- 1Technical Chemistry, Department of Chemistry, Chemical-Biological Centre, Umeå University, Umeå, Sweden
- 2Industrial Chemistry and Reaction Engineering, Department of Chemical Engineering, Johan Gadolin Process Chemistry Centre, Åbo Akademi University, Åbo-Turku, Finland
Ionic liquids (ILs) have been receiving much attention as solvents in various areas of biochemistry because of their various beneficial properties over the volatile solvents and ILs availability in myriad variants (perhaps as many as 108) owing to the possibility of paring one cation with several anions and vice-versa as well as formulations as zwitterions. Their potential as solvents lies in their tendency to offer both directional and non-directional forces toward a solute molecule. Because of these forces, ionic liquids easily undergo intermolecular interactions with a range of polar/non-polar solutes, including biomolecules such as proteins and DNA. The interaction of genomic species in aqueous/non-aqueous states assists in unraveling their structure and functioning, which have implications in various biomedical applications. The charge density of ionic liquids renders them hydrophilic and hydrophobic, which retain intact over long-range of temperatures. Their ability in stabilizing or destabilizing the 3D-structure of a protein or the double-helical structure of DNA has been assessed superior to the water and volatile organic solvents. The aptitude of an ion in influencing the structure and stability of a native protein depends on their ranking in the Hofmeister series. However, at several instances, a reverse Hofmeister ordering of ions and specific ion-solute interaction has been observed. The capability of an ionic liquid in terms of the tendency to promote the coiling/uncoiling of DNA structure is noted to rely on the basicity, electrostatic interaction, and hydrophobicity of the ionic liquid in question. Any change in the DNA's double-helical structure reflects a change in its melting temperature (Tm), compared to a standard buffer solution. These changes in DNA structure have implications in biosensor design and targeted drug-delivery in biomedical applications. In the current review, we have attempted to highlight various aspects of ionic liquids that influence the structure and properties of proteins and DNA. In short, the review will address the issues related to the origin and strength of intermolecular interactions, the effect of structural components, their nature, and the influence of temperature, pH, and additives on them.
Introduction
The energetics of a biological reaction/process change upon perturbing the solvent systems around it (Yancey et al., 1982). Molecular solvents, depending upon its nature, might offer various interactions ranging from dipole-dipole, electrostatic, van der Waals, hydrogen bonding, hydrophobic interactions, and so on. However, it is impossible to accommodate all the said interactions in a single molecular solvent. Water, despite its recognition as a universal solvent, offers only dipole-dipole, hydrogen bonding, and hydrophobic interactions for a solute. Almost all basic biological entities necessarily require a medium for their stabilization and functioning. Out of various basic biological candidates, we are, in particular, addressing the proteins and DNA in detail. As both proteins and DNA possess basic moieties, electrostatic, and hydrophobic centers, they require a medium that has all these interactions for their stabilization, functioning, long-term storage, and separation.
In quest of the “green” solvents, the past few decades have witnessed the upsurge of ionic liquids (ILs) as a suitable replacement of volatile organic solvents (VOCs) in various applications. Ionic liquids (IL) are typically liquid at room temperature and mainly consists of asymmetric organic cation and organic/ inorganic anion (Wilkes and Zaworotko, 1992). Ionic liquids are classified as protic ionic liquids (PILs) and aprotic ionic liquids (AILs), depending on the quaternization of base by proton (H+) or alkyl group (-R), respectively. These two ionic liquids differ a lot in terms of the activity and strength of the directional and non-directional forces between ions. Protic ionic liquids possess higher strength of Coulomb- and hydrogen bonding-interactions as compared to their aprotic counterparts and resembles closely to water, owing to their extensive network of hydrogen bonding (Fumino et al., 2009) Similar to PILs are Brønsted acidic ionic liquids, in which protons occupy either the cation or the anion (not necessarily on the cation as in protic ionic liquids), and therefore, they are categorized with the protic/aprotic ionic liquids. The reputation of ionic liquids in several fields is because of their attractive properties such as significantly low vapor pressures, high thermal stability, wide liquidus range, non-flammability, and recyclability (Welton, 1999; Weingärtner, 2008; Castner and Wishart, 2010; Hayes et al., 2015; Egorova et al., 2017). Their relevance in various chemical and biological processes is owing to their ability to interact through the variety of forces toward a solute. The physicochemical properties of ionic liquids depend on the coordination of asymmetric cation with a symmetric or asymmetric organic/inorganic anion (Rogers and Seddon, 2003). Depending on the charge density of anion, ionic liquids behaves as hydrophilic or hydrophobic in water and therefore can react with both polar and non-polar solutes. Besides, a long alkyl chain either on cation or on anion also imparts hydrophobic character to the ionic liquid. Ionic liquids in neat and in aqueous state affects the structure and stability of proteins and hence are being tried as a potential media in storage and crystallization. Despite the huge interests in ionic liquids, the molecular mechanism involved in protein-ionic liquid are not yet fully comprehended. The availability of different kinds of ionic liquids and various features of proteins make the generalization about the intermolecular interactions very difficult. Addition of water though alleviates the solubility problem and maintains the structure over a longer time period; however, it complicates the understanding of the intermolecular interactions between the ionic liquid and biomolecules as water also undergoes strong hydrogen bonding with both species. The physical parameters for the aqueous ionic liquids, however, cannot be accounted for by the screened forces of the ionic liquid toward the biomolecule. In this regard, different solvation models have been employed to derive the interaction parameters that explain the stabilizing/destabilizing behavior of aqueous ionic liquid systems toward biomolecules. The structure and stability of the DNA's double-helical structure also get affected in the dilute solution of an ionic liquid. Figure 1 and Table 1 shows the interactions between ionic liquids and protein and DNA molecules.
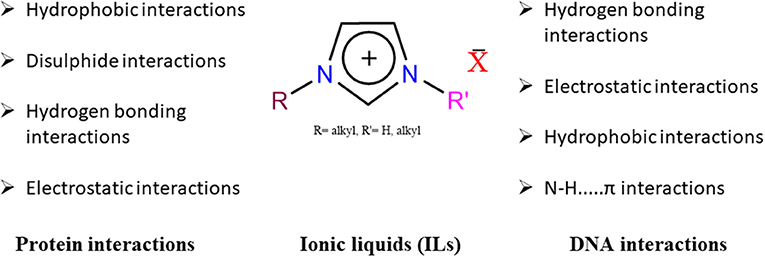
Figure 1. Interactions of a typical ionic liquids with proteins and DNA. Images in the backgroud are taken from the google.
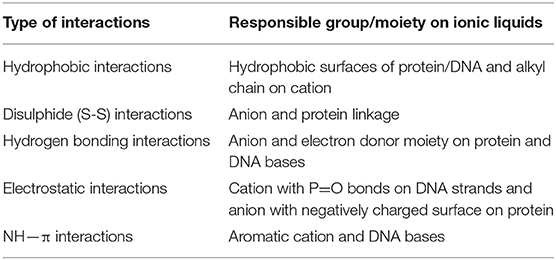
Table 1. Summary of interactions between ionic liquids and biomolecules and responsible functionality/moiety.
There are some excellent review articles available on the use of ionic liquids in the stabilization and functioning of protein and DNA. The review article by Schindl et al. thoroughly discusses the use of different classes of ionic liquids in the dissolution and processing of different types of proteins (Schindl et al., 2019). The review by Gough et al. presents a critical discussion on the use of ionic liquids as potential media for protein and polysaccharide biopolymers fibrillation by different fiber fabrication methods (Gough et al., 2020). The topical review by Smiatek presents detailed discussion about the role of ionic liquids as solvents in deciding the fate of protein to the native and denatured state by using a preferential binding model, where the strength of ions in influencing the protein dynamics is given in terms of the value of preferential binding coefficient (Smiatek, 2017). The role of water on the self-aggregation of ionic liquids is also discussed with recent experimental and theoretical findings in light of the binding coefficient values. Wakayama et al. presented a review based on various model parameters to construct the enzyme-derived catalyst in the production of important chemicals (Wakayama et al., 2019). The review by Saha and Mukherjee enlists the simulation results on the effect of water in altering the structural and dynamical properties of ionic liquids and biomolecules present in it (Saha and Mukherjee, 2018). Reslan and Kayser compiled all the experimental and theoretical results available on the ionic liquid-protein interactions and discussed the effect of toxicity and viscosity on it (Reslan and Kayser, 2018). Oprzeska-Zingrebe and Smiatek discussed the relative importance of the aqueous ionic liquids and standard co-solutes in stabilizing or destabilizing the protein and DNA structure (Oprzeska-Zingrebe and Smiatek, 2018). The extent of stabilization/destabilization by the ionic liquids are discussed in terms of the enthalpic and entropic contributions obtained from the Gibbs free energy change. The review by Schröder gives a detailed account of the various properties of ionic liquids that could affect the protein stability and crystallization in view of the various classical theories (Schröder, 2017). Contrary to the multiple reviews available on the behavior and functioning of protein in ionic liquid systems, information on DNA stabilization in ionic liquid systems is scarce. The review by Zhao though gives a good account of the use of ionic liquids on the DNA stabilization in terms of various specific and non-specific-interactions between them (Zhao, 2015).
In the current review, we aim to discuss the role of intermolecular interactions of ionic liquids on the structure and functioning of various proteins and DNA. These interactions are discussed in terms of the change of cation, anion, length of alkyl chain, and presence of functional group on them. A brief introduction of various theories/concepts in the protein stabilization by electrolytes solutions and their utility in case of ionic liquids is presented. Besides the variation of structural components, the influence of the reaction conditions such as pH, temperature, and effect of water on the intermolecular interactions are also described. The importance of ionic liquid-biomolecules interactions on, stability, solubility and separations are also covered. The negative impact of toxicity of ionic liquids on the storage and functioning of biomolecules and recyclability is also taken into the consideration.
Ion-Protein Interactions
We begin this section with a brief discussion about the solvent property such as polarity and hydrophobicity that have implications on the protein and DNA chemistry. Subsequently, various theories/concepts related to the ion-protein interactions like the Hofmeister series, Collins law of matching water affinity, Jones-Dole coefficient, and the influence of ionic strength will be discussed. The validity and deviation of Hofmeister series is also extended to the ionic liquids. At the end, specific role of water on the protein-ionic liquid interactions is discussed.
Nearly all proteins contain a lower or higher amount of sulfur (3–6%) in their structures that impart rigidity to the 3-D structure owing to the formation of disulfide (-S-S-) bonds (Simpson and Crawshaw, 2002). Besides, various inter- and intra-molecular hydrogen bonds in protein also contribute to the rigidity and hence posing a challenge in their processing. Therefore, a solvent/mixture whose components outshine the –S-S- and inter- and intra-molecular interactions are desired for protein processing. The use of molecular solvents in protein processing has lingered with various issues. They poses a risk of volatility, corrosiveness, environmental impact, recycling, and renewability (Idris et al., 2013). In addition to this, their inapplicability in multi-step processes and the degradation of proteins are the prime issues related to solvent mixtures. Due to these shortcomings, there has been a growing demand to develop a new solvent that can fulfill the entire solvent properties for protein processing. The solvation behavior among different classes of ionic liquids is not similar and can be assessed from the polarity parameters and hydrophobicity. We would discuss in detail the characteristics of ionic liquids such as polarity, hydrophobicity and potential of ion, that come-in-aid to stabilize the protein structure.
Polarity
The solvation capability of a solvent is best to describe in terms of a single polarity parameter that accounts for the entire specific- and non-specific-interactions (Reichardt, 2005). There are various scales of solvent polarity such as static dielectric constant (εr), Reichardt polarity scale (ET(30)), refractive index (η), and permanent dipole moment (μ) (Reichardt, 1994). Out of these, the static dielectric constant and Reichardt polarity scales are largely utilized to account for the “solvation effects/polarity.” The non-specific electrostatic interactions are measured by dielectric spectroscopy (Huang and Weingärtner, 2008). The protic ionic liquids possesses a higher dielectric constant whereas the polarity of aprotic ionic liquids is in the range of lower alcohols. In the case of ionic liquids, which possess charged ions, static conductivity σ (0) dominates in the dielectric constant. However, it is noted that the dielectric constant of ionic liquids is lower than that measured by the diffusion coefficient due to the interaction of ions itself rather than the neutral ion-pair. The mobility of ions poses a problem in the measurements of dielectric constant (Krüger et al., 2010).
The “Reichardt polarity scale” is devoid of any complications and determines all the specific, non-specific, π-π and dipole-dipole interactions of ionic liquids and molecular solvents (Reichardt, 2008). In this method, any change in the electronic transition due to the transfer of the Reichardt's dye from non-polar to polar medium is measured by UV-visible spectrophotometer and is represented as the electronic transition energy (ET(30)) measured in kcal.mol−1. Kamlet, Abboud, and Taft proposed the idea of a complementary polarity scale using a set of dyes that are known as polarity index (π*), hydrogen bond donor acidity (α), and hydrogen bond acceptor basicity (β) (Kamlet and Taft, 1976; Taft and Kamlet, 1976; Kamlet et al., 1977). α denotes the hydrogen bond donor capacity and is a characteristic of cation whereas the β accounts for the hydrogen bond acceptor basicity and depends on the anion. The strength of electrostatic interaction between ions of ionic liquid is determined in terms of the polarity index, π*. Protic ionic liquids possess higher α and lower β than their aprotic counterparts. The interaction of cations and anions of ionic liquids to the protein and DNA depends on the charge and acidity/basicity of the interacting sites on biomolecules.
Hydrophobicity
Hydrophobicity is a phenomenon in which a non-polar solute molecule tends to stay away from water (Zhao, 2006). It has a crucial role in protein solubilization and enzyme functioning. The low charge density on protein and enzymes makes them hydrophobic in water. A saturated 1-octanol-water mixture is used to measure the hydrophobicity of a solute in terms of the partition coefficient shown as log P, which denotes the partitioning of solute in 1-octanol as compared to water. Solvents with high log P are more hydrophobic compared to those with lower log P-values. Ionic liquids possess lower log P-values than short-chain alcohols. For example, 1-butyl-3-methylimidazolium with acetate, nitrate, and hexafluorophosphate anions have log P−2.8,−2.9, and−2.4, respectively than ethanol−0.24. Thus, ionic liquids are less hydrophobic or more hydrophilic than ethanol. The tethering of longer alkyl chain on cation or anion increases the log P (Yamamoto et al., 2011). Though hydrophobicity was found significant in enzyme catalysis, it inversely affects the solubility of the protein (Laane et al., 1987). In the case of proteins, hydrophobicity overshadows the basicity of anion or coulombic interactions (Kaar et al., 2003).
Effect of Ion on Protein Solubility: Hofmeister Series
Ion-induced effects are universal in chemistry and biology and emerged through the alteration in the hydrogen bonding network of water (Hofmeister, 1888). These effects were important in protein and enzyme stabilization and crystallization but the fundamental mechanism behind these effects were not well-understood (Collins, 2004; Kunz et al., 2004; Broering and Bommarius, 2005; Nostro et al., 2005). In 1888, Franz Hofmeister arranged several cations and anions into their capability of precipitating the egg white protein (Hofmeister, 1888). For a given cation, anions' coagulating power was in the order,
A reverse of the above ordering was noted operative in predicting the precipitating power of anions though not universally obeyed. Afterwards several experimental and theoretical reports claimed that protein stabilization in an electrolytic medium depends on the specific solute-ion interactions (Omta et al., 2003a,b; Zhang et al., 2005). Later, Gurney, Washbaugh and Collins, and Green explained the confusion over the validity of the Hofmeister series (Gurney, 1953; Collins, 2004, 2006).
Gurney classified salts as structure–maker (kosmotropic) and structure-breaker (chaotropic) depending on their ability to strongly- or weakly-interact with hydration layer of solute, respectively (Gurney, 1953). Collins and Washbaugh proposed the “law of matching water affinity” which states that a kosmotropic cation forms an ion-pair only with a kosmotropic anion and a chaotropic cation forms ion-pair only with a chaotropic anion (Collins, 2004). The ion-pairs of the mixed types do not exist. A perfect match between the hydration enthalpies of oppositely charged ions inhibits them to interact with water. A mismatch in the hydration enthalpy allows the ions to dissolve in water and make ion-induced effects visible. A kosmotrope (water structure-maker) offers strong interaction to water molecules beyond its hydration layer than a chaotrope (water structure-breaker; Collins, 2006). However, these concepts were soon disproved by spectroscopic and thermodynamic considerations (Omta et al., 2003a; Batchelor et al., 2004; Funkner et al., 2012). According to these evidences, a salt ion only affects its first hydration layer and has no effect on the bulk water as expected.
For a classical ion, the kosmotropic and chaotropic character correlates with the relative viscosity by the Equation (1),
where, η0 is the viscosity of solvent, η is the viscosity of salt solution at concentration c, A is the Falkenhagen coefficient that depends on the electrostatics of the system and are small and B is the Jones-Dole coefficient and represent the characteristic of ions (Collins, 1997). A kosmotropic ion interacts intensely with water layers, consequently increase the viscosity η and thus increase B, whereas the chaotropic ion due to their water-immiscible character possesses lower B. Except for some minor issues with anions, the Jones-Dole coefficient value is quite questionable for ionic liquid cations. The ionic liquid containing a longer alkyl chain on cation exhibits positive B values and hence considered as kosmotropic (more than eight carbon) (Zhao, 2006). The increase in the solution viscosity might be due to the hydrophobic hydration or because of the high viscosity of ionic liquids in water. Nevertheless, kosmotropic/chaotropic assignment of an ionic liquid cation is based on the B values, and their order in the Hofmeister series is different (Zhao, 2005, 2015; Yang, 2009). To avoid any complications, the Hofmeister series for anions used only for accounting the ion-induced effects.
Green noted that the protein solubility does not depend on the concentration of salt but also its ionic strength (I) in the solution (Green, 1932). For a solvent with protein solubility S0, which changes to S after addition of salt, depends on the ionic strength I by the Equation (2),
where, z1 and z2 are the valences of the salt ions and Ks is salt coefficient and depends on the characteristic of salts. As Ks depends on the volume of ion, chaotropic ions noted to decrease the protein solubility (Salis and Ninham, 2014). Based on these factors, it is evident that the Hofmeister series do not depend on the ion but also its concentration and ionic strength in the solution.
Ionic Liquid-Protein Interactions and Hofmeister Series
Similar to aqueous electrolytes, ionic liquids are constituted by oppositely charged ions held together by hydrogen-bonding and van der Waals interactions rather than strong electrostatic attractions as in case of electrolytes. The reduced charge density of ionic liquid ions weakens their interaction with the oppositely charged surfaces of proteins compared to the aqueous electrolytes. However, hydrophobic- and hydrogen bonding-interactions are noted to play anchoring role in the stabilizing protein structure. Hippel and Wong were the first to observe the Hofmeister ordering of tetraalkylammonium and guanidinium salts on the thermal stability of Ribonuclease A (RNase A), gelatin-collagen, DNA and precipitation of benzoic acid from aqueous state (Von Hippel and Wong, 1964). However, they did not overrule the possibility of the specific solute-ion interactions. Zhao et al. observed the Hofmeister ordering of ionic liquid ions during the hydrolysis of enantiomeric phenylalanine methyl ester catalyzed by Bacillus licheniformis protease in different aqueous ionic liquid solutions (Zhao et al., 2006). The kosmotropic anion and chaotropic cation were noted effective in enhancing the enzyme enantioselectivity. The decreasing order of anions: > citrate3−, CH3COO−, , CF3COO− > Br− > OTs−, and for cations: [emim]+ > [bmim]+ > [hmim]+.
An ionic liquid solution with high kosmotropicity (which is given in terms of the difference of Jones-Dole B-coefficient of anion and cation) resulted in high enzyme enantioselectivity (Zhao et al., 2006). Mazzini et al. investigated the role of water, methanol, propylene carbonate (PC), dimethyl sulfoxide (DMSO) and formamide on the Hofmeister ordering of CH3COO−, F−, Cl−, Br−, I−, , and SCN− in the Size Exclusion Chromatography (SEC) of electrolyte solutions on Sephadex® G-10 and the investigation of the conformation of a polymer brush in the presence of the different electrolytes by Quartz Crystal Microbalance with Dissipation (QCM-D) (Mazzini et al., 2018). The fundamental Hofmeister ordering of ions maintained in the methanol and water (CH3COO− > F− > Cl− > Br− > I− > > SCN−) whereas it reversed in case of DMSO and PC. There was no discrete ordering of ions in case of formamide (Mazzini et al., 2018). Constantinescu et al. measured the melting temperature (Tm) of RNase A in presence of major cations such as 1-alkyl-3-methyl imidazolium ([Rmim]+), 1-alkyl-1-methylpyrrolidinium ([Rmpyrr]+), tetraalkylammonium ([R4N]+) and guanidinium ([Guan]+) and anions thiocyanate ([SCN]−), methylsulfate [MeOSO3]−), ethylsulfate ([EtOSO3]−), trifluoromethanesulfonate ([TfO]−), bis(tri-fluoromethanesulfonyl)imide ([Tf2N]−), and dicyanimide ([N(CN)2]−) (Constantinescu et al., 2007). The cation series in terms of decreasing Tm with Br− and Cl− as common anion is as,
The thermal stabilization of RNase A by these cations was in the order of their hydrophobicity (Figure 2). The thermal stability data of lysozyme in presence of three imidazolium chloride-based ionic liquids fortifies this observation (Lange et al., 2005). The different ordering of structurally similar [emim]+ and [bmpyrr]+ ions was attributed to the charge delocalization in [emim]+. For a common cation [emim]+, the order of anions in terms of decreasing Tm values is as,
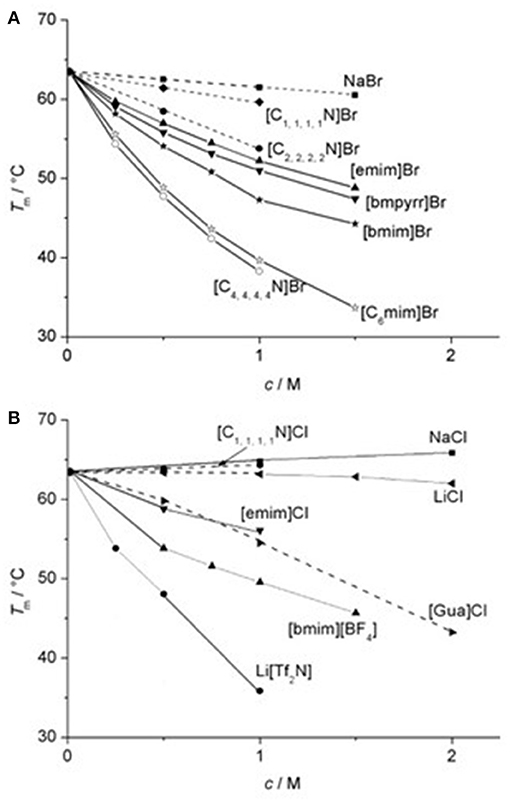
Figure 2. Transition temperatures Tm for the thermal denaturation of RNase A as a function of the concentration c of added ILs with (A) Br− and (B) Cl− as a common anion. Reprinted with permission from Constantinescu et al. (2007).
The series suggests that weak hydration and high hydrophobicity tends to decrease the Tm more (Figure 3). However, this should not be taken as a rule of thumb in arranging the anions on the basis of their effectiveness in stabilizing/destabilizing the RNase A since all these anions belong to different homologous series. Even for the chemically-related anions, the order of hydrophobicity is not valid as observed from their effect on Tm (Zhao, 2006). The reverse ordering at various places in the cation and anion series suggests that the simple hydration theory cannot be applied for the complex ions and thus, this assumption is not valid in case of ionic liquids. Zhao also noted that enzyme activity in aqueous ionic liquids seems to follow the Hofmeister series as ions remain separated but predicting their activity in absence of water is not a straightforward affair (Zhao, 2005). Lau et al. had similar observation upon testing the influence of pure ionic liquids on the activity of Candida antarctica Lipase B (CaL B) (Lau et al., 2004). They noticed that the concept of kosmotropicity/chaotropicity and Jones-Dole B-coefficient cannot be applied to account for the influence of ionic liquids over the activity of CaL B. Even for the similar [NTf2]− anion counter cation such as Li+ destabilizing behavior was noted for Cal B while a stabilizing behavior was evident with [emim]+ (de Diego et al., 2005; Constantinescu et al., 2007). These observations suggest that binding of ionic liquids to protein surface depends on the charge and hydrophobicity and thus indicates specific ion-protein interactions.
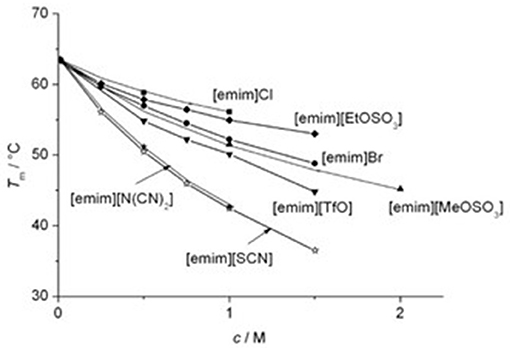
Figure 3. Transition temperature Tm for the thermal denaturation of RNase A as a function of the concentration c of added ILs with [emim]+ as a common cation. Reprinted with permission from Constantinescu et al. (2007).
Beyond Hofmeister: Specific Ion-Solute Interactions
The reports by Omta, Jungwirth, and Funkner on the protein interaction in aqueous electrolytes strongly suggested that the ions interaction toward the protein backbone is driven by specific ion-protein interactions and not necessarily due to the perturbation of the bound water to the protein (Omta et al., 2003a; Batchelor et al., 2004; Heyda et al., 2010; Algaer and van der Vegt, 2011; Funkner et al., 2012; Jungwirth and Cremer, 2014). These observations were confirmed as valid in several studies utilizing ionic liquids. Kumar et al. compared the stabilizing power of SCN−, , Cl−, Br−, I−, and CH3COO− for ionic liquids with 1-butyl-3-methylimidaolium ([bmim]+) cation and for inorganic salt with sodium ion on α-chymotrypsin (CT) (Kumar et al., 2014). The comparison showed that the SCN−, , Cl−, Br−, I−, and CH3COO− of sodium salt have a destabilizing effect whereas the Cl−, Br−, and CH3COO− with [bmim]+ have a stabilizing effect on the CT. The arrangement of these anions in the stabilizing/destabilizing order of CT does not necessarily follow the Hofmeister ordering and depends a lot on the counter cation and nature of the protein in question. Similarly, the influence of ionic liquids on the stability of collagen and activity of laccase do not follow the Hofmeister ordering of anions and indicate specific anion-protein binding (Sun et al., 2017; Tarannum et al., 2019). Reid et al. investigated various theories predicting the behavior of ionic liquid in water by statistical thermodynamic. The structure of water-IL mixture was water dependent (Reid et al., 2015). At a lower concentration, water binds strongly with ion whereas at higher concentrations, water molecules aggregate themselves and interact less with ionic liquid. Kobayashi et al. noted similar behavior of water at the water-ionic liquid interface in their study of ionic liquid-water interaction study by molecular dynamics and sum frequency generation (SFG) spectroscopy, at different concentrations of water (Kobayashi et al., 2017, 2019). Bui-Le et al. used a set of analytical techniques such as circular dichroism (CD), fluorescence, UV-visible, NMR and small-angle X-ray scattering to probe the protein [green fluorescent protein (GFP)] interaction with imidazolium and pyrrolidinium as cation and chloride, acetate and triflate as complementary anions (Bui-Le et al., 2020). The site-specific protein-ionic liquid interactions through various analytical methods exhibited triflate as the stabilizing anion while to chloride and acetate as the destabilizing anions. Singh et al. studied the thermal unfolding of lysozyme in 1-ethyl-3-methylimidazolium ethylsulphate ([emim][EtSO4]) and 1-ethyl-3-methylimidazolium diethylphosphate ([emim][Et2PO4]) both experimentally and theoretically (Singh et al., 2020). The destabilizing power of [emim][EtSO4] and [emim][Et2PO4] was higher than that of a strong denaturant as observed from lowering in the Tm. Among ionic liquids, [emim][Et2PO4] was more destabilizing for lysozyme and required lower energy, as is evident from ΔH, than [emim][EtSO4]. The fluorescence study showed that both ionic liquids interact with the tryptophan residue of lysozyme. MD simulation revealed that cation interacts in a “local-manner” while anion in a “global-manner” due to the negative charge of the lysozyme. The [Et2PO4]− was found to have closer first coordination shell and stronger coulombic interaction with lysozyme than [EtSO4]−.
Besides the experimental work, several theoretical studies on the ionic liquid-protein interactions indicated a trend pointing toward the specific ion-solute interactions. Lesch et al. studied the influence of aqueous [emim][CH3COO] on the stability of β-hairpin peptide using atomistic MD simulation and Kirkwood-Buff theory. The simulation work suggested that the cation ([emim]+) binds with both folded and unfolded peptide but anion ([CH3COO]−) binds only with the unfolded peptide (Lesch et al., 2015). In an another work Diddens et al. compared the behavior of different anions ([BF4]−, [CH3COO]−, and Cl−) with the same cation on the ionic l iquid-protein using the atomistic MD simulation and Kirkwood-Buff theory (Diddens et al., 2017). The simulation outcome suggests that the larger anion interacts with protein surface more strongly, followed by dehydration, than the smaller anion and the interaction was enthalpic in nature. Jaegar and Pfaendtner studied the stability of human serum albumin (HSA) in 1-butyl-3-methylimidazolium tetrafluoroborate ([bmim][BF4]) and choline dihydrogenphosphate ([chol][dhp]) by MD simulations and enhanced sampling techniques (Jaeger and Pfaendtner, 2016). The RMSD and RMSF calculations indicated that at higher ionic liquid concentrations, the protein adopts structure similar to their crystallographic structure. The structure of HSA in 20% [chol][dhp] is similar to that in water and thus it is unlikely that [chol][dhp] destabilize the HSA in its pure state. Burney et al. investigated the modification of the enzyme surface charge for Candida rugosa lipase and Bos taurus α-chymotrypsin in aqueous 1-butyl-3-methylimidazolium chloride ([bmim]Cl) and 1-ethyl-3-methylimidazolium ethylsulphate ([emim][EtSO4]) using MD simulations (Burney et al., 2015). The calculated solvent charge density indicated that for both enzymes in ionic liquids changed their positively charge surface to the negatively charged one upon an increase in the solvent concentration near the enzyme surface. The radial distribution of ionic liquid components with respect to enzyme reveals the decreased interaction of anion with the modified surface and more to the cation. Ghanta et al. showed in their MD simulation on α-lactalbumin in aqueous [bmim][BF4] the conformational changes in the protein as well as the distribution of water and ionic liquid around it (Ghanta et al., 2020). The calculations revealed an enhanced rigidity of protein due to the rearrangement of protein-water hydrogen bond and the formation of protein-ionic liquid hydrogen bond. The formation of greater number of salt bridges in presence of ionic liquid also account for the enhanced rigidity. Jaegar et al., compared the aqueous ionic liquid ([bmim][CH3COO]) tolerance on cellulases from Trichoderma viride, Thermogata maritima, and Pyrococcus horikoshii at different temperatures and different concentrations of ionic liquid in water (Jaeger et al., 2014). The simulation results indicated dissimilar effects of the ionic liquid on enzyme deactivation. The most negatively charged enzyme was least destabilized and had similar behavior in water and binary mixtures of ionic liquids. A summary of interactions between ionic liquid moieties and protein and DNA surfaces are given in Table 2. Majority of research about the protein-ionic liquid interactions do not account the solvation properties of ionic liquids. A correlation between the solvation parameters of ionic liquids and their influence on the fate of protein can be of paramount value in designing the potential optimum ionic liquids. Tomlinson et al. studied the solubility of corn protein zein in aprotic ionic liquids, namely, 1-butyl-3-methylimidazolium acetate ([bmim][CH3COO]), 1-ethyl-3-methylimidazolium acetate ([emim][CH3COO]), and 1-butyl-3-methylimidazolium dicyanamide ([bmim][N(CN)2]) and protic ionic liquids containing 1-methylimidazolium ([Hmim]+) cation with [HSO4]−, [CH3COO]−, and [HCOO]− as anions (Tomlinson et al., 2014). Zein dissolved in all ionic liquids except [Hmim][HSO4]. While comparing the zein solubility among ionic liquids, [bmim][N(CN)2] exhibited the highest solubility owing to the denaturing action of dicyanamide anion. The other aprotic ionic liquids [emim][CH3COO] and [bmim][CH3COO] showed similar solubilization properties despite their different solvation properties. The importance of solvation properties [ET(30), α, β, and π*] is however more evident in protic ionic liquids. At 60°C, zein had a maximum solubility in [Hmim][CH3COO] (26.2 wt%) followed by [Hmim][HCOO] (12.3 wt%) whereas the lowest solubility was found in [Hmim][HSO4] (< 1 wt%). The decreasing order of zein solubility was in line with the increasing order of polarity [ET(30)], indicating that least polar protic ionic liquid are required for higher solubility. Single and multivariate regression using polarity parameters and molar volume on zein's solubility in ionic liquids suggested that a small molecular size and low ET(30), α, β, and π* are required to attain maximum solubility of zein in an ionic liquid. The higher solubility of zein in [bmim][CH3COO] than in [emim][CH3COO] is due to the lower π* for former than latter. The lower correlation coefficient for α suggests that acidity has lower impact on the zein's solubility. π* is noted as the important parameter and at higher temperature its highly responsible for the non-polar character to achieve higher solubility. Despite the effective correlation between zein's solubility and polarity parameters, more studies are needed before reaching any definite conclusions.
Influence of Water on Ionic Liquid-Protein Interactions
An aqueous solution of ionic liquids is used in the storage of biomolecules to alleviate the solubility problem and to maintain their structural features intact. Aqueous ionic liquids have different properties than neat ionic liquids but resemble closely to dilute electrolytes solution. The amphiphilic character of ionic liquids makes them heterogeneous, at the molecular level, that further increases with the size of alkyl chain on cation (Hayes et al., 2015; Bruce et al., 2017). Addition of water alters the heterogeneity of ionic liquids (Jiang et al., 2007). Blesic et al. showed that imidazolium chloride-based ionic liquids with alkyl chain greater than octyl undergoes self-aggregation whereas an ionic liquid with shorter chains (butyl to hexyl) do not show such behavior (Blesic et al., 2007). Liu et al., observed rod-like micelle in imidazolium-based ionic liquids whereas Vincent-Luna et al. noticed that critical micelle concentration required for micelle formation was lowered with an increase in the anisotropy in the imidazolium-based ionic liquids (Liu et al., 2015; Vicent-Luna et al., 2017). Cammarata et al. showed that water binds with ionic liquid anion in 1:2 ratio and the water content of ionic liquids increases with the basicity of anion (Cammarata et al., 2001). Simulation studies also indicate that water cluster size in ionic liquids are independent of the size of alkyl chain length on cation although the hydrophobicity of anion is crucial in deciding the miscibility with water (Méndez-Morales et al., 2011). The orientation of water molecules at the surface of hydrophilic and hydrophobic ionic liquids are different (Anthony et al., 2001; Rivera-Rubero and Baldelli, 2004). In hydrophilic ionic liquids, water molecules experience stable dipole-dipole and hydrogen bonding interactions and remain in solvated state whereas water molecules undergo surface orientation at gas-liquid interface in case of the hydrophobic ionic liquids (Anthony et al., 2001; Rivera-Rubero and Baldelli, 2004). Addition of water in ionic liquids also affects the dynamics of ions as observed in many studies (Araque et al., 2015; Sharma and Ghorai, 2016).
The consequence of water addition to the protein-ionic liquid interactions cannot be generalized based on its influence over the ionic liquids. In reality, both stabilizing and destabilizing effects of water on the protein-ionic liquid interactions were observed. Micaêlo and Soares study on a model protein in presence of [bmim][PF6] suggested that a higher fraction of water molecules resides in the solvation shell of protein and hence higher stability was observed compared to that in case of [bmim][NO3] (Micaêlo and Soares, 2008). Similarly, a decrease in the hydrodynamic radius of lysozyme was observed upon addition of 1-propyl-3-methylimidazolium bromide ([pmim]Br) in aqueous lysozyme (Ghosh et al., 2015). Conversely, higher activity of α-chymotrypsin was observed in [bmim][NTf2] compared to the organic solvents at low water concentration (Eckstein et al., 2002). These contradictory observations about the role of water on protein stability in presence of ionic liquids indicate that the fate of the protein depends on its direct interaction with ionic liquids and do not depend on the interaction of water on the ionic liquids as assumed in the simple hydration theory. However, addition of water alters the stabilizing/destabilizing role of ionic liquids toward proteins and enzymes. As noted by Constatinescu et al., a strong interaction between cation and anion in ionic liquids might reduce the destabilizing effect of cation or anion of ionic liquids (Constantinescu et al., 2010). The role of water in protein stabilization in presence of ionic liquid is also controversial. In some cases, higher concentrations of ionic liquids were needed for the long-term stability. However, a change in the tertiary structure of protein was also reported at higher concentrations of ionic liquids (Byrne et al., 2007; Bihari et al., 2010). Singh et al. showed that long-chain imidazolium-based ionic liquids stabilize the horse heart cytochrome c (h-cyt-c) for a long term and at very low concentrations (1mM) (Singh et al., 2018). However, the presence of water in ionic liquid-protein mixtures is shown to have a distinct effect on the structural features. Proteins with the radius of gyration below 20 Å interact with water and remain in solvated state whereas proteins having radius of gyration above 20 Å do not react with water and undergo protein-protein interactions and result in larger aggregates (Takekiyo et al., 2014). The review articles by Smiatek (2017) and Saha and Mukherjee (2018) present more evidence about the inconclusive role of water on the ionic liquid-protein interactions.
Implications of Ionic Liquid-Protein Interactions
This section accounts for various applications of ionic liquid-protein interactions in protein chemistry. Firstly, the effect of various features of ionic liquid systems on the protein solubility is discussed. Secondly, use of ionic liquids in protein separation is discussed by making use of the ionic liquids-based aqueous biphasic systems (ABSs) and electrophoretic processes. Lastly, protein stability in ionic liquids-based systems is also discussed by using enthalpy-entropy compensation, role of the Hofmeister series on stabilizing protein and thermodynamics of the Hofmeister effects.
Protein Solubility
Though protein is fairly soluble in ionic liquids, however, an aqueous mixture of ionic liquids is used for low cost and high solubility of biomolecules. A mixture of ionic liquid and water better interacts with the polar, non-polar, or amphiphilic surfaces of proteins because of the hydrophilic, hydrophobic, and amphiphilic characters than the neat ionic liquids. These interactions cause either the precipitation or stabilization of proteins. In ionic liquids, the anion remains in the hydrated state more than the cation and hence the concentration of cation at the surface of the protein remains higher as obtained by various simulation results (Haberler and Steinhauser, 2011; Haberler et al., 2011, 2012; Klähn et al., 2011a,b; Lesch et al., 2015). The cation tethered with a long alkyl chain exhibits amphiphilic character; the cation being polar and alkyl moiety acts as a non-polar region (Zhang and Cremer, 2006). Because of the non-polar character, the alkyl chain orients toward the non-polar surface of proteins and the cation remains at the polar surface (Lim et al., 2014). According to the “iceberg model,” water molecules surround the alkyl chain and consequently lower the entropy of water molecules, and result in the hydrophobic hydration (Zangi, 2010). The ionic liquid cation competes with the anion toward the polar surface of proteins. The negatively charged amino acid moiety such as glutamic- and aspartic-acid exclusively attract the cation but as water molecules also remain in the equilibrium toward the site, due to hydrogen bond formation, the extent of attraction remains lower (Haberler and Steinhauser, 2011; Klähn et al., 2011a). As compared to cation, high charge density anion strongly interacts with amino acid moiety per se histidine, arginine, and lysine by the coulombic interactions that decide the fate of protein in aqueous ionic liquid solutions (Seduraman et al., 2012). Overall, the ionic components interact stronger at the protein surface than water hence replacing the bound water from the protein surface and hence resulting in coagulation or crystallization of a protein (Zaks and Klibanov, 1988). On the contrary, hydrophobic molecules stabilize the protein surface owing to their tendency of not interacting with water (Laszlo and Compton, 2002; Dang et al., 2013).
Additionally, the ionic liquid concentration also affects the protein solubility as the ion interacts strongly with the surrounding water hydrogen-bonding network of protein. At moderate ion concentrations up to 1 mol.l−1, the ion breaks the hydrogen bonding network of protein and increases its solubility by the phenomenon called “salting-in” (Thomas and Elcock, 2007). The strong chaotropic anion promotes the salting-in while a reverse effect noticed with the kosmotropic ions referred to as “salting-out.” The energetics of salting-in might be entropic/enthalpic, but in the case of salting-out, it is purely enthalpic (Zangi et al., 2007). Later, several MD simulation results indicated that the salting-in and salting-out not rely on the ion concentration but also depends on the specific ion-protein interactions because of the non-homogenous charge distribution, hydrophobicity/hydrophilicity, and difference in the functional structures of the protein (Zangi, 2010; Schwierz et al., 2013). The Hofmeister ordering was intact in case of the negatively charged ions but completely reversed for cations (Schwierz et al., 2016).
The control of protein precipitation and subsequent crystallization by tuning the salt concentration in protein solution is necessary for the structure determination of protein by X-ray diffraction (Judge et al., 2009). Ionic liquids, because of large availability and recyclability considered as a better co-solvent, and initial results established them as an excellent crystallizing agent for protein. The other benefits using ionic liquids in protein crystallization were less polymorphism and improved tolerance to concomitant impurities in crystals. The method was used to obtain the highly pure crystals of protein at the required salt concentrations. In 1999, Garlitz et al. reported lysozyme crystallization using ethylammonium nitrate (Garlitz et al., 1999). Later, Judge et al. noticed that proteins such as lysozyme, catalase, myoglobin, trypsin, glucose, and isomerase grow bigger crystals with ionic liquids as co-solvents and provide better resolution in x-ray crystallography (Judge et al., 2009). Kowacz et al. observed a reduction in nucleation density and improved crystal quality at higher ionic liquid concentration (Muldoon et al., 2012). In a comparison, choline chloride was noted less efficient than imidazolium-based ionic liquids in protein crystallization. The efficacy of imidazolium-based ionic liquids in crystallization was noted to increase with the length of the alkyl chain upon cation. Green observed the importance of electrostatic interaction at low ion concentrations (Ajaj, 2010). The electrostatic force is first screened by the like-charged biomolecular region that helps in protein crystallization (Muldoon et al., 2012).
Protein Separations
The efficiency and viability of any biotechnological process are in its efficiency to downstream processing to maintain the purity and quality, as it costs 60–90% of the overall process (Kula et al., 1982). The metabolites and bioproducts separation depends on the delicate change in pH, temperature, osmotic pressure, ionic strength, and surface charges; therefore, the techniques/methods utilized for the separation must be reliable and compatible with the bioproducts (Banik et al., 2003; Neves et al., 2009). Conventional methods used for the bioproducts separation from biotechnological processes are cost-ineffective and result in poor yields (Silva and Franco, 2000). In pure ionic liquids, most proteins get dissolved but not homogeneously dispersed. Besides, they can also denature the protein. In place of pure ionic liquids, an aqueous-biphasic solution (ABS) of the ionic liquid has emerged as an effective tool in biomolecular separation. An aqueous solution of ionic liquid with a salt solution can be used to create phase separation between aqueous ionic liquid and aqueous salt solution and therefore allows easier separation of bioproducts than conventional methods. Although hydrophobic ionic liquids form a biphasic solution with water but their high cost and protein denaturation ability render them unsuitable for biomolecule separations.
Aqueous-Biphasic Solution (ABS)
An ABS is formed when two mutually immiscible but water-soluble polymer/polymer, polymer/salt, and salt/salt systems are employed together. For the first time, Gutowaski et al. exhibited that 1-butyl-3-methylimidazolium chloride ([bmim]Cl), a hydrophilic ionic liquid, undergoes aqueous biphasic separation (ABS formation) upon mixing with the concentrated solution of K3PO4, a water-structuring salt (Gutowski et al., 2003). The upper layer remains rich in ionic liquid and lower layer rich in K3PO4. These new ABSs were first supposed to be used in the recycling of hydrophilic ionic liquids and metathesis in the formation of new ionic liquids but were later used in the extraction and separation of biomolecules and transition metal elements. The first use of ionic liquid-based ABS was noted by He et al. where they coupled [bmim]Cl + K2HPO4 + water-based ABS with reversed-phase high-performance liquid chromatography (RP-HPLC) for concentration and analysis of testosterone and epitestosterone (He et al., 2005). Later, Coutinho et al. extensively studied the utilization of ionic liquid-based ternary ABSs in the separation of the amino acid L-tryptophan with the major emphasis on the role of structural features of ionic liquids. They observed that separation of L-tryptophan increases with the length of alkyl chain on the cation because of elevated hydrophobicity. Further, the cation hydrophilicity increases the biphasic separation whereas the tethering of polar group such as hydroxyl, benzylic, and allylic increases the interaction with water and hence decreases the phase separation (Gutowski et al., 2003; Neves et al., 2009). The role of the anion in biphasic separation and extraction of L-tryptophan was studied by Ventura et al. by employing 1-ethyl-3-methylimidazolium- ([emim]) and 1-butyl-3-methylimidazolium ([bmim])-based ionic liquids with chloride, bromide, acetate, hydrogensulfate, methanesulfonate, methylsulfate, ethylsulfate, trifluomethanesulfonate, trifluoroacetate, and dicyanamide as the anion (Ventura et al., 2009). The results indicated that the extraction ability of prepared ABSs depends inversely on the hydrogen bond donor basicity of anion with water. Dreyer et al. pointed out that extraction of protein in ionic liquid (AmmoengTM 110)-based two-phase ABS depends on the electrostatic interactions between the positively charged cation and charged surface of the protein (Dreyer et al., 2009). Rather than polymer-based ABS, where hydrophobicity is a deciding parameter, ionic liquid-based ABSs remain unaffected of the hydrophobicity or surface area. It was concluded that the protein separation using ionic liquid -based ABSs does not simply depend on the biphasic separation but, in reality, is a complex phenomenon. Pei et al. used aqueous K2HPO4 + [Cnmim]Br] (where n = 4, 6, and 8)-based ABSs for the extraction of bovine serum albumin (BSA), cytochrome c, trypsin, and γ-globulin at different temperatures and pH conditions to achieve 75–100% extraction efficiency (Pei et al., 2009). The extraction efficiency was noted to change with the temperature and alkyl chain length on the cation. A small change in the extraction efficiency was observed with the pH change for cytochrome c due to the change of isoelectric point and hence electrostatic interaction between cytochrome c and ionic liquid cation. The thermodynamic study confirmed that increased extraction efficiency with temperature is owing to the endothermic nature of the process in ABSs keeping in mind that the temperature change should be below the denaturation temperature of protein. The standard Gibbs free energy for the extraction process at a given temperature can be correlated by the van't Hoff Equation (3)
where, K is the partition coefficient of protein between two phases at temperature T (K) at gas constant R.
The standard enthalpy (ΔHo)- and standard entropy (ΔSo)- change associated with protein partitioning can be obtained by Equation (4)
The ΔHo and ΔSo can be obtained from slope and intercept by plotting .
The values of both enthalpy (ΔHo) and entropy (ΔSo) are positive but is negative. The TΔSo > ΔHo indicated that the separation process is entropically favored in studied ABSs. The UV-vis- and IR-spectra showed that the protein structure remains intact even after the extraction with ABSs.
Capillary Electrophoresis (CE) and Micro-Capillary Electrophoresis (μCE)
Apart from the use of biphasic solution in protein separation, a capillary electrophoretic method is also used in protein separation and detection. In this method, the ionic liquid is used as a running electrolyte solution that causes a reversal of surface charge on the column wall (Jiang et al., 2003). By employing 1-ethyl-3-methylimidazolium tetrafluoroborate ([emim][BF4]) as a dynamic coating, the absorption of the protein was reduced at the wall of column and paved way for the separation and detection of proteins such as lysozyme, cytochrome c, trypsinogen, and α-chymotrypsinogen A. The separation was observed to facilitate upon increasing the length of alkyl chain on cation. Li et al. used 3% (v/v) ionic liquid solution in capillary electrophoresis for the detection of model protein avidin (Li et al., 2007). Protein detection was bettered by increasing ionic liquid concentration. Corradini et al. in their study showed that flushing a solution of 1-alkyl-3-methylimidazolium-based ionic liquid into a bare-fused silica capillary produces a non-covalent layer on the inner surface of coating that affects the untoward interaction of the protein with the capillary wall even if ionic liquid not present with the electrolyte (BGE) used for separation (Corradini et al., 2009). This effect was attributed to the adsorption of ionic liquid on the silica wall, which subsequently reduces the electrophoretic behavior of basic proteins up to different extent depending on ionic liquid. Xu and Wang published a detailed account on the use of ionic liquids in the improvement of capillary and microchip electrophoresis for the separation and detection of analytes such as phenols and aromatic acids, metal ions, medicines, enantiomers, biological materials, etc. (Xu and Wang, 2009).
Protein Stability
Protein is a heterogeneous class of biomolecule whose stability depends on the preservation of its functional secondary structure, which is α-helical, β-sheet and coil region. The functional structure of protein is a complex phenomenon and depends on the hydrogen bonding, disulfide (S-S) bond, hydrophobic, and intramolecular interactions. To maintain its functionality, a protein requires a specific solvent, pH, co-solvent and temperature. Any change in these conditions alters the functionality of the secondary structure of the protein. Apart from these conditions, viscosity of solvent also affects the dynamics of solvent and protein (van Rantwijk and Sheldon, 2007). At higher viscosity, the diffusion of protein is slow with the insignificant change in the catalytic ability. The hydrolysis of some [PF6]- and [BF4]-based ionic liquids form HF and alters the protein functionality (van Rantwijk and Sheldon, 2007). The enthalpy-entropy compensation and the relevance of the Hofmeister series of ions on protein stabilization is discussed below.
Enthalpy-Entropy Compensation
The marginal/low stability of protein is represented in terms of Gibb's free energy of unfolding (ΔGu) using enthalpic (ΔHu) and entropic (ΔSu) contributions (Equation 5).
The ΔGu accounts for the thermodynamic stability of a protein from native to the unfolded state and should be positive for a stable conformation (Lumry and Rajender, 1970). However, a low ΔGu indicates that protein stabilization largely depends on the higher enthalpic and entropic contributions that are also called “enthalpy-entropy” compensation, whose value can be manipulated by the addition of co-solute in the system (Senske et al., 2014). In general, the co-solute which can be excluded from the protein structure favors folded state while those binds to the protein surface shift the equilibrium toward the unfolded state of the protein. Macromolecular crowders, like polyethylene glycol and dextran, are noted to be excluded co-solute. They protect protein either due to the entropic excluded volume effect or enthalpic effects (Zhou et al., 2008; Sapir and Harries, 2015). Urea and guanidinium salts are chemical denaturant and destabilize the proteins by direct interactions that reduce ΔHu (Benton et al., 2012; Senske et al., 2014).
Similar to the inorganic/organic materials, ionic liquids may also cause a subtle change between the ΔHu and ΔSu when used as a co-solute and hence shift the equilibrium between the folded to unfolded states. For example, choline dihydrogen phosphate stabilizes cytochrome c and lysozymes for months (Fujita et al., 2006, 2007; Vrikkis et al., 2009). The stabilization mechanism involves the protection of the hydrophobic part of protein by the cation of ionic liquids (Summers and Flowers, 2000; Kumar and Venkatesu, 2013, 2014).
Apart from stabilization, ionic liquids have also been employed in accelerating the protein activity. The mechanism may involve either the chemical modification or stabilization or immobilization of the protein surface for chemical reactions (Zhao, 2006; van Rantwijk and Sheldon, 2007). However, the activity of lipase-catalyzed transesterification of methyl methacrylate and the polytransesterification of divinyl adipate and 1,4-butanediol in hydrophilic ionic liquids exhibited no reaction despite the high activity of the enzyme in 1-butyl-3-methylimidazolium hexafluorophosphate ([bmim][PF6]) (Kaar et al., 2003). The ineffectiveness of lipase is tested by adsorption, PEG-modification, and covalent immobilization in polyurethane foams in the hydrophilic ionic liquids.
Protein Stability and Hofmeister Series
Based on the change in the melting temperature (Tm) of ribonuclease A (RNase A) in various ionic liquids and inorganic salts, Weingärtner observed the trend of ions similar to the Hofmeister series (Weingärtner et al., 2012). The series shows that both the stabilizing and destabilizing effects to the native structure of protein depends on the choice of ions and hence to the extent of intermolecular interactions between them (Constantinescu et al., 2007, 2010).
where, chol+ and gua+ are acronyms for choline and guanidinium, respectively.
The above ordering of ions remains valid at the dilute concentration of inorganic salts and ionic liquids; whereas, a higher concentration is required during actual processing that may lead an interchange of the ion position due to the secondary interactions at elevated concentrations. For example, [Chol]Cl has a denaturing effect both for lysozyme and α-lactalbumin only at a lower concentration as exhibited by the dip in their Tm. The nature of [Chol]Cl toward lysozyme and α-lactalbumin start reversing at a higher concentration as suggested by their increasing Tm (Ajaj, 2010).
Klähn et al. studied the solvation stability of enzyme Candida Antarctica lipase B (CAL-B) in eight different ionic liquids and compared the results with that in water (Klähn et al., 2011a). The calculated solvation enthalpy indicated stronger interaction and hence lower solubility of the enzyme in ionic liquids than water. The lower solvation of CAL-B in ionic liquids is owing to the higher cavitation energy that further increases with IL-CAL-B interactions and subsequently denature the enzyme. The stronger interactions between the cation and anion lead to lower surface charge reduction from the surface of CAL-B as indicated by larger electrostatic potential values. Furthermore, charge density was more toward the polar surface of enzyme whereas the non-polar alkyl remains oriented toward the non-polar segment. During the investigation of CAL-B unfolding at a higher temperature in similar ionic liquids, Klähn et al. noted that hydrophobic ionic liquid causes higher stabilization of CAL-B than the hydrophilic ionic liquids (Klähn et al., 2011a). The interaction of CAL-B with ionic liquids was mainly at the polar surface and the non-polar core. The high polarizability of anion increases the extent of hydrogen bonding with the protein surface and destabilizes the CAL-B whereas the hydrophobic alkyl chain interacts with the CAL-B core. In the case of most hydrophobic cation acyclicbutylpentamethylguanidinium ([BAGUA]+), the protein core destabilizes due to the conformation change in the CAL-B. This exposes the protein core to the ionic liquids and stabilizes the unfolded protein. However, these observations could not be observed in the case of other hydrophobic ionic liquids due to the low solubility and weaker dispersion of protein. Fujita et al. reported the stabilizing role of biocompatible ionic liquids in solubilizing and stabilizing the cytochrome c (Fujita et al., 2005). As suggested by the study, the stabilizing role of biocompatible ionic liquids was bound to the dihydrogen phosphate (dhP). The addition of excess water has a negative destabilizing effect as revealed by the DSC spectra. An interesting, unfolding/refolding equilibria for lysozyme was noticed in a sugar solution of ethylammonium nitrate, EAN ([][]) with 97% refolding success by Byrne et al. (2007).
The experimental and theoretical studies indicated the dominance of anion in controlling the unfolding/folding equilibria (Haberler and Steinhauser, 2011; Haberler et al., 2011). The pronouncing role of the anion is attributed to the high polarizability that enables them to undergo coulombic and hydrogen bonding interactions at the protein surface also confirmed by the longer residence time (Haberler, M., and Steinhauser). Despite being the more influential in protein unfolding, anion approach to the positively charged surface of the protein in the form of ion-pair. The repulsive interaction between the surface and cation is compensated by the strong Coulomb interaction between the ions (Klähn et al., 2011b). The unfolding of the hen egg-white lysozyme (HEWL) in a dilute solution of EAN represents one such case where despite a strong coulomb interaction nearly 75% activity of HEWL was restored. The EAN was effective in high doses (1.6 mg/ml) compared to other renaturing agents (e.g., urea and guanidinium chloride) and the refolding efficiency increase up to 90% upon dilution (Summers and Flowers, 2000). The strong efficiency of EAN as a renaturating agent is because of its ability to extend hydrogen bonding network to the active sites similar to water.
Thermodynamics of the Hofmeister Series
Protein stability largely depends on the concentration of the ionic liquid. At lower ionic liquid concentration, say cIL < 0.5 M, all ionic liquids behave as a denaturing agent for proteins. At cIL > 1 M, ion-specific effects become dominant and follow Hofmeister ordering in protein stabilization (Senske et al., 2016). This shift in the behavior of ionic liquids is different than the inorganic salts mainly because of the diffused charges on ions. The folding/refolding behavior is reported as the change in melting temperature (ΔTm) upon transferring the protein from an ionic liquid solution to an ionic liquid-free buffer solution. At lower concentration, cIL < 0.5 M, denaturation starts and results in negative (ΔTm). As the concentration increases beyond 1 M, renaturation starts in protein that brings a positive change in the ΔTm values. However, a positive ΔTm for [Chol][dhp] was observed even at 0.25 and 0.5 M. Tm is defined as the midpoint of the unfolding transition, where ΔGu = 0. The cosolute-induced changes relative to the cosolute-free, buffered solution can be expressed as the excess functions (Equation 6),
where, ΔΔGu is the excess Gibbs free energy and ΔΔHu and TΔΔSu are the enthalpic and entropic contributions, respectively, that provide intriguing thermodynamic fingerprinting of the molecular mechanism involving a solute. Both ΔΔHu and TΔΔSu are cosolute-dependent and favor either the direct or reverse Hofmeister ordering for ions. For the favorable unfolding at cIL > 1 M, the Gu > 0. This trend in ΔΔGu is parallel to that of ΔTm during unfolding of RNase A. An enthalpy-entropy compensation between ΔΔHu and TΔΔSu is observed in case of analyzing the role of cosolute on protein unfolding. Based on the sign of excess function ΔΔGu, ΔΔHu and TΔΔSu eight possibilities are there as shown in Figure 4. According to Figure 4, hydrophobic ionic liquids (alkyl chain length from 4 to 6 and more carbons) have more positive TΔΔSu than ΔΔHu leading to enthalpic stabilization and entropic destabilization. For hydrophilic salts, both TΔΔSu and ΔΔHu were negative and the classification of ionic liquids into the stabilizing and destabilizing salts was depending on the magnitude of the relative values of these values. As the magnitude of thermodynamic parameters of cosolute-protein interactions depends on the pH, isoelectric point, charge on the protein and hydrophobicity, the arrangements of ionic liquid ions into the direct or reverse Hofmeister ordering is inconclusive. The increasing hydrophobicity of the cosolute promotes both the stabilizing enthalpic contribution and the destabilizing entropic contribution.
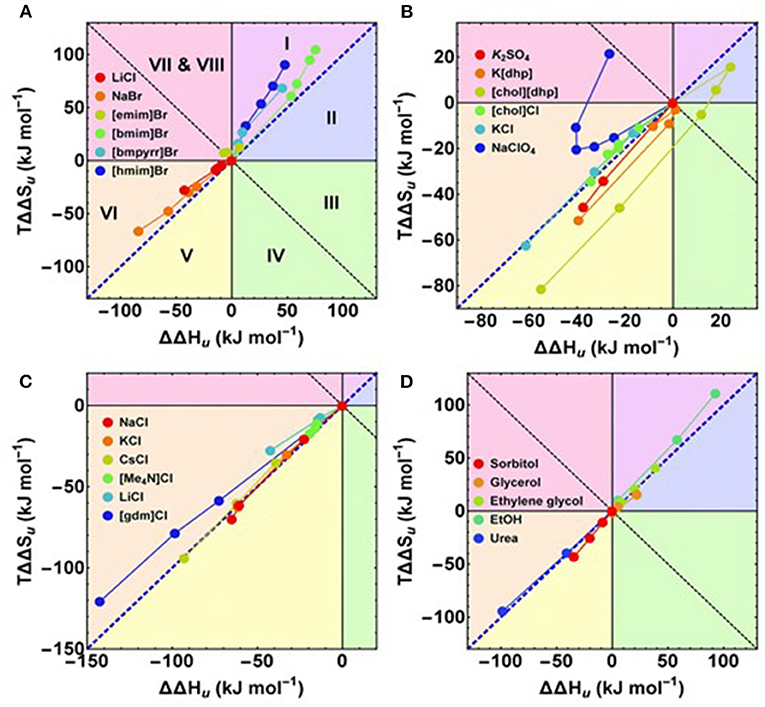
Figure 4. (A–D) Enthalpy–entropy compensation plots. The different segments correspond to different contributions of ΔΔGu, ΔΔHu, and TΔΔSu. The blue diagonal corresponds to a complete enthalpy–entropy compensation. Data points correspond to different concentrations of the respective cosolute. (A,C,D) The first or the first two data points (≤ 0.5 M) of some compounds are omitted for clarity. Adopted from Senske et al. (2016).
Ionic Liquid-Deoxyribonucleic Acid (DNA) Interaction
Deoxyribonucleic acid (DNA) is a negatively charged, naturally occurring double-helical structure made up of phosphate groups, sugars, and nitrogenous bases. The DNA forms duplex via base-pairing in the cells and carries genetic information (Saenger, 1984). In the double-helical structure, nitrogenous bases project into the helix and are responsible for stabilizing the structure through a hydrogen bond, stacking- and charge-charge-interactions. The nucleotide base pairs in DNA are adenine-thymine (A-T) and cytosine-guanine (C-G). The A-T base pair stabilizes the double-helical structure of DNA less efficiently than the G-C base pair owing to the two hydrogen bonds in former than three in later (Yakovchuk et al., 2006). The two strands of DNA connects through phosphodiester bonds (Sinden, 1994). Owing to the phosphodiester bonds, sugars remain oriented in the same plane in the nucleotides and add more stability to the double-helical structure because of more van der Waals interactions. The nitrogenous bases exert a hydrophobic effect on the DNA structure and remain toward the cavity of helix while the phosphate and sugar groups are hydrophilic and orient toward the exterior side and interacts with water. The negatively charged phosphate groups are stabilized by the positively charged moieties for maximum stability. The phosphodiester bonds further add up to the strength of the electrostatic interactions by bringing the bases in close vicinity (Hunter, 1993). Apart from the number of A-T base pairs in the DNA, a base-pair mismatch during replication also affects the stability of DNA double helix. It has been observed that a T-C mismatch destabilizes the duplex by up to 5.8 kcal/mol. If a hydrogen bond stabilizes the double helix by 1.5 kcal/mol, the destabilization due to base-pair mismatch is way more than the stabilization and might cause structural distortion to the helix (Kool et al., 2000).
Depending on the choice of the solvent, nucleic acids [DNA and ribonucleic acid (RNA)] exist in three forms viz. A-, B-, and Z-form. RNA exists exclusively in the A-form while DNA in the B-form. However, under dehydrating conditions DNA exist in the A-form (Tateishi-Karimata and Sugimoto, 2014). The Z-form of DNA is highly unfavorable and noted only upon unfavorable base sequencing, under the high salt concentration, or the influence of a cation (Pan et al., 2014). Although the B-form of DNA is stable in water but owing to its large dependency on the electrostatic interactions; it undergoes denaturation upon changing the pH, temperature and ionic strength (Lerman, 1964; McFail-Isom and Sines, 1999; Bonner and Klibanov, 2000). Besides, co-solutes and osmolytes can also affect the stability of the DNA structure. The concentration of sodium and chloride ions attached to the DNA directly affects its stability as shown by the molecular dynamic simulation (Feig and Pettitt, 1999). The interaction of co-solutes with the unfolded DNA strands is used as a quantitative probe to reveal important information about the changes in structural conformation (Sasaki et al., 2007). In drug delivery application, drug molecules are used to perturb the DNA structure (Neidle et al., 1987). The stability of DNA is reflected in its melting temperature, Tm. In the coming sections, we will discuss the effect of various ionic liquid systems on the stability, and extraction and purification of DNA.
DNA Melting
The stability of DNA helix reflects in its melting temperature, Tm. Because of the dependency of Tm on the polymerase chain reaction (PCR), any change in the double-helical structure during DNA hybridization can be accounted in terms of the Tm (Wittwer, 2009). The stability of the DNA double-helical structure upon rising the temperature changes depending on the mismatch in the DNA strands during hybridization and the strength of the buffer solution (Rouzina and Bloomfield, 2001; Gudnason et al., 2007). The Tm method can be used to account for the effect of solution conditions such as buffers, pH, hydrophobicity, solutes, analytes, or even surfactants. High salt concentration and high molecular weight polyethylene glycol were observed to stabilize the double-helical structure of DNA and, consequently, result in higher Tm (Khimji et al., 2013a). Cationic surfactants have been noticed to stabilize the DNA strands and therefore reduces the Tm (Bhattacharya and Mandal, 1997).
The excluded volume of a solvent is another factor that affects the DNA Tm. The excluded volume is the free space of a solvent that can be occupied by another molecule. The salts containing polyanion possess large excluded volume than alkali halide salts viz. NaCl, NaBr, etc. Upon denaturation, DNA strands require a larger volume to expand than the duplex. In the presence of polyanions, which possess large excluded volume, the expansion of DNA duplex into the single stranded DNA gets thermodynamically less favored due to overcrowding and consequently, an increase in Tm is noticed (Khimji et al., 2013a).
DNA Stability in Ionic Liquid Systems
The effect of ionic liquids on the structure of DNA and its subsequent stability has been a subject of major interest among researchers. Circular dichroism (CD) is normally used to assess any change in the DNA structure. An ionic liquid stabilizes DNA mainly through electrostatic bonding between the negatively charged phosphate group and the cation of the ionic liquid. At a higher concentration of ionic liquid, cation binds to several sites including phosphate and results in the destabilization of the structure. However, an adequate blend of specific and electrostatic interactions in ionic liquids has been noted to stabilize the structure more efficiently.
DNA Stability in Neat Ionic Liquids
The CD spectrum of DNA duplexes from salmon testes in choline dihydrogenphosphate ([ChCl][dhp]) exhibited only B-form of DNA. Besides, both quadruplexes (G-quadruplex and i-motifs) of DNA where noted stable in [ChCl][dhp], similar to water (Fujita and Ohno, 2010; Vijayaraghavan et al., 2010; Tateishi-Karimata et al., 2015). Thermodynamic parameters determination indicated abnormally higher stability of A-T base pairs than G-C base pairs (Watson-Crick base pairs) in 4 M [ChCl][dhp] solution compared to that in buffered NaCl solution due to the differences in the enthalpy contributions (Tateishi-Karimata and Sugimoto, 2012). Molecular dynamics (MD) and NMR results revealed choline binding to the minor groove of A-T base pairs in B-form of DNA and stabilize it via hydrogen bonding (Nakano et al., 2014a; Marusic et al., 2015). In the case of G-C base pairs, choline undergoes for specific binding with the guanine in the single strand of DNA that further inhibits the duplex formation via base pairing (Tateishi-Karimata and Sugimoto, 2012). This unusual behavior of ionic liquid solution toward A-T base pairs was more investigated further by experimental in vitro and MD in silico studies. In this succession, Chandran et al. observed that apart from the electrostatic interactions, groove binding of ionic liquid cation through hydrophobic and polar interactions significantly add up to the stability of DNA duplex (Chandran et al., 2012). The intrusion of DNA's minor groove by ionic liquid cation was further confirmed by the fluorescent intercalation dye replacement experiment. Portella et al. showed using MD simulations that G-C base pairs possess preferably higher solvation energy than the A-T base pairs in an ionic liquid solution and solvation differences greatly affect the DNA stability (Portella et al., 2014). It is concluded that the specific behavior of ionic liquid solution toward the DNA arises from the groove binding. The interaction of DNA with ionic liquid components are shown in Figure 1 and summarized in Tables 1, 2.
Zhang et al. showed that poly[3-butyl-1-vinylimidazolium L-proline salt] could condense plasmid DNA to form stable complexes against enzymatic degradation by deoxyribonuclease l (Zhang et al., 2009). Chen et al. studied the solubility and chain conformation of DNA in 1-allyl-3-methylimidazolium chloride ([Amim]Cl) and 1-butyl-3-methylimidazolium formate ([bmim][HCOO]) using laser light scattering (Chen et al., 2011). The DNA chain was observed in random conformation in [Amim]Cl and denatured and condensed in [bmim][HCOO]. Cardoso and Micaelo investigated the molecular solvation of single-stranded DNA (ssDNA) and double-stranded DNA (dsDNA) in detail in a variety of ionic liquids comprised of imidazolium, oxazolium, pyrrolidinium, pyrimidinium, quaternary ammonium, and choline as cation and tetrafluoroborate ([BF4]) and hexafluorophosphate ([PF6]) as counterpart anion (Cardoso and Micaelo, 2011). The modeling and MD simulation studies exhibited that dsDNA retains its B-form in all ionic liquids as shown by crystallographic data. The most stabilizing ionic liquids toward the dsDNA were based on choline and pyridine as cation and [PF6] as a binding anion. The [BF4] anion was observed to undergo stronger hydrogen bond formation than the [PF6]. The ssDNA was more accessible toward the hydrogen bonding via fluorinated anions. The cation of ionic liquid was located close to the DNA main chain owing to the electrostatic interactions with a phosphate group and hydrogen bonding and edge-to-face NH—π interaction with the bases, while anion forms hydrogen bonding with the cytosine, adenine and guanine bases. Mukesh et al. reported high solubility (3.5%) and long-term stability (up to 6 months) of B-form of DNA, obtained from salmon testes, in bio-based ionic liquid choline indole-3-acetate (Mukesh et al., 2013). Singh et al. reported long term storage capability (1 year) and high solubility (25 w/w%) of salmon tests DNA in 2-hydroxyethylammonium formate (2-HEAF) at ambient temperature (Singh et al., 2017). ITC results indicated the hydrogen bonding between DNA and 2-HEAF responsible for the high concentration solubility and extended stability. The DNA docking analysis exhibited a higher preference for minor-groove binding over DNA surface with 2-HEAF than the major-groove binding and thus confirming the importance of hydrogen bonding in stabilizing DNA. Sarkar and group investigated the role of hydrogen bonding in the DNA stabilization by employing ionic liquids, namely, 1,1,3,3-tetramethylguanidinium acetate (TMG) and 2,2-diethyl-1,1,3,3-tetramethylguanidinium acetate (DETMG), in which former has a hydrogen bonding N-H moiety whereas the latter has a different mode of bonding (Sarkar et al., 2020). MD simulation and spectroscopic results indicated that only the groove binding of ionic liquid to the DNA is not sufficient for stabilization of the structure. The TMG cation stabilized the Watson-Crick pair more efficiently than larger DETMG cation owing to the hydrogen bonding interaction differences with DNA grooves.
DNA Stability in Aqueous Ionic Liquids
As hydration itself has a major impact on DNA stability, ionic liquid as co-solvent in water has been explored as suitable media in DNA stabilization and storage. Wang et al. observed that [bmim][PF6] effectively extracts the salmon tests DNA from aqueous solution; in which [bmim]+ cation displace the Na+ from the phosphate group (Wang et al., 2007). In a further study, they noted that [bmim][PF6] replaces the ethidium bromide from the dsDNA as fortified by the lowering in the signals of the resonance light scattering (RLS) (Cheng et al., 2007). Khimji et al. contrarily observed the inefficiency of the hydrophobic ionic liquids [bmim][PF6] and 1-hexyl-3-methylimidazolium hexafluorophosphate ([hmim][PF6]) in extracting DNA from aqueous solutions despite their efficacy in extracting DNA staining dyes (Khimji et al., 2013b). Guo et al. examined the molecular mechanism and binding characteristics of DNA and [bmim]Cl in the aqueous medium (Ding et al., 2010). They reported a lowering in the critical aggregation concentration of [bmim]Cl in presence of DNA and a reduction in the fluorescence quenching of ethidium bromide upon addition of [bmim]Cl in DNA suggesting a competitive interaction between the dye, [bmim]Cl and DNA. Based on the experimental and quantum chemical results they proposed a DNA-ionic liquid interaction mechanism. At a concentration of [bmim]Cl below 0.06 M, the cationic head group locates at several Å of DNA phosphate with the alkyl chain lying parallel to the DNA surface, whereas, at higher concentrations (>0.06 M), the cationic head group situates nearer to the phosphate while butyl chain attached perpendicularly to the DNA surface. He et al. extensively studied the interaction between the 1-dodecyl-3-methylimidazolium bromide ([ddmim]Br) and DNA in dilute brine using isothermal titration calorimetry (ITC), micropolarity, dynamic light scattering, UV–Vis transmittance, atomic force microscopy (AFM), circular dichroism (CD) and molecular dynamics simulation (He et al., 2013). They observed strong electrostatic interaction between DNA and ionic liquid cation and hydrophobic interaction between the [ddmim]Br alkyl chains. Very high thermal stability (up to 100°C) and long-term storage stability (up to 6 months) observed for salmon tests DNA in [choline][lactate], [choline][H2PO4] (containing 20–50 wt% water) and [choline] [nitrate] (containing 20% water), as defined by CD and fluorescence spectra as well as gel electrophoresis.
Tateishi-Karimata et al. noted that DNA triplexes that are difficult to achieve in aqueous solution at neutral pH, can be stabilized in [ChCl][dhp] solution at neutral pH (Tateishi-Karimata et al., 2014). Surprisingly, the Hoogsteen base pairs and Watson-Crick base pairs exhibited similar stability in hydrated ionic liquid. MD simulation results of DNA triplex in [ChCl][dhp] by Nakano et al. indicated the groove binding of choline to the third strand of DNA (Nakano et al., 2014b). Stable G-quadruplexes were shown to occur in [ChCl][dhp] with choline location at the center of the quadruplex (Fujita and Ohno, 2012). Thermodynamic calculations coupled with MD simulation results showed higher stability of i-motifs than G-quadruplex in [ChCl][dhp] owing to the binding of the choline to the loops and grooves of i-motifs (Tateishi-Karimata et al., 2015). A detailed account of various studies involving ionic liquids and their influences on the DNA structure stability is given by Zhao (2015) and Tateishi-Karimata and Sugimoto (2018) groups.
Extraction and Purification of DNA
The extraction, purification and storage of DNA are required for the biological experiments. The quantification of transcript and protein level of target genes Hyde and Read, 1993 is essential for understanding gene regulatory mechanisms and drug design that controls gene expression. The current liquid-liquid extraction methodology for the separation, purification and storage of nucleic acids involves highly toxic reagents such as phenol and chloroform; therefore, a simpler method involving an environmentally-benign solvent is highly sought (Tan and Yiap, 2009).
DNA is not stable in an aqueous medium at room temperature for a long period owing to the degradation by contaminating nucleases and inherent stability (Sasaki et al., 2007; Armand et al., 2009). Additionally, the lower vapor pressure of the water makes them unsuitable in the small scale operational methods as it quickly vaporizes; therefore, solvents that alleviate the problem of aqueous buffers are desirable for the development of functional devices (Armand et al., 2009). Because of the vaporless characteristic even at zero pressure, the extensive network of hydrogen bonding akin to water and coulombic nature, ionic liquids emerged as the most suitable alternative compared to the toxic organic solvents (Earle and Seddon, 2000). The solvation capability for both polar and apolar moieties and ease of tailoring makes ionic liquid a smarter solvent from the DNA-ionic liquid interaction perspective.
Shi et al. proposed a bicyclic imidazolium-based ionic liquid [b-4C-im][Br] for promoting the PCR signal of G-C rich DNA by minimizing non-specific amplifications (Shi et al., 2012). The ionic liquid also facilitated the PCR of normal-GC DNA under mild conditions because of the destabilization of DNA duplexes under mild conditions. Anderson group reported the efficiency of hydrophobic magnetic ionic liquids (MILs) in the extraction of long and short plasmid DNA from bacterial cell lysate and salmon tests (Clark et al., 2015a). Benzyltrioctylammoniumbromotrichloroferrate(III) ([(C8)3BnN+]-[FeCl3Br−]) MIL was efficient in selective extraction of smaller single-stranded and double-stranded DNA whereas the dicationic 1,12-di(3-hexadecylbenzimidazolium)dodecane bis[(trifluoromethyl)-sulfonyl]imide bromotrichloroferrate(III) ([(C16BnIM)2][, FeCl3Br−]) MIL were excellent in extraction for the higher DNA molecules. In another report, MIL with metal-containing cations (Ni2+, Mn2+, or Co2+) and chloride anion were applied for in situ dispersive liquid–liquid microextractions (DLLME) for the extraction of long and short double-stranded DNA (Bowers et al., 2019). These hydrophilic MILs were further converted to hydrophobic MILs during the extraction by exchanging the chloride anion with that of bis[(trifluoromethyl)sulfonyl]imide anion to ease the separation. To make the whole process faster and sensitive, extraction methodology was coupled with anion-exchange high-performance liquid chromatography with diode array detection (HPLC-DAD) and fluorescence spectroscopy. The developed in situ MIL-DLLME method noted effective than the conventional DLLME method as it required only 3 min for DNA extraction and yielded 1.1–1.5 times higher extraction efficiency (EFs). In another attempt, the Anderson group developed a method to remove the DNA further from MIL by designing the PCR buffer and coupling it with the process (Clark et al., 2015b). Clark et al. further developed a particle-free approach to sequence-specific DNA extraction using a magnetic liquid support and ion-tagged oligonucleotide (ITO) probes that can distinguish nucleotide mismatch and was more sensitive than a commercial magnetic bead-based method for the capture of target DNA from a pool of interfering genomic DNA (Clark et al., 2017).
Another aspect of DNA extraction comes from the bacterial gene transformation that implies the gene cloning technology (Lorenz and Wackernagel, 1994). In this technology, bacteria receives a new genetic trait through foreign DNA. Such gene transformation occurs spontaneously in few prokaryotes naturally; however, most of the bacterial cells require artificial transformation, stemming from the slow diffusion of hydrophilic DNA's entry across the hydrophobic lipid bilayer membrane and the slight electrostatic repulsion due to anionic DNA as well as the anionic head-groups of the bilayer membrane (van Die et al., 1983). Also, DNA molecules undergo hydrolysis and enzymatic degradation during transformation (Caruso et al., 2012). During transformation, DNA molecules are loaded onto the delivery vectors that efficiently transfer the genetic material across the lipid bilayer without any possibility of enzymatic degradation. The DNA delivery vectors mainly involve the encapsulation of DNA within the delivery vectors such as cationic surfactants, triblock copolymer vesicles, viral capsids, protein superstructures, lipid assemblies, polymer nanocapsules, and mesoporous structure and are crafted within the self-assembled superstructures, followed by transformation studies (Kikuchi et al., 1999; Vijayanathan et al., 2002; Guo, 2005; Li et al., 2011; Mingozzi and High, 2011). In addition to this DNA delivery vehicle-mediated transformation, some physical techniques such as a biolistic method, microinjection, electroporation, and heat shock, and poly(ethylene glycol) also required during the transformation to increase the efficiency (Klebe et al., 1983; Smith et al., 1992; Amoozgar and Yeo, 2012). The well-established calcium chloride method proposes that Ca2+ binds with anionic DNA and gets transferred via the transient pores formed during the heat shock. The necessity of heat shock and the inability to induce self-assembly in DNA during transformation renders calcium chloride method less lucrative. Instead, involving a hydrophobic ionic liquid that contains organic cation and also provides electrostatic interaction owing to the oppositely-charged ions and induces DNA self-assembly during the transformation. Soni et al. reported the formation of novel functional nanostructures during the electrostatic interaction between the phosphate group of DNA and the cationic part of hydrophobic ionic liquid [bmim][PF6] (Soni et al., 2015). The self-assembling nanostructures acted as promising synthetic non-viral vectors for the efficient bacterial pGFP gene transformation in cells. TGA analysis of the DNA-IL functional nanostructures revealed that nanostructures consist of about 16 wt% ionic liquid, which may stabilize the pDNA and eventually enhance transformation efficiency. Samarkina et al. studied the behavior of supramolecular systems based on homologous series of amphiphiles bearing imidazolium fragment (CnH2n+1Im+Br−, where n = 14, 16, 18) toward the aggregation behavior, solubilization activity toward the hydrophobic guest, interaction with DNA decamer as well as integration with the lipid bilayer (Samarkina et al., 2017). The elongation of hydrophobic moiety by two methylene groups was noted to decrease critical micelle concentration by 4-folds without any change in the solubility. The ability of amphiphiles to integrate with lipid bilayer strongly depends on the length of the hydrophobic fragments. The lower homolog C14H29Im+Br− increases the permeability of lipid bilayer whereas the higher homologs C16H33Im+Br− and C18H37Im+Br− stabilize it. Serker et al. noted positive zeta potential when employed [bmim][PF6] for plasmid DNA transformation (Sarker et al., 2019). [bmim][PF6] protected the plasmid DNA against ultrasonic shear stress and also enhanced in vitro gene transfection efficiency.
Toxicity and Recyclability of Ionic Liquids
The enormous interests in ionic liquids as biostabilizers necessitates the documentation of their toxicity on the environmental systems and various organisms. The toxicity of different ionic liquids is summarized by Thuy Pham et al. (2010). The inhibitory action of various ionic liquids on the activity of enzyme acetylcholinesterase is reported by many workers (Stock et al., 2004; Jastorff et al., 2005; Matzke et al., 2007; Ranke et al., 2007; Arning et al., 2008). The enzyme acetylcholinesterase has a major role in nerve response and function. The repressive action of ionic liquids on the activity of acetylcholinesterase has been observed to arise from the cation (Arning et al., 2008). However, later, several studies suggested that the toxicity depends on both cation and anion (Thuy Pham et al., 2010). The measurements showed that pyridine-based ionic liquids were less toxic than the imidazolium- and phosphonium-based ionic liquids. Contrary to the cations, anions are non-inhibitory to the enzyme activity except those containing fluoride and complex anions of fluorine ([BF4]−, [PF6]−, [OTf]−, [NTf2]−, [SbF6]−) (Stolte et al., 2006). The hydrolysis of complex anions of fluorine produces hydrofluoric acid which is a potential inhibitor of Na+-K+-ATPase, at the cell surface, and may interfere with essential cellular processes. The non-inhibitory action of anions might arise because of the binding to active site of enzyme (Matzke et al., 2007). However, the presence of alkyl chain on cation or anion increases the toxic effect of ionic liquids. The hydrophobicity of ionic liquids is also indicative of its toxicity (Kumar et al., 2009). The hydrophobicity of ionic liquids mainly arises due to the low charge density of anions. It is linked with the presence of fluorine atom in anion and increase with the number of fluorine atoms. However, a comparison between the alkyl chain length and anion in affecting the toxicity showed that the alkyl chain lengths on the cation gave rise to a larger impact on toxicity than a fluorinated anion. The effect of fluorinated anion ([NTf2]−) vanishes as the alkyl chain length reaches beyond octyl. Similarly, the unsaturation in cationic core and alkyl group on cation promotes toxicity (Kumar et al., 2009). Despite the distinct effect of different features of ionic liquids on toxicity, ionic liquids are noted as less toxic than their precursors (Kumar et al., 2009). Pyrrolidinium- and piperidinium-based ionic liquids containing propyl and butyl alkyl chain and bromide as the counter anion are less toxic than pyrrolidine and piperidine, respectively (Kumar et al., 2009). The task-effective ionic liquids containing nitrile, dimethyldisulphide, hydroxy, and polar ether group on cation reduces the toxicity compared to the non-functionalized ionic liquids (Stolte et al., 2006; Kumar et al., 2009). Thus, aprotic ionic liquids without modification cannot be considered as biocompatible ionic liquids due to their toxicity.
The protic ionic liquids have been shown to possess low cytotoxicity due to the lack of hydrophobic cation and perfluorinated anion (Gouveia et al., 2014). Choline-based protic ionic liquids are excellent example of biocompatible ionic liquids due to the low toxicity of choline and hence, have been involved as stabilizers for biopharmaceuticals (Gouveia et al., 2014). Weaver et al. studied the toxicity of choline-based protic ionic liquids combined with phosphate-based anions. The [Chol][DHP] exhibited the lowest cytotoxicity. Thus, choline family is one of the most suitable candidates of biocompatible protic ionic liquids and can be utilized as medium for protein and DNA stabilization. The development of biocompatible ionic liquids makes the toxicity issue irrelevant for their use as medium in many therapeutic applications and as stabilizers for biomolecules.
The wide applicability of ionic liquid systems in biomolecules stabilization, purification and storage makes them the frontrunner in the quest of the sustainable solvents. However, the efficient recyclability and reuse of ionic liquids are required to reach the economic and environmental goals. The recovery of ionic liquids is also essential as these solvents are toxic and non-biodegradable and releasing them to the aquatic environment might cause sever contamination (Frade and Afonso, 2010). These are the prime issues while using ionic liquids at a large scale. Several methods have been proposed to recover ionic liquids from solution, namely, distillation, adsorption, membrane separation, ion-exchange, and liquid-liquid extraction processes. The choice of the method in recovery depends on the medium characteristics, nature, and concentration of ionic liquids.
The negligible vapor pressure of ionic liquids makes their recovery easy from the ionic liquid-solvent mixtures by evaporation. However, a large difference in their boiling point is needed for adopting this method. Hydrophobic ionic liquids are easier to separate from aqueous mixture than hydrophilic one because of their water-immiscible nature. Membrane separation is a well-known, cost-effective, and commercially applicable method of separation for biomolecules (Haerens et al., 2010). In membrane separations, a membrane capable of separation retains specific compounds while allowing the passage to others. In pressure-driven membrane separation processes viz. microfiltration, ultrafiltration, nanofiltration, etc., when a pressure is applied across the membrane, the feed stream is split into permeate and retentate. Ionic liquids can be separated from permeate. Ultrafiltration method is used widely to separate ionic liquids from the proteins. Ultrafiltration membranes have a pore size 2–100 nm that allows the ionic liquid solution to pass through it while retaining the protein (Van Der Bruggen et al., 2003). The recovery of ionic liquids by ultrafiltration can be further improved by tuning the flow rate, concentration ratio, and temperature as suggested by Liu and Wu (1998).
Ionic liquid recovery from the DNA does not require special techniques. Precipitation of both DNA and ionic liquid upon addition of suitable solvents followed by the filtration and evaporation is normally used. In general, an ice-cold isopropyl alcohol (IPA)/ethanol is added to the ionic liquid-DNA mixture to precipitate the DNA (Mukesh et al., 2013; Singh et al., 2017). Upon adding ethyl acetate, the ionic liquid separates at the bottom and remaining DNA forms a hazy layer at the top that becomes transparent in 3–4 h. The traces of DNA can be completely removed from the ionic liquid by applying the same treatment again.
Conclusions and Outlook
Despite the large volume of research on ionic liquids in various physical and chemical transformations, their employment in the area of biochemistry has only seen growth in the last two decades. The remarkable properties such as insignificantly low vapor pressure and structure-tunability render ionic liquids more superior over the hazardous volatile organic solvents (VOSs) and, therefore, can be employed as a potent medium in controlling the functionality and stability of biomolecules like protein and DNA. Protein solubility, structural stability, crystallization, and separation in ionic liquids are often scaled either in the direct or indirect order of the Hofmeister ordering of ions. However, the overall influence of a particular ionic liquid on a protein cannot be predicted and necessitates further investigation both from the experimental and computational researches. Compared to the dubious conclusions drawn from the ionic liquid-protein interactions owing to the structural variations, ionic liquid-DNA interactions are facile as the expected outcome of a particular ionic liquid on the structure and stability can be foreseen based on the available research. In the case of DNA, ionic liquids emerged as a natural long-term stabilizer and nuclease inhibitor that causes slow degradation to the stored DNA. Ionic liquids provide the five major interacting forces like hydrogen bonding, base stacking, conformational entropy, hydration, and cation binding that determine the structure and stability of ionic liquids. Despite the growing interests of ionic liquids in biochemistry, their moderate toxicity and involvement of volatile organic compounds during synthesis and recyclability are foremost concerns lingered with these coulombic media and ought to be addressed shortly. However, the introduction of biocompatible ionic liquids eliminates the problem of toxicity up to a certain length.
Author Contributions
All authors have made significant contributions to the reading, writing sections of the manuscripts, and approved the final version submitted to the journal.
Conflict of Interest
The authors declare that the research was conducted in the absence of any commercial or financial relationships that could be construed as a potential conflict of interest.
Acknowledgments
We are thankful to the Wallenberg Wood Science Center (WWSC), Kempe Foundations, the Swedish Research Council, the Swedish Energy Agency, and the Bio4Energy (B4E) programme. This work is also part of the activities of the Johan Gadolin Process Chemistry Center at Åbo Akademi University.
References
Ajaj, Y. (2010). Dynamics and thermodynamics of protein folding and interactions in water-cosolvent systems. (Ph.D. thesis). Ruhr-University Bochum, Bochum, Germany.
Algaer, E. A., and van der Vegt, N. F. A. (2011). Hofmeister ion interactions with model amide compounds. J. Phys. Chem. B 115, 13781–13787. doi: 10.1021/jp208583w
Amoozgar, Z., and Yeo, Y. (2012). Recent advances in stealth coating of nanoparticle drug delivery systems. Wiley Interdiscip. Rev. Nanomed. Nanobiotechnol. 4, 219–233. doi: 10.1002/wnan.1157
Anthony, J. L., Maginn, E. J., and Brennecke, J. F. (2001). Solution thermodynamics of imidazolium-based ionic liquids and water. J. Phys. Chem. B 105, 10942–10949. doi: 10.1021/jp0112368
Araque, J. C., Yadav, S. K., Shadeck, M., Maroncelli, M., and Margulis, C. J. (2015). How is diffusion of neutral and charged tracers related to the structure and dynamics of a room-temperature ionic liquid? Large deviations from Stokes–Einstein behavior explained. J. Phys. Chem. B 119, 7015–7029. doi: 10.1021/acs.jpcb.5b01093
Armand, M., Endres, F., MacFarlane, D. R., Ohno, H., and Scrosati, B. (2009). Ionic liquid materials for the electrochemical challenges of the future. Nat. Mater. 8, 621–629. doi: 10.1038/nmat2448
Arning, J., Stolte, S., Böschen, A., Stock, F., Pitner, W.-R., Welz-Biermann, U., et al. (2008). Qualitative and quantitative structure activity relationships for the inhibitory effects of cationic head groups, functionalized side chains and anions of ionic liquids on acetylcholinesterase. Green Chem. 10, 47–58. doi: 10.1039/B712109A
Banik, R. M., Santhiagu, A., Kanari, B., Sabarinath, C., and Upahyay, S. N. (2003). Technological aspects of extractive fermentation using aqueous two-phase systems. World J. Microbiol. Biotechnol. 19, 337–348. doi: 10.1023/A:1023940809095
Batchelor, J. D., Olteanu, A., Tripathy, A., and Pielak, G. J. (2004). Impact of protein denaturants and stabilizers on water structure. J. Am. Chem. Soc. 126, 1958–1961. doi: 10.1021/ja039335h
Benton, L. A., Smith, A. E., Young, G. B., and Pielak, G. J. (2012). Unexpected effects of macromolecular crowding on protein stability. Biochemistry 51, 9773–9775. doi: 10.1021/bi300909q
Bhattacharya, S., and Mandal, S. (1997). Interaction of surfactants with DNA. Role of hydrophobicity and surface charge on intercalation and DNA melting. Biochim. Biophys. Acta Biomembr. 1323, 29–44. doi: 10.1016/S0005-2736(96)00171-X
Bihari, M., Russell, T. P., and Hoagland, D. A. (2010). Dissolution and dissolved state of cytochrome c in a neat, hydrophilic ionic liquid. Biomacromolecules 11, 2944–2948. doi: 10.1021/bm100735z
Blesic, M., Marques, M. H., Plechkova, N. V., Seddon, K. R., Rebelo, L. P. N., and Lopes, A. (2007). Self-aggregation of ionic liquids: micelle formation in aqueous solution. Green Chem. 9, 481–490. doi: 10.1039/b615406a
Bonner, G., and Klibanov, A. M. (2000). Structural stability of DNA in nonaqueous solvents. Biotechnol. Bioeng. 68, 339–344. doi: 10.1002/(sici)1097-0290(20000505)68:3<339::aid-bit12>3.0.co;2-o
Bowers, A. N., Trujillo-Rodríguez, M. J., Farooq, M. Q., and Anderson, J. L. (2019). Extraction of DNA with magnetic ionic liquids using in situ dispersive liquid–liquid microextraction. Anal. Bioanal. Chem. 411, 7375–7385. doi: 10.1007/s00216-019-02163-9
Broering, J. M., and Bommarius, A. S. (2005). Evaluation of Hofmeister effects on the kinetic stability of proteins. J. Phys. Chem. B 109, 20612–20619. doi: 10.1021/jp053618+
Bruce, D. W., Cabry, C. P., Lopes, J. N. C., Costen, M. L., D'Andrea, L., Grillo, I., et al. (2017). Nanosegregation and structuring in the bulk and at the surface of ionic-liquid mixtures. J. Phys. Chem. B 121, 6002–6020. doi: 10.1021/acs.jpcb.7b01654
Bui-Le, L., Clarke, C. J., Bröhl, A., Brogan, A. P. S., Arpino, J. A. J., Polizzi, K. M., et al. (2020). Revealing the complexity of ionic liquid–protein interactions through a multi-technique investigation. Commun. Chem. 3:55. doi: 10.1038/s42004-020-0302-5
Burney, P. R., Nordwald, E. M., Hickman, K., Kaar, J. L., and Pfaendtner, J. (2015). Molecular dynamics investigation of the ionic liquid/enzyme interface: application to engineering enzyme surface charge. Proteins 83, 670–680. doi: 10.1002/prot.24757
Byrne, N., Wang, L.-M., Belieres, J.-P., and Angell, C. A. (2007). Reversible folding–unfolding, aggregation protection, and multi-year stabilization, in high concentration protein solutions, using ionic liquids. Chem. Commun. 2007, 2714–2716. doi: 10.1039/B618943A
Cammarata, L., Kazarian, S. G., Salter, P. A., and Welton, T. (2001). Molecular states of water in room temperature ionic liquids. Phys. Chem. Chem. Phys. 3, 5192–5200. doi: 10.1039/b106900d
Cardoso, L., and Micaelo, N. M. (2011). DNA molecular solvation in neat ionic liquids. ChemPhysChem 12, 275–277. doi: 10.1002/cphc.201000645
Caruso, F., Hyeon, T., and Rotello, V. M. (2012). Nanomedicine. Chem. Soc. Rev. 41, 2537–2538. doi: 10.1039/C2CS90005J
Castner, J. E. W., and Wishart, J. F. (2010). Spotlight on ionic liquids. J. Chem. Phys. 132, 120901–120909. doi: 10.1063/1.3373178
Chandran, A., Ghoshdastidar, D., and Senapati, S. (2012). Groove binding mechanism of ionic liquids: a key factor in long-term stability of DNA in hydrated ionic liquids? J. Am. Chem. Soc. 134, 20330–20339. doi: 10.1021/ja304519d
Chen, Y., Zhang, Y., Ke, F., Zhou, J., Wang, H., and Liang, D. (2011). Solubility of neutral and charged polymers in ionic liquids studied by laser light scattering. Polymer 52, 481–488. doi: 10.1016/j.polymer.2010.11.034
Cheng, D.-H., Chen, X.-W., Wang, J.-H., and Fang, Z.-L. (2007). An abnormal resonance light scattering arising from ionic-liquid/DNA/ethidium interactions. Chem. Eur. J. 13, 4833–4839. doi: 10.1002/chem.200601544
Clark, K. D., Nacham, O., Yu, H., Li, T., Yamsek, M. M., Ronning, D. R., et al. (2015a). Extraction of DNA by magnetic ionic liquids: tunable solvents for rapid and selective DNA analysis. Anal. Chem. 87, 1552–1559. doi: 10.1021/ac504260t
Clark, K. D., Varona, M., and Anderson, J. L. (2017). Ion-tagged oligonucleotides coupled with a magnetic liquid support for the sequence-specific capture of DNA. Angew. Chem. Int. Ed. 56, 7630–7633. doi: 10.1002/anie.201703299
Clark, K. D., Yamsek, M. M., Nacham, O., and Anderson, J. L. (2015b). Magnetic ionic liquids as PCR-compatible solvents for DNA extraction from biological samples. Chem. Commun. 51, 16771–16773. doi: 10.1039/C5CC07253K
Collins, K. D. (1997). Charge density-dependent strength of hydration and biological structure. Biophys. J. 72, 65–76. doi: 10.1016/S0006-3495(97)78647-8
Collins, K. D. (2004). Ions from the Hofmeister series and osmolytes: effects on proteins in solution and in the crystallization process. Methods 34, 300–311. doi: 10.1016/j.ymeth.2004.03.021
Collins, K. D. (2006). Ion hydration: implications for cellular function, polyelectrolytes, and protein crystallization. Biophys. Chem. 119, 271–281. doi: 10.1016/j.bpc.2005.08.010
Constantinescu, D., Herrmann, C., and Weingärtner, H. (2007). Protein denaturation by ionic liquids and the Hofmeister series: a case study of aqueous solutions of Ribonuclease A. Angew. Chem. Int. Ed. 46, 8887–8889. doi: 10.1002/anie.200702295
Constantinescu, D., Herrmann, C., and Weingärtner, H. (2010). Patterns of protein unfolding and protein aggregation in ionic liquids. Phys. Chem. Chem. Phys. 12, 1756–1763. doi: 10.1039/B921037G
Corradini, D., Nicoletti, I., and Bonn, G. K. (2009). Co-electroosmotic capillary electrophoresis of basic proteins with 1-alkyl-3- methylimidazolium tetrafluoroborate ionic liquids as non-covalent coating agents of the fused-silica capillary and additives of the electrolyte solution. Electrophoresis 30, 1869–1876. doi: 10.1002/elps.200800447
Dang, L. P., Fang, W. Z., Li, Y., Wang, Q., Xiao, H. Z., and Wang, Z. Z. (2013). Ionic liquid-induced structural and activity changes in hen egg white lysozyme. Appl. Biochem. Biotechnol. 169, 290–300. doi: 10.1007/s12010-012-9986-z
de Diego, T., Lozano, P., Gmouh, M., Voltier, M., and Iborra, I. M. (2005). Understanding structure-stability relationships of Candida antartica lipase B in ionic liquids. Biomacromolecules 6, 1457–1464. doi: 10.1021/bm049259q
Diddens, D., Lesch, V., Heuer, A., and Smiatek, J. (2017). Aqueous ionic liquids and their influence on peptide conformations: denaturation and dehydration mechanisms. Phys. Chem. Chem. Phys. 19, 20430–20440. doi: 10.1039/C7CP02897K
Ding, Y., Zhang, L., Xie, J., and Guo, R. (2010). Binding characteristics and molecular mechanism of interaction between ionic liquid and DNA. J. Phys. Chem. B 114, 2033–2043. doi: 10.1021/jp9104757
Dreyer, S., Salim, P., and Kragl, U. (2009). Driving forces of protein partitioning in an ionic liquid-based aqueous two-phase system. Biochem. Eng. J. 46, 176–185. doi: 10.1016/j.bej.2009.05.005
Earle, M. J., and Seddon, K. R. (2000). Ionic liquids. Green solvents for the future. Pure Appl. Chem. 72, 1391–1398. doi: 10.1351/pac200072071391
Eckstein, M., Sesing, M., Kragl, U., and Adlercreutz, P. (2002). At low water activity α-chymotrypsin is more active in an ionic liquid than in non-ionic organic solvents. Biotechnol. Lett. 24, 867–872. doi: 10.1023/A:1015564608261
Egorova, K. S., Gordeev, E. G., and Ananikov, V. P. (2017). Biological activity of ionic liquids and their application in pharmaceutics and medicine. Chem. Rev. 117, 7132–7189. doi: 10.1021/acs.chemrev.6b00562
Feig, M., and Pettitt, B. M. (1999). Sodium and chlorine ions as part of the DNA solvation shell. Biophys. J. 77, 1769–1781. doi: 10.1016/S0006-3495(99)77023-2
Figueiredo, A. M., Sardinha, J., Mooreb, G. R., and Cabrita, E. J. (2013). Protein destabilisation in ionic liquids: the role of preferential interactions in denaturation. Phys. Chem. Chem. Phys. 15, 19632–19643. doi: 10.1039/c3cp53395f
Frade, R. F., and Afonso, C. A. (2010). Impact of ionic liquids in environment and humans: an overview. Hum. Exp. Toxicol. 29, 1038–1054. doi: 10.1177/0960327110371259
Fujita, K., Forsyth, M., MacFarlane, D. R., Reid, R. W., and Elliott, G. D. (2006). Unexpected improvement in stability and utility of cytochrome C by solution in biocompatible ionic liquids. Biotechnol. Bioeng. 94, 1209–1213. doi: 10.1002/bit.20928
Fujita, K., MacFarlane, D. R., and Forsyth, M. (2005). Protein solubilizing and stabilizing ionic liquids. Chem. Commun. 2005, 4804–4806. doi: 10.1039/b508238b
Fujita, K., MacFarlane, D. R., Foryth, M., Yoshizawa-Fujita, M., Murata, K., Nakamura, N., et al. (2007). Solubility and stability of cytochrome C in hydrated ionic liquids: effect of oxo acid residues and kosmotropicity. Biomacromolecules 8, 2080–2086. doi: 10.1021/bm070041o
Fujita, K., and Ohno, H. (2010). Enzymatic activity and thermal stability of metalloproteins in hydrated ionic liquids. Biopolymers 93, 1093–1099. doi: 10.1002/bip.21526
Fujita, K., and Ohno, H. (2012). Stable G-quadruplex structure in a hydrated ion pair: cholinium cation and dihydrogen phosphate anion. Chem. Commun. 48, 5751–5753. doi: 10.1039/c2cc30554b
Fumino, K., Wulf, A., and Ludwig, R. (2009). Hydrogen bonding in protic ionic liquids: reminiscent of water. Angew. Chem. Int. Ed. 48, 3184–3186. doi: 10.1002/anie.200806224
Funkner, S., Niehues, G., Schmidt, D. A., Heyden, M., Schwaab, G., Callahan, K. M., et al. (2012). Watching the low-frequency motions in aqueous salt solutions: the terahertz vibrational signatures of hydrated ions. J. Am. Chem. Soc. 134, 1030–1035. doi: 10.1021/ja207929u
Garlitz, J. A., Summers, C. A., Flowers, R. A. I. I., and Borgstahl, G. E. (1999). Ethylammonium nitrate: a protein crystallization reagent. Acta Crystallogr. D 55, 2037–2038. doi: 10.1107/S0907444999011774
Ghanta, K. P., Pal, T., Mondal, S., and Bandyopadhyay, S. (2020). Microscopic understanding of the effect of ionic liquid on protein from molecular simulation studies. J. Phys. Chem. B 124, 3909–3921. doi: 10.1021/acs.jpcb.0c02001
Ghosh, S., Parui, S., Jana, B., and Bhattacharyya, K. (2015). Ionic liquid induced dehydration and domain closure in lysozyme: FCS and MD simulation. J. Chem. Phys. 143:125103. doi: 10.1063/1.4931974
Gough, C. R., Rivera-Galletti, A., Cowan, D. A., Cruz, D. S., and Hu, X. (2020). Protein and polysaccharide-based fiber materials generated from ionic liquids: a review. Molecules 25:3362. doi: 10.3390/molecules25153362
Gouveia, W., Jorge, T. F., Martins, S., Meireles, M., Carolino, M., Cruz, C., et al. (2014). Toxicity of ionic liquids prepared from biomaterials. Chemosphere 104, 51–56. doi: 10.1016/j.chemosphere.2013.10.055
Green, A. A. (1932). Studies in the physical chemistry of the proteins: x. The solubility of hemoglobin in solutions of chlorides and sulfates of varying concentration. J. Biol. Chem. 95, 47–66.
Gudnason, H., Dufva, M., Bang, D. D., and Wolff, A. (2007). Comparison of multiple DNA dyes for real-time PCR: effects of dye concentration and sequence composition on DNA amplification and melting temperature. Nucleic Acids Res. 35:e127. doi: 10.1093/nar/gkm671
Guo, P. (2005). RNA nanotechnology: engineering, assembly and applications in detection, gene delivery and therapy. J. Nanosci. Nanotechnol. 5, 1964–1982. doi: 10.1166/jnn.2005.446
Gutowski, K. E., Broker, G. A., Willauer, H. D., Huddleston, J. G., Swatloski, R. P., Holbrey, J. D., et al. (2003). Controlling the aqueous miscibility of ionic liquids: aqueous biphasic systems of water-miscible ionic liquids and water-structuring salts for recycle, metathesis, and separations. J. Am. Chem. Soc. 125, 6632–6633. doi: 10.1021/ja0351802
Haberler, M., Schröder, C., and Steinhauser, O. (2011). Solvation studies of a zinc finger protein in hydrated ionic liquids. Phys. Chem. Chem. Phys. 13, 6955–6969. doi: 10.1039/c0cp02487b
Haberler, M., Schröder, C., and Steinhauser, O. (2012). Hydrated ionic liquids with and without solute: the influence of water content and protein solutes. J. Chem. Theory Comput. 8, 3911–3928. doi: 10.1021/ct300191s
Haberler, M., and Steinhauser, O. (2011). On the influence of hydrated ionic liquids on the dynamical structure of model proteins: a computational study. Phys. Chem. Chem. Phys. 13, 17994–18004. doi: 10.1039/c1cp22266j
Haerens, K., Van Deuren, S., Matthijs, E., and Van der Bruggen, B. (2010). Challenges for recycling ionic liquids by using pressure driven membrane processes. Green Chem. 12, 2182–2188. doi: 10.1039/c0gc00406e
Hayes, R., Warr, G. W., and Atkin, R. (2015). Structure and nanostructure in ionic liquids. Chem. Rev. 115, 6357–6426. doi: 10.1021/cr500411q
He, C., Li, S., Liu, H., Li, K., and Liu, F. (2005). Extraction of testosterone and epitestosterone in human urine using aqueous two-phase systems of ionic liquid and salt. Chromatogr. A 1082, 143–149. doi: 10.1016/j.chroma.2005.05.065
He, Y., Shang, Y., Liu, Z., Shao, S., Liu, H., and Hu, Y. (2013). Interactions between ionic liquid surfactant [C12mim]Br and DNA in dilute brine. Colloid Surface B 101, 398–404. doi: 10.1016/j.colsurfb.2012.07.027
Heyda, J., Vincent, J. C., Tobias, D. J., Dzubiella, J., and Jungwirth, P. (2010). Ion specificity at the peptide bond: molecular dynamics simulations of N-methylacetamide in aqueous salt solutions. J. Phys. Chem. B 114, 1213–1220. doi: 10.1021/jp910953w
Hofmeister, F. (1888). On the theory of the effects of salts. Arch. Exp. Pathol. Pharmakol. 1888, 247–260. doi: 10.1007/BF01918191
Huang, M.-M., and Weingärtner, H. (2008). Protic ionic liquids with unusually high dielectric permittivities. ChemPhysChem 9, 2172–2173. doi: 10.1002/cphc.200800523
Hunter, C. A. (1993). Sequence-depenent DNA structure: the role of base stacking interactions. J. Mol. Biol. 230, 1025–1054. doi: 10.1006/jmbi.1993.1217
Hyde, J. E., and Read, M. (1993). The extraction and purification of DNA and RNA from in vitro cultures of the malaria parasite plasmodium falciparum. Methods Mol. Biol. 21, 133–143. doi: 10.1385/0-89603-239-6:133
Idris, A., Vijayaraghavan, R., Rana, U. A., Fredericks, D., Pattia, A. F., and MacFarlane, D. R. (2013). Dissolution of feather keratin in ionic liquids. Green Chem. 15, 525–534. doi: 10.1039/c2gc36556a
Jaeger, V. W., Burney, P., and Pfaendtner, J. (2014). Comparison of three ionic liquid-tolerant cellulases by molecular dynamics. Biophys J. 108, 880–892. doi: 10.1016/j.bpj.2014.12.043
Jaeger, V. W., and Pfaendtner, J. (2016). Destabilization of human serum albumin by ionic liquids studied using enhanced molecular dynamics simulations. J. Phys. Chem. B 120, 12079–12087. doi: 10.1021/acs.jpcb.6b09410
Jastorff, B., Mölter, K., Behrend, P., Bottin-Weber, U., Filser, J., Heimers, A., et al. (2005). Progress in evaluation of risk potential of ionic liquids-basis for an eco-design of sustainable products. Green Chem. 7, 362–372. doi: 10.1039/b418518h
Jiang, T.-F-., Gu, Y.-L., Liang, B., Li, J.-B., Shi, Y.-P., and Ou, Q.-Y. (2003). Dynamically coating the capillary with 1-alkyl-3-methylimidazolium-based ionic liquids for separation of basic proteins by capillary electrophoresis. Anal. Chim. Acta 479, 249–254. doi: 10.1016/S0003-2670(02)01537-4
Jiang, W., Wang, Y., and Voth, G. A. (2007). Molecular dynamics simulation of nanostructural organization in ionic liquid/water mixtures. J. Phys. Chem. B 111, 4812–4818. doi: 10.1021/jp067142l
Judge, R. A., Takahashi, S., Longenecker, K. L., Fry, E. H., Abad-Zapatero, C., and Chiu, M. L. (2009). The effect of ionic liquids on protein crystallization and X-ray diffraction resolution. CrystGrowthDes 9, 3463–3469. doi: 10.1021/cg900140b
Jungwirth, P., and Cremer, P. S. (2014). Beyond hofmeister. Nat. Chem. 6, 261–263. doi: 10.1038/nchem.1899
Kaar, J. L., Jesionowski, A. M., Berberich, J. A., Moulton, R., and Russell, A. J. (2003). Impact of ionic liquid physical properties on lipase activity and stability. J. Am. Chem. Soc. 125, 4125–4131. doi: 10.1021/ja028557x
Kamlet, M. J., Abboud, J. L., and Taft, R. W. (1977). The solvatochromic comparison method 6. The π* scale of solvent polarities. J. Am. Chem. Soc. 99, 6027–6038. doi: 10.1021/ja00460a031
Kamlet, M. J., and Taft, R. W. (1976). The solvatochromic comparison method. I. The beta-scale of solvent hydrogen-bond acceptor (HBA) basicities. J. Am. Chem. Soc. 98, 377–383. doi: 10.1021/ja00418a009
Khimji, I., Doan, K., Bruggeman, K., Huang, P.-J. J., Vajha, P., and Liu, J. (2013b). Extraction of DNA staining dyes from DNA using hydrophobic ionic liquids. Chem. Commun. 49, 4537–4539. doi: 10.1039/c3cc41364k
Khimji, I., Shin, J., and Liu, J. (2013a). DNA duplex stabilization in crowded polyanion solutions. Chem. Comm. 49, 1306–1308. doi: 10.1039/c2cc38627e
Kikuchi, H., Suzuki, N., Ebihara, K., Morita, H., Ishii, Y., Kikuchi, A., et al. (1999). Gene delivery using liposome technology. J. Control Rel. 1999, 269–277. doi: 10.1016/S0168-3659(99)00047-4
Klähn, M., Lim, G. S., Seduraman, A., and Wu, P. (2011a). On the different roles of anions and cations in the solvation of enzymes in ionic liquids. Phys. Chem. Chem. Phys. 13, 1649–1662. doi: 10.1039/C0CP01509A
Klähn, M., Lim, G. S., and Wu, P. (2011b). How ion properties determine the stability of a lipase enzyme in ionic liquids: A molecular dynamics study. Phys. Chem. Chem. Phys. 13, 18647–18660. doi: 10.1039/c1cp22056j
Klebe, R. J., Harriss, J. V., Sharp, Z. D., and Douglas, M. G. (1983). A general method for polyethylene-glycol-induced genetic transformation of bacteria and yeast. Gene 25, 333–341. doi: 10.1016/0378-1119(83)90238-X
Kobayashi, T., Kemna, A., Fyta, M., Braunschweig, B., and Smiatek, J. (2019). Aqueous mixtures of room-temperature ionic liquids: entropy-driven accumulation of water molecules at interfaces. J. Phys. Chem. C 123, 13795–13803. doi: 10.1021/acs.jpcc.9b04098
Kobayashi, T., Reid, J. E. S. J., Shimiju, S., Fyta, M., and Smiatek, J. (2017). The properties of residual water molecules in ionic liquids: a comparison between direct and inverse Kirkwood–Buff approaches. Phys. Chem. Chem. Phys. 19, 18924–18937. doi: 10.1039/C7CP03717A
Kool, E., Morales, J., and Guckian, K. (2000). Mimicking the structure and function of DNA: insights into DNA stability and replication. Angew. Chem. Int. Ed. 39, 990–1009. doi: 10.1002/(SICI)1521-3773(20000317)39:6<990::AID-ANIE990>3.0.CO;2-0
Krüger, M., Bründermann, E., Funkner, S., Weingärtner, H., and Havenith, M. (2010). Communications: polarity fluctuations of the protic ionic liquid ethylammonium nitrate in the terahertz regime. J. Chem. Phys. 132, 101101–101104. doi: 10.1063/1.3352585
Kula, M.-R., Kroner, K. H., and Hustedt, H. (1982). Purification of enzymes by liquid–liquid extraction. Adv. Biochem. Eng. 24, 73–118. doi: 10.1007/3-540-11699-0_11
Kumar, A., Rani, A., and Venkatesu, P. (2014). A comparative study of the effects of the Hofmeister series anions of the ionic salts and ionic liquids on the stability of a-chymotrypsin. New J. Chem. 39, 938–952. doi: 10.1039/C4NJ01596G
Kumar, A., and Venkatesu, P. (2013). Prevention of insulin self-aggregation by a protic ionic liquid. RSV Adv. 3, 362–367. doi: 10.1039/C2RA22277A
Kumar, A., and Venkatesu, P. (2014). The stability of insulin in the presence of short alkyl chain imidazolium-based ionic liquids. RSC Adv. 4, 4487–4499. doi: 10.1039/C3RA44477E
Kumar, R. A., Papaiconomou, N., Lee, J.-M., Salminen, J., Clark, D. S., and Prausnitz, J. M. (2009). In vitro cytotoxicities of ionic liquids: effect of cation rings, functional groups, and anions. Environ. Toxicol. 24, 388–395. doi: 10.1002/tox.20443
Kunz, W., Nostro, P. L., and Ninham, B. W. (2004). The present state of affairs with Hofmeister effects. Curr. Opin. Colloid Interface Sci. 9, 1–18. doi: 10.1016/j.cocis.2004.05.004
Laane, C., Boeren, S., Vos, K., and Veeger, C. (1987). Rules for optimization of biocatalysis in organic solvents. Biotechnol. Bioeng. 30, 81–87. doi: 10.1002/bit.260300112
Lange, C., Patil, G., and Rudolph, R. (2005). Ionic liquids as refolding additives: N′-alkyl and N′-(ω-hydroxyalkyl) N-methylimidazolium chlorides. Protein Sci. 14, 2693–2701. doi: 10.1110/ps.051596605
Laszlo, J. A., and Compton, D. L. (2002). Comparison of peroxidase activities of hemin, cytochrome c and microperoxidase-11 in molecular solvents and imidazolium-based ionic liquids. J. Mol. Catal. B Enzym. 18, 109–120. doi: 10.1016/S1381-1177(02)00074-7
Lau, R. M., Sorgetrager, M. J., Carrea, G., van Rantwijk, F., Secundo, F., and Sheldon, R. A. (2004). Dissolution of candida antarctica lipase B in ionic liquids: effects on structure and activity. Green Chem. 6, 483–487. doi: 10.1039/b405693k
Lerman, L. S. (1964). Acridine mutagens and DNA structure. J. Cell. Physiol. 64, 1–18. doi: 10.1002/jcp.1030640403
Lesch, V., Heuer, A., Tatsis, V. A., Holm, C., and Smiatek, J. (2015). Peptides in the presence of aqueous ionic liquids: tunable co-solutes as denaturants or protectants? Phys. Chem. Chem. Phys. 17, 26049–26053. doi: 10.1039/C5CP03838C
Li, T., Li, B., Dong, S., and Wang, E. (2007). Ionic liquids as selectors for the enhanced detection of proteins. Chem. Eur. J. 13, 8516–8521. doi: 10.1002/chem.200700275
Li, X., Xie, Q. R., Zhang, J., Xia, W., and Gu, H. (2011). The packaging of siRNA within the mesoporous structure of silica nanoparticles. Biomaterials 32, 9546–9556. doi: 10.1016/j.biomaterials.2011.08.068
Lim, G. S., Zidar, J., Cheong, D. W., Jaenicke, S., and Klähn, M. (2014). Impact of lonic liquids in aqueous solution on bacterial plasma membranes studied with molecular dynamics simulations. J. Phys. Chem. B 118, 10444–10459. doi: 10.1021/jp5060952
Liu, C., and Wu, X. (1998). Optimization of operation parameters in ultrafiltration process. J. Biotechnol. 66, 195–202. doi: 10.1016/S0168-1656(98)00161-8
Liu, X., Zhou, G., He, H., Zhang, X., Wang, J., and Zhang, S. (2015). Rodlike micelle structure and formation of ionic liquid in aqueous solution by molecular simulation. Ind. Eng. Chem. Res. 54, 1681–1688. doi: 10.1021/ie503109z
Lorenz, M. G., and Wackernagel, W. (1994). Bacterial gene transfer by natural genetic transformation in the environment. Microbiol. Rev. 58, 563–602. doi: 10.1128/MMBR.58.3.563-602.1994
Lumry, R., and Rajender, S. (1970). Enthalpy-entropy compensation phenomena in water solutions of proteins and small molecules: a ubiquitous property of water. Biopolymers 9, 1125–1227. doi: 10.1002/bip.1970.360091002
Mandal, B., Mondal, S., Pan, A., Moulik, S. P., and Ghosh, S. (2015). Physicochemical study of the interaction of lysozyme with surface active ionic liquid 1-butyl-3-methylimidazolium octylsulfate [BMIM] [OS] in aqueous and buffer media. Colloids Surf. A Physicochem. Eng. Asp. 484, 345–353. doi: 10.1016/j.colsurfa.2015.07.052
Marusic, M., Tateishi-Karimata, H., Sugimoto, N., and Plavec, J. (2015). Structural foundation for DNA behavior in hydrated ionic liquid: an NMR study. Biochimie 108, 169–177. doi: 10.1016/j.biochi.2014.11.015
Matzke, M., Stolte, S., Thiele, K., Juffernholz, T., Arning, J., Ranke, J., et al. (2007). The influence of anion species on the toxicity of 1-alkyl-3-methylimidazolium ionic liquids observed in an (eco)toxicological test battery. Green Chem. 9, 1198–1207. doi: 10.1039/b705795d
Mazzini, V., Liu, G., and Craig, V. S. J. (2018). Probing the Hofmeister series beyond water: specific-ion effects in non-aqueous solvents. J. Chem. Phys. 148, 222805–222812. doi: 10.1063/1.5017278
McFail-Isom, L., Sines, C. C., and Williams, L. D. (1999). DNA structure: cations in charge? Curr. Opin. Struc. Boil. 9, 298–304. doi: 10.1016/s0959-440x(99)80040-2
Méndez-Morales, T., Carrete, J., Cabeza, Ó., Gallego, L. J., and Varela, L. M. (2011). Molecular dynamics simulation of the structure and dynamics of water-1-Alkyl-3-methylimidazolium ionic liquid mixtures. J. Phys. Chem. B 115, 6995–7008. doi: 10.1021/jp202692g
Micaêlo, N. M., and Soares, C. M. (2008). Protein structure and dynamics in ionic liquids. Insights from molecular dynamics simulation studies. J. Phys. Chem. B 112, 2566–2572. doi: 10.1021/jp0766050
Mingozzi, F., and High, K. A. (2011). Therapeutic in vivo gene transfer for genetic disease using AAV: progress and challenges. Nat. Rev. Genet. 12, 341–355. doi: 10.1038/nrg2988
Mukesh, C., Mondal, D., Sharma, M., and Prasad, K. (2013). Rapid dissolution of DNA in a novel bio-based ionic liquid with long-term structural and chemical stability: successful recycling of the ionic liquid for reuse in the process. Chem. Commun. 49, 6849–6851. doi: 10.1039/c3cc42829j
Muldoon, M., Lagunas-Castedo, C., and Nockemann, P. (2012). Hofmeister effects of ionic liquids in protein crystallization: direct and water-mediated interactions. Cryst. Eng. Comm. 14, 4912–4921. doi: 10.1039/c2ce25129a
Nakano, M., Tateishi-Karimata, H., Tanaka, S., and Sugimoto, N. (2014a). Choline ion interactions with DNA atoms explain unique stabilization of A-T base pairs in DNA duplexes: a microscopic view. J. Phys. Chem. B 118, 379–389. doi: 10.1021/jp406647b
Nakano, M., Tateishi-Karimata, H., Tanaka, S., and Sugimoto, N. (2014b). Affinity of molecular ions for DNA structures is determined by solvent accessible surface area. J. Phys. Chem. B 118, 9583–9594. doi: 10.1021/jp505107g
Neidle, S., Pearl, L., and Skelly, J. (1987). DNA structure and perturbation by drug binding. Biochem. J. 243, 1–13. doi: 10.1042/bj2430001
Neves, C. M. S. S., Ventura, S. P. M., Freire, M. G., Marrucho, I. M., and Coutinho, J. A. P. (2009). Evaluation of cation influence on the formation and extraction capability of ionic-liquid-based aqueous biphasic systems. J. Phys. Chem. B 113, 5194–5199. doi: 10.1021/jp900293v
Nordwald, E. M., Armstrong, G. S., and Kaar, J. L. (2014). NMR-guided rational engineering of an ionic-liquid-tolerant lipase. ACS Catal. 4, 4057–4064. doi: 10.1021/cs500978x
Nostro, P. L., Ninham, B. W., Nostro, A. L., Pesavento, G., Fratoni, L., and Baglioni, P. (2005). Specific ion effects on the growth rates of Staphylococcus aureus and Pseudomonas aeruginosa. Phys. Biol. 2, 1–7. doi: 10.1088/1478-3967/2/1/001
Omta, A. W., Kropman, M. F., Woutersen, S., and Bakker, H. J. (2003a). Negligible effect of ions on the hydrogen-bond structure in liquid water. Science 301, 347–349. doi: 10.1126/science.1084801
Omta, A. W., Kropman, M. F., Woutersen, S., and Bakker, H. J. (2003b). Influence of ions on the hydrogen-bond structure in liquid water. J. Chem. Phys. 119, 12457–12461. doi: 10.1063/1.1623746
Oprzeska-Zingrebe, E. A., and Smiatek, J. (2018). Aqueous ionic liquids in comparison with standard co-solutes. Biophys. Rev. 10, 809–824. doi: 10.1007/s12551-018-0414-7
Pan, F., Roland, C., and Sagui, C. (2014). Ion distributions around left- and right-handed DNA and RNA duplexes: a comparative study. Nucleic Acids Res. 42, 13981–13996. doi: 10.1093/nar/gku1107
Pei, Y., Wang, J., Wu, K., Xuan, X., and Lu, X. (2009). Ionic liquid-based aqueous two-phase extraction of selected proteins. Sep. Purif. Techol. 64, 288–295. doi: 10.1016/j.seppur.2008.10.010
Portella, G., Germann, M. W., Hud, N. V., and Orozco, M. (2014). MD and NMR analyses of choline and TMA binding to duplex DNA: on the origins of aberrant sequence-dependent stability by alkyl cations in aqueous and water-free solvents. J. Am. Chem. Soc. 136, 3075–3086. doi: 10.1021/ja410698u
Ranke, J., Stolte, S., Störmann, R., Arning, J., and Jastorff, B. (2007). Design of sustainable chemical products – the example of ionic liquids. Chem. Rev. 107, 2183–2206. doi: 10.1021/cr050942s
Reichardt, C. (1994). Solvatochromic dyes as solvent polarity indicators. Chem. Rev. 94, 2319–2358. doi: 10.1021/cr00032a005
Reichardt, C. (2005). Polarity of ionic liquids determined empirically by means of solvatochromic pyridinium N-phenolate betaine dyes. Green Chem. 7, 339–351. doi: 10.1039/b500106b
Reichardt, C. (2008). Pyridinium-N-phenolate betaine dyes as empirical indicators of solvent polarity: some new findings. Pure Appl. Chem. 80, 1415–1432. doi: 10.1351/pac200880071415
Reid, J. E. S. J., Walker, A. J., and Shimizu, S. (2015). Residual water in ionic liquids: clustered or dissociated? Phys. Chem. Chem. Phys. 17, 14710–14718. doi: 10.1039/C5CP01854D
Reslan, M., and Kayser, V. (2018). Ionic liquids as biocompatible stabilizers of proteins. Biophys. Rev. 10, 781–793. doi: 10.1007/s12551-018-0407-6
Rivera-Rubero, S., and Baldelli, S. (2004). Influence of water on the surface of hydrophilic and hydrophobic room-temperature ionic liquids. J. Am. Chem. Soc. 126, 11788–11789. doi: 10.1021/ja0464894
Rogers, R. D., and Seddon, K. R. (2003). Ionic liquids—solvents of the future? Science 302, 792–793. doi: 10.1126/science.1090313
Rouzina, I., and Bloomfield, V. (2001). Force-induced melting of the DNA double helix. 2. Effect of solution conditions. Biophys. J. 80, 894–900. doi: 10.1016/S0006-3495(01)76068-7
Saenger, W. (1984). Principles of Nucleic Acid Structure. New York, NY: Springer-Verlag. doi: 10.1007/978-1-4612-5190-3
Saha, D., and Mukherjee, A. (2018). Effect of water and ionic liquids on biomolecules. Biophys. Rev. 10, 795–808. doi: 10.1007/s12551-018-0399-2
Salis, A., and Ninham, B. W. (2014). Models and mechanisms of Hofmeister effects in electrolyte solutions, and colloid and protein systems revisited. Chem. Soc. Rev. 43, 7358–7377. doi: 10.1039/C4CS00144C
Samarkina, D. A, Gabdrakhmanov, D. R., Lukashenko, S. S., Khamatgalimov, A. R., Kovalenko, V. I., and Zakharova, L. Y. (2017). Cationic amphiphiles bearing imidazole fragment: from aggregation properties to potential in biotechnologies. Colloids Surf. A Physicochem. Eng. Asp. A 529, 990–997. doi: 10.1016/j.colsurfa.2017.07.018
Sapir, L., and Harries, D. (2015). Macromolecular stabilization by excluded cosolutes: mean field theory of crowded solutions. J. Chem. Theory Comput. 11, 3478–3490. doi: 10.1021/acs.jctc.5b00258
Sarkar, S., Rajdev, P., and Singh, P. C. (2020). Hydrogen bonding of ionic liquids in the groove region of DNA controls the extent of its stabilization: synthesis, spectroscopic and simulation studies. Phys. Chem. Chem. Phys. 22, 15582–15591. doi: 10.1039/D0CP01548B
Sarker, S. R., Ball, A. S., Bhargava, S. K., and Soni, S. K. (2019). Evaluation of plasmid DNA stability against ultrasonic shear stress and its in vitro delivery efficiency using ionic liquid [Bmim][PF6]. RSC Adv. 9, 29225–29231. doi: 10.1039/C9RA03414E
Sasaki, Y., Miyoshi, D., and Sugimoto, N. (2007). Regulation of DNA nucleases by molecular crowding. Nucl. Acids Res. 35, 4086–4093. doi: 10.1093/nar/gkm445
Schindl, A., Hagen, M. L., Muzammal, S., Gunasekera, H. A., and Croft, A. K. (2019). Proteins in ionic liquids: reactions, applications and futures. Front. Chem. 7:347. doi: 10.3389/fchem.2019.00347
Schröder, C. (2017). Proteins in ionic liquids: current status of experiments and simulations. Top Curr Chem 375:25. doi: 10.1007/s41061-017-0110-2
Schwierz, N., Horinek, D., and Netz, R. R. (2013). Anionic and cationic Hofmeister effects on hydrophobic and hydrophilic surfaces. Langmuir 29, 2602–2614. doi: 10.1021/la303924e
Schwierz, N., Horinek, D., Sivan, U., and Netz, R. R. (2016). Reversed hofmeister series—the rule rather than the exception. Curr. Opin. Coll. Interf. Sci. 23, 10–18. doi: 10.1016/j.cocis.2016.04.003
Seduraman, A., Wu, P., and Klähn, M. (2012). Extraction of tryptophan with ionic liquids studied with molecular dynamics simulations. J. Phys. Chem. B 116, 296–304. doi: 10.1021/jp206748z
Senske, M., Constantinescu-Aruxandei, D., Havenith, M., Herrmann, C., Weingärtner, H., and Ebbinghaus, S. (2016). The temperature dependence of the Hofmeister series: thermodynamic fingerprints of cosolute–protein interactions. Phys. Chem. Chem. Phys. 18, 29698–29708. doi: 10.1039/C6CP05080H
Senske, M., Törk, L., Born, B., Havenith, M., Herrmann, C., and Ebbinghaus, S. (2014). Protein stabilization by macromolecular crowding through enthalpy rather than entropy. J. Am. Chem. Soc. 136, 9036–9041. doi: 10.1021/ja503205y
Sharma, A., and Ghorai, P. K. (2016). Effect of water on structure and dynamics of [BMIM][PF6] ionic liquid: an all-atom molecular dynamics simulation investigation. J. Chem. Phys. 144:114505. doi: 10.1063/1.4944083
Shi, Y., Liu, Y.-L., Lai, P.-Y., Tseng, M.-C., Tseng, M.-J., Li, Y., et al. (2012). Ionic liquids promote PCR amplification of DNA. Chem. Commun. 48, 5325–5327. doi: 10.1039/c2cc31740k
Silva, M. E., and Franco, T. T. (2000). Liquid-liquid extraction of biomolecules in downstream processing—a review paper. Braz. J. Chem. Eng. 17, 1–17. doi: 10.1590/S0104-66322000000100001
Simpson, W. S., and Crawshaw, G. H. (2002). Wool: Science and Technology. Cambridge; Boca Raton, FL: WOODHEAD Publishing; CRC Press.
Singh, N., Sharma, M., Mondal, D., Pereira, M. M., and Prasad, K. (2017). Very high concentration solubility and long-term stability of DNA in an ammonium-based ionic liquid: a suitable medium for nucleic acid packaging and preservation. ACS Sustain. Chem. Eng. 5, 1998–2005. doi: 10.1021/acssuschemeng.6b02842
Singh, O., Lee, P.-Y., Matysiak, S., and Bermudez, H. (2020). Dual mechanism of ionic liquid-induced protein unfolding. Phys. Chem. Chem. Phys. 22, 19779–19786. doi: 10.1039/D0CP03138K
Singh, T., Bharmoria, P., Morikawa, M.-a., Kimizuka, N., and Kumar, A. (2012). Ionic liquids induced structural changes of bovine serum albumin in aqueous media: a detailed physicochemical and spectroscopic study. J. Phys. Chem. B 116, 11924–11935. doi: 10.1021/jp303609h
Singh, U. K., Kumari, M., Khan, S. H., Bohidar, H. B., and Patel, R. (2018). Mechanism and dynamics of long-term stability of cytochrome c conferred by long-chain imidazolium ionic liquids at low concentration. ACS Sustain. Chem. Eng. 6, 803–815. doi: 10.1021/acssuschemeng.7b03168
Smiatek, J. (2017). Aqueous ionic liquids and their effects on protein structures: an overview on recent theoretical and experimental results. J. Phys. Condens. Matter. 29:233001. doi: 10.1088/1361-648X/aa6c9d
Smith, F. D., Harpending, P. R., and Sanford, J. C. (1992). Biolistic transformation of prokaryotes: factors that affect biolistic transformation of very small cells. J. Gen. Microbiol. 138, 239–248. doi: 10.1099/00221287-138-1-239
Soni, S. K., Sarkar, S., Mirzadeh, N., Selvakannan, P. R., and Bhargava, S. K. (2015). Self-assembled functional nanostructure of plasmid DNA with ionic liquid [Bmim][PF6]: enhanced efficiency in bacterial gene transformation. Langmuir 31, 4722–4732. doi: 10.1021/acs.langmuir.5b00402
Stock, F., Hoffmann, J., Ranke, J., Störmann, R., Ondruschka, B., and Jastorff, B. (2004). Effects of ionic liquids on the acetycholinesterase–a structure-activity relationship consideration. Green Chem. 6, 286–290. doi: 10.1039/B402348J
Stolte, S., Arning, J., Bottin-Weber, U., Matzke, M., Stock, F., Thiele, K., et al. (2006). Anion effects on the cytotoxicity of ionic liquids. Green Chem. 8, 621–629. doi: 10.1039/b602161a
Summers, C. A., and Flowers, R. A. II. (2000). Protein renaturation by the liquid organic salt ethylammonium nitrate. Protein Sci. 9, 2001–2008. doi: 10.1110/ps.9.10.2001
Sun, J., Liu, H., Yang, W., Chen, S., and Fu, S. (2017). Molecular mechanisms underlying inhibitory binding of alkylimidazolium ionic liquids to laccase. Molecules 22, 1353–1366. doi: 10.3390/molecules22081353
Taft, R. W., and Kamlet, M. J. (1976). The solvatochromic comparison method. 2. The α-scale of solvent hydrogen-bond donor (HBD) acidities. J. Am. Chem. Soc. 98, 2886–2894. doi: 10.1021/ja00426a036
Takekiyo, T., Fukudome, K., Yamazaki, K., Abe, H., and Yoshimura, Y. (2014). Protein aggregation and partial globular state in aqueous 1-alkyl-3-methylimidazolium nitrate solutions. Chem. Phys. Lett. 602, 22–27. doi: 10.1016/j.cplett.2014.03.089
Tan, S. C., and Yiap, B. C. (2009). DNA, RNA, and protein extraction: the past and the present. J. Biomed. Biotechnol. 2009, 574398. doi: 10.1155/2009/574398
Tarannum, A., Jonnalagadda, R. R., and Nishter, N. F. (2019). Stability of collagen in ionic liquids: ion specific hofmeister series effect. Spectrochim. Acta A 212, 343–348. doi: 10.1016/j.saa.2019.01.029
Tarannum, A., Rao, J. R., and Fathima, N. N. (2018). Choline-based amino acid ils-collagen interaction: enunciating its role in stabilization/destabilization phenomena. J. Phys. Chem. B 122, 1145–1151. doi: 10.1021/acs.jpcb.7b10645
Tateishi-Karimata, H., Nakano, M., Pramanik, S., Tanaka, S., and Sugimoto, N. (2015). i-Motifs are more stable than G-quadruplexes in a hydrated ionic liquid. Chem. Commun. 51, 6909–6912. doi: 10.1039/C5CC00666J
Tateishi-Karimata, H., Nakano, M., and Sugimoto, N. (2014). Comparable stability of Hoogsteen andWatson-Crick base pairs in ionic liquid choline dihydrogen phosphate. Sci. Rep. 4:3593. doi: 10.1038/srep03593
Tateishi-Karimata, H., and Sugimoto, N. (2012). A-T base pairs are more stable than G-C base pairs in a hydrated ionic liquid. Angew. Chem. Int. Ed. 51, 1416–1419. doi: 10.1002/anie.201106423
Tateishi-Karimata, H., and Sugimoto, N. (2014). Structure, stability and behaviour of nucleic acids in ionic liquids. Nucleic Acids Res. 42, 8831–8844. doi: 10.1093/nar/gku499
Tateishi-Karimata, H., and Sugimoto, N. (2018). Biological and nanotechnological applications using interactions between ionic liquids and nucleic acids. Biophys. Rev. 10, 931–940. doi: 10.1007/s12551-018-0422-7
Thomas, A. S., and Elcock, A. H. (2007). Molecular dynamics simulations of hydrophobic associations in aqueous salt solutions indicate a connection between water hydrogen bonding and the Hofmeister effect. J. Am. Chem. Soc. 129, 14887–14898. doi: 10.1021/ja073097z
Thuy Pham, T. P., Cho, C.-W., and Yun, Y.-S. (2010). Environmental fate and toxicity of ionic liquids: a review. Water Res. 44, 352–372. doi: 10.1016/j.watres.2009.09.030
Tomlinson, S. R., Kehr, C. W., Lopez, M. S., Schlup, J. R., and Anthony, J. L. (2014). Solubility of the corn protein zein in imidazolium-based ionic liquids. Ind. Eng. Chem. Res. 53, 2293–2298. doi: 10.1021/ie403659x
Van Der Bruggen, B., Vandecasteele, C., Van Gestel, T., Doyen, W., and Leysen, R. (2003). A review of pressure-driven membrane processes in wastewater treatment and drinking water production. Environ. Prog. 22, 46–56. doi: 10.1002/ep.670220116
van Die, I. M., Bergmans, H. E. N., and Hoekstra, W. P. M. (1983). Transformation in Escherichia coli: Studies on the role of the heat shock in induction of competence. J. Gen. Microbiol. 129, 663–670. doi: 10.1099/00221287-129-3-663
van Rantwijk, F., and Sheldon, R. A. (2007). Biocatalysis in ionic liquids. Chem. Rev. 107, 2757–2785. doi: 10.1021/cr050946x
Ventura, S. P. M., Neves, C. M. S. S., Freire, M. G., Marrucho, I. M., Oliveira, J., and Coutinho, J. A. P. (2009). Evaluation of anion influence on the formation and extraction capacity of ionic liquid-based aqueous biphasic systems. J. Phys. Chem. B 113, 9304–9310. doi: 10.1021/jp903286d
Vicent-Luna, J. M., Romero-Enrique, J. M., Calero, S., and Anta, J. A. (2017). Micelle formation in aqueous solutions of room temperature ionic liquids: a molecular dynamics study. J. Phys. Chem. B 121, 8348–8358. doi: 10.1021/acs.jpcb.7b05552
Vijayanathan, V., Thomas, T., and Thomas, T. J. (2002). DNA nanoparticles and development of DNA delivery vehicles for gene therapy. Biochemistry 41, 14085–14094. doi: 10.1021/bi0203987
Vijayaraghavan, R., Izgorodin, A., Ganesh, V., Surianarayanan, M., and MacFarlane, D. R. (2010). Long-term structural and chemical stability of DNA in hydrated ionic liquids. Angew. Chem. Int. Ed. 49, 1631–1633. doi: 10.1002/anie.200906610
Von Hippel, P. H., and Wong, K.-Y. (1964). Neutral salts: the generality of their effects on the stability of macromolecular conformations. Science 145, 577–580. doi: 10.1126/science.145.3632.577
Vrikkis, R. M., Fraser, K. J., Fujita, K., MacFarlane, D. R., and Elliott, G. D. (2009). Biocompatible ionic liquids: a new approach for stabilizing proteins in liquid formulation. J. Biomech. Eng. 131, 74514–74517. doi: 10.1115/1.3156810
Wakayama, R., Uchiyama, S., and Hall, D. (2019). Ionic liquids and protein folding—old tricks for new solvents. Biophys. Rev. 11, 209–225. doi: 10.1007/s12551-019-00509-2
Wang, J.-H., Cheng, D.-H., Chen, X.-W., Du, Z., and Fang, Z.-L. (2007). Direct extraction of double-stranded DNA Into ionic liquid 1-butyl-3-methylimidazolium hexafluorophosphate and its quantification. Anal. Chem. 79, 620–625. doi: 10.1021/ac061145c
Weingärtner, H. (2008). Understanding ionic liquids at the molecular level: facts, problems, and controversies. Angew. Chem. Int. Ed. 47, 654–670. doi: 10.1002/anie.200604951
Weingärtner, H., Cabrele, C., and Herrmann, C. (2012). How ionic liquids can help to stabilize native proteins. Phys. Chem. Chem. Phys. 14, 415–426. doi: 10.1039/C1CP21947B
Welton, T. (1999). Room-temperature ionic liquids. Solvents for synthesis and catalysis. Chem. Rev. 99, 2071–2083. doi: 10.1021/cr980032t
Wilkes, J. S., and Zaworotko, M. J. (1992). Air and water stable 1-ethyl-3-methylimidazolium based ionic liquids. J. Chem. Soc. Chem. Commun. 965–967. doi: 10.1039/c39920000965
Wittwer, C. T. (2009). High-resolution DNA melting analysis: advancements and limitations. Hum. Mutat. 30, 857–859. doi: 10.1002/humu.20951
Xu, Y., and Wang, E. (2009). Ionic liquids used in and analyzed by capillary and microchip electrophoresis. J. Chromatogr. A 1216, 4817–4823. doi: 10.1016/j.chroma.2009.04.024
Yakovchuk, P., Protozanova, E., and Frank-Kamenetskii, M. D. (2006). Base-stacking and base-pairing contributions into thermal stability of the DNA double helix. Nucl. Acids Res. 34, 564–574. doi: 10.1093/nar/gkj454
Yamamoto, E., Yamaguchi, S., and Nagamune, T. (2011). Protein refolding by N-alkylpyridinium and N-alkyl-N-methylpyrrolidinium ionic liquids. Appl. Biochem. Biotechnol. 164, 957–967. doi: 10.1007/s12010-011-9187-1
Yancey, P. H., Clark, M. E., Hand, S. C., Bowlus, R. D., and Somero, G. N. (1982). Living with water stress: evolution of osmolyte systems. Science 217, 1214–1222. doi: 10.1126/science.7112124
Yang, Z. (2009). Hofmeister effects: an explanation for the impact of ionic liquids on Biocatalysis. J. Biotechnol. 144, 12–22. doi: 10.1016/j.jbiotec.2009.04.011
Zaks, A., and Klibanov, A. M. (1988). The effect of water on enzyme action in organic media. J. Biol. Chem. 263, 8017–8021.
Zangi, R. (2010). Can salting-in/salting-out ions be classified as chaotropes/kosmotropes? J. Phys. Chem. B 114, 643–650. doi: 10.1021/jp909034c
Zangi, R., Hagen, M., and Berne, B. J. (2007). Effect of ions on the hydrophobic interaction between two plates. J. Am. Chem. Soc. 129, 4678–4686. doi: 10.1021/ja068305m
Zhang, Y., Chen, X., Lan, J., You, J., and Chen, L. (2009). Synthesis and biological applications of imidazolium-based polymerized ionic liquid as a gene delivery vector. Chem. Biol. Drug. Des. 74, 282–288. doi: 10.1111/j.1747-0285.2009.00858.x
Zhang, Y., and Cremer, P. S. (2006). Interactions between macromolecules and ions: the Hofmeister series. Curr. Opin. Chem. Biol. 10, 658–663. doi: 10.1016/j.cbpa.2006.09.020
Zhang, Y. J., Furyk, S., Bergbreiter, D. E., and Cremer, P. S. (2005). Specific ion effects on the water solubility of macromolecules: PNIPAM and the Hofmeister series. J. Am. Chem. Soc. 127, 14505–14510. doi: 10.1021/ja0546424
Zhao, H. (2005). Effect of ions and other compatible solutes on enzyme activity, and its implication for biocatalysis using ionic liquids. J. Mol. Catal. B Enzym. 37, 16–25. doi: 10.1016/j.molcatb.2005.08.007
Zhao, H. (2006). Are ionic liquids kosmotropic or chaotropic? An evaluation of available thermodynamic parameters for quantifying the ion kosmotropicity of ionic liquids. J. Chem. Technol. Biotechnol. 81, 877–891. doi: 10.1002/jctb.1449
Zhao, H. (2015). DNA stability in ionic liquids and deep eutectic solvents. J. Chem. Technol. Biotechnol. 90, 19–25. doi: 10.1002/jctb.4511
Zhao, H., Campbell, S. M., Jackson, L., Song, Z., and Olubajo, O. (2006). Hofmeister series of ionic liquids: kosmotropic effect of ionic liquids on the enzymatic hydrolysis of enantiomericphenylalanine methyl ester. Tetrahedron 17, 377–383. doi: 10.1016/j.tetasy.2006.01.015
Keywords: ionic liquid (IL), DNA, protein, Hofmeister series, intermolecular interaction, circular dichroism, double-helical structure, salting phenomenon
Citation: Shukla SK and Mikkola J-P (2020) Use of Ionic Liquids in Protein and DNA Chemistry. Front. Chem. 8:598662. doi: 10.3389/fchem.2020.598662
Received: 25 August 2020; Accepted: 01 December 2020;
Published: 23 December 2020.
Edited by:
Janno Torop, University of Tartu, EstoniaReviewed by:
Itthipon Jeerapan, Prince of Songkla University, ThailandJens Smiatek, University of Stuttgart, Germany
Ramesh L. Gardas, Indian Institute of Technology Madras, India
Copyright © 2020 Shukla and Mikkola. This is an open-access article distributed under the terms of the Creative Commons Attribution License (CC BY). The use, distribution or reproduction in other forums is permitted, provided the original author(s) and the copyright owner(s) are credited and that the original publication in this journal is cited, in accordance with accepted academic practice. No use, distribution or reproduction is permitted which does not comply with these terms.
*Correspondence: Shashi Kant Shukla, c2hhc2hpLmthbnQuc2h1a2xhJiN4MDAwNDA7dW11LnNl; c2hhc2kyNzEyODMmI3gwMDA0MDtnbWFpbC5jb20=; Jyri-Pekka Mikkola, anlyaS1wZWtrYS5taWtrb2xhJiN4MDAwNDA7dW11LnNl