- Faculty of Chemistry, Jagiellonian University, Kraków, Poland
Air pollution is associated with numerous negative effects on human health. The toxicity of organic components of air pollution is well-recognized, while the impact of their inorganic counterparts in the overall toxicity is still a matter of various discussions. The influence of airborne particulate matter (PM) and their inorganic components on biological function of human alveolar-like epithelial cells (A549) was investigated in vitro. A novel treatment protocol based on covering culture plates with PM allowed increasing the studied pollutant concentrations and prolonging their incubation time without cell exposure on physical suffocation and mechanical disturbance. PM decreased the viability of A549 cells and disrupted their mitochondrial membrane potential and calcium homeostasis. For the first time, the difference in the reactive oxygen species (ROS) profiles generated by organic and inorganic counterparts of PM was shown. Singlet oxygen generation was observed only after treatment of cells with inorganic fraction of PM, while hydrogen peroxide, hydroxyl radical, and superoxide anion radical were induced after exposure of A549 cells to both PM and their inorganic fraction.
Introduction
Poor air quality is one of the global health problems, since it affects around 91% of the world's population (World Health Organization) (http://www.who.int). Numerous reports confirm that exposure to particulate matter (PM) increases the risks of respiratory tract, cardiovascular, immunological, and other diseases (Araujo, 2011; Selmi et al., 2012; Kelly and Fussell, 2017; Zhang et al., 2018; Shen and Lung, 2020; Yang et al., 2020; Wang et al., 2020a). Despite the fact that the health effects of air pollution have been recognized since the last century, the progress in research is still not satisfied and it might arise from the difficulty to reliably assess the impact of air pollutants on human health. The effects of chronic exposure are usually observed only after several or even dozens of years, while acute contact often does not result in detectable biological alterations (Bräuner et al., 2007; Han et al., 2020). The bio-accumulative nature of pollutions makes it possible to reach up the threshold level that is harmful to human health (Ali et al., 2019) and safe levels of exposure are difficult to establish (Liu et al., 2018). Therefore, small constantly applied disturbances to cellular homeostasis can lead to pathological changes and result in the development of various diseases.
Air pollution consists of multi-component and multi-phase chemical systems that include traces of transition and main group metals and compounds (minerals, carbonaceous species, inorganic ions), various gaseous components (O2, O3, NOx, HS−/H2S, CO, CO2, SO2), and organic contaminations [polycyclic aromatic hydrocarbons (PAHs), chlorinated pesticides, quinones, and biological materials such as viruses, endotoxins, cell fragments]. All components are constantly interacting in gas–liquid, liquid–solid, and gas–liquid–solid interfaces. The health problems originating from air pollution are mainly linked to PM of <0.1 and 2.5 μm in diameter (denoted as PM0.1 and PM2.5, respectively), which can easily penetrate the respiratory tract and accumulate more efficiently (Gangwar et al., 2020; Leikauf et al., 2020; Manigrasso et al., 2020; Novák et al., 2020). PM is a complex mixture of particles with a wide range of size and diverse composition, depending on the place and time of sample collection (Shao et al., 2018). The natural sources contributing to high PM emission are windblown dust, volcano eruptions, wildfires, and sea salt aerosols, while industrial, automobile, and construction/demolition sectors are the major anthropogenic contributors to air pollution (Ali et al., 2019). Numerous worldwide government health regulations aimed at the improvement of air quality have shifted PM emission contribution from different sources, for example, from being dominated by coal burning to a mix of vehicle and stationary combustion emissions (Shao et al., 2018). Each PM source generates several potential toxic elements. Such large heterogeneity of the naturally collected PM samples can impede the evaluation of the biological effects of PM and obstruct establishing of underlying toxicological mechanisms. Nowadays, standard reference materials for urban air pollutant are commercially available and widely used as the reference samples in various biological and chemical studies (Park et al., 2013; Courtois et al., 2014; Jiang et al., 2014; Lee et al., 2016; O'Driscoll et al., 2019; Dijkhoff et al., 2020).
In our work, we are focusing on the evaluation of the impact of the inorganic fraction of PM (in particular redox-active transition metal elements) on cells by using the standard reference material for urban air pollutants deprived of organic components. The toxicity of organic compounds is usually based on specific interaction with biomolecules and is widely described in the literature (Hanzalova et al., 2010; Oh et al., 2011; Falco et al., 2017; Qi et al., 2020; Wang et al., 2020b). Among the most hazardous chemical compounds are PAHs, which have carcinogenic, mutagenic, and/or teratogenic effects. They are lipophilic and can easily penetrate tissues, interacting with different enzymes (such as cytochrome P450), producing reactive toxic metabolites and genotoxic DNA adducts, and activating different biological pathways (Baulig et al., 2003; Chew et al., 2020; Longhin et al., 2020). The activity of inorganic PM is predominantly based on its redox chemistry and ability to induce oxidative stress (Kasprzak, 2002; Kawanishi et al., 2002; Visalli et al., 2015; Cui et al., 2019; Haghani et al., 2020; Pardo et al., 2020; Samet et al., 2020). Several studies were performed to establish an association between toxic effects of air pollution and transition metal components of PM such as Fe, V, Cr, Cu, and Zn (Ali et al., 2019; Valacchi et al., 2020). In our opinion, greater attention should be paid to the inorganic components, since they can catalyze different oxidation reactions, whereas organic pollutants can produce reactive oxygen species (ROS) mainly in a stoichiometric way (Risom et al., 2005; Andersson et al., 2009). Different mechanisms of pathological cellular response induced by organic and inorganic fractions of PM are postulated, and serious debate on their roles has been going on in the scientific community in recent years (Stone et al., 2003; Lu et al., 2014; Huang et al., 2015; Kim et al., 2018; Badran et al., 2020). Several reports disclosed the predominant role of organic pollutants due to their ability to induce a pro-inflammatory response in murine macrophages or bronchial epithelial cells (Baulig et al., 2003, 2009; Billet et al., 2007; Gawda et al., 2018; Wang et al., 2018). Alternatively, there are data that indicate the limited importance of endotoxin and organic fraction of air pollutants on inflammatory response to PM, suggesting the involvement of other components of PM, which need to be identified (Herseth et al., 2017). Furthermore, synergistic and antagonistic interactions between PM components could significantly alter their redox properties (Gao et al., 2020). Significant discrepancy in the current literature state of knowledge might be related to the different experimental protocols applied to the performed research. The studied air pollutant samples varied by not only chemical/physicochemical characteristics (different organic and inorganic contents, particle size, zeta potential, and others) but also different experimental models, exposure time, and concentrations used. Hence, direct comparison of the results received for the inorganic and organic PM fractions is challenging.
To improve our knowledge on the direct mechanisms involved in PM-induced toxicity with a particular interest on the role of inorganic components, the influence of PM on type II human alveolar-like epithelial cell line A549 was analyzed in vitro. Several biological parameters such as cells' viability and mechanism of cellular death as well as ROS production, mitochondrial potential, and cytosolic calcium homeostasis were evaluated after exposure to PM samples, both intact (inorganic and organic PM fractions) and plasma treated (mostly inorganic fraction). Additionally to standard exposure protocol involving cell treatment with a suspension of PM, the new protocol based on covering culture plates with PM and coating them with adhesion proteins was applied (Scheme 1). Limited contact of the biggest particles (microparticles) of PM with cells allowed the avoidance of mechanical disturbance of cells. This approach enables a focus on the evaluation of the impact of PM as well as its dissolved compounds on cells without additional contribution from dying physically disturbed cells. Particular attention was paid to use small non-toxic concentrations of PM to avoid false-positive results caused by a fraction of dead cells as well as fluorescent probe oxidation caused by PM.
Materials and Methods
Sample Preparation
Urban PM sample SRM 1648a (encoded as SRM) was purchased from the National Institute of Standards and Technology (USA). This sample is composed of PM collected in the St. Louis, MO, area over a 1-year period (1976–1977). Among organic constituents, SRM contained PAHs, nitro-substituted PAHs, polychlorinated biphenyls (PCBs), and chlorinated pesticides. Analysis of the inorganic fraction revealed, among others, components containing Fe, Mn, Al, Ca, Cr, Ti, Pb, Mg, and others. The sample was fully characterized by numerous analytical techniques, and its specification is available in the manufacturer's certificate. The particle size varies from 0.2 to over 100 μm, with the predominance of particles with a hydrodynamic diameter of 5–20 μm.
The Plasma Zepto system (Diener Electronic GmbH) was used for the removal of organic compounds present in the SRM sample. Low-temperature plasma treatment is a well-known technique for the elimination of organic contamination, which are oxidized, converted into volatile products, and sucked by pump. Samples (17–20 mg) were treated with a low-temperature plasma for 120 min at 100 W power. Content of carbon was determined by the elementary analysis (Ewlementar, Vario Micro Cube) and total organic carbon (TOC) analyzer (Schimadzu, TOC-V series). Upon plasma treatment, the carbon content decreased from 14% in the SRM sample to 1.8% in the plasma-treated sample (encoded as ΔC-SRM) (Mikrut et al., 2018). Plasma treatment did not induce significant changes in the sample morphology or aggregation (Mikrut et al., 2018).
PM samples were weighed on a high-precision microbalance, and stock suspensions were sonicated for a few minutes before use. Fresh suspensions were prepared before each experiment.
Cell Culture Conditions
Biological studies were performed using type II human alveolar-like epithelial cell line A549. Cells were maintained in Dulbecco's modified Eagle's medium (DMEM) supplemented with 10% fetal bovine serum (FBS) and 1% antibiotics (penicillin 100 units/ml, streptomycin 100 μg/ml) and were routinely cultured at 37°C in a humidified incubator in a 5% CO2 atmosphere. Cells were seeded on unmodified plates 24 h before performing the experiments, typically with a density of 3 × 104 cells per cm2. If not stated otherwise, cells were exposed to PM for 24 h (added as a suspension in medium), followed by washing with phosphate buffered saline (PBS) (exposure method 1, Scheme 1). After that, an appropriated test was applied. Alternatively, cells were seeded on plates that were pre-prepared by covering them with PM samples (as described in the following paragraph) and kept for 24 h (exposure method 2, Scheme 1). All experiments were performed at least in triplicate and repeated three times. The mean values ± standard error of the mean (SEM) were calculated.
Covering Plates With Particulate Matter Samples (Exposure Method 2)
To cover the plates with PM samples, SRM, or ΔC-SRM was suspended in cold methanol and added to the wells to achieve different concentrations up to 625 μg/cm2. After methanol evaporation, some plates were additionally coated with fibronectin from human plasma or collagen I from calf skin (Sigma). Fibronectin and collagen were diluted to 25 μg/ml with sterile water, and 50-μl solutions were added to the wells of 96-well-plates. Plates were kept at 4°C overnight, and then they were directly used for seeding cells.
Cell Viability
The evaluation of the A549 cell viability after exposure to PM samples was conducted using 3-[4,5-dimethylthiazol-2-yl]-2,5-diphenyltetrazolium bromide (MTT) or resazurin assays. The MTT assay is based on the reduction of yellow tetrazolium salt (MTT) by dehydrogenases of metabolically active cells into purple water-insoluble formazan dye. The concentration of dye determined spectrophotometrically after dissolution in an organic solvent is proportional to the number of live cells. Cell viability was quantified measuring absorbance at 565 nm using 700 nm as a reference wavelength (Tecan Infinite 200 microplate reader). Resazurin test is based on the reduction of blue and non-fluorescent substrate (resazurin) to a pink and highly fluorescent product (resorufin) by the live cells. Cell viability was quantified at 605 nm using 560-nm excitation light (Tecan Infinite 200 microplate reader). Experiments were performed in triplicate and repeated at least three times to get the mean values ± SEM. The viability was calculated with respect to the control seeded on uncoated/coated plate without PM samples. The viability was determined after incubation of cells with PM samples prepared as suspension in a medium [without or with 2/10% fetal bovine serum (FBS)] at concentrations up to 500 μg/ml (that refers to 156.25 μg/cm2) for 24 or 72 h. Alternatively, viability was assessed after exposing cells to PM samples covering plates at the concentrations up to 625 μg/cm2 for 24 h.
Evaluation of Reactive Oxygen Species Generation by A549 Cells
Total Reactive Oxygen Species Production
To evaluate the total ROS production induced in cells after incubation with non-toxic concentrations of PM, the cyto-ID Hypoxia/Oxidative stress detection kit (Enzo Life Sciences, USA) was used according to the manufacturer's protocols. A549 cells were seeded with a density of 2 × 104 cells per cm2. A day later, the SRM or ΔC-SRM samples at different concentrations suspended in medium without serum were added and incubated in the dark for 24 h. Then, cells were washed with PBS, treated with trypsin, and analyzed by BD FACSVerse cytometer. As a positive control, pyocyanin (300 μM) was used. The level of the oxidative stress was determined as a percentage of the ROS-positive cells of the whole-cell population determined by pyocyanin control. Alternatively, mean fluorescent intensity of the stained treated cells was evaluated.
To mimic the chronic exposure of A549 cells to PM samples, cells were cultured for 18 days. Firstly, cells were seeded with a density of 2 × 104 cells per cm2 on a six-well-plate. Next day, the SRM or ΔC-SRM samples at different concentrations suspended in medium with 2% FBS were added. Cells were incubated with pollutants for 3 days and were reseeded in 1:5 ratio to new plates. The freshly prepared suspensions of PM were added. Cells were incubated in total for 18 days and, every 3 days, cells were reseeded and new suspensions of PM were added. On the 18th day of incubation, A549 cells were washed with PBS, stained with cyto-ID Hypoxia/Oxidative stress detection kit according to the manufacturer's protocols, treated with trypsin, and analyzed by BD FACSVerse cytometer.
To evaluate the influence of PM on the oxidative stress induced by other stimuli, A549 cells were pretreated with PM samples. For this purpose, A549 cells were seeded on a six-well-plate with a density of 2 × 104 cells per cm2 a day before. The suspension of SRM or ΔC-SRM samples at different concentrations in medium without serum was added. After 24 h of incubation, cells were washed with PBS and pyocyanin (300 μM) was used for 30 min to induce oxidative stress. A549 cells were then stained with cyto-ID Hypoxia/Oxidative stress detection kit according to the manufacturer's protocols and analyzed by BD FACSVerse cytometer.
2′,7′-Dichlorodihydrofluorescein Diacetate
2′,7′-Dichlorodihydrofluorescein diacetate (DCF-DA) (Sigma-Aldrich) was used to assess the general ROS production by cells exposed to PM samples at non-toxic concentrations. Cells were stained with DCF-DA (20 μM) for 45 min at 37°C. After the staining, the ROS indicator was washed out with PBS, and fluorescence of cells was quantified by a Tecan Infinite 200 plate reader at 535 nm using 485 nm as an excitation wavelength. Control experiments with identical settings but without cells were made to determine the influence of PM on the dye.
Qualitative Analysis of the Produced Reactive Oxygen Species
Various fluorescent probes, specific to the selected ROS, were used to determine the type of ROS produced by cells after treatment with SRM or ΔC-SRM samples. Each probe was applied for cells exposed to PM-covered surfaces at two non-toxic concentrations, 50 and 100 μg/cm2. After the staining, the ROS indicators were washed twice with PBS, and fluorescence of cells was quantified by a Tecan Infinite 200 plate reader. Controls without cells were made to avoid false-positive results and verify the influence of PM on the used fluorescent probes.
Singlet oxygen sensor green (SOSG, 5 μM, 20 min; ThermoFisher Scientific) was used to assess the production of singlet oxygen in cells. Aminophenyl fluorescein (APF, 5 μM, 30 min, ENZO) was used to evaluate the production of hydroxyl radical, hypochlorite, or peroxynitrite. Dihydrorhodamine 123 (DhR123, 10 μM, 20 min, AAT Bioquest) was used to estimate the production of mainly hydrogen peroxide; however, it can be used to detect additionally hypochlorite, peroxynitrite, or cytochrome c. Hydroethidium (HE, 10 μM, 20 min, AAT Bioquest) was used to check the production of superoxide anion radical. MitoSox Red (5 μM, 10 min, ThermoFisher Scientific) was applied to evaluate the production of superoxide anion radical in mitochondria. Staining cells with probes was performed at 37°C. Fluorescence intensity of cells was measured at 535 nm using 485 nm as an excitation wavelength for SOSG/APF and at 529 nm using 507 nm as an excitation wavelength for DhR123. For HE evaluation, fluorescence intensity of cells was quantified at 605 nm using 520 nm as an excitation wavelength, while 510/595 nm were used as excitation/emission wavelengths for MitoSox Red quantification.
Lipid Peroxidation
Lipid peroxidation was assessed using C11-BODIPY581/591-Lipid Peroxidation Sensor (ThermoFisher Scientific). A549 cells were seeded on PM-covered surfaces at two concentrations, 50 and 100 μg/cm2, 24 h before the experiment. After that, cells were stained with C11-BODIPY581/591 (1 μM) for 30 min in the dark at 37°C. The fluorescence intensity of cells was measured at 484/510 nm (green) and 581/610 nm (red)—the excitation/emission wavelengths.
Mitochondrial Membrane Potential
Mitochondrial membrane potential (ΔΨm) was evaluated using JC-1 probe (AAT Bioquest). The JC-1 probe is a lipophilic cationic dye that exhibits potential-dependent accumulation in the mitochondria. At low ΔΨm, the probe exists as a monomer that emits green fluorescence. At higher ΔΨm, the concentration of dye increases and the probe forms J-aggregates that lead to a shift in fluorescence emission from green to red. A change from red to green fluorescence reflects a decrease in ΔΨm. A549 cells were exposed to PM-covered plates at two concentrations, 50 and 100 μg/cm2. After 24 h of treatment, cells were stained with JC-1 (10 μM) for 30 min in the dark at 37°C. The fluorescence intensity of cells was measured at 525 and 590 nm using 490 nm as an excitation wavelength.
Cytosolic Calcium Homeostasis
Cytosolic calcium concentration was measured using Fluo-8 AM probe (AAT Bioquest). A549 cells were exposed to PM-covered plates at two concentrations, 50 and 100 μg/cm2. After 24 h of treatment, cells were stained with Fluo-8 AM (4 μM) for 30 min in the dark at 37°C. The fluorescence intensity of cells was measured at 525 nm using 490 nm as an excitation wavelength.
The Mechanism of Cellular Death
The mechanism of cellular death was evaluated using Annexin V-fluorescein isothiocyanate (FITC)/propidium iodide (PI) (ThermoFisher Scientific) assay. The phosphatidylserine of the cytoplasmic membrane at the early stage of apoptosis flipped on the outer surface of cell membrane and in the presence of calcium ions is bound with Annexin V. PI was used to assess the necrotic population of cells. A549 cells were seeded on PM-covered plates with a density of 4 × 104 cells per cm2, and 24 h after the seeding, the test was performed. A549 cells were stained with Annexin V-FITC for 10 min in the dark and then with PI (0.5 μM) for 5 min. Cells were analyzed by a BD FACSVerse cytometer.
Caspase Activity
Activation of caspases 3/7 was examined using CellEvent Caspase-3/7 Green Detection Reagent (ThermoFisher Scientific). A549 cells were seeded on PM-covered plates with a density of 4 × 104 cells per cm2. Twenty-four hours later, cells were stained with CellEvent Caspase-3/7 Green Detection Reagent. The fluorescence intensity of cells was analyzed using a BD FACSVerse cytometer.
Statistical Analysis
For in vitro experiments, all data were expressed as the mean ± standard error of the mean (SEM). All the experiments were performed in triplicate and repeated at least three times. Significant differences among groups were determined using t-test or a one-way analysis of variance (ANOVA) using OriginPro 2018 software. Probabilities of p < 0.05 were considered as statistically significant. The following notification is used *p < 0.05, **p < 0.001.
Results and Discussion
For the evaluation of biological effect of PM with and without organic components in vitro, A549 cell line was used. This line was chosen as a simple model of lung epithelial cell line as has been routinely used for this type of studies (Billet et al., 2007; Mehta et al., 2008; Wang et al., 2013; Huang et al., 2014, 2015; Lee et al., 2014; Lu et al., 2014; Schilirò et al., 2015; Kim et al., 2018; Li et al., 2020). SRM 1648a sample (SRM) supplied by the National Institute of Standards and Technology, USA, was used as a standardized sample for urban air pollutants. Sample without organic contaminations (ΔC-SRM) was prepared using low-temperature plasma treatment during which the organic carbon content decreased from 14 to 1.8%, as determined by both elementary analysis and TOC measurements. Neither significant changes in the morphology nor aggregation of particles due to plasma treatment was observed (Mikrut et al., 2018). Two different exposure protocols were used to fully assess the influence of PM on biological parameters of A549 cells: i) suspension of PM in cell culture medium (exposure method 1) and ii) PM-covered plates (exposure method 2).
Cell Viability
The viability of the A549 cells after exposure to SRM and ΔC-SRM samples was assessed using MTT or resazurin tests. It was determined that after 24 h of incubation with either SRM or ΔC-SRM samples suspended in medium, the viability of A549 cells decreased significantly for the concentrations higher than 100 μg/ml (Supplementary Figure 1A). These results are in agreement with other reports (Holian et al., 1998; Gawda et al., 2018). No difference in toxicity between SRM and ΔC-SRM was observed for 24 h of incubation, while after 72 h, SRM sample appeared to be more toxic (Supplementary Figure 1B). Prolonging the incubation time in case of ΔC-SRM sample did not induce additional toxicity. Serum proteins may have substantial impact on the toxicity of numerous compounds by either reducing the toxicity due to the lower accumulation of compounds or increasing the toxicity by facilitating the uptake (Mazuryk et al., 2014). Therefore, the influence of fetal bovine serum (FBS) presence in the incubation medium was studied by performing the viability assay additionally in the presence of 2 or 10% FBS. It was determined that FBS has a negligible impact on the toxicity of SRM sample (results are not shown).
The decrease in viability of the A549 cells after the incubation with SRM or ΔC-SRM samples may not be solemnly caused by chemical nature of PM interaction with cells. Additional factors related to the physical contact and mechanical disturbance of cells by PM sample should be considered (Supplementary Figure 2). PM applied directly on cells, covering them that can efficiently reduce the amount of nutrients and generate mechanical damage, which in consequence may induce cell death.
To eliminate this effect, plates were covered with PM samples at different concentrations and then used for cell seeding (see experimental part). Additionally, some plates covered with PM samples were coated with collagen or fibronectin prior to seeding of cells. Plates prepared in such a way gave cells the ability to interact with PM without limiting a proficient collection of nutrients from cell medium. Covering plates with PM samples and coating them with adhesion proteins next significantly reduced the toxicity of SRM or ΔC-SRM samples (Supplementary Figure 3). No toxicity of PM samples was observed in cells seeded on uncoated plates or plates coated with collagen, while slight toxicity (up to 80% viability) was observed on the surface coated with fibronectin. PM covering technique allowed the use of 20 times more concentrated PM samples (100 μg/ml = 31.25 μg/cm2) as a nontoxic dosage.
Attention should be paid to morphological changes of A549 cells upon contact with PM (Supplementary Figure 4). Flow cytometry results revealed only a slight decrease in cell size (FSC) while cell granularity parameter (SSC) increased. This indicates that PM was collected by cells. Plasma-treated and untreated PM samples induced a similar effect on the SSC parameter.
Evaluation of Reactive Oxygen Species
The generation of ROS in A549 cells upon interaction with PM samples was explored using two different methods of contact between cells and PM. The samples were applied either in the form of suspension in medium or as a layer covering the plate.
ROS production was assessed using small non-toxic concentrations of PM to avoid false-positive results caused by cells' response to toxic effect of PM or PM interference with fluorescent probes. Total ROS production in A549 cells after different incubation times was evaluated using cyto-ID Hypoxia/Oxidative stress detection kit. One hour of incubation with SRM suspension was not a sufficient period of time to produce a significant amount of ROS by cells (Supplementary Figure 5). However, treatment with suspension of inorganic part of pollutants (ΔC-SRM sample) was able to cause a detectable increase in the amount of ROS produced by A549 cells even after 1 h of incubation (Supplementary Figure 5). Twenty-four hours of cell exposure to PM suspensions resulted in an increase in ROS-positive cell population for both applied PM samples, SRM and ΔC-SRM (Figure 1A). The SRM sample induced a stronger effect, confirming relevance of organic pollutants in oxidative stress induction. Semi-chronic exposure (18 days) to PM suspensions caused a different effect (Figure 1B). Incubation of A549 cells with SRM sample did not result in an increase in ROS production, indicating an existence of a compensatory mechanism toward constant small exposure to pollutants. Some of the organic pollutants have ROS-scavenging properties, and they may act as antioxidants. In contrast, ΔC-SRM induced a significant rise in the amount of generated ROS in cells, fairly independent of the used concentration of the sample, suggesting a catalytic mechanism of ROS production. High content of metals such as Fe, Cu, and Mn in ΔC-SRM can participate in catalytic production of ROS.
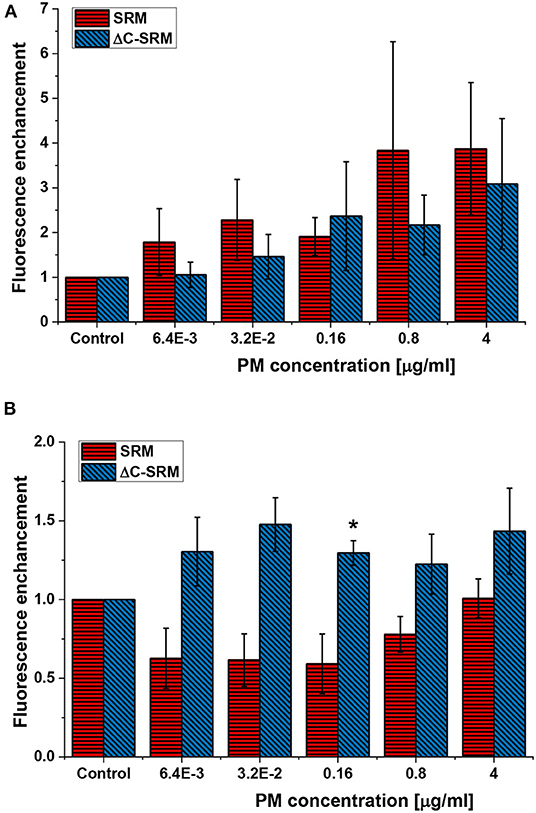
Figure 1. The reactive oxygen species (ROS) production in A549 cells after their exposure to suspensions of SRM or ΔC-SRM for 24 h (A) and 18 days (B) was evaluated using cyto-ID Hypoxia/Oxidative stress detection kit (exposure method 1), *p < 0.05.
To further evaluate the influence of PM on oxidative stress induction, the production of ROS in cells pretreated with PM suspensions (SRM or ΔC-SRM) and exposed to a known ROS inducer was studied. As a ROS inducer, pyocyanin was used, a toxin produced by Pseudomonas aeruginosa (Ando and Yonamoto, 2015; Winter and Zychlinsky, 2018). Pretreatment of cells with PM suspensions for 24 h before exposing them to pyocyanin resulted in a higher response to the used stimuli (Figure 2). Pretreatment with SRM resulted in a strong dose-dependent increase in ROS production upon exposure to pyocyanin, while pretreatment with ΔC-SRM induced a similar effect but independent of concentration. The results are in agreement with literature data, which showed that exposure of murine macrophages to suspension containing low concentrations of PM may prime cells to a hyper-inflammatory response upon contact with the second stimulus (Gawda et al., 2018).
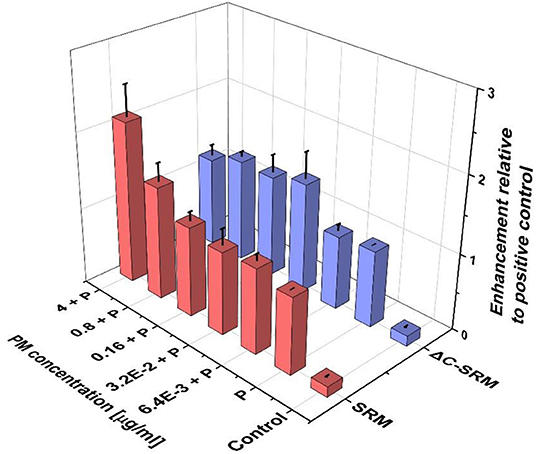
Figure 2. The reactive oxygen species (ROS) production in A549 cells pretreated with particulate matter (PM) suspensions for 24 h and subsequently exposed to pyocyanin (P) was evaluated using cyto-ID Hypoxia/Oxidative stress detection kit (exposure method 1).
In numerous publications, the production of ROS was evaluated using DCF-DA assay (Wilson et al., 2002; Baulig et al., 2004, 2009; Andersson et al., 2009; Lee et al., 2014, 2016; Rodríguez-Cotto et al., 2015; Ahlberg et al., 2016). DCF-DA is a cellular probe that reacts with numerous ROS to produce fluorescent product and is often used to estimate the general ROS production. The level of oxidative stress in A549 cells was measured by this probe after 24- or 72-h incubation with PM suspension. No significant ROS production in A549 cells using DCF-DA probe was observed after 24 h of incubation for each of the samples (Supplementary Figure 6A). Prolonging incubation time up to 72 h resulted only in a slight increase in the level of oxidative stress (Supplementary Figure 6B). Such discrepancy in the results between two tests used for the evaluation of ROS production can be explained by different sensitivities of the probes, since DCF-DA is mostly sensitive toward H2O2, while cyto-ID Hypoxia/Oxidative stress detection kit detects general ROS production (according to the manufacturer's protocol). The amount of ROS generated by suspension of PM may not be high enough to be detected by DCF-DA probe. Further increase in PM concentration was not possible due to its toxicity (Supplementary Figure 1). Additionally, PM concentration higher than 20 μg/ml (for SRM sample) and 10 μg/ml (for ΔC-SRM sample) interfered with DCF-DA assay, causing its oxidation.
To overcome the mentioned problems for further studies, the PM covering technique was used. Covering plates with PM samples and coating them with adhesion proteins resulted in a significant decrease of toxicity of air pollutants (Supplementary Figure 3); therefore, the higher amount of PM samples, still in nontoxic range of concentration, can be used in the evaluation of ROS production in A549 cells by using DCF-DA assay. The production of ROS in A549 cells seeded on the surface covered with PM samples and coated with either fibronectin or collagen is shown in Figure 3. Both SRM and ΔC-SRM samples induce oxidative stress in A549 cells. ΔC-SRM samples were more efficient as ROS inducers. They caused ca. twice higher ROS production than SRM samples when cells were treated with a high concentration of PM (>200 μg/cm2). SRM samples started to induce significant oxidative stress in the concentration higher than 50 μg/cm2, while ΔC-SRM samples caused a similar level of ROS production with a concentration as small as 1.6 μg/cm2.
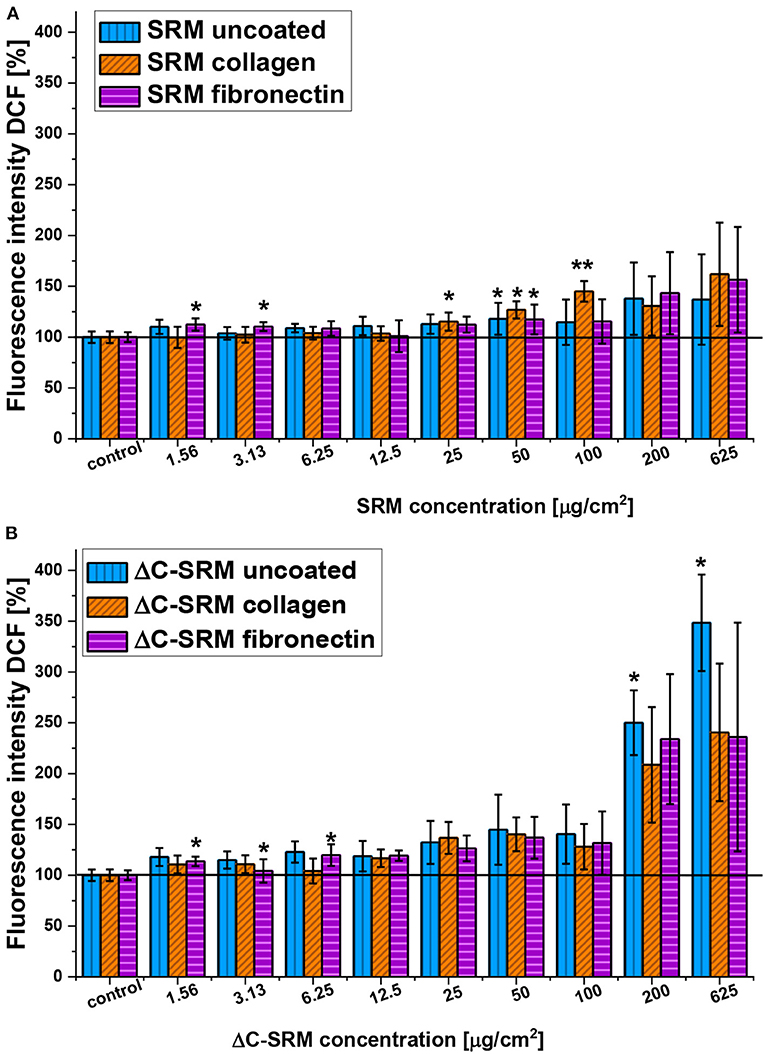
Figure 3. The reactive oxygen species (ROS) production in A549 cells after 24-h incubation with particulate matter (PM) suspensions of different concentrations covering plates either uncoated or coated with fibronectin/collagen measured using 2,7-dichlorodihydrofluorescein diacetate (A, SRM; B, ΔC-SRM) (exposure method 2),*p < 0.05, **p < 0.001.
To thoroughly evaluate the role of inorganic fraction of PM samples in ROS formation in treated cells, the mass reduction of plasma-treated sample was taken into account. During cold plasma treatment, ca. 33% decrease in mass of the sample was observed, so the same mass of ΔC-SRM in comparison to SRM contained much more inorganic particles. To compensate for this effect, the results of DCF-DA evaluation of ROS formation were recalculated, and ROS formation effect of SRM was compared to 67% of ROS generation induced by ΔC-SRM (Supplementary Figure 7). At small and moderate concentrations of PM samples (up to 100 μg/cm2), SRM induced a greater increase in ROS production in A549 cells. However, higher concentrations (>200 μg/cm2) resulted in a similar level of oxidative stress induction by both SRM and ΔC-SRM samples. These results confirmed that at small to moderate concentrations of PM, the organic fraction plays an important role in an oxidative stress formation; however, it is not exclusively responsible for it. At higher concentrations, catalytic activity of inorganic particles became more evident and ΔC-SRM samples induced similar production of ROS as SRM samples.
Qualitative Analysis of the Produced Reactive Oxygen Species
To determine a profile of the produced ROS, the selective fluorescent probes, described in detail in the experimental part, were used. A549 cells were grown on plates covered with PM at concentrations 50 and 100 μg/cm2 to avoid possible interaction of PM samples with fluorescent probes. Incubation of A549 cells on SRM-covered plates resulted in increased fluorescent signals from APF, DhR123, HE (only on fibronectin-coated plate), and MitoSox (only on collagen-coated plate) indicators (Figure 4). This indicated that majority of ROS produced by A549 upon contact with SRM are hydrogen peroxide (H2O2), hydroxyl radical (·OH), and superoxide anion radical (). Plasma-treated air pollutant (ΔC-SRM) sample upon contact with A549 cells additionally to already mentioned ROS induced the production of singlet oxygen, as was manifested by the increased fluorescent signal from the SOSG probe (Figure 4). The lack of singlet oxygen production by cells treated with SRM samples can be a consequence of an antioxidant potential of organic part of PM sample. Singlet oxygen can be quenched by and/or reacts with many organic molecules (DeRosa and Crutchley, 2002).
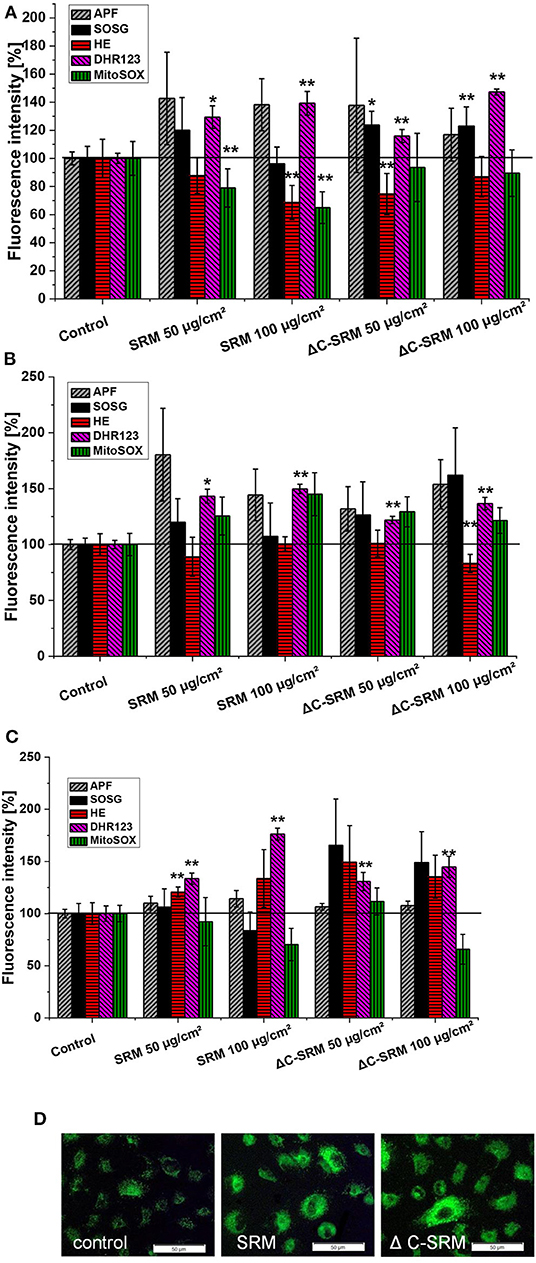
Figure 4. The level of reactive oxygen species (ROS), measured by selective fluorescent probes, induced in A549 cells after their 24-h incubation on particulate matter (PM)-covered plates either uncoated (A) or coated with collagen (B) and fibronectin (C) (exposure method 2). SOSG, Singlet Oxygen Sensor Green (1O2); APF, aminophenyl fluorescein (·OH, ONOO−, HOCl); DhR123, dihydrorhodamine 123 (H2O2, ONOO−, HOCl, cytochrome c); HE, hydroethidine (); MitoSox™ ( in mitochondria). (D) Representative images of ROS production induced in A549 cells on PM-covered plates (100 μg/cm2) measured by DhR123. Scale bar is 50 μm. *p < 0.05, **p < 0.001.
Lipid Peroxidation
Lipid peroxidation was assessed using C11-BODIPY581/591-Lipid Peroxidation Sensor (Figure 5). This liposoluble probe upon oxidation with lipid peroxyl radicals (ROO·) alters its fluorescence properties, shifting emission intensity to shorter wavelengths, from red to green fluorescence. Incubation of A549 cells on the PM-covered surfaces resulted in increased green-to-red fluorescence ratio of C11-BODIPY581/591 probe, displaying the occurrence of lipid peroxidation. The observed effect was similar for SRM and ΔC-SRM samples, which can indicate that the organic part of PM has a negligible effect on lipid peroxidation.
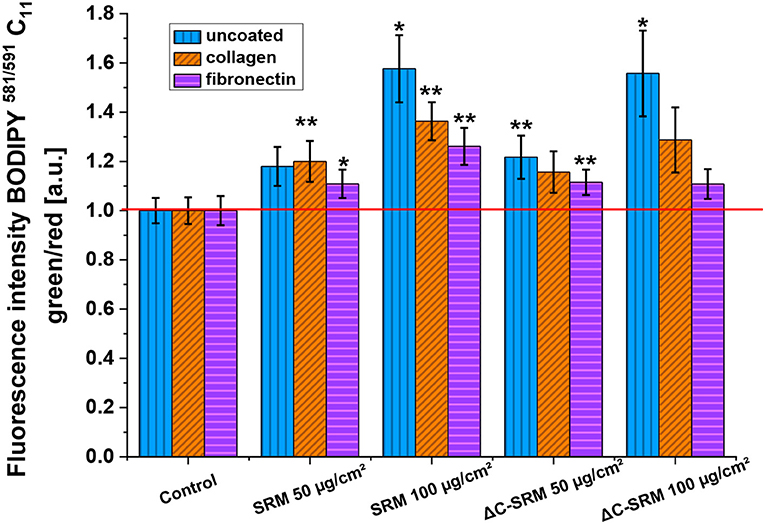
Figure 5. The lipid peroxidation in A549 cells after 24-h incubation with different concentrations of particulate matter (PM)-covered plates either uncoated or coated with fibronectin/collagen measured using C11-BODIPY581/591 probe (exposure method 2), *p < 0.05, **p < 0.01.
Mitochondrial Membrane Potential
To evaluate ΔΨm, JC-1 probe was used (Figure 6). The shift in mitochondrial potential was represented as a change in red/green fluorescence ratio. Both untreated and plasma-treated PM samples caused the decrease in red/green fluorescence intensity ratio, indicating depolarization of the mitochondrial membrane. The effect was concentration dependent and was slightly stronger after exposure cells to SRM samples.
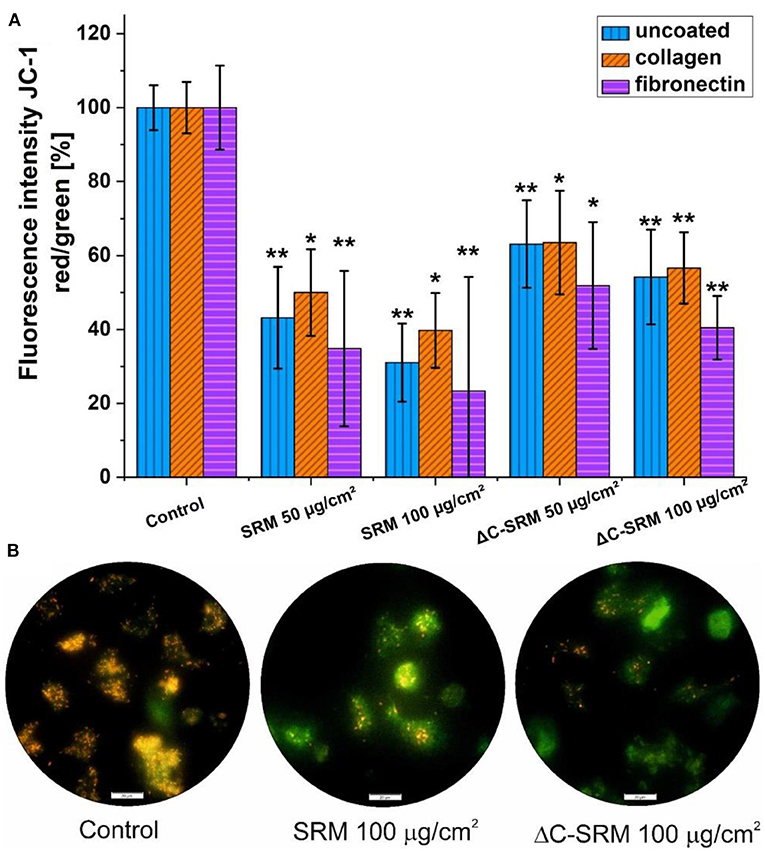
Figure 6. (A) The changes in mitochondrial membrane potential (ΔΨm) in A549 cells after 24-h incubation on particulate matter (PM)-covered plates either uncoated or coated with fibronectin/collagen measured using JC-1 probe (exposure method 2). (B) Representative images of depolarization of ΔΨm of A549 cells induced by PM. Scale bar is 20 μm; *p < 0.05, **p < 0.001.
Intracellular Calcium Concentration
Calcium ion is one of the most important signal transducers in cells, involved in numerous physiological and pathological processes including proliferation, differentiation, and cellular motility. Both calcium spatial localization and magnitude might determine the cell's faith. It is well-known that various metals can modify intracellular Ca2+ signaling (MacNee and Donaldson, 2003). Fluo-8 AM probe was used to determine the changes in intracellular calcium concentration in A549 cells after 24-h incubation on the PM-covered plates uncoated or coated with fibronectin/collagen (Supplementary Figure 8). Incubation of A549 cells on PM samples resulted in the decrease in Fluo-8 AM probe fluorescence, indicating the disruption of calcium homeostasis and the decrease in cytosolic [Ca2+]. The results are more pronounced for cells seeded on uncoated plates than for one coated with adhesion proteins.
Mechanism of Cellular Death
To investigate the mechanism of cellular death, the activity of executioner caspases 3/7 was examined. These enzymes are activated during regulated cellular death (extrinsic or intrinsic apoptosis) and are responsible for many morphological and biochemical changes such as DNA fragmentation, phosphatidylserine exposure, and formation of apoptotic bodies (Galluzzi et al., 2018). The percentage of caspase 3/7-positive cells after 24-h incubation with different concentrations of PM-covered plates is shown in Figure 7A. In A549 cells after exposure to PM samples, the percentage of caspase 3/7-positive cells significantly increased. SRM sample caused activation of executioner caspases in the whole population of the treated cells, while ΔC-SRM sample only in around 30%. The results indicate that apoptosis is a main mechanism of cellular death for SRM-treated cells, while exposure to ΔC-SRM can induce some additional caspase-independent cell death mechanisms.
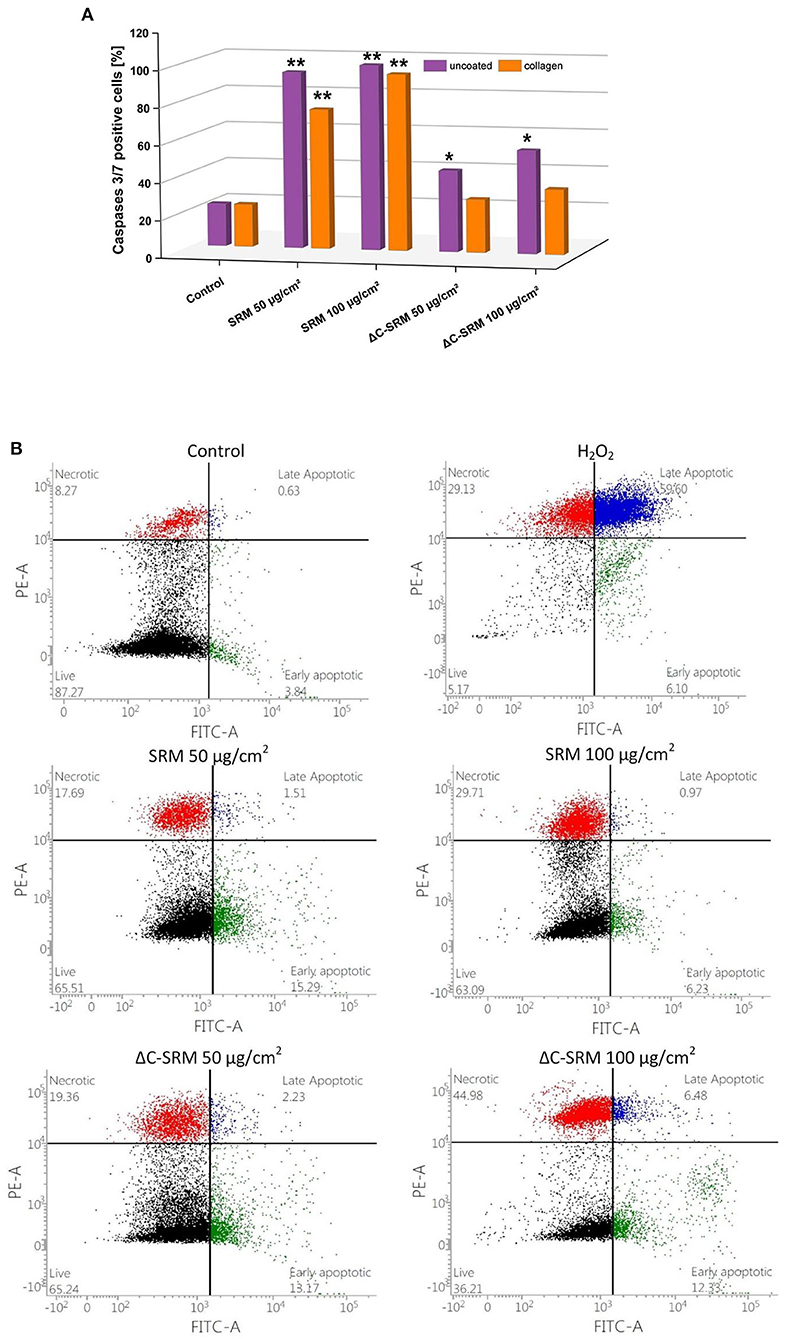
Figure 7. (A) The percentage of caspase 3/7-positive cells after 24-h incubation with different concentrations of particulate matter (PM)-covered plates either uncoated or coated with fibronectin/collagen (exposure method 2). (B) The percentage of living, necrotic, and apoptotic A549 cells after 24-h incubation on PM-covered plates (exposure method 2). *p < 0.05, **p < 0.001.
To further investigate the mechanism of cellular death, morphological signs of apoptosis and necrosis were examined by Annexin V-FITC/PI assay. This assay allows for determination of a potential loss of cellular integrity (during necrosis) and measures transition of phosphatidylserine (PS) on the outer surface of the cell membrane (during apoptosis). The percentage of living, necrotic, and apoptotic A549 cells after 24-h incubation with different concentrations of PM-covered plates is shown in Figure 7B. The presence of SRM and ΔC-SRM samples increased the population of apoptotic and necrotic cells. While some necrotic fraction of the populations can be explained by end-stage apoptosis (Galluzzi et al., 2018), inorganic PM sample, ΔC-SRM, undoubtedly induced a stronger effect on A549 cells than the SRM sample, producing a significantly higher amount of necrotic cells. The results indicate involvement of necrotic cell death as cells' failed responses to adapt to induced stress. Similar results were obtained for collagen-coated surfaces (results are not shown).
Critical Discussion of The Results
Several studies tried to estimate the relative contribution of inorganic and organic components to PM-induced toxicity (Gao et al., 2020). Some studies try to do this by multiplying the intrinsic oxidative potential of individual compounds of PM with their ambient concentrations and provide evidence of the importance of either transition metals (Charrier and Anastasio, 2012) or organic components (Verma et al., 2015). Others compared atmospheric particles with different amounts of organic constituents such as PM2.5, carbon black particles, and diesel exhaust particles (DEPs). Baulig et al. concluded that organic compounds presented in DEP (Baulig et al., 2003) or isolated from PM2.5 (Baulig et al., 2009) mainly contributed to the observed biological effects in human bronchial epithelial cells. Our approach relies on the evaluation of inorganic fraction contribution on biological response to airborne PM by removing organic compounds using cold plasma treatment. Such treatment does not induce changes in particle morphology or size; however, we are not able to gain detailed information on the speciation of the PM components.
Due to the cumulative nature of pollution, adverse health effects are often results of chronic exposure to harmful particles, so when cells are exposed to urban airborne PM at quantities expected from the known content of PM in air, the observed changes are at a very low level or negligibly small. Large quantities of PM that have often been used to detect changes in various biological parameters in vitro can induce additional mechanical damage, which will falsify the obtained results due to the ability of a dying cell to expose or release molecules that alert other cells. In these studies, special emphasis was placed on the elimination of false-positive results arising from mechanical pressure of PM on cell culture. This was achieved through an inverting cell exposure protocol by covering cell culture plates with PM. Such alternative way of cell treatment allowed us to increase 20 times the used PM concentration without causing unnecessary cell death from the mechanical damage.
Another serious issue in this type of research concerns the oxidation of the fluorescent probes by using PM due to the oxidative potential of both organic and inorganic components of airborne air pollution. To eliminate such a possibility, several control experiments were carried out and carefully analyzed to avoid artificial positive results. The combination of these factors allowed for the selection of an appropriate cell treatment protocol and PM concentrations that make it a possibility to study narrow differences between the tested PM samples.
We are aware that such a model system also has some limitations. One of the most relevant is the inability to assess synergistic and antagonistic interactions between different PM components that could significantly alter their redox properties. This method does not consider the cumulative effect of pollution and can underestimate the influence of fraction due to its low atmospheric concentration. Additionally, bulk PM mass measurements do not accurately represent a complex chemical mixture that is air pollution, which additionally varies both spatially and temporally. In vitro studies using lung epithelial cells allowed assessing the effects of dissolved components and components of the studied PM with a size smaller than 2.5 μm. Only such particles are capable of reaching the alveolar epithelium, crossing cell membranes, and directly interacting with cellular structures. SRM predominantly contains particles from 5 to 20 μm, which can lead to underestimation of the observed effects. The negative effects of air pollution on human health is difficult to imitate under laboratory conditions; however, by using thoroughly characterized Standard Reference Materials (available from the National Institute of Standard and Technology), the effect of airborne PM can be studied among different laboratories worldwide.
Conclusions
Air pollution is now recognized as one of the main health risks in the developed world. The presented work is focused on the comparison of biological effects in vitro for the organic and inorganic fractions of PM. To achieve the goal, the influence of intact standardized urban PM (SRM) and plasma-treated SRM containing predominantly inorganic fraction of PM (ΔC-SRM) on various biological parameters of A549 lung epithelial cells was directly compared following the newly elaborated experimental protocols.
The obtained results indicate a significant influence of the inorganic part of PM on oxidative stress generation. Both organic and inorganic fractions of PM are involved in the induction of ROS production by cells. In the case of small and moderate concentrations of PM and shorter incubation time (24 h), the organic fraction of PM plays a pivotal role in oxidative stress induction. The significance of the inorganic fraction is enhanced with increasing PM concentrations and prolonging incubation time (up to 18 days), mimicking in this way chronic exposure. Covering plates with PM allowed the use of higher concentrations of air pollutants in the studied suspensions without observing the toxic effect of the particles. At high concentrations, treatment with ΔC-SRM demonstrated a similar effect on ROS production as SRM, and the catalytic activity of inorganic particles became more apparent.
Both air pollutant samples decreased the viability of A549 cells by decreasing the ΔΨm and disrupting calcium homeostasis. Despite the SRM sample being slightly more toxic toward A549 cells, the inorganic fraction (ΔC-SRM) appeared to be more dangerous due to its superior ability to induce necrosis in the studied cell line. The biological alterations observed in vitro might contribute to lung inflammation and increased probability of pulmonary diseases.
Detected changes in the observed biological effects of SRM and ΔC-SRM might be related to differences in the ROS profile induced in cells. Singlet oxygen production was observed only after treatment of cells with ΔC-SRM. Hydrogen peroxide, hydroxyl radical, and superoxide anion radical are the most abundant ROS produced after exposure of A549 cells to SRM, while ΔC-SRM preferably induced the production of H2O2, singlet oxygen, and hydroxyl radical.
The obtained findings are of importance for our future understanding how different organic and inorganic components of PM contribute to the adverse health effects of PM and could help to develop a strategy to identify the most harmful components. The proposed novel research protocol is crucial for accurate evaluation of the biological effects of PM in vitro without cell exposure to additional stressful factors such as physical disturbance.
Data Availability Statement
The raw data supporting the conclusions of this article will be made available by the authors, without undue reservation.
Author Contributions
All authors listed have made substantial, direct and intellectual contribution to the work, and approved it for publication.
Funding
The authors would like to thank SYMPHONY project (Grant No. 2015/16/W/ST5/00005) for the financial support.
Conflict of Interest
The authors declare that the research was conducted in the absence of any commercial or financial relationships that could be construed as a potential conflict of interest.
Acknowledgments
The authors are grateful to Magdalena Mikrut for preparation of the ΔC-SRM sample.
Supplementary Material
The Supplementary Material for this article can be found online at: https://www.frontiersin.org/articles/10.3389/fchem.2020.581752/full#supplementary-material
References
Ahlberg, S., Rancan, F., Epple, M., Loza, K., Höppe, D., Lademann, J., et al. (2016). Comparison of different methods to study effects of silver nanoparticles on the pro- and antioxidant status of human keratinocytes and fibroblasts. Methods 109, 55–63. doi: 10.1016/j.ymeth.2016.05.015
Ali, M. U., Liu, G., Yousaf, B., Ullah, H., Abbas, Q., and Munir, M. A. M. (2019). A systematic review on global pollution status of particulate matter-associated potential toxic elements and health perspectives in urban environment. Environ. Geochem. Health 41, 1131–1162. doi: 10.1007/s10653-018-0203-z
Andersson, H., Piras, E., Demma, J., Hellman, B., and Brittebo, E. (2009). Low levels of the air pollutant 1-nitropyrene induce DNA damage, increased levels of reactive oxygen species and endoplasmic reticulum stress in human endothelial cells. Toxicology 262, 57–64. doi: 10.1016/j.tox.2009.05.008
Ando, T., and Yonamoto, Y. (2015). In situ EPR detection of reactive oxygen species in adherent cells using polylysine-coated glass plate. Appl. Magn. Reson. 46, 977–986. doi: 10.1007/s00723-015-0688-x
Araujo, J. A. (2011). Particulate air pollution, systemic oxidative stress, inflammation, and atherosclerosis. Air Qual. Atmos. Health 4, 79–93. doi: 10.1007/s11869-010-0101-8
Badran, G., Ledoux, F., Verdin, A., Abbas, I., Roumie, M., Genevray, P., et al. (2020). Toxicity of fine and quasi-ultrafine particles: focus on the effects of organic extractable and non-extractable matter fractions. Chemosphere 243:125440. doi: 10.1016/j.chemosphere.2019.125440
Baulig, A., Poirault, J. J., Ausset, P., Schins, R., Shi, T., Baralle, D., et al. (2004). Physicochemical characteristics and biological activities of seasonal atmospheric particulate matter sampling in two locations of Paris. Environ. Sci. Technol. 38, 5985–5992. doi: 10.1021/es049476z
Baulig, A., Singh, S., Marchand, A., Schins, R., Barouki, R., Garlatti, M., et al. (2009). Role of Paris PM2.5 components in the pro-inflammatory response induced in airway epithelial cells. Toxicology 261, 126–135. doi: 10.1016/j.tox.2009.05.007
Baulig, A., Sourdeval, M., Meyer, M., Marano, F., and Baeza-Squiban, A. (2003). Biological effects of atmospheric particles on human bronchial epithelial cells. comparison with diesel exhaust particles. Toxicol. in vitro 17, 567–573. doi: 10.1016/S0887-2333(03)00115-2
Billet, S., Garçon, G., Dagher, Z., Verdin, A., Ledoux, F., Cazier, F., et al. (2007). Ambient particulate matter (PM2.5): physicochemical characterization and metabolic activation of the organic fraction in human lung epithelial cells (A549). Environ. Res. 105, 212–223. doi: 10.1016/j.envres.2007.03.001
Bräuner, E. V., Forchhammer, L., Möller, P., Simonsen, J., Glasius, M., Wåhlin, P., et al. (2007). Exposure to ultrafine particles from ambient air and oxidative stress-induced DNA damage. Environ. Health Perspect. 115, 1177–1182. doi: 10.1289/ehp.9984
Charrier, J. G., and Anastasio, C. (2012). On dithiothreitol (DTT) as a measure of oxidative potential for ambient particles: evidence for the importance of soluble /newline transition metals. Atmosph. Chem. Phys. 12, 9321–9333. doi: 10.5194/acp-12-9321-2012
Chew, S., Kolosowska, N., Saveleva, L., Malm, T., and Kanninen, K. M. (2020). Impairment of mitochondrial function by particulate matter: Implications for the brain. Neurochem. Int. 135:104694. doi: 10.1016/j.neuint.2020.104694
Courtois, A., Prouillac, C., Baudrimont, I., Ohayon-Courtes, C., Freund-Michel, V., Dubois, M., et al. (2014). Characterization of the components of urban particulate matter mediating impairment of nitric oxide-dependent relaxation in intrapulmonary arteries. J. Appl. Toxicol. 34, 667–674. doi: 10.1002/jat.2909
Cui, X., Zhou, T., Shen, Y., Rong, Y., Zhang, Z., Liu, Y., et al. (2019). Different biological effects of PM 2.5 from coal combustion, gasoline exhaust and urban ambient air relate to the PAH/metal compositions. Environ. Toxicol. Pharmacol. 69, 120–128. doi: 10.1016/j.etap.2019.04.006
DeRosa, M. C., and Crutchley, R. J. (2002). Photosensitized singlet oxygen and its applications. Coord. Chem. Rev. 233-234, 351–371. doi: 10.1016/S0010-8545(02)00034-6
Dijkhoff, I. M., Drasler, B., Karakocak, B. B., Petri-Fink, A., Valacchi, G., Eeman, M., et al. (2020). Impact of airborne particulate matter on skin: a systematic review from epidemiology to in vitro studies. Part. Fibre Toxicol. 17:35. doi: 10.1186/s12989-020-00366-y
Falco, G. D., Colarusso, C., Terlizzi, M., Popolo, A., Pecoraro, M., Commodo, M., et al. (2017). Chronic obstructive pulmonary disease-derived circulating cells release IL-18 and IL-33 under ultrafine particulate matter exposure in a caspase-1/8-independent manner. Front. Immunol. 8:1415. doi: 10.3389/fimmu.2017.01415
Galluzzi, L., Vitale, I., Aaronson, S. A., Abrams, J. M., Adam, D., Agostinis, P., et al. (2018). Molecular mechanisms of cell death: recommendations of the nomenclature committee on cell death 2018. Cell Death Differ. 25, 486–541. doi: 10.1038/s41418-017-0012-4
Gangwar, R. S., Bevan, G. H., Palanivel, R., Das, L., and Rajagopalan, S. (2020). Oxidative stress pathways of air pollution mediated toxicity: recent insights. Redox Biol. 34:101545. doi: 10.1016/j.redox.2020.101545
Gao, D., Ripley, S., Weichenthal, S., and Godri Pollitt, K. J. (2020). Ambient particulate matter oxidative potential: chemical determinants, associated health effects, and strategies for risk management. Free Radic. Biol. Med. 151, 7–25. doi: 10.1016/j.freeradbiomed.2020.04.028
Gawda, A., Majka, G., Nowak, B., Sróttek, M., Walczewska, M., and Marcinkiewicz, J. (2018). Air particulate matter SRM 1648a primes macrophages to hyperinflammatory response after LPS stimulation. Inflamm. Res. 67, 765–776. doi: 10.1007/s00011-018-1165-4
Haghani, A., Johnson, R., Safi, N., Zhang, H., Thorwald, M., Mousavi, A., et al. (2020). Toxicity of urban air pollution particulate matter in developing and adult mouse brain: comparison of total and filter-eluted nanoparticles. Environ. Internat. 136:105510. doi: 10.1016/j.envint.2020.105510
Han, C., Oh, J., Lim, Y. H., Kim, S., and Hong, Y. C. (2020). Long-term exposure to fine particulate matter and development of chronic obstructive pulmonary disease in the elderly. Environ. Int. 143:105895. doi: 10.1016/j.envint.2020.105895
Hanzalova, K., Rossner, P. Jr, and Sram, R. J. (2010). Oxidative damage induced by carcinogenic polycyclic aromatic hydrocarbons and organic extracts from urban air particulate matter. Mutat. Res. Genet.Toxicol. Environ. Mutagen. 696, 114–121. doi: 10.1016/j.mrgentox.2009.12.018
Herseth, J. I., Volden, V., and Bolling, A. K. (2017). Particulate matter-mediated release of long pentraxin 3 (PTX3) and vascular endothelial growth factor (VEGF) in vitro: limited importance of endotoxin and organic content. J. Toxicol. Env. Heal. A 80, 105–119. doi: 10.1080/15287394.2016.1257399
Holian, A., Hamilton, R. F. Jr, Morandi, M. T., Brown, S. D., and Li, L. (1998). Urban particle-induced apoptosis and phenotype shifts in human alveolar macrophages. Environ. Health Perspect. 106, 127–132. doi: 10.1289/ehp.98106127
Huang, M., Kang, Y., Wang, W., Chan, C. Y., Wang, X., and Wong, M. H. (2015). Potential cytotoxicity of water-soluble fraction of dust and particulate matters and relation to metal(loid)s based on three human cell lines. Chemosphere 135, 61–66. doi: 10.1016/j.chemosphere.2015.04.004
Huang, Q., Zhang, J., Peng, S., Tian, M., Chen, J., and Shen, H. (2014). Effects of water soluble PM2.5 extracts exposure on human lung epithelial cells (A549): a proteomic study. J. Appl. Toxicol. 34, 675–687. doi: 10.1002/jat.2910
Jiang, M., Nakamatsu, Y., Jensen, K. A., and Utsunomiya, S. (2014). Multi-scale analysis of the occurrence of Pb, Cr and Mn in the NIST standards: urban dust (SRM 1649a) and indoor dust (SRM 2584). Atmos. Environ. 82, 364–374. doi: 10.1016/j.atmosenv.2013.10.035
Kasprzak, K. S. (2002). Oxidative DNA and protein damage in metal-induced toxicity and carcinogenesis. Free Radic. Biol. Med. 32, 958–967. doi: 10.1016/S0891-5849(02)00809-2
Kawanishi, S., Hiraku, Y., Murata, M., and Oikawa, S. (2002). The role of metals in site-specific DNA damage with reference to carcinogenesis. Free Radic. Biol. Med. 32, 822–832. doi: 10.1016/S0891-5849(02)00779-7
Kelly, F. J., and Fussell, J. C. (2017). Role of oxidative stress in cardiovascular disease outcomes following exposure to ambient air pollution. Free Radic. Biol. Med. 110, 345–367. doi: 10.1016/j.freeradbiomed.2017.06.019
Kim, W., Jeong, S. C., Shin, C. Y., Song, M. K., Cho, Y., Lim, J. H., et al. (2018). A study of cytotoxicity and genotoxicity of particulate matter (PM2.5) in human lung epithelial cells (A549). Mol. Cell. Toxicol. 14, 163–172. doi: 10.1007/s13273-018-0018-0
Lee, C. W., Lin, Z. C., Hu, S. C. S., Chiang, Y. C., Hsu, L. F., Lin, Y. C., et al. (2016). Urban particulate matter down-regulates filaggrin via COX2 expression/PGE2 production leading to skin barrier dysfunction. Sci. Rep. 6:27995. doi: 10.1038/srep27995
Lee, K. Y., Wong, C. K. C., Chuang, K. J., Bien, M. Y., Cao, J. J., Han, Y. M., et al. (2014). Methionine oxidation in albumin by fine haze particulate matter: an in vitro and in vivo study. J. Hazard. Mater. 274, 384–391. doi: 10.1016/j.jhazmat.2014.04.029
Leikauf, G. D., Kim, S. H., and Jang, A. S. (2020). Mechanisms of ultrafine particle-induced respiratory health effects. Exp. Mel. Med. 52, 329–337. doi: 10.1038/s12276-020-0394-0
Li, R., Wang, Y., Qiu, X., Xu, F., Chen, R., Gu, W., et al. (2020). Difference on oxidative stress in lung epithelial cells and macrophages induced by ambient fine particulate matter (PM2.5). Air Qual. Atmos. Health 13, 789–796. doi: 10.1007/s11869-020-00835-5
Liu, H. Y., Dunea, D., Iordache, S., and Pohoata, A. (2018). A review of airborne particulate matter effects on young children's respiratory symptoms and diseases. Atmosphere 9:150. doi: 10.3390/atmos9040150
Longhin, E. M., Mantecca, P., and Gualtieri, M. (2020). Fifteen years of airborne particulates in vitro toxicology in milano: lessons and perspectives learned. Int. J. Mol. Sci. 21:2489. doi: 10.3390/ijms21072489
Lu, Y., Su, S., Jin, W., Wang, B., Li, N., Shen, H., et al. (2014). Characteristics and cellular effects of ambient particulate matter from Beijing. Environ. Pollut. 191, 63–69. doi: 10.1016/j.envpol.2014.04.008
MacNee, W., and Donaldson, K. (2003). Mechanism of lung injury caused by PM10 and ultrafine particles with special reference to COPD. Eur. Respir. J. 40, 47S−51S. doi: 10.1183/09031936.03.00403203
Manigrasso, M., Costabile, F., Liberto, L. D., Gobbi, G. P., Gualtieri, M., Zanini, G., et al. (2020). Size resolved aerosol respiratory doses in a Mediterranean urban area: from PM10 to ultrafine particles. Environ. Int. 141:105714. doi: 10.1016/j.envint.2020.105714
Mazuryk, O., Magiera, K., Rys, B., Suzenet, F., Kieda, C., and Brindell, M. (2014). Multifaceted interplay between lipophilicity, protein interaction and luminescence parameters of non-intercalative ruthenium(II) polypyridyl complexes controlling cellular imaging and cytotoxic properties. J. Biol. Inorg. Chem. 19, 1305–1316. doi: 10.1007/s00775-014-1187-5
Mehta, M., Chen, L. C., Gordon, T., Rom, W., and Tang, M.s. (2008). Particulate matter inhibits DNA repair and enhances mutagenesis. Mutat. Res. Genet. Toxicol. Environ. Mutagen. 657, 116–121. doi: 10.1016/j.mrgentox.2008.08.015
Mikrut, M., Regiel-Futyra, A., Samek, L., Macyk, W., Stochel, G., and van Eldik, R. (2018). Generation of hydroxyl radicals and singlet oxygen by particulate matter and its inorganic components. Environ. Pollut. 238, 638–646. doi: 10.1016/j.envpol.2018.03.068
Novák, J., Vaculovič, A., Klánová, J., Giesy, J. P., and Hilscherová, K. (2020). Seasonal variation of endocrine disrupting potentials of pollutant mixtures associated with various size-fractions of inhalable air particulate matter. Environ. Pollut. 264:114654. doi: 10.1016/j.envpol.2020.114654
O'Driscoll, C. A., Owens, L. A., Hoffmann, E. J., Gallo, M. E., Afrazi, A., Han, M., et al. (2019). Ambient urban dust particulate matter reduces pathologic T cells in the CNS and severity of EAE. Environ. Res. 168, 178–192. doi: 10.1016/j.envres.2018.09.038
Oh, S. M., Kim, H. R., Park, Y. J., Lee, S. Y., and Chung, K. H. (2011). Organic extracts of urban air pollution particulate matter (PM2.5)-induced genotoxicity and oxidative stress in human lung bronchial epithelial cells (BEAS-2B cells). Mutat. Res. Genet. Toxicol. Environ. Mutagen. 723, 142–151. doi: 10.1016/j.mrgentox.2011.04.003
Pardo, M., Qiu, X., Zimmermann, R., and Rudich, Y. (2020). Particulate matter toxicity is nrf2 and mitochondria dependent: The roles of metals and polycyclic aromatic hydrocarbons. Chem. Res. Toxicol. 33, 1110–1120. doi: 10.1021/acs.chemrestox.0c00007
Park, Y. J., Lim, L., and Song, H. (2013). Distinct oxidative damage of biomolecules by arrays of metals mobilized from different types of airborne particulate matters; SRM1648, fine (PM2.5), and coarse (PM10) fractions. Environ. Eng. Res. 18, 139–143. doi: 10.4491/eer.2013.18.3.139
Qi, Z., Zhang, Y., Chen, Z. F., Yang, C., Song, Y., Liao, X., et al. (2020). Chemical identity and cardiovascular toxicity of hydrophobic organic components in PM2.5. Ecotoxicol. Environ. Saf. 201:110827. doi: 10.1016/j.ecoenv.2020.110827
Risom, L., Møller, P., and Loft, S. (2005). Oxidative stress-induced DNA damage by particulate air pollution. Mutat. Res. Fund. Mol. Mech. Mut. 592, 119–137. doi: 10.1016/j.mrfmmm.2005.06.012
Rodríguez-Cotto, R. I., Ortiz-Martínez, M. G., and Jiménez-Vélez, B. D. (2015). Organic extracts from African dust storms stimulate oxidative stress and induce inflammatory responses in human lung cells through Nrf2 but not NF-κB. Environ. Toxicol. Pharmacol. 39, 845–856. doi: 10.1016/j.etap.2015.02.015
Samet, J. M., Chen, H., Pennington, E. R., and Bromberg, P. A. (2020). Non-redox cycling mechanisms of oxidative stress induced by PM metals. Free Rad. Biol. Med. 151, 26–37. doi: 10.1016/j.freeradbiomed.2019.12.027
Schilirò, T., Bonetta, S., Alessandria, L., Gianotti, V., Carraro, E., and Gilli, G. (2015). PM10 in a background urban site: chemical characteristics and biological effects. Environ. Toxicol. Pharmacol. 39, 833–844. doi: 10.1016/j.etap.2015.02.008
Selmi, C., Leung, P. S. C., Sherr, D. H., Diaz, M., Nyland, J. F., Monestier, M., et al. (2012). Mechanisms of environmental influence on human autoimmunity: a national institute of environmental health sciences expert panel workshop. J. Autoimmun. 39, 272–284. doi: 10.1016/j.jaut.2012.05.007
Shao, P., Tian, H., Sun, Y., Liu, H., Wu, B., Liu, S., et al. (2018). Characterizing remarkable changes of severe haze events and chemical compositions in multi-size airborne particles (PM1, PM2.5 and PM10) from January 2013 to 2016–2017 winter in Beijing, China. Atmosph. Environ. 189, 133–144. doi: 10.1016/j.atmosenv.2018.06.038
Shen, Y. S., and Lung, S. C. C. (2020). Multiple impacts and pathways of urban form and environmental factors on cardiovascular mortality. Sci. Total Environ. 738:139512. doi: 10.1016/j.scitotenv.2020.139512
Stone, V., Wilson, M. R., Lightbody, J., and Donaldson, K. (2003). Investigating the potential for interaction between the components of PM(10). Environ. Health Prev. Med. 7, 246–253. doi: 10.1007/BF02908883
Valacchi, G., Magnani, N., Woodby, B., Ferreira, S. M., and Evelson, P. (2020). Particulate matter induces tissue oxinflammation: from mechanism to damage. Antioxid. Redox Signal. 33, 308–326. doi: 10.1089/ars.2019.8015
Verma, V., Fang, T., Xu, L., Peltier, R. E., Russell, A. G., Ng, N. L., et al. (2015). Organic aerosols associated with the generation of reactive oxygen species (ROS) by water-soluble PM2.5. Environ. Sci. Technol. 49, 4646–4656. doi: 10.1021/es505577w
Visalli, G., Baluce, B., Bertuccio, M., Picerno, I., and Di Pietro, A. (2015). Mitochondrial-mediated apoptosis pathway in alveolar epithelial cells exposed to the metals in combustion-generated particulate matter. J. Toxicol. Environ. Health Part A 78, 697–709. doi: 10.1080/15287394.2015.1024081
Wang, B., Li, K., Jin, W., Lu, Y., Zhang, Y., Shen, G., et al. (2013). Properties and inflammatory effects of various size fractions of ambient particulate matter from Beijing on A549 and J774A.1 cells. Environ. Sci. Technol. 47, 10583–10590. doi: 10.1021/es401394g
Wang, H., Shen, X., Tian, G., Shi, X., Huang, W., Wu, Y., et al. (2018). AMPKα2 deficiency exacerbates long-term PM 2.5 exposure-induced lung injury and cardiac dysfunction. Free Rad. Biol. Med. 121, 202–214. doi: 10.1016/j.freeradbiomed.2018.05.008
Wang, N., Mengersen, K., Tong, S., Kimlin, M., Zhou, M., Liu, Y., et al. (2020a). County-level variation in the long-term association between PM2.5 and lung cancer mortality in China. Sci. Total Environ. 738:140195. doi: 10.1016/j.scitotenv.2020.140195
Wang, W., Ding, X., Turap, Y., Tursun, Y., Abulizi, A., Wang, X., et al. (2020b). Distribution, sources, risks, and vitro DNA oxidative damage of PM2.5-bound atmospheric polycyclic aromatic hydrocarbons in Urumqi, NW China. Sci. Total Environ. 739:139518. doi: 10.1016/j.scitotenv.2020.139518
Wilson, M. R., Lightbody, J. H., Donaldson, K., Sales, J., and Stone, V. (2002). Interactions between ultrafine particles and transition metals in vivo and in vitro. Toxicol. Appl. Pharmacol. 184, 172–179. doi: 10.1006/taap.2002.9501
Winter, S. V., and Zychlinsky, A. (2018). The bacterial pigment pyocyanin inhibits the NLRP3 inflammasome through intracellular reactive oxygen and nitrogen species. J. Biol. Chem. 293, 4893–4900. doi: 10.1074/jbc.RA117.001105
World Health Organization [Online]. Available online at: http://www.who.int (accessed January 06 2020).
Yang, L., Li, C., and Tang, X. (2020). The impact of PM2.5 on the host defense of respiratory system. Front. Cell Dev. Biol. 8:91. doi: 10.3389/fcell.2020.00091
Keywords: air pollution, particulate matter PM, reactive oxygen species, inorganic fraction of PM, treatment protocol
Citation: Mazuryk O, Stochel G and Brindell M (2020) Variations in Reactive Oxygen Species Generation by Urban Airborne Particulate Matter in Lung Epithelial Cells—Impact of Inorganic Fraction. Front. Chem. 8:581752. doi: 10.3389/fchem.2020.581752
Received: 09 July 2020; Accepted: 03 November 2020;
Published: 17 December 2020.
Edited by:
Loredana Serpe, University of Turin, ItalyReviewed by:
Ankush Prasad, Palacký University, Olomouc, CzechiaAngela Di Pietro, University of Messina, Italy
Copyright © 2020 Mazuryk, Stochel and Brindell. This is an open-access article distributed under the terms of the Creative Commons Attribution License (CC BY). The use, distribution or reproduction in other forums is permitted, provided the original author(s) and the copyright owner(s) are credited and that the original publication in this journal is cited, in accordance with accepted academic practice. No use, distribution or reproduction is permitted which does not comply with these terms.
*Correspondence: Olga Mazuryk, bWF6dXJ5a0BjaGVtaWEudWouZWR1LnBs