome, gene therapy has made noteworthy progress in the past few decades. In particular, the combination of gene therapy and chemotherapy has been widely studied and has been proven to enhance therapeutic efficiency and reduce side effects, achieving a synergistic effect in cancer treatment (Shen et al., 2014). While promising, a crucial step for this combination therapy is the development of suitable carriers for the precise delivery and controlled release of gene therapy agents such as plasmids, DNA, small interfering RNA (siRNA), micro RNA (miRNA), and short-hairpin RNA (shRNA). MSNs are easily functionalized with positively charged polymers to enable electrostatic interactions with nucleic acid and their cavities are able to load chemotherapeutic drugs effectively. As such, they are an extremely promising carrier for gene/drug codelivery (Table 2).
p53 is a tumor suppressor gene, the disfunction of which has been found to have the highest correlation with human tumors, making it an ideal target for combination chemo-gene therapy. Lin et al. (2017) conjugated chitosan with poly (amidoamine) (PAMAM), which can absorb the p53 plasmid and then modified the chitosan derivatives onto the surface of MSNs to be the gatekeeper of DOX loaded into the pores (average diameter 2.3 nm) by a redox-responsive disulfide bond. The size of the nanosystem was about 100 nm, which is suitable for cell uptake. The nanocarrier proved to have excellent DOX/p53 codelivery ability and showed a satisfactory transfection efficiency (27.6%), very close to PEI −25 k (29.8%) in vitro. Importantly, the drug/gene dual delivery nanosystem showed a better inhibition for Hela cell (36% cell viability) than the drug (51%) or gene (75%) used alone, exhibiting the synergistic effect of chemotherapy and gene therapy. Zhang et al. (2017) also constructed a redox-responsive silica-based nanosystem which enable codelivery of DOX and p53. The primary difference being that the disulfide bond was directly inserted into the silica backbone and covalently linked to DOX by the one pot method, allowing the nanosystem to achieve redox-responsiveness, controlled release, and self-degradation. Recently, a smart drug/gene nanocarrier was developed by Zhou et al. (2020), which utilized UV crosslinked/pH de-crosslinked coumarin as the gatekeeper of MSNs loaded with the chemotherapy drug 5-FU and p53 carried by cationic poly(glycidylmethacrylate)-b-poly(2-(dimethylamino)ethylmethacrylate) (PGMA-b-PDMAEMA). In addition to achieving a synergistic effect of chemotherapy and gene therapy (21.33% apoptosis rate of cancer cells, compared to 14.32% for 5-Fu and 9.41% for p53 monotherapies), coumarins can also emit blue fluorescence, enabling the nanocarrier to function as a fluorescent probe to detect trace drugs concentrations.
In addition to p53 which is associated with most cancers, there are also genes associated with specific cancers. Hepatocyte nuclear factor 4α (HNF4α) is an important transcription protein that regulates the differentiation of hepatocytes and maintains the biological function of hepatocytes. Based on this, Tsai et al. (2019) investigated an approach to deliver the gene encoding HNF4α and the chemotherapeutic drug cisplatin to hepatocellular carcinoma (HCC), via polyethyleneimine-modified MSNs (PMSNs). After treatment with PMSN/HNF4α plasmid DNA/cisplatin, HNF4α in Huh7 cells was over expressed and resulted in the proportion of CD133 enriched cells decreasing significantly.
Since Fire et al. (1998) first proposed RNA interference (RNAi) technology its application in cancer therapy has grown rapidly. RNAi molecules include siRNA, shRNA and miRNA, of which siRNA have been studied most. siRNA is a type of chemically synthesized double-stranded RNA. It is transported into cells and then incorporated into the RNA-induced silencing complex (RISC), a protein-RNA complex which separates the strands of the RNA and discards the sense strand. The anti-sense strand then guides RISC to cut the target messenger RNA (mRNA), resulting in hindrance of the production of its encoded protein (Deng et al., 2014). Researchers have used mesoporous silica-based multifunctional carriers to deliver DOX and Bcl-2 siRNA to treat a variety of cancers (Zhou et al., 2016; Zhao et al., 2017; Lee et al., 2018; Pan et al., 2018). For example, Pan et al. (2018) developed a smart nanoplatform based on DOX loaded mesoporous silica as core and Bcl-2 siRNA loaded zeolitic imidazole framework-8 (ZIF-8) as its shell, in which ZIF-8 acted as the gatekeeper of DOX with its pH sensitivity controlling the release of DOX and siRNA. Flow cytometry analysis demonstrated that the apoptosis rate of MDR cells reached 88.2% after incubation with Dox-MSN-COOH@ZIF-8/Bcl-2 siRNA but was only 36.3% without Bcl-2 siRNA.
Survivin, which is highly expressed in many types of human tumors, is a member of the inhibitor of apoptosis protein (IAP) family. Dilnawaz and Sahoo (2018) demonstrated that the combination of the chemotherapeutic drug (etoposide or docetaxel) or the proteasome inhibitor carfilzomib with survivin siRNA could induce a 12.4 or 14.6% increase in apoptosis, respectively, in A549 cells. Considering that the overexpression of multidrug resistance protein 1 (MRP1) is significantly related to the clinical drug resistance of many kinds of tumors, Song et al. (2020) loaded MRP-1 siRNA and myricetin into MSNs and modified the nanoparticles with folic acid to target lung cancer cells. In vitro experiments showed that Myr-MRP-1/MSN-FA can significantly inhibit the proliferation of cancer cells and in vivo experiments further verified this therapeutic effect, in which the tumor volume of mice treated with Myr-MRP-1/MSN-FA decreased the most.
As precursors of siRNA, shRNA are often co-transported by MSNs-based carriers along with chemotherapeutic drugs to enhance their therapeutic effects against cancer (Li et al., 2016). Most notably, this approach is taken when reversing MDR (Chen Z. et al., 2016; Wu et al., 2018). An interesting nanovehicle was developed by Wu et al. (2018), in which they loaded the DOX prodrug with nitrobenzyl into the pores of MSNs, then covalently linked MSNs to cationic poly[2-(N,N-dimethylaminoethyl)-methacrylate] (PDMAEMA) modified by light sensitive coumarin. P-gp shRNA was then electrostatically adsorbed unto the particle surface and could be released upon activation by 405 nm light. After which, the release of DOX could be triggered by exposure to 365 nm light. In this study, the sequential release of gene agent and drug can be activated in a controllable manner via external illumination and this sequential release greatly increased the accumulation of drugs in the tumor sites, reversed MDR and improved overall therapeutic effect.
miRNA is a type of endogenous short RNA molecule, which can be used to regulate the cleavage of target mRNA post-transcriptionally or inhibit its translation (Bartel, 2004). miRNA approaches and anti-miRNA approaches have been applied in cancer therapies, the effectiveness of these methods mostly associated with the efficacy of gene vectors. Liu et al. (2018) developed a smart silica-based nanosystem with high efficiency loading, stimulation responsivity, active targeting, and biocompatibility. Their MSNs system (Figure 4) was composed of PEI covalently linked inside the silica cavity via disulfide bond, then electrostatically bound to miRNA-145. Meanwhile acting as the gatekeeper of DOX a WL8-PEG shell is coated on the outside of the MSNs, improving the stability and targeting to SW480 cells of the combination therapy. The nanosystem showed a remarkable antitumor effect both in in vivo and in vitro experiments, with an especially excellent antimetastatic effect in an orthotopic colorectal tumor model. The expression of miR211 is upregulated in many kinds of tumors, especially in gliomas; conversely, the downregulation of miR211 can make glioma cells sensitive to temozolomide (TMZ). Working off this, Bertucci et al. (2015) used MSNs incorporated with Cy5 to transport TMZ and anti-miR221/polyarginine-peptide nucleic acid (R8-PNA221) complex to drug-resistant glioma cells. In accelerated survival experiment, MSNs with TMZ and anti-miR211 synergistically decreased the C6 glioma cells survival rate more than the sum of the MSNs-TMZ and MSNs-PNA221. The same trend was observed in their apoptosis experiment. Several studies have indicated that anti-miRNA therapy combined with chemotherapy is a potential strategy for reversing MDR. In order to improve the sensitivity of glioma cells to TMZ, Nie et al. (2020) used 93.5 ± 6.7 nm Mn-doped MSNs to deliver TMZ and10–23 DNAzyme. In acidic and reductive environments the Mn-MSNs decompose, enabling Mn2+ to assist 10–23 DNAzyme in silencing the O6-methylguanine-DNA methyltransferase (MGMT) gene. Western blot experiments demonstrated the gene silencing effect of Mn-MSNs/TMZ/10-23 DNAzyme and a significant decrease in IC50 (>3.8-fold) validated that the MDR T98G cells became more sensitive to TMZ after chemo-gene combination therapy.
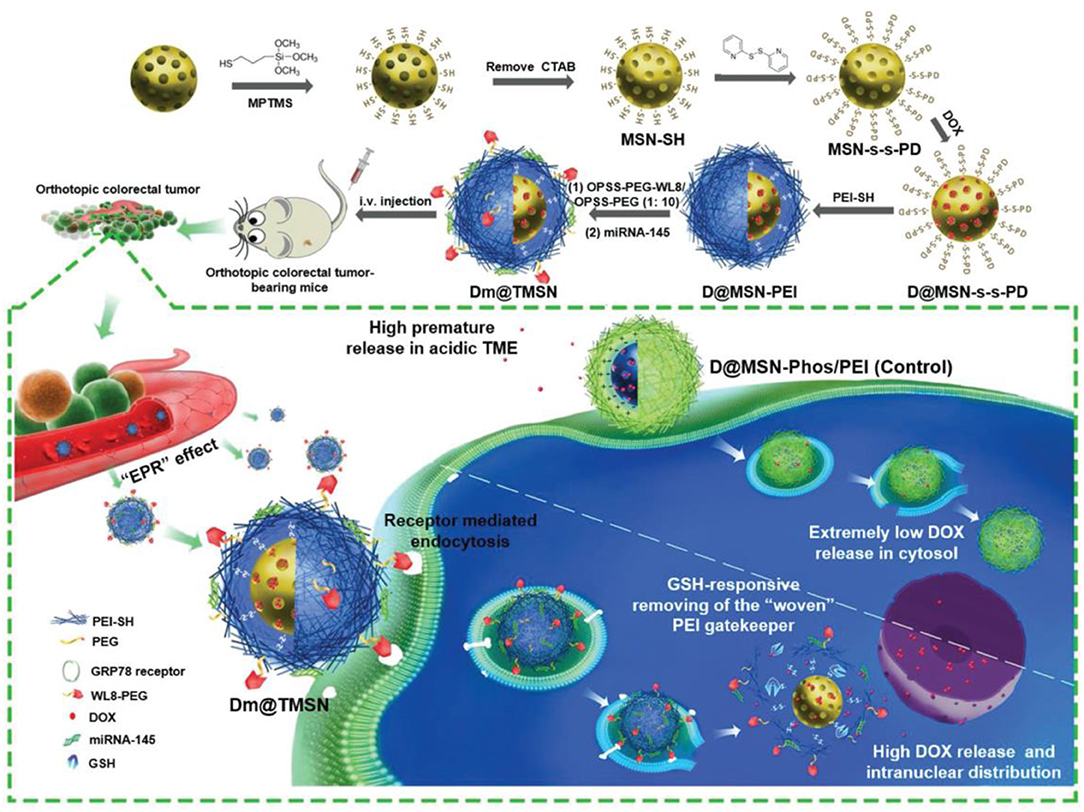
Figure 4. Schematic illustration of the preparation (top) and proposed mechanism (bottom) of the MSN-based DOX and miRNA-145 smart delivery system (Liu et al., 2018). Copyright 2018, Wiley.
Chemotherapy and Immunotherapy
Immunotherapy, utilizing the body's natural immune system to inhibit tumor, is also a potential treatment option for combination with chemotherapy. The immune system has the dual role of inhibiting and promoting tumor growth (Schreiber et al., 2011) and immune checkpoint therapy has become a research hotspot of cancer therapy in recent days (Dyck and Mills, 2017). Compared to other treatment modes, immunotherapy can more specifically target the primary tumor and secondary tumor metastasis, it can also prolong anti-tumor response through immune memory cells to inhibit tumor recurrence (Luo et al., 2017). However, immunotherapy has a low response rate, making it ineffective for some patients (Zheng et al., 2020). Considering the limitations of chemotherapy alone as well as immunotherapy alone mentioned above, the idea of combining chemotherapy and immunotherapy came into being naturally. Though chemotherapeutic agents can induce immunogenic cells death (ICD) (Kroemer et al., 2013), most chemotherapies would induce lymphopaenia, which hampers the anticancer immune response (ACIR) (Lake and Robinson, 2005), making the combination of chemotherapy and immunotherapy difficult to realize. Zheng's group has broken the barrier between the two therapies by designing DOX@HIMSNs, a DOX-loaded and MSN-based nanoplatform (Zheng et al., 2016). The tumor volume in 4T1 tumor bearing Balb/c mice of DOX@HIMSN group was five times smaller than that of DOX group. And the fluorescent overlap between granzyme-B and caspase-3 of DOX@HIMSN group had a Mander overlap coefficient of 0.95, which was higher than the DOX group (0.88), indicating the enhanced immunological cells killing ability of DOX@HIMSN. These results showed that the highly integrated MSNs can increase tumor cell cytotoxicity as well as stimulate ACIR, indicating the potential of MSN-based nanosystems in immunotherapy combined chemotherapy. There is increasing efforts to combine the two modalities together into an MSN-based nanoplatform to achieve an enhanced therapeutic effect (Choi et al., 2019). Kong et al. (2017) developed a HMSN-mediated nanosystem called A/D/I-dHMLB to co-delivery DOX, all-trans retinoic acid (ATRA) and interleukin-2 (IL-2) for chemo-immunotherapy. A/D/I-dHMLB had a higher tumor inhibitory rate of 84.8 ± 13.0% compared to DOX group of 17.1 ± 12.4%. After the treatment of A/D/I-dHMLB, the number of myeloid-derived suppressor cells (MDSC), which impede ICD, showed a 2.7-fold decrease, while the number of mature DC and activated CD8+ T cell increased 14.3-fold and 3.93-fold, respectively. Other cytokines like IL-12p70 and TNF-α also increased while inhibitory cytokines like IL-10 and TGF-β decreased. All the results indicated that the design of A/D/I-dHMLB can effectively kill cancer cells and reach an enhanced antitumor immunity. Dong et al. (2017) developed a pathogen-mimicking nanocomplex (MSN-SP-LPS) with a mean hydrodynamic diameter of 167.1 ± 3.9 nm by conjugating the sodium phthalate salt of the parent LPS to MSN. The amount of TNF-α detected in RAW 264.7 cells treated with MSN-SP-LPS (~5.5 × 103 pg/mL) or SP-LPS (~5.6 × 103 pg/mL) was higher than that released by MSN (~0.3 × 103 pg/mL) due to the presence of LPS, indicating a stronger activation of macrophages. In addition, the high amount of INF-γ secretion (900 pg/ml) provided the evidence of T cell activation, showing a strong inflammation response by MSN-SP-LPS. When treated with 1.25 μg SP-LPS/mL and 0.5 μg DOX/mL, the cell viability of splenocytes treated with MSN-DOX-SP-LPS combination was the lowest (~72%), compared to ~74% for MSN-DOX and ~95% for SP-LPS, indicating the superiority of the synergistic effect of immuno-chemotherapy.
Chemotherapy and Sonodynamic Therapy
Sonodynamic therapy (SDT), another non-invasive therapeutic modality, has shown specific advantages in cancer therapy when compared to its counterparts like PDT or PTT, since SDT can reach deeper tumor sites due to the high tissue penetrating nature of ultrasound (US) waves (Qian et al., 2016). SDT can kill cancer cells by producing cytotoxic ROS through the combination of US with a sonosensitizer, while minimizing damage to the surrounding normal cells (Chen Y.-W. et al., 2016). Sonosensitizers include organic materials such as porphyrins (Hachimine et al., 2007; Yumita et al., 2010), erythrosine B (EB) (Yumita et al., 2002) and Rose Bengal (RB) (Sugita et al., 2015), as well as inorganic materials like titanium dioxide (TiO2) (Harada et al., 2011) and silicon nanoparticles (Osminkina et al., 2015). Interesting, MSNs have been shown to have impressive SDT activity due to their high porosities, which allow the free diffusion of molecules to generate ROS.
Since MSNs can simultaneously play the role of sonosensitizer and drug carrier, there is exciting potential for a synergistic nanoplatform integrating SDT and chemotherapy. While promising, SDT monotherapy still has some limitations such as lacking tumor-targeting ability, hypersensitivity to light (Lafond et al., 2019), tumor hypoxia (Zhao et al., 2020) and insufficient lethality to kill all cancer cells. The US used in SDT promotes drug release in chemotherapy (Ding et al., 2017), while the chemotherapy could compensate for the weaknesses of sonodynamic monotherapy, creating the ideal synergistic environment. Ding et al. (2017) reported a 50 nm core-shell MSN-based nanocomplex with DOX loading and targeting group methacrylated hyaluronic acid (m-HA) gel functionalization to realize a synergistic therapy combining chemotherapy and SDT. The surviving percent of cells treated by DOX@MSN-HA under US was only about 5% compared to that of DOX@MSN-HA (35%) and MSN under US (70%), highlighting the synergistic potential of SDT and chemotherapy.
Chemotherapy and Magnetic Hyperthermia Therapy
Recently, magnetic hyperthermia therapy has been proven to be effective tool in the struggle against cancer. Magnetic hyperthermia utilizes the heat from the energy dissipation of magnetic particles to cause the irreversible necrosis of cancer cells, while leaving normal tissues undamaged (Kobayashi, 2011; Brollo et al., 2016). Additionally, magnetic hyperthermia has been shown to accelerate the release of the anticancer drug DOX from nanoplatforms, making it a potential partner to improve the efficacy of chemotherapy (Tian et al., 2018). In return, chemotherapy as a whole-body treatment can make up the limitation of magnetic hyperthermia therapy as a treatment only for local oncology. Therefore, combining chemotherapy with magnetocaloric therapy is a promising method to inhibit tumor growth and many MSN-based systems have been reported to make this a reality.
Tian et al. (2018) developed poly(N-isopropylacrylamide-co-methacrylic acid) [P(NIPAM-co-MAA)] coated magnetic mesoporous silica nanoparticles (MMSNs) with particle size 255 ± 28 nm and pore size 2.6 nm to achieve a combination chemo-magnetic therapy. Under exposure to an alternating magnetic field (AMF) at a frequency of 409 kHz and magnetic field strength of 180 Gauss, the MMSNs generated enough heat to raise the cell temperature to 64.2°C within 15 min, inducing both hyperthermia and the controlled release of loaded DOX. A CCK-8 assay showed that the cell viability of Hela cells after treatment with the synthesized DOX-MMSN@P (NIPAM-co-MAA) nanoparticles decreased to only 23%, which was significantly lower than that of cells after treatment with DOX (76%) or AMF (42%) alone. This shows the strong synergistic therapeutic effect of chemo-magnetic hyperthermia therapy and provides a promising platform for combined chemotherapy.
Iron nanomaterials are used as magnetic therapeutics in many MSN-based synergistic systems due to their strong response to AMFs (Zhu and Tao, 2015; Guisasola et al., 2018). Cai et al. (2019) successfully synthesized CSiFePNs (220 nm) by loading superparamagnetic ferroferric oxide and paclitaxel (PTX) into MSNs coated with MDA-MB-231 cell membranes. The combination system showed the highest anticancer ability (IC50 value of 0.8 μgL−1) compared to CSiFeNs with AMF (IC50 value of 3.6 μgL−1) or CSiFePNs without AMF (>0.8 μgL−1), further demonstrating that the combination of magnetotherapy and chemotherapy possesses great potential for the treatment of carcinomas.
Chemotherapy, Chemodynamic Therapy, and Starvation Therapy
Chemodynamic therapy (CDT) is a novel modality to treat tumors by using transition metals to convert local hydrogen peroxide (H2O2) into highly toxic hydroxyl radicals (•OH) to kill cancer cells (Huo et al., 2017). Because CDT responds to the acidic and hydrogen peroxide rich microenvironment of tumors, it is highly selective. However, there are still challenges to face in CDT such as insufficient intratumor H2O2, inadequate H+, as well as the unsatisfactory catalytic capacity of chemodynamic agents (Cheng K. et al., 2020; Wang W. et al., 2020). As a local treatment modal, CDT could be a supplement to chemotherapy to enhance overall therapeutic efficacy. Combinations of chemotherapy and CDT with MSNs as the DDS have reported strong potential in anticancer treatment (Kankala et al., 2017; Zhang et al., 2020a).
Another oncotherapy strategy, starvation therapy, also responds to the tumor microenvironment, is a superb strategy to treat cancer, and may address the shortcomings of CDT (Hao et al., 2019). In contrast to normal cells, the glycolysis of cancer cells is upregulated even in an oxygen-sufficient situation (Warburg et al., 1927). Starvation therapy attempts to exploit this by utilizing glucose oxidase (GOx) to cut off nutrients to cancer cells, starving them to death. Several synergistic MSN-based nanoplatforms integrating chemo and starvation therapy have been reported (Cheng K. et al., 2019; Zhang et al., 2020b). As discussed above, CDT is limited by the concentrations of H2O2 and H+. Starvation therapy produces excess H2O2 and causes a decrease in pH, making it a perfect pair for CDT. Furthermore, starvation therapy itself is not enough for completely eliminate cancer cells, necessitating combination with additional treatment modalities to achieve the desired therapeutic effect (Fu et al., 2018). As both CDT and starvation therapy have been successfully integrated with chemotherapy and GOx-triggered starvation therapy can induce sequential CDT and chemotherapy, it is possible to construct an all-in-one system featuring tri-modal therapy.
In one such example, starvation therapy and chemodynamic therapy were reported to combine with chemotherapy in a single nanosystem designed by Cheng K. et al. (2020). Hypoxic prodrug tirapazamine (TPZ) and high efficiency catalyst Fe3O4 were loaded into MSNs and the MSNs surface functionalized with GOx. The drug loading rate of TPZ and GOx achieved by the authors were 14.9 and 5.8%, respectively and the hydrodynamic diameter size of Fe3O4@MSN was 88 nm. When GOx consumes the oxygen and glucose in the tumor microenvironment, it causes increased endogenous H2O2, decreased acidity, and more extreme hypoxia. Then, through the iron ion-mediated Fenton reaction (Lafond et al., 2019) using the Fe3O4 catalyst, H2O2 is transformed into cytotoxic •OH and induces chemodynamic therapy. The hypoxia then activates the hypoxia-responsive TPZ, to kill cancer cells while avoiding healthy cells (Figure 5). Under hypoxic conditions, MTT assays showed that the cell viability of MCF-7 cells after treatment with TPZ/Fe3O4@MSN-GOX was 8.6%, while that of the TPZ/Fe3O4@MSN group was 29.9% and the TPZ group was 33.3%, indicating the as-synthesized TPZ/Fe3O4@MSN-GOX displayed excellent tumor inhibition, with the GOx-induced starvation therapy triggering synergistic enhancements with chemodynamic and chemotherapy.
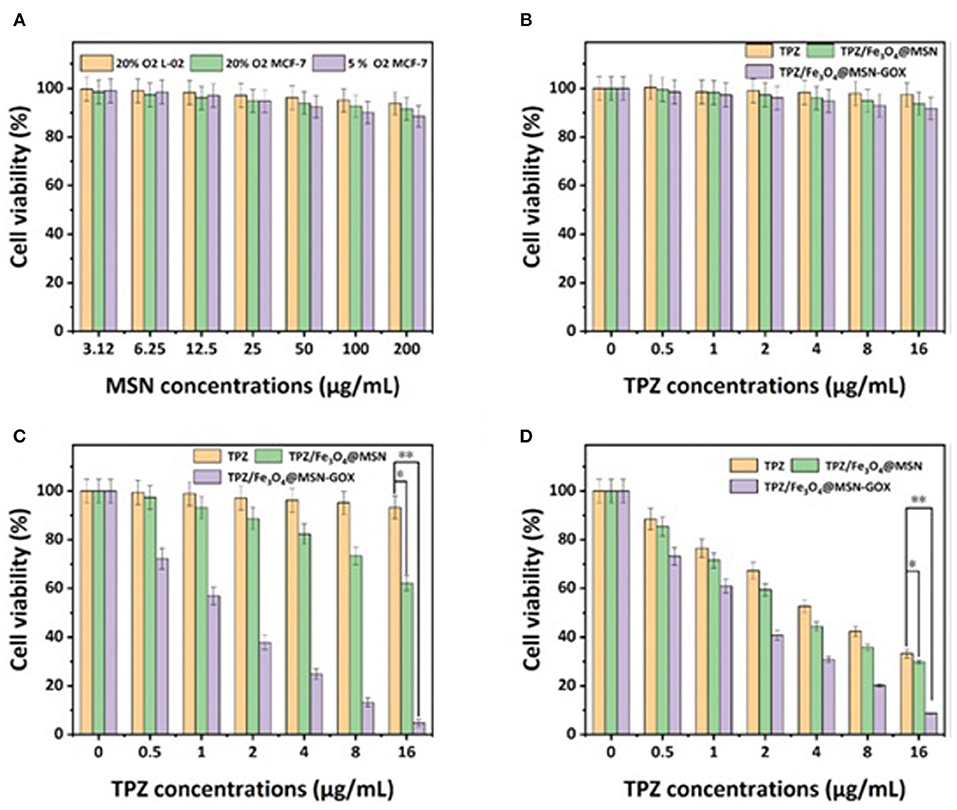
Figure 5. (A) Cell viability rates of different MSN concentrations at different oxygen concentrations. (B) L-02 cell viability rates of different TPZ dose-dependent concentrations of different nanoparticles in 20% O2 concentration with glucose (1 mg mL−1). (C) MCF-7 cell viability rates of different TPZ dose-dependent concentrations of different nanoparticles in 5% O2 concentration. (D) MCF-7 cell viability rates of different TPZ dose-dependent concentrations of different nanoparticles in 5% O2 concentration, which was treated by different nanoparticles calculated by the MTT assay (Cheng K. et al., 2020). Copyright 2020, RSC.
Cross-Modal Chemo-Combination Therapies
The development of multifunctional nanocarriers has enabled the development of chemotherapeutic combination therapies that include more than one other synergistic modality. In order to reverse multidrug resistance, Yang H. et al. (2017) assembled sodium alginate/chitosan polyelectrolyte multilayers onto Fe3O4/Au/MSNs loaded with DOX and photosensitizer Ce6 to adsorb P-gp shRNA. After incubation with the nanoparticles and laser irradiation, the survival rate of drug-resistant cells MCF-7/ADR was inhibited by >60% compared with any monotherapy. Demonstrating that chemo-gene-photodynamic therapy had a synergistic anti-tumor effect and the ability to reverse MDR. Additionally, Fe3O4/Au endowed the nanoparticles with dual imaging modes of magnetic resonance and CT imaging, enabling real-time guided therapy.
Gold nanotriangles are excellent radiation/PTT therapeutic agents but possess high toxicity and poor drug loading capacity (Bhattaraia et al., 2017). To overcome these limitations, a kind of “Hedgehog like” Janus gold triangle-MSNs were developed by Wang and co-works to deliver the hypoxia-activated prodrug TPZ, with surface functionalized FA-PEG to improve targeting and biocompatibility (Wang Z. et al., 2019). In vivo and in vitro experimentation revealed that FA-GT-MSNs@TPZ nanoplatforms showed superior anti-tumor effects to monotherapies alone, demonstrating that hypoxia-activated radio-chemo-photothermal therapy is a very promising strategy for cancer treatment.
Lu et al. (2020) prepared mesoporous silica nanorods of a specific width (100 nm) and precisely controlled aspect ratio (AR: length/width). They loaded DOX and GOx with AR6 into MSNs, then coated the nanoparticles with an a polydopamine (PDA) layer to absorb Siramesine, a drug that can damage lysosomes and induce apoptosis. The multifunctional nanoplatform integrated chemotherapy, PTT, CDT and ST, and targeted cancer cells with FA, exhibit in a much higher lethality to cancer cells than any single therapy. These studies highlight the potential for MSN chemotherapy combinations to go beyond a two pronged assault on cancer and incorporate a whole host of therapeutic modalities.
Conclusions and Outlook
As a standard therapy modality for cancer treatment, chemotherapy urgently needs a more targeted drug accumulation in tumor sites and strategies to overcome MDR in order to improve its practical application in the clinic. Recently, chemotherapy-based combination therapies have become an irresistible trend due to the superiority in therapeutic efficacy compared to monotherapies. MSNs with large pore sizes, diverse functionality, ease of modification and good biocompatibility are ideal materials to realize such synergistic nanoplatforms, since they can not only serve as drug carriers but also function as therapeutic agents in therapies complementary to chemotherapy. In this review, we have discussed many MSN-based nanosystems featuring the integration of chemotherapy with other therapy modals namely immunotherapy, gene therapy, phototherapy, magnetic hyperthermia therapy and sonodynamic therapy and emphasized the effects of dual- or multi-modal therapy. As expected, most of the reported cases demonstrate that MSN mediated combination therapy achieved at least 1 + 1 > 1 effect in cellular or animal level, providing experimental evidence for further promising applications of these MSN-based delivery systems. Naturally, there is no objectively best combination, as each combination has its advantages and disadvantages. However, the chemo-immuno combination therapy may have the most promising future for further clinical translation considering that immunotherapy using PD-1/PD-L1 antibodies, CTAL-4 antibodies and CAR-T treatment has been recently revealed as a powerful clinical strategy for treating cancer.
Though MSN-based combination chemotherapies have shown preliminary success in in vitro and in vivo testing, several challenges remain before these nanoparticles can put into clinical use. As highlighted in this review, nanocomposites serve as the multimodal therapy platforms, necessitating long-term biosafety tests as well as more detailed pharmacokinetic/pharmacodynamic analyses for each participating component in the complexes. Additionally, the optimal dosing ratio between chemotherapeutics and other therapeutic agents must be investigated further. Furthermore, the integration of combination therapies should be strategic, that is, they should achieve interlocking effects and smart drug delivery and release systems to maximize the synergistic benefits. Lastly, developing simpler syntheses of MSN-based nanocomplexes is a high priority, as is improving their cancer targeting capabilities.
The existing researches about MSN-based chemotherapy combination therapies are immature, however, as our understanding of materials and diseases deepens, potential applications of MSN-based DDS broaden. It is sure that chemotherapy-based nanosystems utilizing biocompatible MSNs have a bright adaptable future and great potential for clinical translation.
Author Contributions
YG and DG: wrote—original draft preparation. JS and QW: wrote—review and editing and funding acquisition. All authors have read and agreed to the published version of the manuscript.
Funding
National Natural Science Foundation of China (51603184 and 81800442), Zhejiang Post-doctoral Research Project (zj20180129), and China Post-doctoral Science Foundation funded project (2019M652121).
Conflict of Interest
The authors declare that the research was conducted in the absence of any commercial or financial relationships that could be construed as a potential conflict of interest.
References
Ajnai, G., Chiu, A., Kan, T., Cheng, C.-C., Tsai, T.-H., and Chang, J. (2014). Trends of gold nanoparticle-based drug delivery system in cancer therapy. J. Exp. Clin. Med. 6, 172–178. doi: 10.1016/j.jecm.2014.10.015
Akhtar, M. J., Ahamed, M., Alhadlaq, H. A., Alrokayan, S. A., and Kumar, S. (2014). Targeted anticancer therapy: overexpressed receptors and nanotechnology. Clin. Chim. Acta 436, 78–92. doi: 10.1016/j.cca.2014.05.004
Allen, T. M., and Cullis, P. R. (2013). Liposomal drug delivery systems: from concept to clinical applications. Adv. Drug Deliv. Rev. 65, 36–48. doi: 10.1016/j.addr.2012.09.037
Alsehli, M. (2020). Polymeric nanocarriers as stimuli-responsive systems for targeted tumor (cancer) therapy: recent advances in drug delivery. Saudi Pharm. J. 28, 255–265. doi: 10.1016/j.jsps.2020.01.004
Bartel, D. P. (2004). MicroRNAs: genomics, biogenesis, mechanism, and function. Cell 116, 281–297. doi: 10.1016/S0092-8674(04)00045-5
Bertucci, A., Prasetyanto, E. A., Septiadi, D., Manicardi, A., Brognara, E., Gambari, R., et al. (2015). Combined delivery of temozolomide and anti-miR221 PNA using mesoporous silica nanoparticles induces apoptosis in resistant glioma cells. Small 11, 5687–5695. doi: 10.1002/smll.201500540
Bhattaraia, S. R., Derry, P. J., Aziz, K., Singh, P. K., Khoo, A. M., Chadha, A. S., et al. (2017). Gold nanotriangles: scale up and x-ray radiosensitization effects in mice. Nanoscale. 9, 5085–5093. doi: 10.1039/c6nr08172j
Bouchoucha, M., Côté, M.-F., Gaudreault, R. C., Fortin, M.-A., and Kleitz, F. (2016). Size-controlled functionalized mesoporous silica nanoparticles for tunable drug release and enhanced anti-tumoral activity. Chem. Mater. 28, 4243–4258. doi: 10.1021/acs.chemmater.6b00877
Brollo, M. E. F., Orozco-Henao, J. M., López-Ruiz, R., Muraca, D., Dias, C. S. B., Pirota, K. R., et al. (2016). Magnetic hyperthermia in brick-like Ag@Fe3O4 core–shell nanoparticles. J. Magn. Magn. Mater. 397, 20–27. doi: 10.1016/j.jmmm.2015.08.081
Cai, D., Liu, L., Han, C., Ma, X., Qian, J., Zhou, J., et al. (2019). Cancer cell membrane-coated mesoporous silica loaded with superparamagnetic ferroferric oxide and Paclitaxel for the combination of chemo/magnetocaloric therapy on MDA-MB-231 cells. Sci. Rep. 9:14475. doi: 10.1038/s41598-019-51029-8
Chang, B., Chen, D., Wang, Y., Chen, Y., Jiao, Y., Sha, X., et al. (2013). Bioresponsive controlled drug release based on mesoporous silica nanoparticles coated with reductively sheddable polymer shell. Chem. Mater. 25, 574–585. doi: 10.1021/cm3037197
Chen, C., Tang, W., Jiang, D., Yang, G., Wang, X., Zhou, L., et al. (2019). Hyaluronic acid conjugated polydopamine functionalized mesoporous silica nanoparticles for synergistic targeted chemo-photothermal therapy. Nanoscale 11, 11012–11024. doi: 10.1039/C9NR01385G
Chen, D., Dougherty, C. A., Zhu, K., and Hong, H. (2015). Theranostic applications of carbon nanomaterials in cancer: focus on imaging and cargo delivery. J. Controlled Release 210, 230–245. doi: 10.1016/j.jconrel.2015.04.021
Chen, F., Hong, H., Goel, S., Graves, S. A., Orbay, H., Ehlerding, E. B., et al. (2015). In vivo tumor vasculature targeting of CuS@MSN based theranostic nanomedicine. ACS Nano 9, 3926–3934. doi: 10.1021/nn507241v
Chen, J., Ning, C., Zhou, Z., Yu, P., Zhu, Y., Tan, G., et al. (2019). Nanomaterials as photothermal therapeutic agents. Prog. Mater. Sci. 99, 1–26. doi: 10.1016/j.pmatsci.2018.07.005
Chen, K., Chang, C., Liu, Z., Zhou, Y., Xu, Q., Li, C., et al. (2020). Hyaluronic acid targeted and pH-responsive nanocarriers based on hollow mesoporous silica nanoparticles for chemo-photodynamic combination therapy. Colloids Surf. B Biointerfaces 194:111166. doi: 10.1016/j.colsurfb.2020.111166
Chen, L., Zhou, X., Nie, W., Zhang, Q., Wang, W., Zhang, Y., et al. (2016). Multifunctional redox-responsive mesoporous silica nanoparticles for efficient targeting drug delivery and magnetic resonance imaging. ACS Appl. Mater. Interfaces 8, 33829–33841. doi: 10.1021/acsami.6b11802
Chen, Y.-W., Chen, P.-J., Hu, S.-H., Chen, I.-W., and Chen, S.-Y. (2014). NIR-triggered synergic photo-chemothermal therapy delivered by reduced graphene oxide/carbon/mesoporous silica nanocookies. Adv. Funct. Mater. 24, 451–459. doi: 10.1002/adfm.201301763
Chen, Y.-W., Liu, T.-Y., Chang, P.-H., Hsu, P.-H., Liu, H.-L., Lin, H.-C., et al. (2016). A theranostic nrGO@MSN-ION nanocarrier developed to enhance the combination effect of sonodynamic therapy and ultrasound hyperthermia for treating tumor. Nanoscale 8, 12648–12657. doi: 10.1039/C5NR07782F
Chen, Z., Zhu, P., Zhang, Y., Liu, Y., He, Y., Zhang, L., et al. (2016). Enhanced sensitivity of cancer stem cells to chemotherapy using functionalized mesoporous silica nanoparticles. Mol. Pharm 13, 2749–2759. doi: 10.1021/acs.molpharmaceut.6b00352
Cheng, K., Ling, C., Gu, D., Gao, Z., Li, Y., An, P., et al. (2020). Multimodal therapies: glucose oxidase-triggered tumor starvation-induced synergism with enhanced chemodynamic therapy and chemotherapy. N. J. Chem. 44, 1524–1536. doi: 10.1039/C9NJ05469C
Cheng, K., Zhang, Y., Li, Y., Gao, Z., Chen, F., Sun, K., et al. (2019). A novel pH-responsive hollow mesoporous silica nanoparticle (HMSN) system encapsulating doxorubicin (DOX) and glucose oxidase (GOX) for potential cancer treatment. J. Mater. Chem. B 7, 3291–3302. doi: 10.1039/C8TB03198C
Cheng, Y.-J., Hu, J.-J., Qin, S.-Y., Zhang, A.-Q., and Zhang, X.-Z. (2020). Recent advances in functional mesoporous silica-based nanoplatforms for combinational photo-chemotherapy of cancer. Biomaterials 232:119738. doi: 10.1016/j.biomaterials.2019.119738
Cheng, Y.-J., Luo, G.-F., Zhu, J.-Y., Xu, X.-D., Zeng, X., Cheng, D.-B., et al. (2015). Enzyme-induced and tumor-targeted drug delivery system based on multifunctional mesoporous silica nanoparticles. ACS Appl. Mater. Interfaces 7, 9078–9087. doi: 10.1021/acsami.5b00752
Cheng, Y.-J., Qin, S.-Y., Ma, Y.-H., Chen, X.-S., Zhang, A.-Q., and Zhang, X.-Z. (2019). Super-pH-sensitive mesoporous silica nanoparticle-based drug delivery system for effective combination cancer therapy. ACS Biomater. Sci. Eng. 5, 1878–1886. doi: 10.1021/acsbiomaterials.9b00099
Cheng, Y. J., Zhang, A. Q., Hu, J. J., He, F., Zeng, X., and Zhang, X. Z. (2017). Multifunctional peptide-amphiphile end-capped mesoporous silica nanoparticles for tumor targeting drug delivery. ACS Appl. Mater. Interfaces 9, 2093–2103. doi: 10.1021/acsami.6b12647
Choi, B., Jung, H., Yu, B., Choi, H., Lee, J., and Kim, D.-H. (2019). Chemo-immunotherapy: sequential MR image-guided local immune checkpoint blockade cancer immunotherapy using ferumoxytol capped ultralarge pore mesoporous silica carriers after standard chemotherapy (small 52/2019). Small 15:1970281. doi: 10.1002/smll.201970281
Croissant, J. G., Fatieiev, Y., Almalik, A., and Khashab, N. M. (2018). Mesoporous silica and organosilica nanoparticles: physical chemistry, biosafety, delivery strategies, and biomedical applications. Adv. Healthcare Mater. 7. doi: 10.1002/adhm.201700831
Dai, L., Liu, J., Luo, Z., Li, M., and Cai, K. (2016). Tumor therapy: targeted drug delivery systems. J. Mater. Chem. B 4, 6758–6772. doi: 10.1039/C6TB01743F
Deng, Y., Wang, C. C., Choy, K. W., Du, Q., Chen, J., Wang, Q., et al. (2014). Therapeutic potentials of gene silencing by RNA interference: principles, challenges, and new strategies. Gene 538, 217–227. doi: 10.1016/j.gene.2013.12.019
Dias, A. P., Da Silva Santos, S., Da Silva, J. V., Parise-Filho, R., Igne Ferreira, E., Seoud, O. E., et al. (2020). Dendrimers in the context of nanomedicine. Int. J. Pharm 573:118814. doi: 10.1016/j.ijpharm.2019.118814
Dilnawaz, F., and Sahoo, S. K. (2018). Augmented anticancer efficacy by si-RNA complexed drug-loaded mesoporous silica nanoparticles in lung cancer therapy. ACS Appl. Nano Mater. 1, 730–740. doi: 10.1021/acsanm.7b00196
Ding, Y., Song, Z., Liu, Q., Wei, S., Zhou, L., Zhou, J., et al. (2017). An enhanced chemotherapeutic effect facilitated by sonication of MSN. Dalton Trans. 46, 11875–11883. doi: 10.1039/C7DT02600E
Ding, Y.-F., Li, S., Liang, L., Huang, Q., Yuwen, L., Yang, W., et al. (2018). Highly biocompatible chlorin e6-loaded Chitosan nanoparticles for improved photodynamic cancer therapy. ACS Appl. Mater. Interfaces 10, 9980–9987. doi: 10.1021/acsami.8b01522
Dong, K., Li, Z., Sun, H., Ju, E., Ren, J., and Qu, X. (2017). Pathogen-mimicking nanocomplexes: self-stimulating oxidative stress in tumor microenvironment for chemo-immunotherapy. Mater. Today 20, 346–353. doi: 10.1016/j.mattod.2017.06.003
Dyck, L., and Mills, K. H. G. (2017). Immune checkpoints and their inhibition in cancer and infectious diseases. Eur. J. Immunol. 47, 765–779. doi: 10.1002/eji.201646875
Fan, W., Yung, B., Huang, P., and Chen, X. (2017). Nanotechnology for multimodal synergistic cancer therapy. Chem. Rev. 117, 13566–13638. doi: 10.1021/acs.chemrev.7b00258
Fang, J., Wang, Q., Yang, G., Xiao, X., Li, L., and Yu, T. (2019). Albumin-MnO2 gated hollow mesoporous silica nanosystem for modulating tumor hypoxia and synergetic therapy of cervical carcinoma. Colloids Surf. B Biointerfaces 179, 250–259. doi: 10.1016/j.colsurfb.2019.03.070
Fang, S., Lin, J., Li, C., Huang, P., Hou, W., Zhang, C., et al. (2017). Dual-stimuli responsive nanotheranostics for multimodal imaging guided trimodal synergistic therapy. Small 13:1602580. doi: 10.1002/smll.201602580
Farjadian, F., Roointan, A., Mohammadi-Samani, S., and Hosseini, M. (2019). Mesoporous silica nanoparticles: synthesis, pharmaceutical applications, biodistribution, and biosafety assessment. Chem. Eng. J. 359, 684–705. doi: 10.1016/j.cej.2018.11.156
Fire, A., Xu, S., Montgomery, M. K., Kostas, S. A., Driver, S. E., and Mello, C. C. (1998). Potent andspecific genetic interferenceby double-strandedRNA in Caenorhabditiselegans. Nat. Nanotechnol. 391, 806–811. doi: 10.1038/35888
Fu, L.-H., Qi, C., Lin, J., and Huang, P. (2018). Catalytic chemistry of glucose oxidase in cancer diagnosis and treatment. Chem. Soc. Rev. 47, 6454–6472. doi: 10.1039/C7CS00891K
Fu, X., Yang, Z., Deng, T., Chen, J., Wen, Y., Fu, X., et al. (2020). A natural polysaccharide mediated MOF-based Ce6 delivery system with improved biological properties for photodynamic therapy. J. Mater. Chem. B 8, 1481–1488. doi: 10.1039/C9TB02482D
Guisasola, E., Asín, L., Beola, L., De La Fuente, J. M., Baeza, A., and Vallet-Regí, M. (2018). Beyond traditional hyperthermia: in vivo cancer treatment with magnetic-responsive mesoporous silica nanocarriers. ACS Appl. Mater. Interfaces 10, 12518–12525. doi: 10.1021/acsami.8b02398
Guo, D., Bao, Y., Zhang, Y., Yang, H., and Chen, L. (2020). Reduction-responsive Au decorated mesoporous silica-based nanoplatform for photodynamic-chemotherapy. Microporous Mesoporous Mater. 292:109729. doi: 10.1016/j.micromeso.2019.109729
Hachimine, K., Shibaguchi, H., Kuroki, M., Yamada, H., Kinugasa, T., Nakae, Y., et al. (2007). Sonodynamic therapy of cancer using a novel porphyrin derivative, DCPH-P-Na(I), which is devoid of photosensitivity. Cancer Sci. 98, 916–920. doi: 10.1111/j.1349-7006.2007.00468.x
Hao, H., Sun, M., Li, P., Sun, J., Liu, X., and Gao, W. (2019). In situ growth of a cationic polymer from the N-terminus of glucose oxidase to regulate H2O2 Generation for cancer starvation and H2O2 therapy. ACS Appl. Mater. Interfaces 11, 9756–9762. doi: 10.1021/acsami.8b20956
Harada, Y., Ogawa, K., Irie, Y., Endo, H., Feril, L. B., Uemura, T., et al. (2011). Ultrasound activation of TiO2 in melanoma tumors. J. Controlled Release 149, 190–195. doi: 10.1016/j.jconrel.2010.10.012
Hauck, T. S., Jennings, T. L., Yatsenko, T., Kumaradas, J. C., and Chan, W. C. W. (2008). Enhancing the toxicity of cancer chemotherapeutics with gold nanorod hyperthermia. Adv. Mater. 20, 3832–3838. doi: 10.1002/adma.200800921
Huang, X., Wu, S., Ke, X., Li, X., and Du, X. (2017). Phosphonated pillar[5]arene-valved mesoporous silica drug delivery systems. ACS Appl. Mater. Interfaces 9, 19638–19645. doi: 10.1021/acsami.7b04015
Huo, M., Wang, L., Chen, Y., and Shi, J. (2017). Tumor-selective catalytic nanomedicine by nanocatalyst delivery. Nat. Commun. 8:357. doi: 10.1038/s41467-017-00424-8
Kankala, R. K., Liu, C.-G., Chen, A.-Z., Wang, S.-B., Xu, P.-Y., Mende, L. K., et al. (2017). Overcoming multidrug resistance through the synergistic effects of hierarchical pH-sensitive, ROS-generating nanoreactors. ACS Biomaterials Sci. Eng. 3, 2431–2442. doi: 10.1021/acsbiomaterials.7b00569
Kobayashi, T. (2011). Cancer hyperthermia using magnetic nanoparticles. Biotechnol. J. 6, 1342–1347. doi: 10.1002/biot.201100045
Kong, M., Tang, J., Qiao, Q., Wu, T., Qi, Y., Tan, S., et al. (2017). Biodegradable hollow mesoporous silica nanoparticles for regulating tumor microenvironment and enhancing antitumor efficiency. Theranostics 7, 3276–3292. doi: 10.7150/thno.19987
Kroemer, G., Galluzzi, L., Kepp, O., and Zitvogel, L. (2013). Immunogenic cell death in cancer therapy. Annu. Rev. Immunol. 31, 51–72. doi: 10.1146/annurev-immunol-032712-100008
Lafond, M., Yoshizawa, S., and Umemura, S.-I. (2019). Sonodynamic therapy: advances and challenges in clinical translation. J. Ultrasound Med. 38, 567–580. doi: 10.1002/jum.14733
Lake, R. A., and Robinson, B. W. S. (2005). Immunotherapy and chemotherapy—a practical partnership. Nat. Rev. Cancer 5, 397–405. doi: 10.1038/nrc1613
Lee, J. H., Kang, S., Ahn, M., Jang, H., and Min, D. H. (2018). Development of dual-pore coexisting branched silica nanoparticles for efficient gene-chemo cancer therapy. Small 14. doi: 10.1002/smll.201702564
Leonidova, A., Pierroz, V., Rubbiani, R., Lan, Y., Schmitz, A. G., Kaech, A., et al. (2014). Photo-induced uncaging of a specific Re(i) organometallic complex in living cells. Chem. Sci. 5, 4044–4056. doi: 10.1039/C3SC53550A
Li, D., Zhang, T., Min, C., Huang, H., Tan, D., and Gu, W. (2020). Biodegradable theranostic nanoplatforms of albumin-biomineralized nanocomposites modified hollow mesoporous organosilica for photoacoustic imaging guided tumor synergistic therapy. Chem. Eng. J. 388:124253. doi: 10.1016/j.cej.2020.124253
Li, T., Shen, X., Geng, Y., Chen, Z., Li, L., Li, S., et al. (2016). Folate-functionalized magnetic-mesoporous silica nanoparticles for drug/gene codelivery to potentiate the antitumor efficacy. ACS Appl. Mater. Interfaces 8, 13748–13758. doi: 10.1021/acsami.6b02963
Li, T., Shi, S., Goel, S., Shen, X., Xie, X., Chen, Z., et al. (2019). Recent advancements in mesoporous silica nanoparticles towards therapeutic applications for cancer. Acta Biomater. 89, 1–13. doi: 10.1016/j.actbio.2019.02.031
Li, X., He, G., Jin, H., Tao, J., Li, X., Zhai, C., et al. (2019). Dual-therapeutics-loaded mesoporous silica nanoparticles applied for breast tumor therapy. ACS Appl. Mater. Interfaces 11, 46497–46503. doi: 10.1021/acsami.9b16270
Li, X., Xie, C., Xia, H., and Wang, Z. (2018). pH and ultrasound dual-responsive polydopamine-coated mesoporous silica nanoparticles for controlled drug delivery. Langmuir 34, 9974–9981. doi: 10.1021/acs.langmuir.8b01091
Li, Z., Li, Z., Sun, L., Du, B., Wang, Y., Zhao, G., et al. (2018). Core–Shell Bi2Se3@mSiO2-PEG as a multifunctional drug-delivery nanoplatform for synergistic thermo-chemotherapy with infrared thermal imaging of cancer cells. Part. Part. Syst. Characterization 35:1700337. doi: 10.1002/ppsc.201700337
Liao, P.-Y., Gao, Y.-H., Wang, X.-R., Bao, L.-L., Bian, J., Hu, T.-S., et al. (2016). Tetraphenylporphyrin derivatives possessing piperidine group as potential agents for photodynamic therapy. J. Photochem. Photobiol. B Biol. 165, 213–219. doi: 10.1016/j.jphotobiol.2016.10.031
Lin, J.-T., Liu, Z.-K., Zhu, Q.-L., Rong, X.-H., Liang, C.-L., Wang, J., et al. (2017). Redox-responsive nanocarriers for drug and gene co-delivery based on chitosan derivatives modified mesoporous silica nanoparticles. Colloids Surf. B Biointerfaces 155, 41–50. doi: 10.1016/j.colsurfb.2017.04.002
Liu, H.-J., Luan, X., Feng, H.-Y., Dong, X., Yang, S.-C., Chen, Z.-J., et al. (2018). Integrated combination treatment using a “smart” chemotherapy and MicroRNA delivery system improves outcomes in an orthotopic colorectal cancer model. Adv. Funct. Mater. 28:1801118. doi: 10.1002/adfm.201801118
Liu, J., Detrembleur, C., De Pauw-Gillet, M.-C., Mornet, S., Jérôme, C., and Duguet, E. (2015). Gold nanorods coated with mesoporous silica shell as drug delivery system for remote near infrared light-activated release and potential phototherapy. Small 11, 2323–2332. doi: 10.1002/smll.201402145
Liu, R., Zhang, H., Zhang, F., Wang, X., Liu, X., and Zhang, Y. (2019). Polydopamine doped reduced graphene oxide/mesoporous silica nanosheets for chemo-photothermal and enhanced photothermal therapy. Mater. Sci. Eng. C 96, 138–145. doi: 10.1016/j.msec.2018.10.093
Lu, J., Liu, F., Li, H., Xu, Y., and Sun, S. (2020). Width-consistent mesoporous silica nanorods with a precisely controlled aspect ratio for lysosome dysfunctional synergistic chemotherapy/photothermal therapy/starvation therapy/oxidative therapy. ACS Appl. Mater. Interfaces 12, 24611–24622. doi: 10.1021/acsami.0c06117
Luo, G.-F., Chen, W.-H., Lei, Q., Qiu, W.-X., Liu, Y.-X., Cheng, Y.-J., et al. (2016). A triple-collaborative strategy for high-performance tumor therapy by multifunctional mesoporous silica-coated gold nanorods. Adv. Funct. Mater. 26, 4339–4350. doi: 10.1002/adfm.201505175
Luo, L., Shu, R., and Wu, A. (2017). Nanomaterial-based cancer immunotherapy. J. Mater. Chem. B 5, 5517–5531. doi: 10.1039/C7TB01137G
Mu, W., Chu, Q., Liu, Y., and Zhang, N. (2020). A review on nano-based drug delivery system for cancer chemoimmunotherapy. Nano Micro Lett. 12:142. doi: 10.1007/s40820-020-00482-6
Murugan, C., Rayappan, K., Thangam, R., Bhanumathi, R., Shanthi, K., Vivek, R., et al. (2016). Combinatorial nanocarrier based drug delivery approach for amalgamation of anti-tumor agents in breast cancer cells: an improved nanomedicine strategy. Sci. Rep. 6:34053. doi: 10.1038/srep34053
Murugan, C., Venkatesan, S., and Kannan, S. (2017). Cancer therapeutic proficiency of dual-targeted mesoporous silica nanocomposite endorses combination drug delivery. ACS Omega 2, 7959–7975. doi: 10.1021/acsomega.7b00978
Nie, C., Chu, X., Pan, Q., Zhang, J., Hu, Y., Yi, J., et al. (2020). Engineering a biodegradable nanocarrier for enhancing the response of T98G cells to temozolomide. ACS Appl. Bio Mater. 3, 3337–3344. doi: 10.1021/acsabm.0c00253
Oh, J. S., You, Y., Park, K. C., Gupta, G., Kang, D.-K., and Lee, C. Y. (2019). Toward an efficient photosensitizer for photodynamic therapy: incorporating BODIPY into porphyrinic nanoscale MOFs through the solvent-assisted ligand incorporation. Dyes Pigments 170:107576. doi: 10.1016/j.dyepig.2019.107576
Osminkina, L. A., Nikolaev, A. L., Sviridov, A. P., Andronova, N. V., Tamarov, K. P., Gongalsky, M. B., et al. (2015). Porous silicon nanoparticles as efficient sensitizers for sonodynamic therapy of cancer. Microporous Mesoporous Mater. 210, 169–175. doi: 10.1016/j.micromeso.2015.02.037
Pan, Q. S., Chen, T. T., Nie, C. P., Yi, J. T., Liu, C., Hu, Y. L., et al. (2018). In situ synthesis of ultrathin ZIF-8 film-coated MSNs for codelivering Bcl 2 siRNA and doxorubicin to enhance chemotherapeutic efficacy in drug-resistant cancer cells. ACS Appl. Mater. Interfaces 10, 33070–33077. doi: 10.1021/acsami.8b13393
Peng, S., He, Y., Er, M., Sheng, Y., Gu, Y., and Chen, H. (2017). Biocompatible CuS-based nanoplatforms for efficient photothermal therapy and chemotherapy in vivo. Biomater. Sci. 5, 475–484. doi: 10.1039/C6BM00626D
Qian, X., Zheng, Y., and Chen, Y. (2016). Micro/nanoparticle-augmented sonodynamic therapy (SDT): breaking the depth shallow of photoactivation. Adv. Mater. 28, 8097–8129. doi: 10.1002/adma.201602012
Qin, S.-Y., Cheng, Y.-J., Lei, Q., Zhang, A.-Q., and Zhang, X.-Z. (2018). Combinational strategy for high-performance cancer chemotherapy. Biomaterials 171, 178–197. doi: 10.1016/j.biomaterials.2018.04.027
Qiu, L., Hu, X., Jing, Q., Zeng, X., Chan, K.-M., and Han, J. (2018). Mechanism of cancer: Oncohistones in action. Journal of Genetics and Genomics 45, 227–236. doi: 10.1016/j.jgg.2018.04.004
Qiu, M., Ren, W. X., Jeong, T., Won, M., Park, G. Y., Sang, D. K., et al. (2018). Omnipotent phosphorene: a next-generation, two-dimensional nanoplatform for multidisciplinary biomedical applications. Chem. Soc. Rev. 47, 5588–5601. doi: 10.1039/C8CS00342D
Rahman, Z. U., Zhang, T., Feng, Y., Ye, W., and Wang, D. (2017). Preparation of gold shells on hollow mesoporous silica nanospheres and application to photothermal-chemotherapy. ChemistrySelect 2, 3969–3975. doi: 10.1002/slct.201700200
Ramasamy, T., Ruttala, H. B., Sundaramoorthy, P., Poudel, B. K., Youn, Y. S., Ku, S. K., et al. (2018). Multimodal selenium nanoshell-capped Au@mSiO2 nanoplatform for NIR-responsive chemo-photothermal therapy against metastatic breast cancer. NPG Asia Mater. 10, 197–216. doi: 10.1038/s41427-018-0034-5
Ren, X., Shi, L., Yu, X., Liu, W., Sheng, J., Wan, J., et al. (2020). Multifunctional hierarchical mesoporous silica and black phosphorus nanohybrids as chemo-photothermal synergistic agents for enhanced cancer therapy. Nanoscale 12, 12578–12588. doi: 10.1039/D0NR02044C
Robertson, C. A., Evans, D. H., and Abrahamse, H. (2009). Photodynamic therapy (PDT): a short review on cellular mechanisms and cancer research applications for PDT. J. Photochem. Photobiol. B Biol. 96, 1–8. doi: 10.1016/j.jphotobiol.2009.04.001
Schreiber, R. D., Old, L. J., and Smyth, M. J. (2011). Cancer immunoediting: integrating immunity's roles in cancer suppression and promotion. Science 331:1565. doi: 10.1126/science.1203486
Shen, J., Liu, H., Mu, C., Wolfram, J., Zhang, W., Kim, H. C., et al. (2017). Multi-step encapsulation of chemotherapy and gene silencing agents in functionalized mesoporous silica nanoparticles. Nanoscale 9, 5329–5341. doi: 10.1039/C7NR00377C
Shen, J., Wang, Q., Hu, Q., Li, Y., Tang, G., and Chu, P. K. (2014). Restoration of chemosensitivity by multifunctional micelles mediated by P-gp siRNA to reverse MDR. Biomaterials 35, 8621–8634. doi: 10.1016/j.biomaterials.2014.06.035
Shrestha, B., Tang, L., and Romero, G. (2019). Nanoparticles-mediated combination therapies for cancer treatment. Adv. Ther. 2:1900076. doi: 10.1002/adtp.201900076
Shu, Y., Song, R., Zheng, A., Huang, J., Chen, M., and Wang, J. (2018). Thermo/pH dual-stimuli-responsive drug delivery for chemo-/photothermal therapy monitored by cell imaging. Talanta 181, 278–285. doi: 10.1016/j.talanta.2018.01.018
Siegel, R. L., Miller, K. D., and Jemal, A. (2020). Cancer statistics, 2020. CA Cancer J. Clin. 70, 7–30. doi: 10.3322/caac.21590
Singh, R. K., Patel, K. D., Mahapatra, C., Kang, M. S., and Kim, H.-W. (2016). C-dot generated bioactive organosilica nanospheres in theranostics: multicolor luminescent and photothermal properties combined with drug delivery capacity. ACS Appl. Mater. Interfaces 8, 24433–24444. doi: 10.1021/acsami.6b07494
Song, Y., Zhou, B., Du, X., Wang, Y., Zhang, J., Ai, Y., et al. (2020). Folic acid (FA)-conjugated mesoporous silica nanoparticles combined with MRP-1 siRNA improves the suppressive effects of myricetin on non-small cell lung cancer (NSCLC). Biomed. Pharmacother. 125:109561. doi: 10.1016/j.biopha.2019.109561
Sugita, N., Hosokawa, M., Sunaga, N., Iwase, Y., Yumita, N., Ikeda, T., et al. (2015). Sonodynamically-induced cytotoxicity by rose bengal derivative and microbubbles in isolated sarcoma 180 cells. Jap. J. Appl. Phys. 54:07HF16. doi: 10.7567/JJAP.54.07HF16
Sun, L., Wang, D., Chen, Y., Wang, L., Huang, P., Li, Y., et al. (2017). Core-shell hierarchical mesostructured silica nanoparticles for gene/chemo-synergetic stepwise therapy of multidrug-resistant cancer. Biomaterials 133, 219–228. doi: 10.1016/j.biomaterials.2017.04.028
Sun, Q., You, Q., Wang, J., Liu, L., Wang, Y., Song, Y., et al. (2018). Theranostic nanoplatform: triple-modal imaging-guided synergistic cancer therapy based on liposome-conjugated mesoporous silica nanoparticles. ACS Appl. Mater. Interfaces 10, 1963–1975. doi: 10.1021/acsami.7b13651
Sun, X., Jin, Y., Wang, H., Feng, N., Li, Z., Liu, D., et al. (2018). A NIR-light activated nanoplatform for sensitizing triple negative breast cancer against therapeutic resistance to enhance the treatment effect. J. Mater. Chem. B 6, 6950–6956. doi: 10.1039/C8TB01723A
Tang, Y., Hu, H., Zhang, M. G., Song, J., Nie, L., Wang, S., et al. (2015). An aptamer-targeting photoresponsive drug delivery system using “off–on” graphene oxide wrapped mesoporous silica nanoparticles. Nanoscale 7, 6304–6310. doi: 10.1039/C4NR07493A
Tian, Z., Yu, X., Ruan, Z., Zhu, M., Zhu, Y., and Hanagata, N. (2018). Magnetic mesoporous silica nanoparticles coated with thermo-responsive copolymer for potential chemo- and magnetic hyperthermia therapy. Microporous Mesoporous Mater. 256, 1–9. doi: 10.1016/j.micromeso.2017.07.053
Tran, A.-V., Shim, K., Vo Thi, T.-T., Kook, J.-K., An, S. S. A., and Lee, S.-W. (2018). Targeted and controlled drug delivery by multifunctional mesoporous silica nanoparticles with internal fluorescent conjugates and external polydopamine and graphene oxide layers. Acta Biomater. 74, 397–413. doi: 10.1016/j.actbio.2018.05.022
Tsai, P. H., Wang, M. L., Chang, J. H., Yarmishyn, A. A., Nhi Nguyen, P. N., Chen, W., et al. (2019). Dual Delivery of HNF4alpha and cisplatin by mesoporous silica nanoparticles inhibits cancer pluripotency and tumorigenicity in hepatoma-derived CD133-expressing stem cells. ACS Appl. Mater. Interfaces 11, 19808–19818. doi: 10.1021/acsami.9b04474
Wang, F., Wang, Z., Li, Y., Zhao, L., Wen, Y., and Zhang, X. (2018). Cap-free dual stimuli-responsive biodegradable nanocarrier for controlled drug release and chemo-photothermal therapy. Journal of Materials Chemistry B 6, 8188–8195. doi: 10.1039/C8TB02698J
Wang, H., Zhang, C., Zhang, Y., Tian, R., Cheng, G., Pan, H., et al. (2020). An efficient delivery of photosensitizers and hypoxic prodrugs for a tumor combination therapy by membrane camouflage nanoparticles. J. Mater. Chem. B 8, 2876–2886. doi: 10.1039/D0TB00235F
Wang, J., Seebacher, N., Shi, H., Kan, Q., and Duan, Z. (2017). Novel strategies to prevent the development of multidrug resistance (MDR) in cancer. Oncotarget 8, 84559–84571. doi: 10.18632/oncotarget.19187
Wang, L., Guan, H., Wang, Z., Xing, Y., Zhang, J., and Cai, K. (2018). Hybrid mesoporous–microporous nanocarriers for overcoming multidrug resistance by sequential drug delivery. Mol. Pharm. 15, 2503–2512. doi: 10.1021/acs.molpharmaceut.7b01096
Wang, S., Liu, X., Chen, S., Liu, Z., Zhang, X., Liang, X. J., et al. (2019). Regulation of Ca(2+) signaling for drug-resistant breast cancer therapy with mesoporous silica nanocapsule encapsulated doxorubicin/siRNA cocktail. ACS Nano 13, 274–283. doi: 10.1021/acsnano.8b05639
Wang, T. T., Lan, J., Zhang, Y., Wu, Z. L., Li, C. M., Wang, J., et al. (2015). Reduced graphene oxide gated mesoporous silica nanoparticles as a versatile chemo-photothermal therapy system through pH controllable release. J. Mater. Chem. B 3, 6377–6384. doi: 10.1039/C5TB00824G
Wang, W., Jin, Y., Xu, Z., Liu, X., Bajwa, S. Z., Khan, W. S., et al. (2020). Stimuli-activatable nanomedicines for chemodynamic therapy of cancer. WIREs Nanomed. Nanobiotechnol. 12:e1614. doi: 10.1002/wnan.1614
Wang, X., Ma, Y., Chen, H., Wu, X., Qian, H., Yang, X., et al. (2017). Novel doxorubicin loaded PEGylated cuprous telluride nanocrystals for combined photothermal-chemo cancer treatment. Colloids Surf. B Biointerfaces 152, 449–458. doi: 10.1016/j.colsurfb.2017.02.002
Wang, Y., Wang, L., Guo, L., Yan, M., Feng, L., Dong, S., et al. (2019). Photo-responsive magnetic mesoporous silica nanocomposites for magnetic targeted cancer therapy. N. J. Chem. 43, 4908–4918. doi: 10.1039/C8NJ06105J
Wang, Z., Chang, Z. M., Shao, D., Zhang, F., Chen, F., Li, L., et al. (2019). Janus gold triangle-mesoporous silica nanoplatforms for hypoxia-activated radio-chemo-photothermal therapy of liver cancer. ACS Appl. Mater. Interfaces 11, 34755–34765. doi: 10.1021/acsami.9b12879
Warburg, O., Wind, F., and Negelein, E. (1927). The metabolism of tumors in the body. J. Gen. Physiol. 8, 519–530. doi: 10.1085/jgp.8.6.519
Wong, R. C. H., Ng, D. K. P., Fong, W.-P., and Lo, P.-C. (2020). Glutathione- and light-controlled generation of singlet oxygen for triggering drug release in mesoporous silica nanoparticles. J. Mater. Chem. B 8, 4460–4468. doi: 10.1039/D0TB00636J
Wu, M., Lin, X., Tan, X., Li, J., Wei, Z., Zhang, D., et al. (2018). Photoresponsive nanovehicle for two independent wavelength light-triggered sequential release of p-gp shrna and doxorubicin to optimize and enhance synergistic therapy of multidrug-resistant cancer. ACS Appl. Mater. Interfaces 10, 19416–19427. doi: 10.1021/acsami.8b03823
Xiao, J., Cheng, L., Fang, T., Zhang, Y., Zhou, J., Cheng, R., et al. (2019). Nanoparticle-embedded electrospun fiber–covered stent to assist intraluminal photodynamic treatment of oesophageal cancer. Small 15:1904979. doi: 10.1002/smll.201904979
Xing, Y., Zhou, Y., Zhang, Y., Zhang, C., Deng, X., Dong, C., et al. (2020). Facile fabrication route of janus gold-mesoporous silica nanocarriers with dual-drug delivery for tumor therapy. ACS Biomater. Sci. Eng. 6, 1573–1581. doi: 10.1021/acsbiomaterials.0c00042
Xu, X., Ho, W., Zhang, X., Bertrand, N., and Farokhzad, O. (2015). Cancer nanomedicine: from targeted delivery to combination therapy. Trends Mol. Med. 21, 223–232. doi: 10.1016/j.molmed.2015.01.001
Yan, T., Cheng, J., Liu, Z., Cheng, F., Wei, X., and He, J. (2018). pH-sensitive mesoporous silica nanoparticles for chemo-photodynamic combination therapy. Colloids Surf. B Biointerfaces 161, 442–448. doi: 10.1016/j.colsurfb.2017.11.006
Yan, T., He, J., Liu, R., Liu, Z., and Cheng, J. (2020). Chitosan capped pH-responsive hollow mesoporous silica nanoparticles for targeted chemo-photo combination therapy. Carbohydr. Polym. 231:115706. doi: 10.1016/j.carbpol.2019.115706
Yang, D., Yang, G., Gai, S., He, F., Lv, R., Dai, Y., et al. (2016). Imaging-guided and light-triggered chemo-/photodynamic/photothermal therapy based on Gd (III) chelated mesoporous silica hybrid spheres. ACS Biomater. Sci. Eng. 2, 2058–2071. doi: 10.1021/acsbiomaterials.6b00462
Yang, G., Sun, X., Liu, J., Feng, L., and Liu, Z. (2016). Light-responsive, singlet-oxygen-triggered on-demand drug release from photosensitizer-doped mesoporous silica nanorods for cancer combination therapy. Adv. Funct. Mater. 26, 4722–4732. doi: 10.1002/adfm.201600722
Yang, H., Chen, Y., Chen, Z., Geng, Y., Xie, X., Shen, X., et al. (2017). Chemo-photodynamic combined gene therapy and dual-modal cancer imaging achieved by pHresponsive alginate/chitosan multilayer-modified magnetic mesoporous silica nanocomposites. Biomater. Sci. 5, 1001–1013. doi: 10.1039/C7BM00043J
Yang, Y., Lin, Y., Di, D., Zhang, X., Wang, D., Zhao, Q., et al. (2017). Gold nanoparticle-gated mesoporous silica as redox-triggered drug delivery for chemo-photothermal synergistic therapy. J. Colloid Interface Sci. 508, 323–331. doi: 10.1016/j.jcis.2017.08.050
Yao, X., Chen, X., He, C., Chen, L., and Chen, X. (2015). Dual pH-responsive mesoporous silica nanoparticles for efficient combination of chemotherapy and photodynamic therapy. J. Mater. Chem. B 3, 4707–4714. doi: 10.1039/C5TB00256G
Yu, S., He, C., and Chen, X. (2018). Injectable hydrogels as unique platforms for local chemotherapeutics-based combination antitumor therapy. Macromol. Biosci. 18:1800240. doi: 10.1002/mabi.201800240
Yumita, N., Kawabata, K.-I., Sasaki, K., and Umemura, S.-I. (2002). Sonodynamic effect of erythrosin B on sarcoma 180 cells in vitro. Ultrason. Sonochem. 9, 259–265. doi: 10.1016/S1350-4177(02)00080-9
Yumita, N., Okudaira, K., Momose, Y., and Umemura, S.-I. (2010). Sonodynamically induced apoptosis and active oxygen generation by gallium–porphyrin complex, ATX-70. Cancer Chemother. Pharmacol. 66, 1071–1078. doi: 10.1007/s00280-010-1264-6
Zhang, H., Wang, X., Wang, P., Liu, R., Hou, X., Cao, W., et al. (2018). One-pot synthesis of biodegradable polydopamine-doped mesoporous silica nanocomposites (PMSNs) as pH-sensitive targeting drug nanocarriers for synergistic chemo-photothermal therapy. RSC Adv. 8, 37433–37440. doi: 10.1039/C8RA07467D
Zhang, L., Li, Y., Jin, Z., Chan, K. M., and Yu, J. C. (2015a). Mesoporous carbon/CuS nanocomposites for pH-dependent drug delivery and near-infrared chemo-photothermal therapy. RSC Adv. 5, 93226–93233. doi: 10.1039/C5RA19458J
Zhang, L., Li, Y., Jin, Z., Yu, J. C., and Chan, K. M. (2015b). An NIR-triggered and thermally responsive drug delivery platform through DNA/copper sulfide gates. Nanoscale 7, 12614–12624. doi: 10.1039/C5NR02767E
Zhang, L., Zhang, Y., Xue, Y., Wu, Y., Wang, Q., Xue, L., et al. (2019). Transforming weakness into strength: photothermal-therapy-induced inflammation enhanced cytopharmaceutical chemotherapy as a combination anticancer treatment. Adv. Mater. 31:1805936. doi: 10.1002/adma.201805936
Zhang, Q., Shen, C., Zhao, N., and Xu, F.-J. (2017). Redox-responsive and drug-embedded silica nanoparticles with unique self-destruction features for efficient gene/drug codelivery. Adv. Funct. Mater. 27:1606229. doi: 10.1002/adfm.201606229
Zhang, W., Shen, J., Su, H., Mu, G., Sun, J.-H., Tan, C.-P., et al. (2016). Co-delivery of cisplatin prodrug and chlorin e6 by mesoporous silica nanoparticles for chemo-photodynamic combination therapy to combat drug resistance. ACS Appl. Mater. Interfaces 8, 13332–13340. doi: 10.1021/acsami.6b03881
Zhang, Y., Eltayeb, O., Meng, Y., Zhang, G., Zhang, Y., Shuang, S., et al. (2020a). Tumor microenvironment responsive mesoporous silica nanoparticles for dual delivery of doxorubicin and chemodynamic therapy (CDT) agent. New Journal of Chemistry 44, 2578–2586. doi: 10.1039/C9NJ05427H
Zhang, Y., Hou, Z., Ge, Y., Deng, K., Liu, B., Li, X., et al. (2015). DNA-hybrid-gated photothermal mesoporous silica nanoparticles for NIR-responsive and aptamer-targeted drug delivery. ACS Appl. Mater. Interfaces 7, 20696–20706. doi: 10.1021/acsami.5b05522
Zhang, Y., Li, Y., Gao, Z., Ding, B., An, P., Zhang, X., et al. (2020b). Mesoporous silica-coated silver nanoframes as drug-delivery vehicles for chemo/starvation/metal ion multimodality therapy. Langmuir 36, 6345–6351. doi: 10.1021/acs.langmuir.0c00191
Zhang, Z., Wang, L., Wang, J., Jiang, X., Li, X., Hu, Z., et al. (2012). Theranostics: mesoporous silica-coated gold nanorods as a light-mediated multifunctional theranostic platform for cancer treatment (Adv. Mater. 11/2012). Adv. Mater. 24, 1349–1349. doi: 10.1002/adma.201290063
Zhao, H., Zhao, B., Li, L., Ding, K., Xiao, H., Zheng, C., et al. (2020). Biomimetic decoy inhibits tumor growth and lung metastasis by reversing the drawbacks of sonodynamic therapy. Adv. Healthc. Mater 9:1901335. doi: 10.1002/adhm.201901335
Zhao, S., Xu, M., Cao, C., Yu, Q., Zhou, Y., and Liu, J. (2017). A redox-responsive strategy using mesoporous silica nanoparticles for co-delivery of siRNA and doxorubicin. J. Mater. Chem. B 5, 6908–6919. doi: 10.1039/C7TB00613F
Zhao, T., Wu, H., Yao, S. Q., Xu, Q.-H., and Xu, G. Q. (2010). Nanocomposites containing gold nanorods and porphyrin-doped mesoporous silica with dual capability of two-photon imaging and photosensitization. Langmuir 26, 14937–14942. doi: 10.1021/la102556u
Zheng, D.-W., Chen, J.-L., Zhu, J.-Y., Rong, L., Li, B., Lei, Q., et al. (2016). Highly integrated nano-platform for breaking the barrier between chemotherapy and immunotherapy. Nano Lett. 16, 4341–4347. doi: 10.1021/acs.nanolett.6b01432
Zheng, M., Yue, C., Ma, Y., Gong, P., Zhao, P., Zheng, C., et al. (2013). Single-step assembly of DOX/ICG loaded lipid–polymer nanoparticles for highly effective chemo-photothermal combination therapy. ACS Nano 7, 2056–2067. doi: 10.1021/nn400334y
Zheng, Y., Zhang, Z., Liu, Q., Zhao, Y., Zheng, C., Hao, J., et al. (2020). Multifunctional nanomodulators regulate multiple pathways to enhance antitumor immunity. ACS Appl. Bio Mater. 3, 4635–4642. doi: 10.1021/acsabm.0c00513
Zhi, D., Yang, T., O'hagan, J., Zhang, S., and Donnelly, R. F. (2020). Photothermal therapy. J. Controlled Release 325, 52–71. doi: 10.1016/j.jconrel.2020.06.032
Zhou, S., Ding, C., Wang, C., and Fu, J. (2020). UV-light cross-linked and pH de-cross-linked coumarin-decorated cationic copolymer grafted mesoporous silica nanoparticles for drug and gene co-delivery in vitro. Mater. Sci. Eng. C Mater. Biol. Appl. 108:110469. doi: 10.1016/j.msec.2019.110469
Zhou, X., Chen, L., Nie, W., Wang, W., Qin, M., Mo, X., et al. (2016). Dual-responsive mesoporous silica nanoparticles mediated codelivery of doxorubicin and Bcl-2 SiRNA for targeted treatment of breast cancer. J. Phys. Chem. C 120, 22375–22387. doi: 10.1021/acs.jpcc.6b06759
Zhu, D., Hu, C., Liu, Y., Chen, F., Zheng, Z., and Wang, X. (2019). Enzyme-/redox-responsive mesoporous silica nanoparticles based on functionalized dopamine as nanocarriers for cancer therapy. ACS Omega. 4, 6097–6105. doi: 10.1021/acsomega.8b02537
Keywords: mesoporous silica nanoparticles (MSNs), drug delivery systems (DDS), chemotherapy, combined cancer therapies, phototherapy, gene therapy
Citation: Gao Y, Gao D, Shen J and Wang Q (2020) A Review of Mesoporous Silica Nanoparticle Delivery Systems in Chemo-Based Combination Cancer Therapies. Front. Chem. 8:598722. doi: 10.3389/fchem.2020.598722
Received: 25 August 2020; Accepted: 20 October 2020;
Published: 24 November 2020.
Edited by:
Xijian Liu, Shanghai University of Engineering Sciences, ChinaReviewed by:
Ajay Singh Karakoti, The University of Newcastle, AustraliaCao Li, Hubei University, China
Copyright © 2020 Gao, Gao, Shen and Wang. This is an open-access article distributed under the terms of the Creative Commons Attribution License (CC BY). The use, distribution or reproduction in other forums is permitted, provided the original author(s) and the copyright owner(s) are credited and that the original publication in this journal is cited, in accordance with accepted academic practice. No use, distribution or reproduction is permitted which does not comply with these terms.
*Correspondence: Jie Shen, c2hlbmpAenVjYy5lZHUuY24=; Qiwen Wang, d2FuZ3Fpd2VuQHpqdS5lZHUuY24=